- 1Laboratory of Vision Neurobiology, Nencki Institute of Experimental Biology, Polish Academy of Sciences, Warsaw, Poland
- 2Ophtalmic Biology Group, International Centre for Translational Eye Research, Institute of Physical Chemistry, Polish Academy of Sciences, Warsaw, Poland
Repetitive visual stimulation is successfully used in a study on the visual evoked potential (VEP) plasticity in the visual system in mammals. Practicing visual tasks or repeated exposure to sensory stimuli can induce neuronal network changes in the cortical circuits and improve the perception of these stimuli. However, little is known about the effect of visual training at the subcortical level. In the present study, we extend the knowledge showing positive results of this training in the rat’s Superior colliculus (SC). In electrophysiological experiments, we showed that a single training session lasting several hours induces a response enhancement both in the primary visual cortex (V1) and in the SC. Further, we tested if collicular responses will be enhanced without V1 input. For this reason, we inactivated the V1 by applying xylocaine solution onto the cortical surface during visual training. Our results revealed that SC’s response enhancement was present even without V1 inputs and showed no difference in amplitude comparing to VEPs enhancement while the V1 was active. These data suggest that the visual system plasticity and facilitation can develop independently but simultaneously in different parts of the visual system.
Introduction
Repetitive visual training is a rapidly developing tool to modulate neuronal plasticity in the visual system for both research and clinical application (Sabel, 2008). Appropriate visual training protocols can strengthen residual vision in patients with visual impairments such as glaucoma (Sabel and Gudlin, 2014), optic nerve neuropathy (Mueller et al., 2007), or hemianopia (Gall et al., 2008; Poggel et al., 2008; Sabel, 2008).
Simultaneously repetitive visual training is successfully used in studies on the visually evoked potential (VEP) plasticity in the visual system of mammals. Repeated exposure to sensory stimuli can induce neuronal plasticity and leads to an enhanced visual response of these stimuli. Numerous researches have shown that the VEP enhancement might reflect synaptic plasticity (Heynen and Bear, 2001; Sawtell et al., 2003; Teyler et al., 2005; Frenkel et al., 2006; Ross et al., 2008; Cooke and Bear, 2010, 2012). A few days long, repeated presentation of gratings with a single orientation resulted in a potentiation of the cortical VEPs amplitude to the presented stimuli (Frenkel et al., 2006). The mechanism underlying this form of training-dependent plasticity is known as long-term potentiation (LTP) of the cortical response (Frenkel et al., 2006; Kuo and Dringenberg, 2009; Hager and Dringenberg, 2010). There are well-described types of rapid VEP plasticity evoked by a few minutes of “photic tetanus” stimulation (Clapp et al., 2006a). The aforementioned repetitive stimulation results in positive changes in the visual system, for example, an expansion of neuronal receptive fields into unresponsive regions of the visual field (Eysel et al., 1998). The enhancement of the cortical response is an effect of sensory LTP dependent on NMDA-receptors in animals (Clapp et al., 2006a) and humans (Teyler et al., 2005; Clapp et al., 2006b; Ross et al., 2008). Studies on humans showed that repeated exposure to a checkerboard reversal stimulation leads to an increase of cortical VEP amplitude (Teyler et al., 2005; Normann et al., 2007; Elvsshagen et al., 2012).
Little is known about the effect of visual training at the subcortical level. A study carried out on the rat’s dorsal lateral geniculate nucleus (dLGN), the primary recipient of visual information, suggests that the response properties of thalamic neurons are subject to experience-dependent long-term plasticity (Jaepel et al., 2017; Sommeijer et al., 2017). Similarly, in the superior colliculus (SC), the second major target of retinal input, repetitive exposure to dimming stimuli effectively induced the LTP of developing retinotectal synapses in Xenopus tadpole (Zhang et al., 2000). These reports suggest that appropriate sensory stimulation may induce plasticity also at the subcortical level. To test this hypothesis, we used 3 h of repetitive visual training to induce and determine the VEP plasticity in the primary visual cortex (V1) and the SC. Our study showed that visual training evokes enhancement of visual responses both at the cortical and subcortical levels. Further, we revealed that repetitive visual training evokes response enhancement in the SC even if cortical input is turned off by xylocaine.
Materials and Methods
Subjects
We used 14 adults (200–250 g) male Wistar rats obtained from the Mossakowski Medical Research Centre. All animals were housed in the Animal House of the Nencki Institute of Experimental Biology and maintained on a 12 h light/dark cycle (light on 7:00 am). All experimental procedures were conducted in accordance with the ARVO Statement for the Use of Animals in Ophthalmic and Vision Research and the EC Directive 86/609/EEC for animal experiments using protocols and methods accepted by the First Warsaw Local Ethical Commission for Animal Experimentation (521/2018).
Surgical Procedures
Rats were deeply anesthetized with urethane (1.5 g/kg, Sigma-Aldrich, Germany, administered intraperitoneally) and placed in a stereotaxic apparatus. Additional doses of urethane (0.15 g/kg) were administered when necessary. Body temperature was maintained between 36 and 38°C using a heating blanket (Harvard Apparatus, MA, United States). Every hour fluid requirements were fulfilled by subcutaneous injection of 0.9% NaCl. The skin on the head was disinfected with iodine, and local anesthetic (1% lidocaine hydrochloride; Polfa Warszawa S.A, Poland) was injected. The craniotomy was done above the binocular V1 [6.5–7 mm posterior to Bregma; 4.5 mm lateral; (Paxinos and Watson, 2007)] and the SC contralateral to the stimulated eye [7.0 mm posterior to Bregma; 1.5 mm lateral; (Paxinos and Watson, 2007)]. During recording, the right eye (not stimulated) was covered with black tape. The Vidisic gel (Polfa Warszawa S.A, Poland) was applied to prevent the cornea from drying.
Local Field Potential Recordings and Visual Stimulation
Local field potentials (LFPs) were collected using linear electrodes made of 25 μm tungsten microwire in HML (Heavy Polyimide, 0.1–0.3 mΩ) insulation (California Fine Wire, United States). The ground-reference electrode (Ag/AgCl wire) was located in the neck muscles. The cortical electrode (eight channels) was made with an inter-channel distance ranging from 100 to 300 μm and inserted 1.8 mm below the dura, passing through all cortical layers (supragranular, granular, and infragranular). The SC electrode was located in the upper layers; stratum griseum superficiale—SGS, stratum opticum—SO. The SC electrode consisted of seven wires with a ∼ 200 μm vertical recording site arrangement and inserted 4 mm (tip) below the cortical surface. After the initial insertion, the electrode was let to stabilize for about 60 min. After that, we presented several flashes of light to obtain the VEP cortical profile and compare response shapes in all channels. The same procedure was repeated in every animal. The cortical profile was adjusted to see the most similar shapes of the responses on the same levels in each experiment. Signals were recorded with a multichannel data acquisition system (USB-ME64-System, Multichannel Systems, Germany), amplified 100 times (USB-ME-PGA, Multichannel Systems, Germany), filtered at 0.1–100 Hz, digitized (1 kHz sampling rate) and stored on the computer for offline analysis. Multichannel recordings allow us to choose the channels that corresponded to a specific layer, which was similar in all animals. The multi-unit spiking activity was obtained by high pass filtering using Butterworth filter with 500 Hz cutoff frequency of raw signal recorded at a 20 kHz sampling rate. The multi-unit activity was extracted from the filtered signal using the 3.5 standard deviation threshold for spike detection. Visual stimulation was controlled by Spike2 software (Cambridge Electronic Design, United Kingdom). Stimulation marks were recorded along with the electrophysiological signals. Flash VEPs were evoked using light-emitting diodes (LEDs, 2200 lx) positioned 15 cm in front of the rat’s left eye. The repeated visual training consisted of 300 flashes at 0.5 Hz repeated every 15 min for 3 h (Figure 1A; Foik et al., 2015). Control recordings were carried out (100 flash repetitions at 0.1 Hz) before and after visual training to investigate the effect of training. A schematic diagram of the experimental protocol is shown in Figure 1.
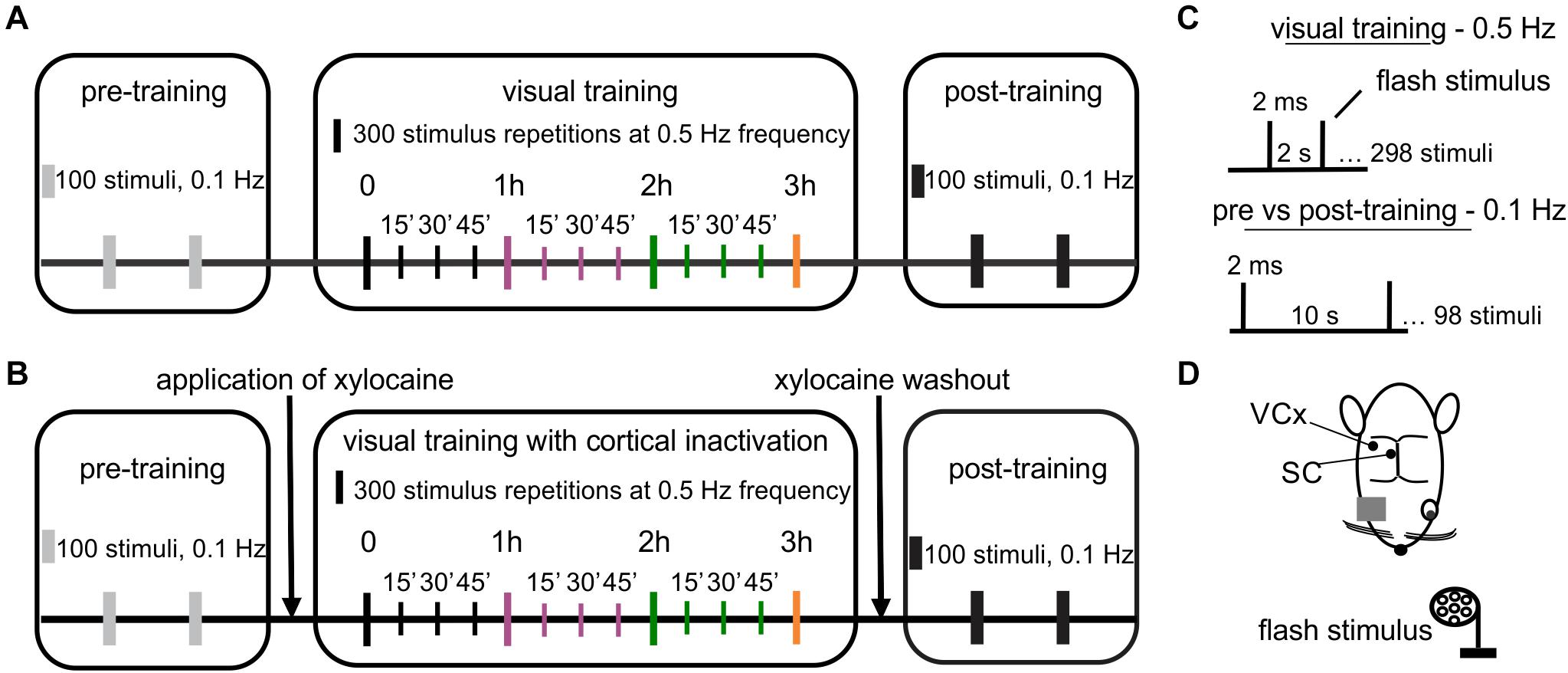
Figure 1. Design of an experiment revealing visual system plasticity caused by visual training. (A) The visual training paradigm: 100 repetitions of light flashes at 0.1 Hz during pre- and post-training controls and 300 repetitions of light flashes every 15 min through 3 h at 0.5 Hz during visual training. (B) Visual training paradigm paired with cortical inactivation. The graph presents the same parameters of visual stimuli as in (A). (C) Visual stimulus parameters used in training. (D) Schematic representation of the top view of the rat head with marked electrodes locations for the SC and the V1, both contralateral to the stimulated eye.
Temporal Inactivation of the Cortex
A plastic chamber placed above the right hemisphere was filled with a xylocaine solution (2.5%, Lidocainum Hydrochloricum WZF, Polfa Warszawa S.A, Poland) to inactivate cortical activity (action potential blockage) during visual training (Wagman et al., 1967). The solution was replaced every 30 min during electrophysiological recordings.
Data Processing
Collected data (seven—visual training, seven–visual training with cortical inactivation) was analyzed using Matlab (Mathworks, Natick, MA, United States). The LFP signal was preprocessed using the 1st order band-stop Butterworth filter at 50 Hz, a high-pass filter at 0.1 Hz, and a low-pass filter at 100 Hz. Continuous signals were divided into trials 1.2 s long (from 0.2 s before to 1 s after stimulus). The peak-to-peak amplitude of VEPs was calculated at every hour during visual training and control recordings in the 0–0.2 s time range, where 0 is a stimulus onset time. Each channel was normalized in the following way: the training data (VEP amplitude from 1, 2, and 3 h of training) was compared to the mean response magnitude of the first series of visual stimulation (300 light flashes, time 0), which was assumed as a 100%. For every hour, the percent increase of the VEP amplitude (%) was computed. In the same way, the difference between the post and pre-training controls was measured, where the pre-training was assumed as 100%. The LFP data is presented in the mV unit. Cortical LFPs were analyzed in the delta (1–4 Hz), and theta (4–7 Hz) frequency ranges to monitor brain state. The mean frequency of the EEG signal occurring during recording was estimated at 1–5.6 Hz level. The signal to noise ratio as a percent change was obtained by dividing the VEP amplitude by the peak to peak amplitude of the whole particular time recording (raw signal; %). This analysis was performed to check whether the VEP amplitude during a few hours of training will separate more from the noise generated by spontaneous activity of the brain. The VEP area for each averaged VEP was calculated as a sum of an absolute value of a voltage from each averaged evoked potential in a time range of 0–0.5 s. For statistical analysis, two recording channels for each layer from a given structure were taken in each animal. The VEPs amplitudes and signal to noise ratio factor of pre- and post-training recordings were compared using two-tailed paired t-tests. To compare mean collicular VEP amplitude between the two experimental paradigms: V1 activated and inactivated, the two-tailed unpaired t-tests with Welch correction was used. One-way repeated-measures ANOVA with greenhouse Geisser correction was used with the Dunnett’s post hoc test to investigate changes in the mean VEPs responses and signal to noise ratio for three h of visual training. The statistical comparison between the average difference of pre and post-training responses for SC and V1 was computed by the Mann–Whitney test. Results are presented as a mean % ± SEM.
Histology
Electrodes coated with DiI (1,I’-dioctadecyl-3.3,3’,3’ tetramethyl-indocarbocyanine perchlorate; Sigma-Aldrich, Germany) were used to facilitate electrode tract reconstruction (DiCarlo et al., 1996). At the end of the experiments, rats were given an overdose of Nembutal (150 mg/kg) and transcardially perfused with 4% paraformaldehyde in 0.1 M PBS (Sigma-Aldrich, Germany). The brains were removed, postfixed for 24 h in 4% paraformaldehyde, stored successively in 10, 20, and then in 30% sucrose in 0.1 M PBS before sectioning. Brains were cut into 40 μm slices and stained with cresyl violet. The data from the experiments with incorrect electrode placements were excluded from further data analysis.
Results
Analysis of VEP amplitude for the V1 included the granular layer (400–800 μm), with a typical reversal of potential polarity and infragranular layers (1–1.8 mm), identified by negative components. We considered layer V and VI as infragranular layers. We also analyzed the two retino-recipient layers of the SC:SGS (2.8–3.4 mm) and SO (3.6–4 mm), which were characterized by a biphasic oscillatory pattern of the recorded signal.
Effect of Visual Training on the Magnitude of VEPs Amplitude
To test the effect of repetitive visual training, we compared pre- and post-training responses to light flashes. Examples of cortical and collicular VEPs from a single rat and averaged for all animals are shown in Figures 2A–H. The comparison of pre- and post-training VEPs showed a larger response magnitude after 3 h training session (see section “Materials and Methods” for more details), both in the cortex and the SC (Figures 2I–L). In the SGS layers of the SC, the amplitude of the post-training response was significantly greater (180 ± 9 %, n = 10, p < 0.001) than the pre-training amplitude. The increase in the SO layers was even stronger (208 ± 17 %, n = 10, p = 0.004). Results for both layers in the V1 revealed a significant increase of the VEP amplitude after visual training. The potentiated response in the granular layer after visual training was higher (179 ± 18 %, n = 12, p = 0.005) than the increase seen in the infragranular layers (144 ± 10 %, n = 14, p = 0.003). The potentiation following 3 h training was also indicated by an increase of the signal to noise ratio for post-training control compared to pre-training both in the V1 and the SC (Figures 2M–P). Analysis of VEP area difference both for collicular and cortical pre vs. post-training response revealed statistical significance for SC SGS (p = 0.007) and SC SO (p = 0.004, Figures 2Q–T).
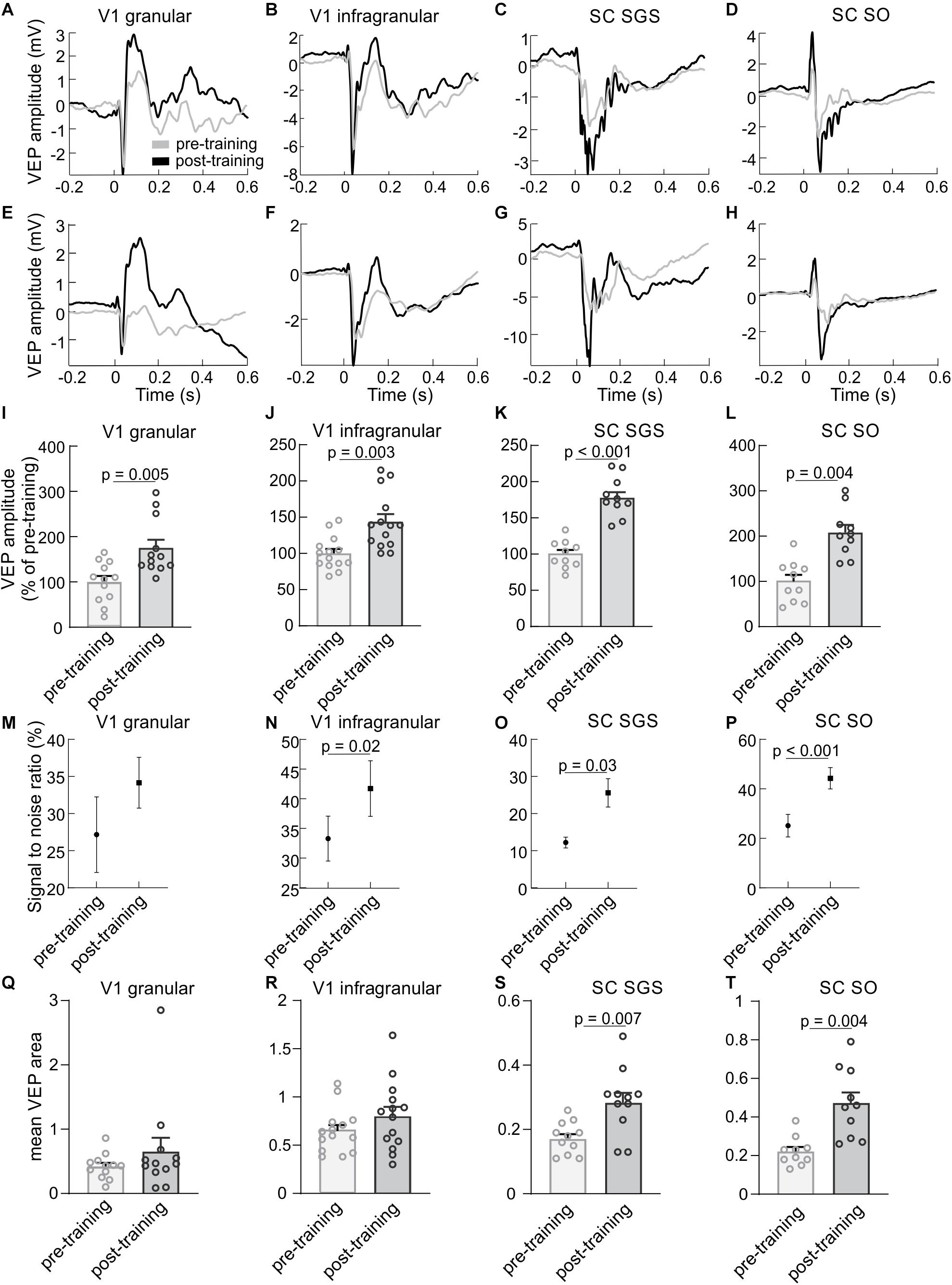
Figure 2. Visual training enhances visually evoked responses in the V1 and the SC. The first column presents results for a granular layer of the V1, second column for infragranular layers for the V1, the third column for the SGS layer and the last column for the SO layer of the SC. (A–D) Average VEPs (n = 100) recorded in the V1 and SC for a single experiment before (gray line) and after (black line) visual training for the same animal. (E–H) Average responses in the V1 and SC for all rats before and after visual training. Time 0 is a stimulus onset. (I–L) Comparison between the average pre- (gray bars) and post-training (black bars) VEP amplitudes for the V1 and SC. (M–P) Signal to noise ratio changes for pre- and post-training recordings for the V1 and SC. (Q–T) Comparison of VEP areas of pre- and post- training evoked potentials.
Figure 3 presents VEPs obtained for every hour of visual training in the V1 and the SC, including division into layers. Examples of cortical and collicular VEPs from a single rat and averaged for all animals are shown in Figures 3A–H. The largest increase of VEP amplitudes was observed after three hours of stimulation in all studied cases. Response in granular layer (Figure 3I; F(3,11) = 4.76, p = 0.009) was significantly greater after second hour (183 ± 17 %, p = 0.04) and thrid hour of training (215 ± 33 %, p = 0.04) in comparison to control. In the infragranular layers [Figure 3J; F(3,13) = 4.76, p = 0.0006] the greatest amplitude was during third hour (163 ± 8 %, p = 0.0005) as well as second hour of training (138 ± 6 %, p = 0.009) than in control.
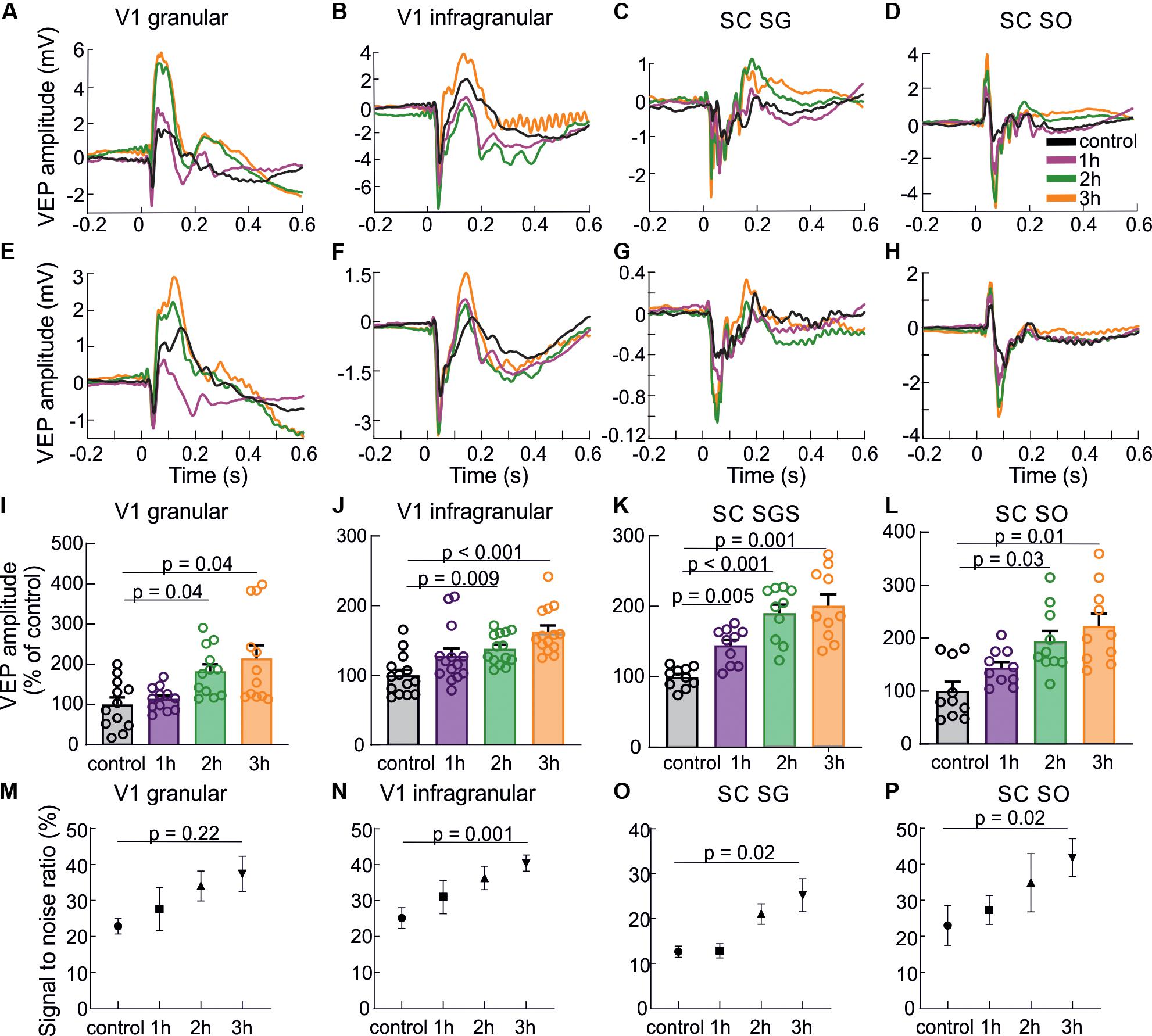
Figure 3. Response enhancement during visual training. The results are presented in the following order: the first column shows the results for a granular layer of the V1, second column for infragranular layers for the V1, the third column for the SGS layer and the last column for the SO layer of the SC. (A–D) VEPs for each hour of visual training recorded in different layers of the V1 and the SC for a single experiment. (E–H) Average population responses for each hour (every fourth sensory stimulation) during visual training recorded in different layers of the V1 and the SC and encoded by different colors. (I–L) Mean VEP amplitudes for all rats and every hour of visual training for the V1 and SC. (M–P) Signal to noise ratio changes for every hour of visual training for all layers.
Also in the SC, the visual training evoked significant VEP potentiation [for SGS; F(3,9) = 6.59, p < 0.0001, for SO; F(3,9) = 4.76, p = 0.005]. In the SGS (Figure 3K) the response was the highest after thrid hour of the training (201 ± 18 %, p = 0.001), then second hour (190 ± 14 %, p = 0.0005), and first hour (145 ± 9 %, p = 0.005) in comparison to control. The same tendency was observed in the SO layer (Figure 3L), where the greatest visual response was after thrid hour of training (223 ± 24 %, p = 0.01), then second hour (194 ± 20 %, p = 0.03), and first hour (144 ± 10 %, p = 0.2). Mean VEP amplitude after the first hour of training compared to control was not significant. Increase of the signal to noise ratio during 3 h of visual training (Figures 3M–P) was statistical significant for V1 infragranular layers (p = 0.001), SC SGS (p = 0.02) and for SC SO (p = 0.02).
Effect of V1 Inactivation on Visual Training Efficiency in the SC
The potentiation in the SC following visual training may be a result of two phenomena: (1) as a result of changes to retinotectal synapses independently of the V1; (2) or enhancement in the V1 that modulates response in the SC. To resolve this problem, we selectively inactivated the V1 through the application of xylocaine solution on the surface of the V1 for the duration of visual training. Chemical inactivation of the V1 caused strong silencing of cortical responses during visual training (Figures 4A,C), leaving only the incoming volley (the incoming thalamic information). This phenomenon was also observed in a study with the barrel cortex inactivated through the cooling cortical surface (Kublik et al., 2001). The effect of xylocaine (sodium channel blocker) application was also visible as a multi-unit activity drop shown in Figure 4B in the form of a raster plot and comparison of two histograms from the same recording site. The chemical inactivation prevented cortex from response enhancement as presented in Figure 4D and revealed no difference in amplitude before and after training (83 ± 4%, n = 14, p = 0.25). This result confirms an effective deactivation of the V1 during training and blocking the learning effect in this structure. The comparison between pre- and post-training recordings in the SC again, showed significant enhancement of the response as a result of the visual training while V1 deactivation. In the SGS layer, the amplitude of the visual response was significantly greater in post-training control compared to pre-training recording (Figure 4D; 212 ± 26%, n = 10, p = 0.0025). Moreover, the amplitude was higher than changes evoked in the SO layers (Figure 4C; 161 ± 23 %, n = 10, p = 0.03). We observed an increase of the collicular VEP amplitudes during visual training even when cortical activity was silenced [for SGS; F(3,9) = 6.59, p = 0.005, for SO; F(3,9) = 9.28, p = 0.02]. For the SGS layers (Figure 4E) VEP amplitude was the highest after 3 h of visual training compared to control time (262 ± 36%, p = 0.01), then second hour (222 ± 25 %, p = 0.02) and after first hour (152 ± 10 %, p = 0.08). We found similar tendency in the SO layer (Figure 4F) where the potentiation of visual response was the greatest after third hour of stimulation (222 ± 31 %, p = 0.04), then second hour (213 ± 36 %, p = 0.1), and after first hour (173 ± 24%, p = 0.1). A comparison of the visual training effects of the two conditions (V1 activated and inactivated) did not show a significant difference in the SC (Figure 4F). The increase of visual response for every hour of training in both SC layers was similar for both V1 conditions. This result indicates that the temporary blocking of V1 did not inhibit VEP plasticity in SC evoked by repeated visual training. We can also conclude that the enhancement of VEP amplitude in the SC occurred mainly through a retinotectal synapse. The stronger enhancement occurred in the SC compared to the V1. The average difference between pre and post-training responses for SC = 106 ± 17% was higher than for V1 = 17 ± 4 % without xylocaine (Figure 4H; p = 0.002) and even higher with xylocaine application during the training (Figure 4I, p < 0.0001).
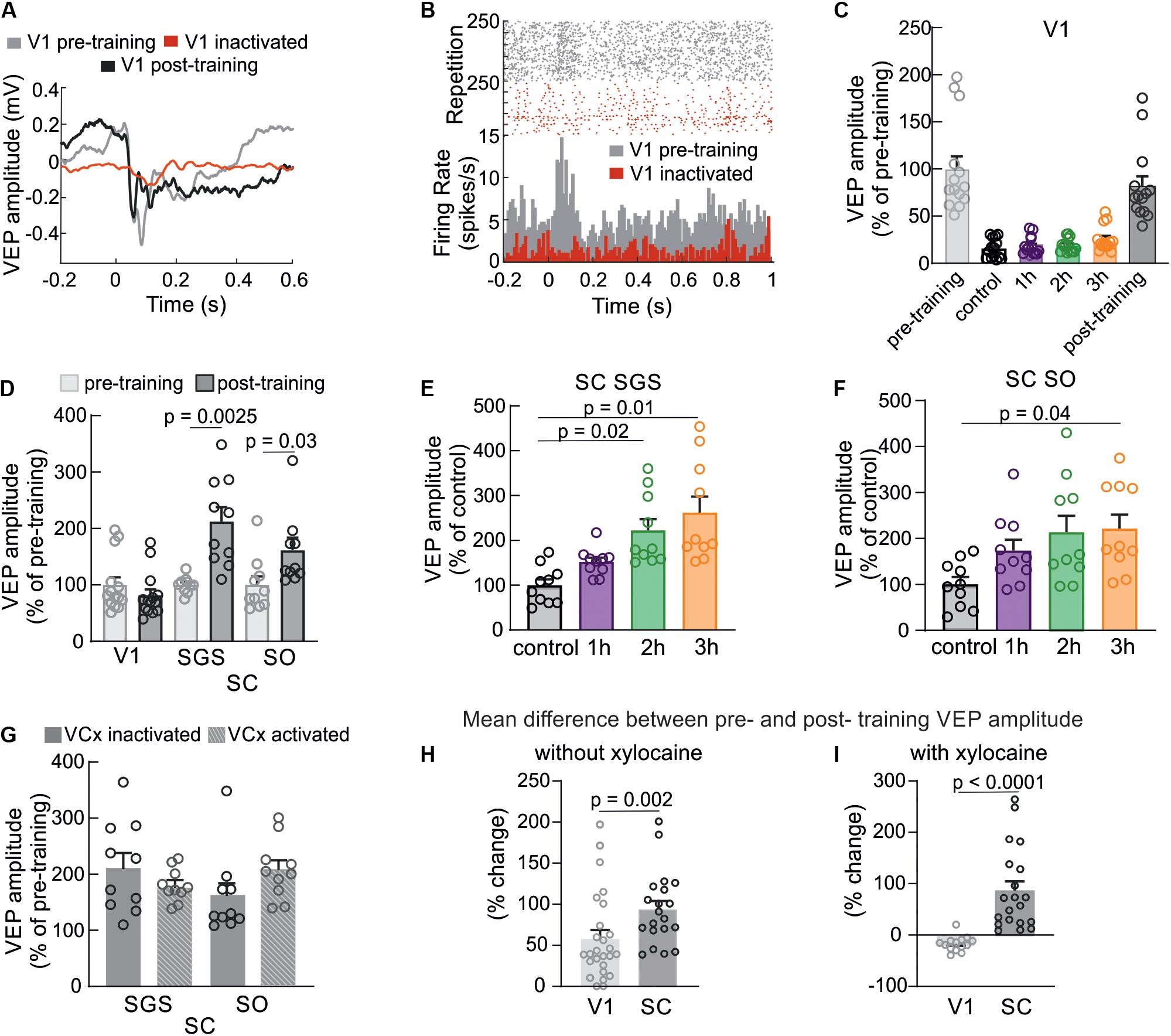
Figure 4. Cortical inactivation does not affect plasticity in the SC. (A) Typical example of the visual cortex VEP recorded from the single experiment before (gray line), during visual training when xylocaine was applied (red line), and after training when xylocaine was washed out (black line). (B) Raster plots and histograms of multi-unit spiking activity showing reduced spiking activity and lack of response in the V1 during xylocaine application. (C) Silencing of cortical responses during visual training as a result of xylocaine application. (D) Comparison of average responses before (gray) and after (black) visual training in the V1, SGS, and SO layers of the SC when cortical activity was suppressed. (E,F) Average response amplitudes in the SC (SGS and SO, respectively) for every hour of the visual training while V1 silencing. (G) Comparison of mean values of post-training VEP amplitudes in the SC between two experimental conditions: with V1 active (n = 10) and V1 inactive (n = 10). (H) The comparison of mean differences between pre- and post- training potential amplitudes for V1 and SC without xylocaine. (I) The same comparison as in H, when xylocaine was used to silence the V1 during training.
Discussion
Our results showed that the 3 h visual training evoked strong enhancement of the VEPs both in the V1 and the SC. Moreover, our paradigm of repetitive visual training evoked a stronger increase in the response in the SC than in the V1 (Figure 2). We confirm that visual training causes enhancement of VEP amplitude in the V1 as it was described before (Sawtell et al., 2003; Teyler et al., 2005; Frenkel et al., 2006; Ross et al., 2008; Cooke and Bear, 2010, 2012) and extends the knowledge showing positive results of this training in the rat’s SC. Specifically, we showed in an electrophysiological study that single training session, lasting several hours, induces plasticity of visual responses.
Based on the literature we can enumerate several well-described paradigms of repetitive visual stimulation which differ from each other mainly in the type of visual stimulus, presentation timing, a number of repetitions and the frequency of the stimulus (Furmanski et al., 2004; Clapp et al., 2006a; Frenkel et al., 2006; Kuo and Dringenberg, 2009; Cooke and Bear, 2010; Hager and Dringenberg, 2010). One well–known protocol uses repeated presentations of a specifically oriented visual stimulus through several days that causes stimulus-selective potentiation of a cortical response in awake mice and enhancement of a signal detection power in humans (Seitz et al., 2009). This type of plasticity is well described in layer IV of the V1 (Frenkel et al., 2006; Cooke and Bear, 2012). In our study, we found that the potentiation of the visual response to a flash stimulus also occurs in the infragranular layers. Specific types of repeated visual stimulation (shorter and more intense) are also able to induce the modulation of VEP plasticity and modifications of synaptic connectivity in the mature V1. Studies carried out on humans demonstrated how 10 min presentation of checkerboard reversals (Teyler et al., 2005) resulted in sustained amplitude modulation of early components of subsequent VEPs, whereas a rapid (9 Hz, for 2 min) checkerboard stimulation might induce the enhancement of visual response in the adult rats V1 (Clapp et al., 2006a). Our experimental paradigm provides a form of rapid visual training consisted of a series of flashes repeated every 15 min through 3 h. The frequency of stimulus presentation (0.5 Hz) is lower than was used by Clapp et al. (2006a); nevertheless, it was sufficient to evoke significantly enhanced responses in the VC and SC.
Flashing stimuli compared to drifting sinusoidal gratings or reversal checkerboards are rather strong stimuli, thus can evoke changes faster. It was shown before that in adult mice flashing stimuli evoke robust long-term changes in the V1 neuronal response and increase the broadband power of LFP signal (Funayama et al., 2015, 2016). Thus, the flash stimulus might be successfully used for the induction of modulation in the neuronal response (Minamisawa et al., 2017), which we confirmed in our study.
It is considered that the reinforcement of neuronal response occurring following repetitive stimulation in the visual system in awake animals, including humans might be triggered by increasing the number or gain of neurons involved in response to the trained stimulus (Furmanski et al., 2004; Hager and Dringenberg, 2010). In our study, we observed facilitation of visual response to a flash stimulus, although the experiments were carried out on anesthetized rats. Previous studies also confirmed the occurrence of learning processes in the visual system in animals under deep anesthesia, where LTP dependent of NMDA receptors was effectively induced in the V1 through electrical theta-burst stimulation of the visual pathway (Heynen and Bear, 2001; Kuo and Dringenberg, 2009) or via repetitive visual stimulation (Clapp et al., 2006a).
We found that 3 h of repeated visual stimulation evoked enhancement not only in the cortex but also in the SC (Figures 2, 3). We also show for the first time that, stronger response enhancement occurred in the SC than in the V1. So far, little attention has been devoted to the investigation of this effect. Zhang et al. (2000) shown that the repetitive exposure to dimming stimuli effectively induced the LTP of developing retinotectal synapses in Xenopus tadpoles. This effect is attributed mainly to changes in synaptic efficacy at retinotectal projections. However, potentiation of neuronal response at this level of the visual processing can also derive from the cortex due to inputs from layer 5 of the V1 (Waleszczyk et al., 2004; May, 2006). Our results revealed that collicular response potentiation is not changed when V1 is blocked during repetitive visual training. This indicates that the increase of neuronal responses in the SC is most likely due to the enhancement of the retinotectal projection. There are supportive studies performed on rats where ablation of the V1 caused facilitation of the LTP formation in the SC, indicating a suppressive influence of the V1 inputs into the SC (Shibata et al., 1990; Okada, 1993). In our studies, we observed potentiation of the SC response during visual training regardless of whether the V1 was activated or inactivated (Figures 3, 4).
In summary, the data presented here show a new form of plasticity occurring after 3 h of repeated visual training in the primary VC and SC. We demonstrated that the enhancement of neuronal responses in the SC following our paradigm of visual stimulation occurred independently of the V1, most likely through retinotectal projection. Further research will be needed to better understand the mechanisms responsible for this phenomenon.
Data Availability Statement
The datasets presented in this article are not readily available because the datasets can be available only in collaboration with authors. Requests to access the datasets should be directed to KK, ay5rb3JkZWNrYUBuZW5ja2kuZWR1LnBs.
Ethics Statement
The animal study was reviewed and approved by the First Warsaw Local Ethical Commission for Animal Experimentation (521/2018).
Author Contributions
KK, AF, and WW designed the study. KK performed all the electrophysiological recordings. KK and AW analyzed the data. KK and AF interpreted results and wrote the manuscript. KK received funding. All authors edited and approved the manuscript.
Funding
This work was funded by the National Science Centre Poland Grant 2017/25/N/NZ4/02914 for KK. AF was financed by the International Centre for Translational Eye Research (MAB/2019/12), a project carried out within the International Research Agendas program of the Foundation for Polish Science co-financed by the European Union under the European Regional Development Fund and the National Science Centre Grant 2019/34/E/NZ5/00434.
Conflict of Interest
The authors declare that the research was conducted in the absence of any commercial or financial relationships that could be construed as a potential conflict of interest.
Acknowledgments
We dedicate this work to WW, our friend, mentor, and supervisor. This manuscript has been released as a pre-print at the bioRxiv server (Kordecka et al., 2020).
References
Clapp, W. C., Eckert, M. J., Teyler, T. J., and Abraham, W. C. (2006a). Rapid visual stimulation induces N-methyl-D-aspartate receptor-dependent sensory long-term potentiation in the rat cortex. Neuroreport 17, 511–515. doi: 10.1097/01.wnr.0000209004.63352.10
Clapp, W. C., Muthukumaraswamy, S. D., Hamm, J. P., Teyler, T. J., and Kirk, I. J. (2006b). Long-term enhanced desynchronization of the alpha rhythm following tetanic stimulation of human visual cortex. Neurosci. Lett. 398, 220–223. doi: 10.1016/j.neulet.2005.12.081
Cooke, S. F., and Bear, M. F. (2010). Visual Experience Induces Long-Term Potentiation in the Primary Visual Cortex. J. Neurosci. 30, 16304–16313. doi: 10.1523/jneurosci.4333-10.2010
Cooke, S. F., and Bear, M. F. (2012). Stimulus-selective response plasticity in the visual cortex: an assay for the assessment of pathophysiology and treatment of cognitive impairment associated with psychiatric disorders. Biol. Psychiatry 71, 487–495. doi: 10.1016/j.biopsych.2011.09.006
DiCarlo, J. J., Lane, J. W., Hsiao, S. S., and Johnson, K. O. (1996). Marking microelectrode penetrations with fluorescent dyes. J. Neurosci. Methods 64, 75–81. doi: 10.1016/0165-0270(95)00113-1
Elvsshagen, T., Moberget, T., Bøen, E., Boye, B., Englin, N. O. A., Pedersen, P., et al. (2012). Evidence for impaired neocortical synaptic plasticity in bipolar II disorder. Biol. Psychiatry 71, 68–74. doi: 10.1016/j.biopsych.2011.09.026
Eysel, U. T., Eyding, D., and Schweigart, G. (1998). Repetitive optical stimulation elicits fast receptive field changes in mature visual cortex. Neuroreport 9, 949–954. doi: 10.1097/00001756-199803300-00034
Foik, A. T., Kublik, E., Sergeeva, E. G., Tatlisumak, T., Rossini, P. M., Sabe, B. A., et al. (2015). Retinal origin of electrically evoked potentials in response to transcorneal alternating current stimulation in the rat. Investig. Ophthalmol. Vis. Sci. 56, 1711–1718. doi: 10.1167/iovs.14-15617
Frenkel, M. Y., Sawtell, N. B., Diogo, A. C. M., Yoon, B., Neve, R. L., and Bear, M. F. (2006). Instructive effect of visual experience in mouse visual cortex. Neuron 51, 339–349. doi: 10.1016/j.neuron.2006.06.026
Funayama, K., Hagura, N., Ban, H., and Ikegaya, Y. (2016). Functional organization of flash-induced V1 offline reactivation. J. Neurosci. 36, 11727–11738. doi: 10.1523/JNEUROSCI.1575-16.2016
Funayama, K., Minamisawa, G., Matsumoto, N., Ban, H., Chan, A. W., Matsuki, N., et al. (2015). Neocortical rebound depolarization enhances visual perception. PLoS Biol. 13:e1002231. doi: 10.1371/journal.pbio.1002231
Furmanski, C. S., Schluppeck, D., and Engel, S. A. (2004). Learning Strengthens the Response of Primary Visual Cortex to Simple Patterns and after training with fMRI. Subjects practiced for more than 14,000 trials during more than 20 daily training sessions on a pattern that was oriented 45 clockwise. Curr. Biol. 14, 573–578. doi: 10.1016/j
Gall, C., Mueller, I., Gudlin, J., Lindig, A., Schlueter, D., Jobke, S., et al. (2008). Vision- and health-related quality of life before and after vision restoration training in cerebrally damaged patients. Restor. Neurol. Neurosci. 26, 341–353.
Hager, A. M., and Dringenberg, H. C. (2010). Training-induced plasticity in the visual cortex of adult rats following visual discrimination learning. Learn. Mem. 17, 394–401. doi: 10.1101/lm.1787110
Heynen, A. J., and Bear, M. F. (2001). Long-term potentiation of thalamocortical transmission in the adult visual cortex in vivo. J. Neurosci. 21, 9801–9813. doi: 10.1523/jneurosci.21-24-09801.2001
Jaepel, J., Hübener, M., Bonhoeffer, T., and Rose, T. (2017). Lateral geniculate neurons projecting to primary visual cortex show ocular dominance plasticity in adult mice. Nat. Neurosci. 20, 1708–1714. doi: 10.1038/s41593-017-0021-0
Kordecka, K., Foik, A. T., Wierzbicka, A., and Waleszczyk, W. J. (2020). Cortical inactivation does not block response enhancement in the superior colliculus. bioRxiv [Preprint] doi: 10.1101/2020.03.19.998807
Kublik, E., Musial, P., and Wróbel, A. (2001). Identification of principal components in cortical evoked potentials by brief surface cooling. Clin. Neurophysiol. 112, 1720–1725. doi: 10.1016/S1388-2457(01)00603-4
Kuo, M. C., and Dringenberg, H. C. (2009). Short-term (2 to 5 h) dark exposure lowers long-term potentiation (LTP) induction threshold in rat primary visual cortex. Brain Res. 1276, 58–66. doi: 10.1016/j.brainres.2009.04.042
May, P. J. (2006). The mammalian superior colliculus: laminar structure and connections. Prog. Brain Res. 151, 321–378. doi: 10.1016/S0079-6123(05)51011-2
Minamisawa, G., Funayama, K., Matsumoto, N., Matsuki, N., and Ikegaya, Y. (2017). Flashing lights induce prolonged distortions in visual cortical responses and visual perception. eNeuro 4:ENEURO.0304-16. doi: 10.1523/ENEURO.0304-16.2017
Mueller, I., Mast, H., and Sabel, B. A. (2007). Recovery of visual field defects: a large clinical observational study using vision restoration therapy. Restor. Neurol. Neurosci. 25, 563–572.
Normann, C., Schmitz, D., Fürmaier, A., Döing, C., and Bach, M. (2007). Long-term plasticity of visually evoked potentials in humans is altered in major depression. Biol. Psychiatry 62, 373–380. doi: 10.1016/j.biopsych.2006.10.006
Okada, Y. (1993). The properties of the long-term potentiation (LTP) in the superior colliculus. Prog. Brain Res. 95, 287–296. doi: 10.1016/s0079-6123(08)60376-3
Paxinos, G., and Watson, C. (2007). The rat brain in stereotaxic coordinates sixth edition. Elsevier Acad. Press 170, 547–612.
Poggel, D. A., Mueller, I., Kasten, E., and Sabel, B. A. (2008). Multifactorial predictors and outcome variables of vision restoration training in patients with post-geniculate visual field loss. Restor. Neurol. Neurosci. 26, 321–339.
Ross, R. M., McNair, N. A., Fairhall, S. L., Clapp, W. C., Hamm, J. P., Teyler, T. J., et al. (2008). Induction of orientation-specific LTP-like changes in human visual evoked potentials by rapid sensory stimulation. Brain Res. Bull. 76, 97–101. doi: 10.1016/j.brainresbull.2008.01.021
Sabel, B. A. (2008). Plasticity and Restoration of Vision After Visual System Damage: An Update. Amsterdam: IOS Press.
Sabel, B. A., and Gudlin, J. (2014). Vision restoration training for glaucoma: a randomized clinical trial. JAMA Ophthalmol. 132, 381–389. doi: 10.1001/jamaophthalmol.2013.7963
Sawtell, N. B., Frenkel, M. Y., Philpot, B. D., Nakazawa, K., Tonegawa, S., and Bear, M. F. (2003). NMDA receptor-dependent ocular dominance plasticity in adult visual cortex. Neuron 38, 977–985. doi: 10.1016/s0896-6273(03)00323-4
Seitz, A. R., Kim, D., and Watanabe, T. (2009). Rewards evoke learning of unconsciously processed visual stimuli in adult humans. Neuron 61, 700–707. doi: 10.1016/j.neuron.2009.01.016
Shibata, Y., Tomita, H., and Okada, Y. (1990). The effects of ablation of the visual cortical area on the formation of LTP in the superior colliculus of the rat. Brain Res. 537, 345–348.
Sommeijer, J.-P., Ahmadlou, M., Saiepour, M. H., Seignette, K., Min, R., Heimel, J. A., et al. (2017). Thalamic inhibition regulates critical-period plasticity in visual cortex and thalamus. Nat. Neurosci. 20, 1715–1721. doi: 10.1038/s41593-017-0002-3
Teyler, T. J., Hamm, J. P., Clapp, W. C., Johnson, B. W., Corballis, M. C., and Kirk, I. J. (2005). Long-term potentiation of human visual evoked responses. Eur. J. Neurosci. 21, 2045–2050. doi: 10.1111/j.1460-9568.2005.04007.x
Wagman, I. H., De Jong, R. H., and Prince, D. A. (1967). Effects of lidocaine on the central nervous system. Anesthesiology 28, 155–172. doi: 10.1097/00000542-196701000-00017
Waleszczyk, W. J., Wang, C., Benedek, G., Burke, W., and Dreher, B. (2004). Motion sensitivity in cat’s superior colliculus: contribution of different visual processing channels to response properties of collicular neurons. Acta Neurobiol. Exp. 64, 209–228.
Keywords: visual evoked potential, plasticity, repetitive visual training, visual cortex, superior colliculus, electrophysiology
Citation: Kordecka K, Foik AT, Wierzbicka A and Waleszczyk WJ (2020) Cortical Inactivation Does Not Block Response Enhancement in the Superior Colliculus. Front. Syst. Neurosci. 14:59. doi: 10.3389/fnsys.2020.00059
Received: 21 May 2020; Accepted: 22 July 2020;
Published: 07 August 2020.
Edited by:
Alessandra Angelucci, The University of Utah, United StatesReviewed by:
Sarah L. Pallas, University of Massachusetts Amherst, United StatesGregor Rainer, Université de Fribourg, Switzerland
Copyright © 2020 Kordecka, Foik, Wierzbicka and Waleszczyk. This is an open-access article distributed under the terms of the Creative Commons Attribution License (CC BY). The use, distribution or reproduction in other forums is permitted, provided the original author(s) and the copyright owner(s) are credited and that the original publication in this journal is cited, in accordance with accepted academic practice. No use, distribution or reproduction is permitted which does not comply with these terms.
*Correspondence: Katarzyna Kordecka, ay5rb3JkZWNrYUBuZW5ja2kuZWR1LnBs; Andrzej T. Foik, YWZvaWtAaWNoZi5lZHUucGw=
†These authors have contributed equally to this work
‡Deceased