- 1Research Center for Sports, Exercise and Human Development, University of Trás-os-Montes and Alto Douro, Vila Real, Portugal
- 2Faculty of Biology and Medicine, ISSUL, Institute of Sport Sciences, University of Lausanne, Lausanne, Switzerland
- 3INEFC-Barcelona Sport Sciences Research Group, Institut Nacional d'Educació Física de Catalunya, University of Barcelona, Barcelona, Spain
Oxygen uptake () kinetics has been reported to be influenced by the activity mode. However, only few studies have compared O2 kinetics between activities in the same subjects in which they were equally trained. Therefore, this study compared the O2 kinetics response to swimming, arm cranking, and cycling within the same group of subjects within the heavy exercise intensity domain. Ten trained male triathletes (age 23.2 ± 4.5 years; height 180.8 ± 8.3 cm; weight 72.3 ± 6.6 kg) completed an incremental test to exhaustion and a 6-min heavy constant-load test in the three exercise modes in random order. Gas exchange was measured by a breath-by-breath analyzer and the on-transient O2 kinetics was modeled using bi-exponential functions. O2peak was higher in cycling (65.6 ± 4.0 ml·kg−1·min−1) than in arm cranking or swimming (48.7 ± 8.0 and 53.0 ± 6.7 ml·kg−1·min−1; P < 0.01), but the O2 kinetics were slower in swimming (τ1 = 31.7 ± 6.2 s) than in arm cranking (19.3 ± 4.2 s; P = 0.001) and cycling (12.4 ± 3.7 s; P = 0.001). The amplitude of the primary component was lower in both arm cranking and swimming (21.9 ± 4.7 and 28.4 ± 5.1 ml·kg−1·min−1) compared with cycling (39.4 ± 4.1 ml·kg−1·min−1; P = 0.001). Although the gain of the primary component was higher in arm cranking compared with cycling (15.3 ± 4.2 and 10.7 ± 1.3 ml·min−1·W−1; P = 0.02), the slow component amplitude, in both absolute and relative terms, did not differ between exercise modes. The slower O2 kinetics during heavy-intensity swimming is exercise-mode dependent. Besides differences in muscle mass and greater type II muscle fibers recruitment, the horizontal position adopted and the involvement of trunk and lower-body stabilizing muscles could be additional mechanisms that explain the differences between exercise modalities.
Introduction
Muscular exercise requires large changes in the metabolic rate often exceeding 10-fold the resting steady-state values (Poole and Jones, 2012). At the onset of constant moderate to heavy intensity exercise there is an early fast increase in oxygen consumption (O2), which is usually completed within the ~15–25 s of exercise (Phase I or cardio-dynamic phase). This early response is attributed to the increase in cardiac output and thus pulmonary blood, not reflecting the muscular O2 (Rossiter et al., 1999). After this initial phase, a rapid and exponential increase in O2, with a time constant between ~20–45 s, occurs (Phase II—primary or fast component), in which pulmonary O2 kinetics largely reflect the kinetics of O2 consumption in the exercising muscles (Grassi et al., 1996). This transitory phase, prior to the achievement of any steady state (moderate intensity) or prior to the development of the slow component phase (heavy and severe intensities), provides a window into the fundamental processes of muscle energetics and metabolic control that are otherwise not accessible (Poole and Jones, 2012).
The comparison of O2 kinetics between various sport activities, body posture, ergometers or groups has been used for providing insights to the fundamental mechanisms of the different phases (Poole and Jones, 2012). Among many facts the O2 kinetics are broadly altered when different ergometers are utilized, being the majority of studies in this field conducted by comparing running and cycling exercise. Aiming to explore the relationship between the O2 slow component (O2sc) and the muscle contraction regimen used, Carter et al. (2000) reported that the magnitude of the O2sc phase was less for running than for cycling at heavy exercise. This could be related to differences in the muscle contraction regimen, which may have caused a relatively greater recruitment of the less efficient type II muscle fibers in cycling compared with running. At the severe intensity domain, Hill et al. (2003) reported that the time constant of the fast component phase was faster (reflecting the differences in the muscle contraction type involved) and its amplitude was greater (associated with higher oxygen demand) in running than in cycling. Moreover, the amplitude of the O2sc phase was 40% smaller in running, concluding that the exercise modality affects the O2 kinetics even within the severe intensity. Extending the O2 kinetics analysis beyond the O2sc phase with triathletes, Caputo and Denadai (2004) concluded that the time constant of the fast component phase during running and cycle exercise tests performed at maximal oxygen consumption (O2max) intensity were independent of the exercise mode performed, but dependent on the training status of the subjects These data suggest that the mechanical differences between cycling and running modes do not influence the time constant in the severe domain, contrarily to the O2sc.
Other hypothesis could explain differences in O2 kinetics during distinct exercise modes. The recruitment of a greater muscle mass could potentially compromise muscle perfusion (Saltin et al., 1998). Cycling engages the major muscle groups of the lower body, such that performance could be compromised compared with other exercise modes such as arm cranking, where a lower fraction of the total muscle mass is recruited (Drescher et al., 2015). In this latter exercise modality, the O2sc can also be exercise mode-dependent due to the recruitment of type II muscle fibers, which are in higher proportions in the upper body (Bernasconi et al., 2006). Therefore, arm cranking exercise could be a valuable paradigm exercise to examine whether the additional type II muscle fibers recruitment could contribute to possible differences in O2sc.
Typically swimming requires also lower muscle mass (predominantly upper body) and induces lower maximal O2 and HR responses than cycling (Roels et al., 2005), which might be due to several additional factors such as different body position inducing greater hydrostatic pressure and lower perfusion in the capillary bed of the working muscle, resulting in a reduction in both blood flow and oxygen transport. Thus, the horizontal body position adopted by swimmers, with lower muscle perfusion pressure, presumably as a consequence of lower arterial pressure, may be a key difference between swimming and upright exercise modes (Koga et al., 1999), as suggested by the slower kinetics of ventilation and gas exchange during supine compared with upright cycling exercise (Hughson et al., 1991). Hence, this postural factor could influence peripheral oxygen extraction and O2max.
Although the influence of the mechanical differences between running and cycling on O2 kinetics are well known, few studies have compared the O2 kinetics within other exercise modes within the same group of athletes. Even fewer included intensities that were clearly within the heavy intensity domain. There are methodological constraints for comparing O2 kinetics in different exercise modes: it is well-known that faster O2 kinetics and reduced O2sc amplitude are highly correlated with higher levels of aerobic fitness and improved training status (Koppo et al., 2004), whereas larger adaptations occur in the specific training mode —e.g., principle of specificity: swimming for swimmers (Roels et al., 2005). In other words, direct comparison between exercise modes might be valid only in subjects trained to the same extent in these tested modes. For these purposes, well-trained triathletes represent a unique and valuable group since they train indistinctly in swimming, cycling and running. This training pattern influences O2max and other physiological responses in different exercise modes as an effect of cross-training transfer between the upper and lower body exercise modes (Millet et al., 2002).
It is presently unknown whether the different mechanical and physiological factors interplay in the three modes of exercise does influence the O2 kinetics within the heavy intensity domain, when the same group of athletes, trained to a similar extent in the different exercise modalities, are involved. The analysis of such influences would provide new insights into the underlying control mechanisms in each exercise mode. Therefore, the purpose of this study was to compare the on-O2 kinetic response during swimming, arm cranking and cycling within the same group of trained triathletes when exercising within the heavy intensity domain. It was hypothesized that the exercise mode would contribute to distinct on-transient O2 kinetic patterns.
Materials and Methods
Participants
Ten well-trained male triathletes (age 23.2 ± 4.5 years; height 180.8 ± 8.3 cm; weight 72.3 ± 6.6 kg) participated in the study. The participants had a training background in triathlon for 7 ± 2 years, and underwent intensive training during 14 ± 4 h/week for at least 3 years. The study was approved by the by the institutional ethics committee of Montpellier (France) and conducted in accordance with the Declaration of Helsinki. All participants provided written informed consent before participation. They were familiarized with the incremental test procedure during training sessions performed prior to the testing, and were encouraged to give their best effort.
Design
The subjects completed three different maximal incremental exercise tests to assess maximal exercise capacity and cardiorespiratory parameters during free swimming, arm cranking, and cycling. Peak oxygen uptake (O2peak, ml·kg−1·min−1), ventilatory threshold (VT, % O2peak), peak power output (PPO, W) for cycling and arm cranking, and mean swimming velocity (v = d/t, m·s−1) were determined. Based on these parameters, workloads were calculated to eliciting equal relative intensity across the three exercise modes in a 6-min square-wave transition from rest to heavy-intensity for the assessment of on-transient O2 kinetic parameters. All tests were performed in the same conditions, that is, at the same time of day (±2 h) and with identical pre-test warm up: 10 min at the intensity corresponding to 50% of O2peak. The three different incremental tests were separated by a minimum of 72 h and completed in randomized order. The submaximal 6-min bouts mode were performed the day after the incremental test in the specific exercise mode. In the heavy-intensity bouts, parameters were estimated for the on-transient O2 kinetics and compared across the three exercise modes. Figure 1 shows a schematic of the testing protocol used.
Incremental Tests
In swimming, the incremental test comprised five consecutive 200-m efforts at increasing speed with a 15-s rest interval (Bentley et al., 2005; Libicz et al., 2005). All tests involved in-water starts and open turns without underwater gliding, and took place in the same indoor 50-m pool. The speed of each swim was determined for each swimmer using personal best time (PB) in 400 m freestyle measured in the preceding month. The first 200-m stage was swum 30 s slower than the PB, and the three subsequent steps had to be completed 5 s slower per stage. The final swim was performed at maximal speed. The swimmer's speed was controlled using an Aquapacer “Solo” (Challenge and Response, Inverurie, UK) and subject's swimming pace was set by auditory signals at 12.5-m intervals, delineated by visual marks along the bottom of the pool. The incremental arm crank test was completed on an arm ergometer (Monark Exercise AB 881E, Monark-Crescent AB, Varberg, Sweden). The initial workload was 17.5 W and was increased by 17.5 W every minute until exhaustion. The incremental sitting cycle test was completed on an electromagnetic cycle ergometer (Ergoline, Bitz, Germany). The initial workload was 60 W and was increased by 30 W every minute until exhaustion. Criteria for exhaustion were: (i) heart rate (HR) attaining maximal theoretical HR (HRmax = 220 − age), and (ii) O2 leveling off even despite an increase in workload. PPO was defined as the highest mean external power output maintained during 1 min.
Sub-Maximal 6-min Exercise Bouts
On subsequent days, the subjects performed 6-min of square-wave transition from rest to heavy intensity: constant workload at the metabolic intensity of 25%Δ above VT, i.e., calculated as VTΔ25% = [VT + 0.25 x (O2peak − VT)].
Physiological Measurements
Gas exchanges during the three incremental tests were measured breath-by-breath (bxb) using a portable system (K4 b2, Cosmed, Rome, Italy). During the swimming tests, the gas analyzer was attached to a swimming snorkel (Aquatrainer, Cosmed, Rome, Italy) that has been previously validated (Rodríguez et al., 2008) and used to measure gas exchange during swimming. In the incremental tests, bxb data were reduced to 30-s bin averages and O2peak was determined as the highest 30-s O2 average in either the swimming, arm cranking or cycling incremental tests. Using this protocol, the O2peak was always attained at the final stage of the incremental test. VT was calculated using the v-slope method and coincided with O2 that elicited the first departure from linearity in E/O2. VT was expressed as % O2peak in each exercise mode.
In the sub-maximal 6-min bouts, bxb data were first examined to exclude from the analysis the values greater than 3 SD from the local mean. Then data of the two square-wave transitions from rest to heavy-intensity were interpolated into 1-s values. In order to enhance the reliability in determining the parameters describing the O2 kinetics, data were filtered through a 4th Butterworth low-pass digital filter with a cut-off frequency equal to 0.05 Hz (Robergs et al., 2010). To remove the influence of the cardiodynamic phase on the subsequent response we chose to exclude the first 20 s of data from the analysis. The on-transient O2 kinetics was modeled according to the Equation (1):
where O2 (t) represents the weight-related O2 at a given time t; O2base is the baseline, resting O2 (taken as the first 30-s average O2 of the last minute before the start of exercise); tdp, τp, Ap represent the time delay, the time constant and the amplitude of the primary component, respectively; and tdsc, τsc, Asc, represent the equivalent parameters for the slow component. Ap was used to determine the gain (Gp = Ap/P) of the primary component in cycling and arm cranking exercise.
Because the asymptotic value of the second function is not necessarily reached at the end of the exercise, the amplitude of the O2 slow component was defined as:
where te was the time at the end of the exercise bout.
To estimate O2 kinetics parameters, equations were fitted to the exercise data (Microsoft Excel Solver) using an iterative procedure (Generalized Reduced Gradient), by minimizing the sum of the mean squares of the differences between modeled and measured O2 values. The relative contribution of the slow component to the net increase in O2 (%Asc') was calculated as the ratio between the amplitude of Asc' and the total increase of O2 during exercise. Effort intensity perception was rated recorded using the Borg 6–20 RPE Scale during all maximal incremental tests.
Statistical Analysis
All values are reported as mean ± standard deviation (SD). After analysis of normality (Kolmogorov-Smirnov test) and homogeneity of variance (Levene test) of the data, analysis of variance with repeated measures (RM-ANOVA) was used to compare the values from the three incremental tests (swimming, arm cranking, and cycling), as well as the O2 kinetics parameters obtained from the three square-wave transition from rest to heavy-intensity exercise. Significant main effects were subsequently analyzed using the Student-Newman-Keuls post-hoc test. Before the analysis, significance level was set at 5%.
The bootstrap method was used in the present study to assess the coefficient of variation (CV%) of the model parameters. It consists in resampling the original data set with replacement to create a number of “bootstrap replicate” data sets of the same size as the original data set. A random number generator to determine which data of the original data set will be included in a replicate data set was used. This was repeated 1,000 times, and the parameters estimated were retained. The CV of these 1,000 replicates was calculated.
Results
Maximal Incremental Exercise
The maximal workload reached at exhaustion was 353 ± 42 W in cycling, 142 ± 22 W in arm cranking, and 1.214 ± 0.064 m·s−1 in swimming. Both weight-related and absolute O2peak were higher in cycling (65.6 ± 4.0 ml·kg−1·min−1 and 4.7 ± 0.6 l.min−1) than in arm cranking (48.7 ± 8.0 ml·kg−1·min−1 and 3.4 ± 0.4 l.min−1; P < 0.001, 0.007, respectively) and swimming (53.0 ± 6.7 ml·kg−1·min−1 and 3.8 ± 0.3 l.min−1; P < 0.001, 0.024, respectively). With the exception of weight-related O2peak arm cranking-swimming (r = 0.77, P = 0.026), no correlations were found for cycling-arm cranking and cycling-swimming (r = 0.40 and 0.36; P = 0.24 and 0.31). Absolute O2peak values (r = −0.12, 0.25, and 0.23; P = 0.77, 0.48 and 0.23, respectively) for cycling-arm cranking, cycling-swimming, and arm cranking-swimming were not correlated. No differences were found in maximal HR values (188 ± 7, 179 ± 8 and 175 ± 9 bpm; P > 0.05) or RPE (18.3 ± 0.6, 18.6 ± 0.6 and 17.3 ± 0.9 a.u.; P > 0.05), for cycling, arm cranking and swimming, respectively.
O2 Kinetics during Heavy-Intensity Exercise
The average workload during the submaximal 6-min bouts was 266 ± 37 W in cycling, 98 ± 18 W in arm cranking, and 1.044 ± 0.048 m·s−1 in swimming, corresponding to 75 ± 2, 70 ± 6, and 86 ± 4 percent of maximal workload (but to 76 ± 2, 69 ± 6, and 71 ± 4 percent of O2peak) for cycling, arm cranking and swimming, respectively. O2peak attained in these submaximal tests was higher in cycling (55.2 ± 5.9 ml·kg−1·min−1) than in arm cranking, or swimming (31.9 ± 5.6 and 41.5 ± 5.5 ml·kg−1·min−1, respectively; P < 0.001 and 0.001 respectively), but values were not correlated (r = 0.40, 0.36, and 0.25 for cycling-arm cranking, cycling-swimming, and arm cranking-swimming, P = 0.40, 0.36, and 0.29, respectively).
Figure 2 shows representative breath-by-breath and best-fit O2 kinetics curves for the three exercise modes, expressed both in weight-related O2 values (A), and in percentage of O2 at the end of the exercise (B).
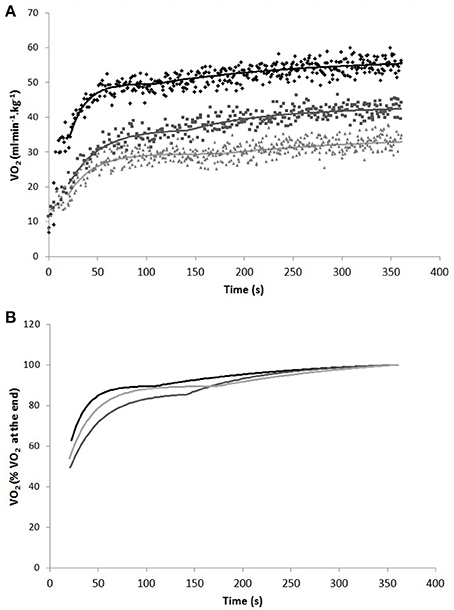
Figure 2. Representative breath-by-breath and best fit O2 kinetics curves in weight-related O2 values (A) and best fit O2 kinetics curves in percentage of O2 at the end of the exercise (B) in one subject during cycling (black), swimming (dark gray), and arm cranking (light gray). To remove the influence of the cardiodynamic phase on the subsequent response, the first 20 s of data were excluded from the analysis.
Table 1 shows the estimated O2 kinetic parameters and the associated CV (%) during the 6-min square-wave transition from rest to heavy-intensity for all modes of exercise.
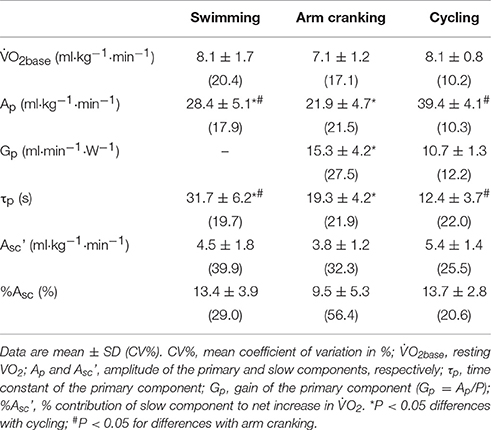
Table 1. Values for the estimated O2 kinetic parameters in the 6-min square-wave transition from rest to heavy-intensity (to Δ25%) for swimming, arm cranking and cycling).
O2base was not different between modes of exercise, but Ap was lower in both arm cranking and swimming compared with cycling (P < 0.001, for both), but higher in swimming compared with arm cranking (P < 0.005). Contrarily, gain was higher in arm cranking compared with cycling (P < 0.05). During swimming, subjects exhibited slower O2 kinetics compared with arm cranking (P < 0.05) and cycling (P < 0.001), but faster during cycling compared with arm cranking (P < 0.05). The Asc and Asc' values were not different among exercise modes.
Discussion
This study compared the on-transient O2 kinetics response during swimming, arm cranking and cycling in 6-min square-wave transition from rest to heavy-intensity. The novelty of our approach lies in that the same group of triathletes—whose training involves swimming, cycling and running, and thus the musculature of all body segments— exercised in the three modalities at comparable levels of metabolic intensity (i.e., 25% between VT and O2peak). Albeit exercising at a similar relative workload and for an equal duration, (i) the O2 kinetics was faster in cycling, followed by arm cranking and swimming; (ii) the amplitude of the fast component was largest in cycling, followed by swimming and arm cranking; and (iii) the gain of the primary component was greater in arm cranking compared with cycling.
O2peak and Related Parameters
The mean O2peak values observed are in accordance with data reported previously for swimmers (Rodríguez, 2000), however higher than healthy subjects familiarized with arm cranking exercise (Bernasconi et al., 2006). As would be expected, O2peak in cycling was 26 and 19% higher than in arm cranking and swimming, respectively, in line with previous studies. Sawka (1986), in a review of 18 studies, reported an average O2peak during arm cranking corresponding to ~22% of O2peak during cycling. Two recent studies with well-trained triathletes also reported average O2peak values during cycling that were 22% (Roels et al., 2005) and 36% (Barrero et al., 2014) higher compared with those in swimming. The smaller skeletal muscle mass involved during (mostly but not exclusively) upper body exercise is likely to be the main contributor to this ~20–35% lower O2peak. Consequently, cardiorespiratory responses to swimming exercise will be influenced, as well, by other factors, such as HRmax and E (Roels et al., 2005), which have been related with lower O2peak measured in triathletes while swimming compared with cycling (Roels et al., 2005; Barrero et al., 2014) and running (Barrero et al., 2014). Although HRmax was not significantly different in our study, a trend for lower values in swimming (~175 bpm) and arm cranking (~179 bpm) compared with cycling (~188 bpm) was observed. Interestingly, in a study comparing trained swimmers and triathletes, the former exhibited 12% higher O2peak in swimming than in cycling, whereas the opposite was found in the triathletes (22% higher O2peak in cycling), highlighting the relevance of specific training (Roels et al., 2005). Therefore, both central and peripheral factors seem to interplay in determining the O2peak attained during maximal exercise. Not withstanding the similar relative intensity performed during the heavy-intensity bouts, the subjects were also capable of performing well in the different exercise modes, and were similarly well trained in cycling and swimming;, therefore, the absence of differences in-between their O2peak (~76, 69, and 71% in cycling, arm cranking and swimming, respectively) were observed.
O2 Primary Component Response
Within the heavy intensity exercise domain, τp reflects the rate at which the O2 response achieves the steady state before the appearance of the “excess” O2 of the slow component phase. Previous studies have reported a slower adjustment of O2 in arm exercise compared with leg exercise occurs, either when expressed as the half-time for the initial adaptation of O2 at exercise onset (Cerretelli et al., 1977), or as the mean response time for the overall response (Koga et al., 1996; Koppo et al., 2002), as long as the power output does equally elevate blood lactate (Casaburi et al., 1992). This difference in the kinetics was corroborated with the present results; i.e., arm cranking and swimming showing a substantially slower adjustment in O2, i.e. higher τp, compared with cycling exercise (~19 and 32 vs. ~12 s, respectively). When comparing highly trained subjects of four sport modalities exercising in a time-to-exhaustion test at 100% of O2peak, Sousa et al. (2015) also found slower O2 kinetics for swimming (~21 s) compared with cycling (~16 s), rowing (~12 s), and running (~10 s). The faster rate of O2 increase compared with the present study can be attributed to the dissimilar intensity and duration of the exercise (i.e., ~3.7 min at 100% O2max vs. 6 min of heavy intensity exercise). Similarly, Rodríguez et al. (2015), in a recent study with swimmers comparing 100-m all-out arm stroke, leg kick and whole stroke swims, showed also slower O2 kinetics in arm exercise (~12 s) compared with whole stroke (~9 s) and leg kicking exercise (~10 s). Testing physical education students who practiced a variety of sports, including those that predominantly utilize the upper body muscles, Koppo et al. (2002) also showed slower O2 kinetics for arm cranking exercise, compared with 6-min high intensity cycling exercise. Therefore, this study extends previous findings by corroborating that the O2 kinetics pattern in-between arm and leg exercises is different, even with trained triathletes performing well in these exercise modes—and not only with physically active or highly trained subjects not specifically and concomitantly trained in these modes—are tested.
This new feature of our study may help to explain the possible mechanisms responsible for the slower O2 kinetics in arm exercise. It was previously suggested by Casaburi et al. (1992) and Koga et al. (1996) that such a slower response during arm exercise could be due to the lower training status of the upper-body muscles compared with the lower-body muscles, since the untrained subjects tested in both studies could have had a higher conditioning status in these than those of the upper limbs. Collectively, this and the above cited studies suggest that the “training status” of the subject's musculature seems not to be, or at least, not to a great extent, determinant in the slower O2 kinetics of the upper vs. the lower body exercise at heavy intensity. However, it should be taking into account that although the present study was conducted with triathletes capable of performing well in different modes of exercise, arm cranking involves a distinct muscle recruitment pattern. This latter could, in some extend, reflect a different movement efficiency, and therefore, influence O2 kinetics.
Another possible explanation for the modality dependence of O2 slower kinetics could rely on the skeletal muscle fiber type found in the upper and lower body. On the one hand, this is supported by the fact that upper-arm muscles contain generally a higher proportion of type II fibers —e.g., in tennis players (Sanchis-Moysi et al., 2010) or cross-country skiers (Mygind, 1995)—, the proportions of type II fibers was shown to be higher in the triceps brachii compared to vastus lateralis muscles. On the other hand, it has also been reported that the percentage of type I fibers in the gastrocnemius, vastus lateralis, and deltoid was high in all, but not different among muscles, while the muscle respiratory capacity (O2) and mean citrate synthase activity of the deltoid were lower than the gastrocnemius (Flynn et al., 1987). Type II fibers are metabolically less efficient, and not only the time constant of O2 primary component is significantly longer in upper-body muscles with a predominance of type II fibers (Kushmerick et al., 1992), but also is longer in subjects with a high proportion of type II fibers in the vastus lateralis (Pringle et al., 2002). Muraki et al. (2004), by studying triceps brachii muscle oxygen saturation using NIRS during arm cranking and cycling exercise in young women, noted a faster increase in the respiratory exchange ratio and a lower VT in arm compared with leg exercise, suggesting accelerated anaerobic glycolysis. They also concluded that the oxidative capacity of the arm muscles was limited due to early O2 utilization rather than poor O2 supply to the exercising musculature. The influence of fiber types composition and O2 primary component was previously addressed by Doria et al. (2011) in an elegant study, where the authors showed that a prolonged and active sojourn in hypoxia promoted an increase in the expression of slow isoforms of both heavy and light myosin subunits of vastus lateralis muscle in mountaineers. Moreover, this shift in muscle fiber type was accompanied by a decrease in mean response time of O2 kinetics at the onset of step submaximal cycling exercise. Therefore, in swimming exercise, the larger recruitment of type II muscle fibers could also have contributed to the slower O2 kinetics observed.
The fiber type controlling mechanism was also previously investigated within animal models. In a study with rats, muscles comprised of a high proportion of type II fibers have been shown to optimally increase their fractional O2 extraction during intensive exercise compared with predominantly slow-twitch muscles, which may be important for ensuring high blood-myocyte O2 flux and, therefore, a greater oxidative contribution to energetic requirements (McDonough et al., 2005). Moreover, for the same power output, “steady-state” O2 was reported to be larger in arm than in leg exercise, implying the mechanical efficiency to be lower during arm exercise (Vokac et al., 1975). Of interest are the reports that in vitro O2 on-kinetics of single muscle fibers at high intensity differ between high and intermediate oxidative muscle fiber with high oxidative muscle fibers yielding shorter time constant values than intermediate muscle fibers (30 vs. 50 s, respectively) during stimulated isometric contractions (Wüst et al., 2013). This difference seems to be in the same order of magnitude as that shown between arm cranking and cycling in the present study. Although one cannot infer full-body exercise responses from results on isolated fiber, this indirectly supports the speculation that fiber type differences underlie the differences in fast component between the different exercise modes. Collectively, these studies point out that one likely explanation for the slower O2 kinetics in arm cranking and swimming heavy intensity exercise, compared with cycling, is the greater recruitment of type II muscle fibers in the upper-body musculature.
Another potential explanation for the slower rate of O2 increase during swimming is related to the horizontal body position adopted during exercise. In the supine position, the blood flow to the working leg muscles is reduced, presumably as a consequence of lower arterial pressure in the legs when the effect of gravity (i.e., hydrostatic gradient effect) is removed (Koga et al., 1999). The same authors, Koga et al. (1999) reported that heavy exercise in the supine position was associated with a reduced amplitude of the fast component, which may be due, at least in part, to an attenuated early rise in HR in the supine position. The resultant fall in perfusion pressure to the active muscles leads to reduced exercise tolerance and slower O2 kinetics compared to upright exercise (Hughson et al., 1991). Results from MacDonald et al. (1998), who found that slower O2 kinetics at the onset of knee extension and flexion exercise in the supine compared with the upright position was accompanied by a slower increase of lower femoral artery blood flow, seem to support this hypothesis. In addition, the inability to produce maximal muscle contractions due to environmental constraints —i.e., body posture and propulsion is achieved by applying forces in a fluid medium— could also have limited the rate of increase in O2 during swimming (Sousa et al., 2015). Other possible contributing mechanisms could be the involvement of static muscle actions for trunk and leg stabilization and differences in the muscular action regime (i.e., concentric vs. eccentric exercise), whether by themselves or related to fiber type recruitment or decreased muscle perfusion to the working muscles (see previous discussion).
Other differences were found in the fast component response: (i) the amplitude in cycling was ~37% larger than in swimming and ~50% greater than in arm cranking, which is proportional to the greater O2peak in cycling compared with swimming (~20%) and arm cranking (~25%); and (ii) the gain was higher in arm cranking (~15 ml·min−1·W−1) compared with cycling (~11 ml·min−1·W−1). This latter trend corroborates previous findings for high intensity exercise, although the values reported in our study are slightly higher (Koppo et al., 2002). These findings are consistent with the hypothesis of a greater recruitment of type II fibers during arm cranking and swimming than during cycling exercise. In fact, Bernasconi et al. (2006) documented an increase in the electromyography signal of biceps brachii, triceps brachii, anterior deltoid, and infraspinatus muscles during heavy arm cranking exercise. In addition, Pringle et al. (2002) reported that Gp during heavy cycle exercise was positively correlated with the percentage of type I fibers in the vastus lateralis. Therefore, differences in fiber type composition between the muscles of the upper and lower body play an important role in the fast component gain.
O2 Slow Component Response
No significant differences were found in the O2sc amplitude (absolute and relative) or the time constant in-between modes of exercise, which does not corroborate previous reports conducted in cycling and arm cranking exercise (Koga et al., 1999). Multiple putative mechanisms of the O2sc phenomenon have been postulated (Poole and Jones, 2012), the discussion of which exceeds the scope of this paper. However, in a recent work where a computer model of the skeletal muscle bioenergetic system was used, Korzeniewski and Zoladz (2015) postulated that the generation of O2sc during heavy exercise could be related with: (i) the progressive inhibition of anaerobic glycolysis by accumulating protons (together with a slow decrease of the net creatine kinase reaction rate), (ii) the gradual increase of ATP usage during exercise, and (iii) perhaps, a decrease in the P/O ratio (ATP molecules/O2 molecules). It follows that a greater recruitment of type II muscle fibers would theoretically have an effect on O2sc. Koga et al. (1999) reported that heavy exercise in the supine position was associated with a greater amplitude of O2sc but also, concomitantly, with a reduced amplitude of the fast component; this latter effect may be due, at least in part, to an attenuated early rise in HR in the supine position. These and other data seem to indicate that the amplitudes of the primary and slow components are sensitive to changes in muscle blood flow, which may influence, in turn, motor unit recruitment patterns. However, assuming that our subjects were equally well trained in all modes of exercise, we interpreted our results to indicate that either an equivalent anaerobic energy contribution to muscle metabolism, or that a similar progressive loss of muscle contractile efficiency—associated with the development of fatigue—occurred in the three exercise modalities, not measurably affecting the amplitude of the fast or slow components.
A limitation of the present study should be acknowledged, as the athletes performed only one transition to heavy exercise in each of the three modalities. Despite the number of transitions necessary for an adequate confidence in model parameter estimates is not clearly defined, it should be recognized that three (Spencer et al., 2011) or more exercise transitions might have improved such confidence. However, in endurance trained subjects, the number of repetitions may be reduced due to higher O2max and lactate threshold, as suggested by Koga et al. (2005). Moreover, since we did not perform muscle biopsies on the subjects, it is impossible to determine the exact fiber type composition or phenotype of the arm vs. legs muscles. We agree that knowing the fiber type distribution and the oxidative capacity (e.g., mitochondrial content/efficiency, CS activity) would help clarifying some above-speculated mechanistic differences between the three activities modes used in this study.
In conclusion, this study provides further evidence of meaningful differences in the O2 kinetics across exercise modalities, noted in the same group of athletes similarly trained in-between them. Specifically, (i) O2peak in cycling was ~26 and ~19% higher than in arm cranking and swimming, respectively, mainly attributable to the smaller skeletal muscle mass involved during upper body exercise; (ii) albeit the same trained athletes performed heavy intensity cycling, arm cranking and swimming exercises at a similar relative intensity, substantially slower O2 kinetics in swimming (~31 s) and arm cranking (~19 s) compared with cycling exercise (~12 s) was observed; (iii) also the amplitude of the primary component in cycling was ~37% larger than in swimming and ~50% greater than in arm cranking, in proportion to the greater O2peak in cycling compared with swimming, although muscular efficiency (i.e., Gp) was higher in arm cranking compared with cycling; (iv) although the slow component was evident in all exercise modes, unlike in previous studies, no significant differences were noted in the amplitude of the slow component (absolute and relative); and (v) overall, it is suggested that the muscle mass involved, the greater and/or faster recruitment of type II muscle fibers during upper body exercise, the dynamics of fatigue onset, the horizontal position adopted during swimming, differences in muscle perfusion, and the involvement of trunk and lower-body stabilizing muscles are plausible explanations for the differences found in the O2 kinetics patterns during heavy exercise, independently of the “training status” of the subjects.
Author Contributions
FB and GM contributed to the conception or design of the work and the acquisition of data. AS and FR contributed to data analysis and interpretation. AS, FB, FR, and GM contributed to the drafting of the manuscript or revising it critically for important intellectual content. AS, FB, FR, and GM contributed to the final approval of the version to be published. AS, FB, FR, and GM contributed to the agreement to be accountable for all aspects of the work in ensuring that questions related to the accuracy or integrity of any part of the work are appropriately investigated and resolved.
Conflict of Interest Statement
The authors declare that the research was conducted in the absence of any commercial or financial relationships that could be construed as a potential conflict of interest.
Acknowledgments
The authors would like to thank Belle Roels for her outstanding technical support.
References
Barrero, A., Chaverri, D., Erola, P., Iglesias, X., and Rodríguez, F. A. (2014). Intensity profile during an ultra-endurance triathlon in relation to testing and performance. Int. J. Sports Med. 35, 1170–1178. doi: 10.1055/s-0034-1374601
Bentley, D., Roels, B., Hellard, P., Fauquet, C., Libicz, S., and Millet, G. (2005). Physiological responses during submaximal interval swimming training: effects of interval duration. J. Sci. Med. Sport 8, 392–402. doi: 10.1016/S1440-2440(05)80054-4
Bernasconi, S., Tordi, N., Perrey, S., Parratte, B., and Monnier, G. (2006). Is the O2 slow component in heavy arm-cranking exercise associated with recruitment of type II muscle fibres as assessed by an increase in surface EMG? Appl. Physiol. Nut. Metabol. 31, 414–422. doi: 10.1139/h06-021
Caputo, F., and Denadai, B. S. (2004). Effects of aerobic endurance training status and specificity on oxygen uptake kinetics during maximal exercise. Eur. J. Appl. Physiol. 93, 87–95. doi: 10.1007/s00421-004-1169-3
Carter, H., Jones, A. M., Barstow, T. J., Burnley, M., Williams, C. A., and Doust, J. H. (2000). Oxygen uptake kinetics in treadmill running and cycle ergometry: a comparison. J. Appl. Physiol. 89, 899–907. Available online at: http://jap.physiology.org/content/89/3/899
Casaburi, R., Barstow, T. J., Robinson, T., and Wasserman, K. (1992). Dynamic and steady-state ventilatory and gas exchange responses to arm exercise. Med. Sci. Sports Exerc. 24, 1365–1374. doi: 10.1249/00005768-199212000-00010
Cerretelli, P., Shindell, D., Pendergast, D., Prampero, P., and Rennie, D. (1977). Oxygen uptake transients at the onset and offset of arm and leg work. Respir. Physiol. 30, 81–97. doi: 10.1016/0034-5687(77)90023-8
Doria, C., Toniolo, L., Verratti, V., Cancellara, P., Pietrangelo, T., Marconi, V., et al. (2011). Improved O2 uptake kinetics and shift in muscle fiber type in high-altitude trekkers. J. Appl. Physiol. 111, 1597–1605. doi: 10.1152/japplphysiol.01439.2010
Drescher, U., Koschate, J., and Hoffmann, U. (2015). Oxygen uptake and heart rate kinetics during dynamic upper and lower body exercise: an investigation by time-series analysis. Eur. J. Appl. Physiol. 115, 1665–1672. doi: 10.1007/s00421-015-3146-4
Flynn, M. G., Costill, D. L., Kirwan, J. P., Fink, W. J., and Dengel, D. R. (1987). Muscle fiber composition and respiratory capacity in triathletes. Int. J. Sports Med. 8, 383–386. doi: 10.1055/s-2008-1025690
Grassi, B., Poole, D. C., Richardson, R. S., Knight, D. R., Erickson, B. K., and Wagner, P. D. (1996). Muscle O2 uptake kinetics in humans: implications for metabolic control. J. Appl. Physiol. 80, 988–998.
Hill, D. W., Halcomb, J. N., and Stevens, E. C. (2003). Oxygen uptake kinetics during severe intensity running and cycling. Eur. J. Appl. Physiol. 89, 612–618. doi: 10.1007/s00421-002-0779-x
Hughson, R. L., Xing, H. C., Borkhoff, C., and Butler, G. C. (1991). Kinetics of ventilation and gas exchange during supine and upright cycle exercise. Eur. J. Appl. Physiol. Occup. Physiol. 63, 300–307. doi: 10.1007/BF00233866
Koga, S., Shiojiri, T., and Kondo, N. (2005). “Measuring O2 kinetics: the practicalities,” in Oxygen Uptake Kinetics in Sport, Exercise and Medicine, eds A. M. Jones and D. C. Poole (Oxon: Routledge Poole), 39–61.
Koga, S., Shiojiri, T., Shibasaki, M., Fukuba, Y., Fukuoka, Y., and Kondo, N. (1996). Kinetics of oxygen uptake and cardiac output at onset of arm exercise. Respir. Physiol. 103, 195–202. doi: 10.1016/0034-5687(95)00082-8
Koga, S., Shiojiri, T., Shibasaki, M., Kondo, N., Fukuba, Y., and Barstow, T. J. (1999). Kinetics of oxygen uptake during supine and upright heavy exercise. J. Appl. Physiol. 87, 253–260.
Koppo, K., Bouckaert, J., and Jones, A. M. (2002). Oxygen uptake kinetics during high-intensity arm and leg exercise. Respir. Physiol. Neurobiol. 133, 241–250. doi: 10.1016/S1569-9048(02)00184-2
Koppo, K., Bouckaert, J., and Jones, A. M. (2004). Effects of training status and exercise intensity on phase II O2 kinetics. Med. Sci. Sports Exerc. 36, 225–232. doi: 10.1249/01.MSS.0000113473.48220.20
Korzeniewski, B., and Zoladz, J. A. (2015). Possible mechanisms underlying slow component of O2 on-kinetics in skeletal muscle. J. Appl. Physiol. 118, 1240–1249. doi: 10.1152/japplphysiol.00027.2015
Kushmerick, M. J., Meyer, R. A., and Brown, T. R. (1992). Regulation of oxygen consumption in fast-and slow-twitch muscle. Am. J. Physiol. Cell Physiol. 263, C598–C606.
Libicz, S., Roels, B., and Millet, G. (2005). O2 responses to intermittent swimming sets at velocity associated with O2max. Can. J. Appl. Physiol. 30, 543–553. doi: 10.1139/h05-140
MacDonald, M. J., Shoemaker, J. K., Tschakovsky, M. E., and Hughson, R. L. (1998). Alveolar oxygen uptake and femoral artery blood flow dynamics in upright and supine leg exercise in humans. Eur. J. Appl. Physiol. 85, 1622–1628.
McDonough, P., Behnke, B. J., Padilla, D. J., Musch, T. I., and Poole, D. C. (2005). Control of microvascular oxygen pressures in rat muscles comprised of different fibre types. J. Physiol. 563(Pt 3), 903–913. doi: 10.1113/jphysiol.2004.079533
Millet, G. P., Candau, R. B., Barbier, B., Busso, T., Rouillon, J. D., and Chatard, J. C. (2002). Modelling the transfers of training effects on performance in elite triathletes. Int. J. Sports Med. 23, 55–63. doi: 10.1055/s-2002-19276
Muraki, S., Tsunawake, N., and Yamasaki, M. (2004). Limitation of muscle deoxygenation in the triceps during incremental arm cranking in women. Eur. J. Apl. Physiol. 91, 246–252. doi: 10.1007/s00421-003-0962-8
Mygind, E. (1995). Fibre characteristics and enzyme levels of arm and leg muscles in elite cross-country skiers. Scand. J. Med. Sci. Sports 5, 76–80. doi: 10.1111/j.1600-0838.1995.tb00016.x
Poole, D. C., and Jones, A. M. (2012). Oxygen uptake kinetics. Compr. Physiol. 2, 933–996. doi: 10.1002/cphy.c100072
Pringle, J., Doust, J., Carter, H., Campbell, I., Tolfrey, K., and Jones, A. (2002). Influence of muscle fibre type on oxygen uptake kinetics during submaximal cycle exercise in humans. J. Physiol. 539, 55–56.
Robergs, R., Dwyer, D., and Astorino, T. (2010). Recommendations for improved data processing from expired gas analysis indirect calorimetry. Sports Med. 40, 95–111. doi: 10.2165/11319670-000000000-00000
Rodríguez, F. A. (2000). Maximal oxygen uptake and cardiorespiratory response to maximal 400-m free swimming, running and cycling tests in competitive swimmers. J. Sport Med. Phys. Fit 40, 87–95. Available online at: http://europepmc.org/abstract/MED/11034427
Rodríguez, F. A., Keskinen, K. L., Kusch, M., and Hoffmann, U. (2008). Validity of a swimming snorkel for metabolic testing. Int. J. Sports Med. 29, 120–128. doi: 10.1055/s-2007-964973
Rodríguez, F. A., Lätt, E., Jürimäe, J., Mäestu, J., Purge, P., Rämson, R., et al. (2015). O2 kinetics in all-out arm stroke, leg kick and whole stroke front crawl 100-m swimming. Int. J. Sports Med. 37, 191–196 doi: 10.1055/s-0035-1554695
Roels, B., Schmitt, L., Libicz, S., Bentley, D., Richalet, J. P., and Millet, G. (2005). Specificity of O2max and the ventilatory threshold in free swimming and cycle ergometry: comparison between triathletes and swimmers. Br. J. Sports Med. 39, 965–968. doi: 10.1136/bjsm.2005.020404
Rossiter, H., Ward, S., Doyle, V., Howe, F., Griffiths, J., and Whipp, B. (1999). Inferences from pulmonary O2 uptake with respect to intramuscular [phosphocreatine] kinetics during moderate exercise in humans. J. Physiol. 518, 921–932. doi: 10.1111/j.1469-7793.1999.0921p.x
Saltin, B., Rådegran, G., Koskolou, M., and Roach, R. (1998). Skeletal muscle blood flow in humans and its regulation during exercise. Acta Physiol. Scand. 162, 421–436. doi: 10.1046/j.1365-201X.1998.0293e.x
Sanchis-Moysi, J., Idoate, F., Olmedillas, H., Guadalupe-Grau, A., Alayon, S., Carreras, A., et al. (2010). The upper extremity of the professional tennis player: muscle volumes, fiber-type distribution and muscle strength. Scand. J. Med. Sci. Sports 20, 524–534. doi: 10.1111/j.1600-0838.2009.00969.x
Sawka, M. N. (1986). Physiology of upper body exercise. Exerc. Sport Sci. Rev. 14, 175–211. doi: 10.1249/00003677-198600140-00009
Sousa, A., Figueiredo, P., Zamparo, P., Pyne, D. B., Vilas-Boas, J. P., and Fernandes, R. J. (2015). Exercise modality effect on bioenergetical performance at O2max intensity. Med. Sci. Sports Exerc. 47, 1705–1713. doi: 10.1249/MSS.0000000000000580
Spencer, M. D., Murias, J. M., Lamb, H. P., Kowalchuk, J. M., and Paterson, D. H. (2011). Are the parameters of O2, heart rate and muscle deoxygenation kinetics affected by serial moderate-intensity exercise transitions in a single day? Eur. J. Appl. Physiol. 111, 591–600. doi: 10.1007/s00421-010-1653-x
Vokac, Z., Bell, H., Bautz-Holter, E., and Rodahl, K. (1975). Oxygen uptake/heart rate relationship in leg and arm exercise, sitting and standing. J. Appl. Physiol. 39, 54–59.
Keywords: exercise modes, triathletes, kinetics, gas exchange, modeling
Citation: Sousa A, Borrani F, Rodríguez FA and Millet GP (2017) Oxygen Uptake Kinetics Is Slower in Swimming Than Arm Cranking and Cycling during Heavy Intensity. Front. Physiol. 8:639. doi: 10.3389/fphys.2017.00639
Received: 03 April 2017; Accepted: 15 August 2017;
Published: 01 September 2017.
Edited by:
Giuseppe D'Antona, University of Pavia, ItalyReviewed by:
Stefanos Volianitis, Aalborg University, DenmarkRichard Jaspers, VU University Amsterdam, Netherlands
Copyright © 2017 Sousa, Borrani, Rodríguez and Millet. This is an open-access article distributed under the terms of the Creative Commons Attribution License (CC BY). The use, distribution or reproduction in other forums is permitted, provided the original author(s) or licensor are credited and that the original publication in this journal is cited, in accordance with accepted academic practice. No use, distribution or reproduction is permitted which does not comply with these terms.
*Correspondence: Grégoire P. Millet, gregoire.millet@unil.ch