- 1Department of Reproductive Medicine, Department of Prenatal Diagnosis, Changchun, China
- 2Hand Surgery Department, Changchun, China
- 3Department of Clinical Laboratory, Changchun, China
- 4Department of Breast Surgery, Changchun, China
- 5Public Research Platform, The First Hospital of Jilin University, Jilin, China
Premature ovarian failure (POF), or premature ovarian insufficiency (POI), is a multifactorial and heterogeneous disease characterized by amenorrhea, decreased estrogen levels and increased female gonadotropin levels. The incidence of POF is increasing annually, and POF has become one of the main causes of infertility in women of childbearing age. The etiology and pathogenesis of POF are complex and have not yet been clearly elucidated. In addition to genetic factors, an increasing number of studies have revealed that epigenetic changes play an important role in the occurrence and development of POF. However, we found that very few papers have summarized epigenetic variations in POF, and a systematic analysis of this topic is therefore necessary. In this article, by reviewing and analyzing the most relevant literature in this research field, we expound on the relationship between DNA methylation, histone modification and non-coding RNA expression and the development of POF. We also analyzed how environmental factors affect POF through epigenetic modulation. Additionally, we discuss potential epigenetic biomarkers and epigenetic treatment targets for POF. We anticipate that our paper may provide new therapeutic clues for improving ovarian function and maintaining fertility in POF patients.
Introduction
Premature ovarian failure (POF) is a reproductive endocrine disease that occurs before the age of 40 in women. POF is the main cause of female infertility and is characterized by increased gonadotropin levels and decreased estrogen levels, accompanied by primary or secondary amenorrhea. POF is highly heterogeneous, and abnormal follicular development at all stages may lead to POF (Collins et al., 2017). Approximately 1% of women under 40% and .1% of women under 30 are estimated to suffer from POF (Coulam et al., 1986; Barbarino-Monnier 2000). POF has become a serious problem that affects the reproductive health of women of reproductive age. The occurrence of POF may be related to various factors, such as an insufficient primordial follicle pool reserve, accelerated follicular atresia, changes in dominant follicle recruitment, and follicular maturation disorders (Webber et al., 2016). The etiology of POF is mostly idiopathic (approximately 80%), and the other causes of POF involve genetic factors, iatrogenic diseases, autoimmune and endocrine diseases, mitochondrial dysfunction, infection and environmental factors (Vujovic 2009). Approximately 20%–25% of POF cases are caused by genetic abnormalities (Qin et al., 2015). The genetic factors conclusively discovered in POF patients to date include autosomal and X chromosome abnormalities and variants in POF candidate genes such as follicle-stimulating hormone receptor (FSHR), newborn ovary homeobox gene (NOBOX), forkhead box L2/O3 (FOXL2/FOX O 3), spermatogenesis- and oogenesis-specific basic helix-loop-helix 1 (SOHLH1), folliculogenesis-specific BHLH transcription factor (FIGLA), growth differentiation factor 9 (GDF9) and bone morphogenetic protein 15 (BMP15). In addition to these causative genes, variations in epigenetic and epigenetic regulators also add complexity to the etiology of POF. With the assistance of new technologies represented by next-generation sequencing (NGS), new causative genes, such as non-coding RNAs, have been identified, and researchers have proposed an epigenetic explanation for POF (Jiao et al., 2018).
Epigenetic modification is an indispensable part of cell differentiation, development and activity maintenance (Skvortsova et al., 2018). Epigenetic hallmarks, such as DNA methylation, histone modification and non-coding RNA regulation, may alter chromatin structure without changing the DNA sequence, conferring a differential program of gene expression (Lee et al., 2014) (Figure 1). Since the epigenome of cells is highly plastic and reprogrammable, epigenetic modifications may dynamically and reversibly control gene expression. Epigenetic reprogramming can change cell fate throughout the developmental phase and adulthood, and epigenetic regulation is also an important molecular mechanism by which organisms respond to external environmental factors (Safi-Stibler and Gabory 2020). Epigenetics are closely associated with many diseases, including various developmental disorders, such as Beckwith-Wiedemann, Silver-Russell and Fragile X syndromes (Inoue et al., 2020; Nobile et al., 2021; Tüysüz et al., 2021), and complex and multifactorial diseases, such as cancer, diabetes and obesity (Nebbioso et al., 2018; Ling and Rönn 2019). An increasing numbers of studies has revealed that epigenetic changes are a universal phenomenon in the occurrence and development of POF (Figure 2). In this article, we will provide an overview of the epigenetic mechanism of POF and introduce some potential epigenetic biomarkers and epigenetic treatment targets for POF. Our aim is to provide new therapeutic clues for improving ovarian function and preserving fertility in POF patients.
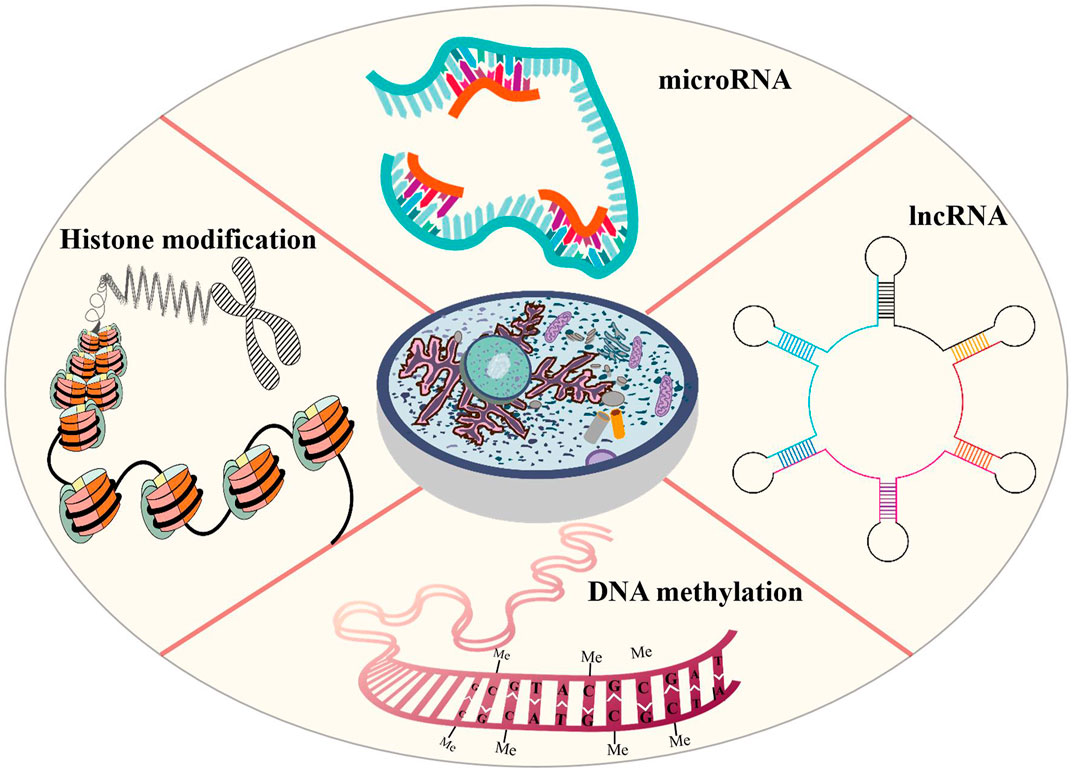
FIGURE 1. Epigenetic modifications that are probably involved in POI includes DNA methylation, histone modification and non-coding RNA regulation. Altered DNA methylation may lead to gene silencing, reduce gene transcription levels, remodel chromatin and regulate important developmental processes. In POF patients, aberrant DNA methylation may induce GC apoptosis, reduce follicles number and change hormone expression. Histone modifications affect chromatin structure that are conducive to the expression or repression of target genes. Histone modifications may hinder oocyte development and maturation and reduce oocyte number. Non-coding RNAs control gene expression by binding to DNA or RNA sequences and proteins. In POF patients, the abnormal non-coding RNA expression may promote GC apoptosis and promote follicular atresia.
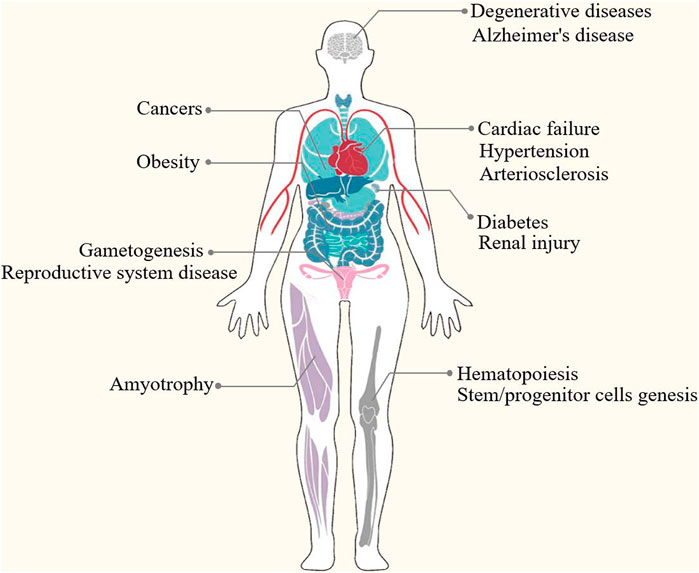
FIGURE 2. Epigenetics are closely associated with many diseases, such as developmental disorders (e.g., DNA methylation perturbations and loss-of-function mutations of imprinted genes in Beckwith-Wiedemann, Silver-Russell and Fragile X syndromes), complex and multifactorial diseases (e.g., DNA methylation, histone modifications, microRNAs and nucleosome remodeling in cancers, diabetes and obesity) and degenerative disease (e.g., epigenetic modifications in Alzheimer’s disease and amyotrophy).
Epigenetic mechanisms regulating the occurrence and development OF POF/POI
Epigenetic modifications play a crucial role in reproductive aging (Chamani and Keefe 2019; Li et al., 2021a). Under physiological conditions, epigenetic modification is indispensable in the development of germ cells and early embryos. Many studies have shown that abnormal epigenetic modifications, such as changes in methylation levels, histone modifications and non-coding RNA expression, occur in germ cells in the aging process.
The effect of DNA methylation on germ cell senescence
DNA methylation is one of the most typical epigenetic modifications and participates in regulating many life activities. Altered DNA methylation is a major epigenetic marker of epigenetic reprogramming during germ cell aging, which leads to gene silencing, reduces gene transcription levels, remodels chromatin, and subsequently regulates important developmental processes, including genomic imprinting and X chromosome inactivation (Das et al., 2009; Deaton and Bird 2011; Duncan et al., 2018; Marshall and Rivera 2018). Aberrant DNA methylation is a classic feature of mammalian aging (Bjornsson et al., 2008; Maegawa et al., 2010). Insufficiency of follicles in the primordial follicle pool is associated with primary POI, and epigenetic modification exerts a strong effect on controlling programmed oocyte death during the establishment of the primordial follicle pool (PF) (Sun et al., 2017; Sun et al., 2018). Liu et al. found that the methylation levels of GATCG sites in oocytes decreased from primary follicles to secondary follicles, while the methylation levels of CCGG and GATCG in ovarian granulosa cells (GCs) decreased significantly from primary to secondary follicles and then increased in tertiary follicles. Moreover, the authors observed marked demethylation of CCGG sites in terminal deoxynucleotidyl transferase dUTP nick-end labeling (TUNEL)-positive GCs, suggesting that failure of the follicular stage-dependent increase in CCGG and GATCG methylation may induce GC apoptosis and follicular atresia (Liu et al., 2018b). In addition, Yu et al. conducted mRNA-Seq and genome-wide DNA methylation studies in human ovarian GCs and found that compared with young healthy donors, older women with a natural age-related decline in ovarian function showed lower gene expression (1,809 genes were downregulated) that was correlated with higher gene body methylation and 3′-end GC density (Yu et al., 2015). According to Marshall et al., the expression level of the DNA methyltransferase Dnmt1 in oocytes increased in 69- to 70-week-old mice compared with 10- to 13-week-old mice, and the DNA and histone H3K9me2 methylation levels also increased (Marshall et al., 2018). However, Yue et al. found that the DNA methylation level of metaphase II (MII) oocytes in 35- to 40-week-old mice decreased compared with six- to 8 week-old mice, and the expression levels of Dnmt1, Dnmt3a, Dnmt3b, and Dnmt3L decreased (Yue et al., 2012).
Several genes associated with ovarian function are regulated by epigenetics, and their epigenetic variations may lead to POF. Anti-Mullerian hormone (AMH), a GC-rich gene, is a biomarker for diminished ovarian reserve (Moolhuijsen and Visser 2020). As shown in the study by Yu et al., AMH expression was strikingly downregulated in the poor responder group, and a partially methylated CpG island was identified close to its transcriptional end site (TES) (Yu et al., 2015). Gao et al. examined pigs and found that excess sodium fluoride (NaF) increased the DNA methylation level and downregulated the expression of the maternal imprinted gene NNAT (neuronatin), which affects glucose transport by inhibiting the phosphoinositide 3-kinase (PI3K)- AKT serine/threonine kinase 2 (Akt2) signaling pathway. Suppression of NNAT disrupts glucose metabolism in oocytes, hinders oocyte maturation, affects follicular development and reduces ovarian reserve (Gao et al., 2016; Liu et al., 2018c). Kordowitzki et al. studied the DNA methylation-based biomarkers of aging (epigenetic clocks) in bovine oocytes and blood to reveal the epigenetic mechanisms underlying the effects of aging on the female reproductive system, and they found that the rate of epigenetic aging was slower in oocytes than in blood, but oocytes appeared to begin aging at an older epigenetic age. Their findings suggested that epigenetic clocks for oocytes are promising markers to address questions of reproductive aging, including methods to slow the aging of oocytes (Kordowitzki et al., 2021). Guglielmino et al. observed significantly lower expression of TAp73 (encoded by the P73 gene, which belongs to the P53 family) in the oocytes of women over 38 years old than in women under 36 years old; they also indicated that the methylation level of CpG sites in the P73 promoter decreased in aging mouse oocytes, indicating that CpG hypomethylation may be involved in oocyte senescence (Guglielmino et al., 2011). Qian et al. measured the expression levels of 5-cytosine (5 mC) and ten-eleven-translocation (Tet)/thymine DNA glycosylase (Tdg) in mouse oocytes obtained under natural and accelerated aging conditions, and they found that the levels of intermediates produced by demethylation-modified cytosine (5mC, 5 hmC, 5 fC and 5caC) increased. Additionally, Tet expression levels increased and Tdg expression levels decreased. Notably, genomic DNA demethylation was more significant in chemically induced senescent oocytes (Qian et al., 2015).
DNA methylation also occurs at sites other than CpG, known as non-CpG methylation or asymmetric methylation. Yu et al. showed that human genomic CpG methylation is mostly stable during oocyte maturation, but non-CpG methylation increases in local genomic regions and gradually accumulates (Yu et al., 2017). This finding is consistent with conclusions drawn by Tomizawa, who reported that the methylation of non-CpG sites changes dynamically during the maturation of mouse oocytes (Tomizawa et al., 2011).
Female X -chromosome inactivation (XCI) is an important epigenetic mark and leads to differences in epigenetic marks on the active and inactive X -chromosome (Boumil and Lee 2001). The CpG island-containing promoters of genes subject to XCI are approximately 50% methylated in females (Cotton et al., 2011). XCI is a random process, and the number of cells expressing maternal and paternal chromosomes is 50:50. When XCI is not random, an imbalance of cells expressing either the paternal or maternal X–chromosome occurs, which is known as skewed X -chromosome inactivation (sXCI) (Liehr et al., 2018). Studies have suggested that POF correlates with sXCI. For example, Susana et al. suggested that sXCI and X -chromosome deletion may induce abnormal expression of some crucial ovarian development-associated genes and induce POF (Ferreira et al., 2010). Sato et al. also implied that sXCI disrupts the expression of genes involved in ovarian development (such as bone morphogenetic protein 15 (BMP15), a member of the transforming growth factor-β (TGF-β) superfamily that regulates follicular survival/atresia and oocyte maturation), which in turn causes ovarian dysfunction and subsequently results in POF (Sato et al., 2004). sXCI is commonly observed in diploid cell lines arising from trisomy rescue events (Peñaherrera et al., 2000). Oocytes may contain high levels of trisomic cells, and these cells are more likely to suffer early atresia, resulting in a reduced follicular pool size and the occurrence of primary amenorrhea (Bretherick et al., 2007). However, Silvia et al. analyzed 151 POF patients and found that the distribution of sXCI in the POF population was similar to that in the control population. They also suggested that small deletions or mutations in X-linked genes do not appear to be a common feature of POF patients and that X-linked genes involved in POF may be too few or are unable to interfere with XCI (Bione et al., 2006). Marian et al., Pu et al. and Sang et al. also reported that sXCI may not be associated with POF (Kline et al., 2006; Yoon et al., 2008; Pu et al., 2010; Spath et al., 2010).
DNA methylation, one of the most classic epigenetic regulation patterns, affects a variety of cellular behaviors by regulating gene transcription. Numerous studies have widely confirmed that DNA methylation contributes to various diseases, particularly degenerative diseases. However, all the literature we can find is focused on DNA methylation of germ cells (Table 1), and the large-scale detection of DNA methylation in the occurrence and development of POF and in-depth systematic functional research of POF have not been conducted.
The effect of histone modifications on germ cell senescence
Histone modifications are covalent posttranslational epigenetic modifications that alter the chromatin structure and subsequently regulate gene expression. Histones are responsible for packing DNA into small structures called nucleosomes within the nucleus. Histones are composed of octamers with two copies each of H2A, H2B, H3 and H4 encapsulating DNA and a linker histone (H1). Chemical modifications of histone tails, such as acetylation, methylation, phosphorylation and ubiquitination, alter the affinity of chromatin for those transcription factors, thereby affecting gene transcription and cellular phenotypes (Barth and Imhof 2010). Histone acetylation is a switch that allows interconversion between permissive and repressive chromatin domains (Verdone et al., 2005); histone methylation contributes to the regulation of genome integrity, replication and accessibility (Li et al., 2021b); histone phosphorylation and ubiquitination facilitate DNA damage repair and maintain genome stability (Mattiroli and Penengo 2021; Zhu et al., 2021); and errors in histone posttranslational marks have been implicated in the pathogenesis of human disorders (Eiras et al., 2022). Although direct evidence that histone modifications are associated with POI/POF is unavailable, we can obtain some proof showing how histone modifications regulate mammalian oocyte meiosis, growth, maturation and activation during the normal aging process (Swain et al., 2007; Gu et al., 2010; Yang et al., 2012a). For example, Bui et al. investigated the changes in histone H3 in pig oocytes and found that it undergoes the acetylation/deacetylation and phosphorylation/dephosphorylation modifications during the growth, maturation, and activation of pig oocytes. They also noted that phosphorylation of histone H3 is a key event in oocyte meiosis (Bui et al., 2007). The decreases in oocyte competence with maternal aging is a major factor contributing to mammalian infertility, and one of the factors contributing to this infertility is changes in chromatin modifications. Shao et al. compared H3K4 methylation (H3K4me1/2/3) in young (6–8 weeks old) and older (42–44 weeks old) mouse oocytes and found that H3K4me2/3 levels decreased in older germinal vesicles (GVs), while H3K4me2 levels subsequently increased in older MII oocytes. The authors also found that the expression level of Kdm1a (the gene encoding the H3K4 demethylase lysine (K)-specific demethylase 1A) was increased in older GV oocytes but decreased in older MII oocytes, which was negatively correlated with H3K4me2 levels. These epigenetic changes represent a molecular mechanism underlying human infertility caused by aging (Shao et al., 2015). Suo et al. studied acetylated H4K12 (acH4K12) levels in oocytes during mouse aging and evaluated their effect on the developmental potential of oocytes. They stated that the acH4K12 levels in oocytes were significantly increased during mouse aging. When histone acetylation of oocytes was artificially increased by trichostatin A (TSA) treatment in young mice, a large number of oocytes failed to form pronuclei or formed morphologically abnormal pronuclei (Suo et al., 2010). Manosalva et al. reported that acH4K12 and acH4K16 levels decreased in old GV oocytes, while acH4K12 levels subsequently increased in old MII oocytes. Additionally, the expression of cell division cycle gene 2a (Cdc2a, a gene related to H4K12 acetylation) increased in old oocytes with a non-surrounded nucleolus but decreased in old MII oocytes. Correction of the histone deacetylation of old oocytes at the GV stage restores young-like levels of H4K12 acetylation and CDC2A protein at the MII stage. These data provide evidence for the mechanism by which histone modification affects aging-induced infertility (Manosalva and González 2009). Similar conclusions were reported in the studies by Akiyama et al., Zhang et al., van den Berg et al. and Eslami et al., who suggested that histone modification may affect the aging of germ cells (Akiyama et al., 2006; Eslami et al., 2018; van den Berg et al., 2011; Zhang et al., 2014).
Histone modifications mediate a variety of critical biological processes through chromatin modifications that are conducive to the expression or repression of target genes. Table 2 summarizes the effects of histone modifications on germ cells. Unfortunately, few studies have directly addressed the association of histone modifications with POF. Additionally, the bulk of the literature describing ovarian function has focused on the acetylation and methylation of histones, but other modifications, such as phosphorylation, ubiquitination, and lactylation, have received little attention, although they are also crucial histone epigenetic modifications.
Epigenetic enzymes involved in germ cell senescence
Epigenetic-related enzymes directly regulate epigenetic-based gene expression and therefore exert considerable effects on the aging process and age-associated diseases. DNA methyltransferases are a class of crucial epigenetic enzymes, and include DNMT1, DNMT2, DNMT3A, DNMT3B, DNMT3C and DNMT3L. DNMT1 maintains the methylation of DNA that has been established in the genome, while DNMT3a and DNMT3b are essential for de novo methylation (Finnegan and Kovac 2000). Numerous studies have confirmed the role of DNMT enzymes in transcriptional silencing through their ability to methylate gene promoters and change chromatin states (Klose and Bird 2006; Lyko 2018). However, recent studies found that under certain circumstances, DNMTs may cooperate with transcription factors to activate gene transcription (Rinaldi et al., 2016; Yin et al., 2017). DNA methyltransferases are essential for mammalian development and have been proven to exhibit remarkable differences in spatial and temporal expressional levels and subcellular localizations (Petrussa et al., 2014; Uysal et al., 2017; Chen and Zhang 2020). Yu et al. measured the expression of DNA methyltransferases (Dnmt1/3a/3b/3L) in MII oocytes and found that Dnmt protein levels in the old group were lower than those in the young group, and the DNA methylation levels were also decreased significantly during mouse aging, suggesting that the decreased expression of DNA methyltransferases and the change in genome-wide DNA methylation in oocytes may be related to lower reproductive potential in old female mice (Yue et al., 2012).
TET enzymes include TET1, TET2 and TET3, which regulate DNA demethylation and transcription (Dai et al., 2016; Wu and Zhang 2017). TET-mediated DNA demethylation occurs in various biological contexts, including primordial germ cell development, somatic cell reprogramming, embryonic stem cell maintenance and tumorigenesis (Wu and Zhang 2017). Studies have also revealed the involvement of TET and active DNA demethylation in genomic instability and DNA damage repair (An et al., 2015; Georges et al., 2022). TET1 is necessary for maintaining oocyte quality, oocyte number and the follicle reserve. Tet1 deficiency promotes DNA demethylation in primordial germ cells, leading to downregulation of meiotic gene expression, which in turn leads to abnormal ovarian cell meiosis and a reduced follicle reserve (Yamaguchi et al., 2012). Liu et al. also found that mouse Tet1 deficiency downregulates the expression of X -chromosome-linked genes, such as fragile X messenger ribonucleoprotein 1 (Fmr1), substantially reducing the follicle reserve of Tet1-deficient mice at a young age, and the follicle reserve further decreases with age, a phenomenon consistent with POF (Liu et al., 2021a). Wang et al. showed that Tet2 deficiency increases the DNA methylation levels of genes involved in oocyte meiosis, such as meiotic double-stranded break formation protein 1 (Mei1), cyclin B3 (Ccnb3), meiosis specific with coiled-coil domain (Meioc), synaptonemal complex protein 1/2 (Sycp1/Sycp2), mutS homolog 5 (Msh5), RAD21 cohesin complex component like 1 (Rad21l) and PR domain containing 9 (Prdm9), which significantly delays meiotic progression, reduces oocyte quality and mouse fertility, and thereby accelerates reproductive aging in adult female mice (Wang et al., 2021a).
Primordial follicles (PFs) are the initial stage of follicle development, and their number determines the length of the female reproductive lifespan (Adhikari and Liu 2009). The balance between the quiescent and activated states of PFs is crucial to female fertility, and thus excessive activation may deplete quiescent follicle reserves (Reddy et al., 2010). Histone deacetylases (HDACs) include HDAC1, HDAC2, HDAC3, SIRT1, HDAC6 and the lysine-specific demethylase 1 (LSD1). Tighter wrapping of DNA around histones diminishes accessibility for transcription factors and leads to transcriptional repression. HDAC enzymes remove the acetyl group from histones, resulting in a decrease in the space between the nucleosome and the DNA (de Ruijter et al., 2003). HDACs regulate PF maintenance, oocyte maturation, ovulation and early embryonic development (Vaquero et al., 2007; Matoba et al., 2014; Ma and Schultz 2016; Wang et al., 2019; He et al., 2020). HDAC6 is expressed at high levels in germ cells and is involved in maintaining primordial follicle dormancy in neonatal mice (Verdel et al., 2000; Kawaguchi et al., 2003; Bertos et al., 2004; Zhang et al., 2007; Aslan et al., 2013; Li et al., 2013). Zhang et al. found that overexpression of HDAC6 in mouse embryonic stem cells may reduce the level of H3K9me3, increase follicle numbers (especially antral and secondary follicles) and prolong the reproductive lifespan of mice (Zhang et al., 2017). Zhang et al. support these conclusions and stated that HDAC6 may maintain mouse primordial follicles in a dormant state by regulating the mechanistic target of rapamycin (mTOR)- KIT ligand (KITL) signaling pathway (Zhang et al., 2021). Conversely, low expression of Hdac6 may diminish ovarian reserve. Sirtuin 1 (Sirt1, an NAD-dependent deacetylase) is upregulated during mouse PF activation and activates the Akt/mTOR pathway, suggesting that Sirt1 participates in maintaining PF quiescence (Zhang et al., 2019c). According to He et al., specific disruption of Lsd1 resulted in significantly increased autophagy through its H3K4me2 demethylase activity and a decreased oocyte number in perinatal mice, leading to the depletion of oocytes (He et al., 2020). In contrast, some studies confirm that treatment with HDAC inhibitors, such as TSA or butyrate, may promote steroid hormone synthesis in GCs during follicular development by differentially regulating gene expression (Xing et al., 2018; Ye et al., 2021).
Epigenetic enzymes recognize, add and remove epigenetic marks on DNA and histones. Changes in the structure and activity of epigenetic enzymes significantly affect life expectancy and are associated with aging-related structural and functional declines and senile diseases (Prachayasittikul et al., 2017). Over the past few years, epigenetic drugs, as represented by inhibitors of DNMTs and HDACs, have emerged and have been proven to potentially affect aging-associated diseases and longevity (Pasyukova et al., 2021). Unfortunately, none of these DNMT inhibitors have been proven to prevent POF, and some of them may even impair ovarian function (Table 3). Therefore, relevant research and market development are urgently needed and promising.
Non-coding RNAs involved in the occurrence and development of POF
Non-coding RNAs (ncRNAs) are epigenetic marks that control gene expression by binding to DNA or RNA sequences (at the transcriptional level) and proteins (at the posttranscriptional level). The main types of ncRNAs are microRNAs (miRNAs, ∼20 nt in length) and long ncRNAs (lncRNAs, >200 nt in length), which are involved in multiple physiological and pathological processes (Esteller 2011; Lee and Young 2013).
miRNAs and POF
MiRNAs play a pivotal role in mammalian follicular cell physiology, ovarian function and oocyte maturation by regulating the expression of fertility-related genes (Tesfaye et al., 2018). Studies have suggested that miRNAs are associated with multiple reproductive diseases, such as infertility, polycystic ovary syndrome (PCOS), POF and repeated implantation failure (RIF) (Mezzanzanica et al., 2011; Kamalidehghan et al., 2020). However, the underlying mechanisms remain unclear.
Many researchers have focused on how miRNAs modulate POF occurrence and development. The most popular topic is how miRNAs affect ovarian GCs. Follicular atresia removes most follicles from the ovaries before ovulation and thus limits mammalian follicle utilization. However, GC apoptosis is the basic physical mechanism of follicular atresia. Currently, the broad consensus is that miRNAs participate in the regulation of GC apoptosis. Zhang et al. compared the miRNA profiles of different mammalian species and found that the let-7 family, miR-23–27–24 cluster, miR-183–96–182 cluster, miR-17–92 cluster and their related pathways are involved in GC apoptosis and follicular atresia (Zhang et al., 2019b). Liu et al. observed the downregulation of miR-92a expression in atretic porcine follicles, and miR-92a expression inhibited GC apoptosis by targeting the Smad family member 7 (Smad7)-TGFβ pathway (Liu et al., 2014). Yang et al. analyzed the miRNA-regulated signaling pathways and related genes in POF patients by performing Gene Ontology (GO) and Kyoto Encyclopedia of Genes and Genomes (KEGG) pathway analyses and found that miR-23a was overexpressed in POF patients. They reported that miR-23a may induce GC apoptosis by activating the X-linked inhibitor of apoptosis protein (XIAP) and caspase-3 pathways (Yang et al., 2012b). Chen et al. observed the significant upregulation of miR-146a expression in GC cells from POF patients, and miR-146a induced GC apoptosis by targeting IRAK1 (interleukin-1 receptor-associated kinase) and TRAF6 (tumor necrosis factor receptor-associated factor 6) (Chen et al., 2015). Zhang et al. reported significantly higher miR-181a expression in blood from POF patients. They also suggested that overexpression of miR-181a downregulates cyclin D2 and inhibits mouse GC proliferation (Zhang et al., 2013). According to Zhang et al. and Dang et al., the expression of miR-127-5p and miR-379-5p in biochemical POI patients was significantly upregulated. Overexpression of these two miRNAs may inhibit mouse GC proliferation and attenuate DNA repair by targeting high mobility group box 2 (Hmgb2), poly(ADP-ribose) polymerase 1 (Parp1) and X-ray repair cross complementing 6 (Xrcc6) (Dang et al., 2018; Zhang et al., 2020). Other similar studies investigating the correlation between miRNA variations, gene regulation, GC apoptosis, folliculogenesis and POF development are listed in Table 4.
The use of drugs is a negligible risk factor for POF. A clear understanding of the molecular mechanism by which these drugs induce POF is important for protecting the female reproductive system in women of childbearing age (especially female cancer patients). Multiple studies have revealed that miRNAs are involved in drug-induced POF. For example, Liu et al. found that cyclophosphamide may upregulate the expression of miR-15b, silence endogenous α-Klotho (KL) and stimulate the TGFβ1/Smad pathway, attenuating the autophagy of mouse GCs, inducing GC apoptosis and reducing ovarian reserve (Liu et al., 2019b). Wang et al. reported that cisplatin may upregulate miR-125a-5p expression and induce mouse GC apoptosis by inhibiting the expression of signal transducer and activator of transcription 3 (Stat3) (Wang et al., 2016). As shown in the study by Ai et al., triptolide induces the expression of endogenous miR-15a and inhibits the Hippo-yes-associated protein (Hippo-YAP)/TAZ pathway, leading to the cytotoxicity, senescence and apoptosis of ovarian GCs (Ai et al., 2018). Additionally, epigenetic therapy is a promising approach for the treatment of cancers (Matthews et al., 2021; Xu et al., 2021; Brown et al., 2022; Hoang and Landi 2022). Since DNA methylation plays an important role in the formation of primordial germ cells, epigenetic therapy, e.g., DNMT inhibitor-based (such as decitabine) therapies, may hamper oocyte differentiation and development and thus induce POI (Yang et al., 2019). However, studies and experimental results in this area are still lacking and needed.
LncRNAs and POF
LncRNAs function in various diseases (Yang et al., 2014). Studies have found that lncRNAs may participate in the development of POF, but the underlying mechanism remains unclear.
Two mechanisms may explain the role of lncRNAs in POF. One is the interactions between lncRNAs and miRNAs, which may induce ovarian GC apoptosis. For example, Zheng et al. found that in POF patients, the lncRNA deleted in lymphocytic leukemia 1 (DLEU1) is upregulated and its overexpression promotes GC apoptosis (Zheng et al., 2021). Zhang et al. observed a decreased expression level of translation regulatory long non-coding RNA 1 (TRERNA1) in POF patients, and TRERNA1 may sponge miR-23a and suppress GC apoptosis (Zhang et al., 2022). Yu et al. found that the lncRNA BBOX1 antisense RNA 1 (BBOX1-AS1) in GC was unregulated in POF patients. BBOX1-AS1 may directly interact with miR-146b, and overexpression of BBOX1-AS1 may increase GC apoptosis in POF by sponging miR-146 b (Yu et al., 2022). Yao et al. documented that non-coding RNA that was highly expressed in atretic follicles (NORHA), which is related to follicular atresia, induces GC apoptosis by inhibiting the activities of the miR-183–96–182 cluster and FoxO1 axis (Yao et al., 2021).
The other mechanism is that some lncRNAs are involved in regulating several critical proteins and signaling pathways. For example, Xiong et al. indicated that cyclophosphamide induces mouse ovarian atrophy and inhibits the proliferation of ovarian GCs. The lncRNA maternally expressed gene 3 (Meg3) was unregulated in cyclophosphamide-treated mouse ovarian GCs, and inhibition of Meg3 effectively reduced the effect of cyclophosphamide through the p53-p66Shc pathway (Xiong et al., 2017). Li et al. observed the significant downregulation of the expression of the lncRNA NEAT1 (nuclear enriched abundant transcript 1) in the ovarian tissue of POF patients. Cytological experiments indicated that overexpression of NEAT1 may inhibit ovarian cell apoptosis by inhibiting P53 (Li et al., 2020a). Zhao et al. detected significantly lower expression of the lncRNA HOX antisense intergenic RNA (HOTAIR) in ovarian tissue and serum samples from POF patients than in healthy controls. Overexpression of HOTAIR in hamster ovary cell lines upregulated Notch-1 expression and reduced cell apoptosis (Zhao and Dong 2018). Table 5 summarizes the lncRNAs involved in POF and their targets.
External factors involved in POF development and their epigenetic mechanisms
Environmental, social, psychological and lifestyle factors may accelerate the decline in ovarian reserve (Richardson et al., 2014) (Figure 3). Based on accumulating evidence, exposure to reproductively toxic environmental chemicals (RTECs) leads to premature menopause and POF. RTEC exposure during fetal and neonatal periods reduces ovarian reserve, whereas exposure during the prepubertal period and adulthood accelerate follicular pool depletion, and the mechanism may be ascribed to changes in the germ cell epigenome (Béranger et al., 2012; Grindler et al., 2015; Vabre et al., 2017; Ge et al., 2019). According to a recent study, multiple chemicals (such as phthalates (PAEs), polychlorinated biphenyls (PCBs), and bisphenol A (BPA), which are widely used in electronic transformers, capacitors, coolants and food packaging), ionizing radiation, smoking and drinking may affect ovarian reserve via epigenetic regulation and induce POF (Yang et al., 2021). High-dose BPA exposure during the neonatal period is associated with the occurrence of POF in adulthood. Exposure to BPA during pregnancy has been suggested alter the expression of steroid hormone synthesis-related genes and microRNA profiles related to gonadal differentiation and follicle synthesis in offspring and ultimately disrupt the fertility of offspring (Yang et al., 2021). Zhang et al. found that exposure to BPA reduced the methylation levels of imprinted genes insulin-like growth factor 2 receptor (Igf2r), paternally expressed gene 3 (Peg3) and H19 in fetal rat germ cells, which in turn affected gametogenesis (Zhang et al., 2012). Qiu et al. reported decreased fertility and progesterone levels in neonatal mice exposed to BPA, along with increased serum testosterone and estradiol levels in their adult period. Exposure to BPA may interfere with the function of the hypothalamic-pituitary-ovarian axis (HPOA), decrease the production of sex hormones and reduce the number of oocytes (Qiu et al., 2020). Currently, researchers postulate that the reproductive toxicity of BPA is closely correlated with its epigenetic regulation of gene expression. Zama et al. found that when fetal rats were exposed to 100 mg/kg methoxychlor (MXC, an organochlorine pesticide with weak estrogenicity) in utero every day, the DNA methylation level in the estrogen receptor (ER)-β promoter region was increased, and the Dnmt3b level was elevated in postnatal rat ovarian tissue (Zama and Uzumcu 2009). Li et al. examined diethylhexyl phthalate (DEHP)-treated mouse oocytes and observed a reduced percentage of methylated CpG sites in the imprinted genes Igfr2r and Peg3, and the DNA methylation modification was inherited by the F2 generation and thus decreased the number of primordial follicles in puberty and adulthood (Li et al., 2014). Nilsson et al. reported that exposure to the environmental toxicants vinclozolin and dichlorodiphenyltrichloroethane (DDT) promotes epigenetic susceptibility in the ovaries of F0 generation rats, altering DNA methylation and ncRNAs in the ovarian GCs of the F3 generation; these results indicated that environmental toxicants may induce transgenerational inheritance of the ovarian GC epigenome (Nilsson et al., 2018). As shown in the study by Liu et al., a HFHS (high-fat and high-sugar) diet promotes ovarian GC aging and POF by inhibiting endogenous miR-146b-5p expression, activating the disabled homolog 2-interacting protein (DAB2ip)/apoptosis signal-regulating kinase 1 (ASK1)/p38 signaling pathway and γ-H2A.X phosphorylation (Liu et al., 2021c). Rhon-Calderón et al. found that daily exposure to low doses of 3-methylcholanthrene (3 MC) in adolescent mice decreases follicle numbers, blocks follicle development and inhibits ovulation (Rhon-Calderón et al., 2016). They indicated that 3 MC increases the H3K4me3 trimethylation levels at the cytochrome P450 family one subfamily A member 1 (Cyp1a1), jagged canonical notch ligand 1 (Jag1), dnaJ heat shock protein family (Hsp40) member B6 (Dnajb6), Igf2, Notch receptor 2 (Notch2), ADAM with thrombospondin type 1 motif 1 (Adamts1), BCL2-associated X (Bax) and Caspase-9 genes and the H3K9Ac acetylation levels at the Cyp1A1, Jag1, cyclin-dependent kinase 2 (Cdk2), Dnajb6, Igf2, intercellular cell adhesion molecule-1 (Icam1), and Sp1 genes, suggesting that mechanisms may play an important role in POF development (Rhon-Calderón et al., 2018). Additionally, ionizing radiation alters DNA methylation patterns, modifies histone/chromatin structure and changes miRNA profiles (Pogribny et al., 2005; Halimi et al., 2012; Kumar et al., 2012; Metheetrairut and Slack 2013; Miousse et al., 2017). Ionizing radiation may also damage the female reproductive system and decrease ovarian reserve (Quan et al., 2020). Filkowski et al. found that exposure to 2.5 of Gy X-rays upregulated the expression level of miR-29 in mouse germ cells, resulting in a decrease in Dnmt3a levels, which in turn increased the susceptibility of the ovary in offspring and the risk of POF (Filkowski et al., 2010).
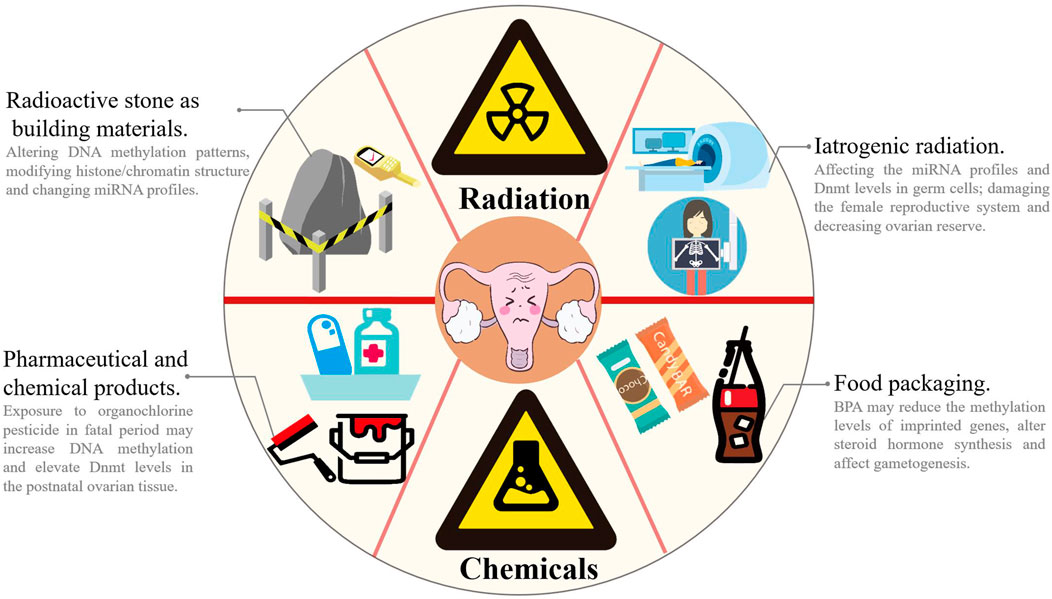
FIGURE 3. Environmental and lifestyle factors accelerates the decline of ovarian function. In our daily life, chemical and radiation pollutions are very common. Chemical pollutions come from paint (e.g., PCBs), drugs/pesticide (e.g., MXC and DDT), electronic equipment and food packaging (e.g., PAEs and BPA), and radiation pollutions mainly come from decoration materials (especially stones) and iatrogenic radiation (e.g., MRI and CT scan). Exposure to RTECs or ionizing radiation may alter DNA methylation patterns, modify histone/chromatin structure and change miRNA profiles and thus impairs sex hormone synthesis, affects gametogenesis and decreases fertility. MRI: magnetic resonance imaging; CT: computerized tomography.
Transgenerational epigenetic changes may be inherited through germ cells (sperm or eggs) and occur in early embryonic and stem cells, affecting all somatic cells and tissues and increasing disease susceptibility in adulthood. Therefore, ovarian disease may be partially induced by ancestral environmental exposures and the corresponding epigenetic changes (Anway and Skinner 2008). With industrialization, globalization and the growing economy, people’s lifestyles have become more convenient than ever before. However, currently, people are exposed to various chemicals, and the potential hazards of these chemicals should not be ignored.
Epigenetic targets OF POF therapy
Currently, the most common treatment for POF in the clinic is hormone replacement therapy (HRT). However, HRT only relieves low estrogen-related symptoms such as vaginal dryness, hot flashes and genitourinary tract atrophy but does not improve ovarian reproductive function (Sullivan et al., 2016). Therefore, an understanding of the pathogenesis of POF is crucial to develop corresponding treatment regimens targeting those key pathogenic factors. In many cases, POF patients harbor epigenetic alterations in their reproductive system instead of genetic alterations (e.g., gene mutations). Therefore, precision therapy targeting epigenetic variations is a probable and valuable approach for POF clinical treatment in the future.
Epigenetic strategies for POF treatment targeting DNA and histone modifications are still in the exploratory stage. Previous studies have confirmed that butyrate, an HDAC inhibitor, can loosen the chromosome structure and enhance gene transcription by increasing the level of histone acetylation (Corfe 2012). Ye et al. found that butyrate increases estradiol and progesterone synthesis in rat and human GCs by increasing the acetylation of histone H3K9 (H3K9ac) and stimulating the peroxisome proliferator activated receptor (PPARγ)/CD36/steroidogenic acute regulatory protein (StAR) pathways, which enhances mitochondrial dynamics and alleviates oxidative damage in GCs (Ye et al., 2021). As shown in the study by Liu et al., thymopentin promotes the transcription and expression of Lin28A (a marker of ovarian GC proliferation), inhibits the activity of let-7 family miRNAs and alleviates the aging of ovarian GCs, which provides a valuable therapeutic target for POF (Liu et al., 2021b). Zhao and others found that hyaluronic acid (HA) blocks Tripterygium glycoside-induced POI-like presentations in rats, including delayed or irregular estrous cycles, reduced E2 concentrations, decreased numbers of follicles, destruction of the follicle structure, and damage to the reproductive ability. Regarding the molecular mechanism, they indicated that HA upregulated progesterone receptor membrane component 1 (Pgrmc 1) expression in GCs by suppressing of miR-139-5p. Moreover, HA downregulated miR-139-5p expression via histone deacetylation at its promoter (Zhao et al., 2014; Zhao et al., 2015).
Natural products and traditional Chinese medicine may exert unexpected effects on the treatment of POF. For example, Zhu et al. showed that American ginseng treatment regulates the expression levels of phospholipase A2 group IVA (Pla2g4a), miR-29a and miR-144 in POF rats. After POF rats were administered American ginseng for 1 month, the levels of all hormones (prostaglandin E2 (PGE2), FSH, and luteinizing hormone (LH)) and E2 secretion approached normal levels, and POF symptoms were improved (Zhu et al., 2015). Liu et al. treated POF rats with 10 ml/kg/day modified Bazhen decoction (MBD, a traditional Chinese medicine mixing Ginseng, Atractylodes macrocephala Koidz, Poria cocos, Licorice, Angelica sinensis, Radix Rehmanniae, Radix Paeoniae Alba and Ligusticum wallichii) for 4 weeks and found that MDB substantially activated X-linked inhibitor of apoptosis protein (Xiap) but inhibited the expression of miR-23a and miR-27a and effectively prevented the apoptosis of oocytes and GCs (Liu et al., 2019a).
Mesenchymal stem cell (MSC) transplantation is the most promising option to treat POF. Preliminary clinical trials have shown that MSCs improve ovarian function and increase the pregnancy rate of POI/POF patients (Wang et al., 2021b). We systematically described the mechanism and application of MSC therapy for POF in our previous review (Wang et al., 2021b). By synthesizing the current research conclusions, we consider that MSC therapy for POF relies mainly on miRNA-dependent epigenetic regulation and its related cellular signal transduction pathways. According to Fu et al., transplantation of MSCs overexpressing miR-21 in rats with chemotherapy-induced POF increases ovarian weight and follicle counts, increases E2 levels and decreases FSH levels. They also indicated that miR-21 inhibits GC apoptosis by targeting phosphatase and tensin homolog (Pten) and programmed cell death 4 (Pdcd4) (Fu et al., 2017). EL-Derany et al. showed that bone marrow MSCs (BMMSCs) increase the ovarian follicle pool and preserve ovarian function in rats with γ-ray-induced POF. They indicated that BMMSC miRNAs epigenetically regulate the TGF-β, Wnt/β-catenin and Hippo signaling pathways, which control the apoptosis, proliferation, and differentiation of ovarian follicles (El-Derany et al., 2021).
Exosomes and their incorporated miRNAs have been proven to be functional components in MSC therapy. Yang et al. found that BMSC-derived exosomes prevent ovarian follicular atresia and inhibit GC apoptosis in cyclophosphamide-treated rats via the delivery of miR-144-5p and suppression of its related Pten/Pi3k/Akt pathway (Yang et al., 2020). Sun et al. found that miR-644-5p carried by BMSC-derived exosomes represses GC apoptosis and increase cell viability in mice with cisplatin-induced POF by negatively regulating p53 (Sun et al., 2019). In the study by Xiao et al., amniotic fluid stem cell (AFSC)-derived exosomes prevented ovarian follicular atresia in chemotherapy-treated mice via the delivery of miR-146a (targeting Irak1 and Traf6) and miR-10a (targeting Bim) and inhibition of GC apoptosis (Xiao et al., 2016).
Currently, hundreds of potential epigenetic drugs targeting DNMTs and histone methyltransferase have been developed or have undergone clinical trials, and some of them have been approved by the FDA or EMA. However, the diseases for which these drugs have been approved as treatments are limited to cancers, Alzheimer’s disease, and diabetes (Pasyukova et al., 2021), and no epigenetic drug is definitely suitable for the prevention or treatment of POF (Table 6). Additionally, since aging is highly complex, the side effects of some epigenetic drugs have been introduced into clinical practice to treat aging-associated diseases (e.g., neurological disturbances, and cardiac and metabolic abnormalities) are unignorable (Nervi et al., 2015).
Conclusion and prospects
POF is different from aging-associated diseases, such as Alzheimer’s disease, diabetes and cancers. Although non-fatal, it is very harmful to women’s bodies and minds, especially those women of childbearing age. In China, with the continuous development of the economy and revolution of traditional culture, women are increasingly participating in all aspects of social life. Due to increasing academic, economic and living pressure, the maternal age at first pregnancy of Chinese women continues to increase. If this phenomenon continues, it will inevitably lead to an aging population and a substantial decrease in the social labor force. Moreover, many women find themselves suffering from POF by the time they want to have a child, and an indisputable fact is that the number of POF patients is increasing in the clinic.
Due to the non-lethal nature of POF, many women choose to remain silent about the disease. The complexity of the disease prevents researchers and doctors from determining a clear and definite cause of the disease. However, the spread of this disease will clearly harm society in the future. In many cases, the occurrence of POF is not caused by an exact gene or protein variation; it is more likely induced by the combined effect of the patient’s physical condition, psychological condition and living environment. In this case, patients’ epigenetic changes are the dominant factor contributing to this disease. In this review, by summarizing the research achievements published in the past 2 decades, we draw several conclusions, which are listed below. 1) Epigenetic variation exists objectively in POF patients and is closely correlated with the occurrence and development of POF. 2) Epigenetic variations in POF patients mainly include changes in DNA, histones, enzymes, and profiles of ncRNAs. 3) Studies examining ncRNA expression profiles are significantly more common than studies on assessing other aspects, which may be because next-generation sequencing is easier, faster and cheaper to implement. Meanwhile, we strongly recommend that the following issues are considered: 1) research on the epigenetic etiology of POF is still far from sufficient; 2) although closely related to epigenetic variations, no epigenetic drug specific for POF has been developed. In other words, the treatment of this disease is still not receiving sufficient attention; 3) as a chronic disease, stem cell therapy and natural medicine (mainly targeting non-coding RNA and immunomodulation) appear to be milder and more appropriate than epigenetic enzyme inhibitors.
Author contributions
DY conceived the manuscript. JW and DY drafted the manuscript. ZY drew the figures. DY, XS, SL, and JW proofread the manuscript and made revisions. JW, DY, YW, RR, and ZL collected the references. All authors read and approved the final manuscript.
Funding
This work was supported in part by Jilin International Collaboration Grant (20220402066GH to DY), the Key Research and Development Program of Changchun Science and Technology Bureau (21ZGY04 to XS), the Subject Arrangement Program from Science and Technology Department of Jilin Province (20200201123JC to DY and 20190201209JC to SL), the National Natural Science Foundation of China Grant (81801227 to XS), the Health Talent Project of Jilin Province (JLSWSRCZX2021-011 to SL); Innovation and Entrepreneurship Training Program for College Students (S202210183552, to YW, RR, and ZL).
Conflict of interest
The authors declare that the research was conducted in the absence of any commercial or financial relationships that could be construed as a potential conflict of interest.
Publisher’s note
All claims expressed in this article are solely those of the authors and do not necessarily represent those of their affiliated organizations, or those of the publisher, the editors and the reviewers. Any product that may be evaluated in this article, or claim that may be made by its manufacturer, is not guaranteed or endorsed by the publisher.
References
Adhikari D., Liu K. (2009). Molecular mechanisms underlying the activation of mammalian primordial follicles. Endocr. Rev. Aug 30, 438–464. Epub 2009/07/11. doi:10.1210/er.2008-0048
Ai A., Xiong Y., Wu B., Lin J., Huang Y., Cao Y., et al. (2018). Induction of miR-15a expression by tripterygium glycosides caused premature ovarian failure by suppressing the Hippo-YAP/TAZ signaling effector Lats1. Gene 15, 155–163. Epub 2018/08/10. doi:10.1016/j.gene.2018.08.018
Akiyama T., Nagata M., Aoki F. (2006). Inadequate histone deacetylation during oocyte meiosis causes aneuploidy and embryo death in mice. Proc. Natl. Acad. Sci. U. S. A. 103, 7339–7344. Epub 2006/05/03. doi:10.1073/pnas.0510946103
Aldakheel F. M., Abuderman A. A., Alduraywish S. A., Xiao Y., Guo W. W. (2021). MicroRNA-21 inhibits ovarian granulosa cell proliferation by targeting SNHG7 in premature ovarian failure with polycystic ovary syndrome. J. reproductive Immunol. 146, 103328. Epub 2021/05/22. doi:10.1016/j.jri.2021.103328
An J., González-Avalos E., Chawla A., Jeong M., López-Moyado I. F., Li W., et al. (2015). Acute loss of TET function results in aggressive myeloid cancer in mice. Nat. Commun. 26 (6), 10071. Epub 2015/11/27. doi:10.1038/ncomms10071
Anway M. D., Skinner M. K. (2008). Epigenetic programming of the germ line: Effects of endocrine disruptors on the development of transgenerational disease. Reprod. Biomed. online 16, 23–25. Epub 2008/02/07. doi:10.1016/s1472-6483(10)60553-6
Aslan J. E., Phillips K. G., Healy L. D., Itakura A., Pang J., McCarty O. J. (2013). Histone deacetylase 6-mediated deacetylation of α-tubulin coordinates cytoskeletal and signaling events during platelet activation. Am. J. physiology Cell physiology 305, C1230–C1239. Epub 2013/09/13. doi:10.1152/ajpcell.00053.2013
Barbarino-Monnier P. (2000). Premature ovarian failure. J. de Gynecol. obstetrique Biol. de reproduction 29, 316–318. Epub 2000/05/11.
Barth T. K., Imhof A. (2010). Fast signals and slow marks: The dynamics of histone modifications. Trends Biochem. Sci. 35, 618–626. Epub 2010/08/06. doi:10.1016/j.tibs.2010.05.006
Béranger R., Hoffmann P., Christin-Maitre S., Bonneterre V. (2012). Occupational exposures to chemicals as a possible etiology in premature ovarian failure: A critical analysis of the literature. Reprod. Toxicol. (Elmsford, NY) 33, 269–279. Epub 2012/01/28. doi:10.1016/j.reprotox.2012.01.002
Bertos N. R., Gilquin B., Chan G. K., Yen T. J., Khochbin S., Yang X. J. (2004). Role of the tetradecapeptide repeat domain of human histone deacetylase 6 in cytoplasmic retention. J. Biol. Chem. 12 (279), 48246–48254. Epub 2004/09/07. doi:10.1074/jbc.M408583200
Bione S., Benedetti S., Goegan M., Menditto I., Marozzi A., Ferrari M., et al. (2006). Skewed X-chromosome inactivation is not associated with premature ovarian failure in a large cohort of Italian patients. Am. J. Med. Genet. Part A 140, 1349–1351. Epub 2006/05/13. doi:10.1002/ajmg.a.31312
Bjornsson H. T., Sigurdsson M. I., Fallin M. D., Irizarry R. A., Aspelund T., Cui H., et al. (2008). Intra-individual change over time in DNA methylation with familial clustering. Jama 25 (299), 2877–2883. Epub 2008/06/26. doi:10.1001/jama.299.24.2877
Boumil R. M., Lee J. T. (2001). Forty years of decoding the silence in X-chromosome inactivation. Hum. Mol. Genet. 10, 2225–2232. Epub 2001/10/24. doi:10.1093/hmg/10.20.2225
Bretherick K. L., Metzger D. L., Chanoine J. P., Panagiotopoulos C., Watson S. K., Lam W. L., et al. (2007). Skewed X-chromosome inactivation is associated with primary but not secondary ovarian failure. Am. J. Med. Genet. Part A 143a, 945–951. Epub 2007/04/14. doi:10.1002/ajmg.a.31679
Brown L. J., Achinger-Kawecka J., Portman N., Clark S., Stirzaker C., Lim E. (2022). Epigenetic therapies and biomarkers in breast cancer. Cancers 14, 474. Epub 2022/02/16. doi:10.3390/cancers14030474
Bui H. T., Van Thuan N., Kishigami S., Wakayama S., Hikichi T., Ohta H., et al. (2007). Regulation of chromatin and chromosome morphology by histone H3 modifications in pig oocytes. Reprod. Camb. Engl. 133, 371–382. Epub 2007/02/20. doi:10.1530/REP-06-0099
Chamani I. J., Keefe D. L. (2019). Epigenetics and female reproductive aging. Front. Endocrinol. 10, 473. Epub 2019/09/26. doi:10.3389/fendo.2019.00473
Chen X., Xie M., Liu D., Shi K. (2015). Downregulation of microRNA-146a inhibits ovarian granulosa cell apoptosis by simultaneously targeting interleukin-1 receptor‑associated kinase and tumor necrosis factor receptor-associated factor 6. Mol. Med. Rep. 12, 5155–5162. Epub 2015/07/08. doi:10.3892/mmr.2015.4036
Chen Z., Zhang Y. (2020). Role of mammalian DNA methyltransferases in development. Annu. Rev. Biochem. 89, 135–158. Epub 2019/12/10. doi:10.1146/annurev-biochem-103019-102815
Cho S. H., An H. J., Kim K. A., Ko J. J., Kim J. H., Kim Y. R., et al. (2017). Single nucleotide polymorphisms at miR-146a/196a2 and their primary ovarian insufficiency-related target gene regulation in granulosa cells. PloS one 12, e0183479. Epub 2017/08/26. doi:10.1371/journal.pone.0183479
Collins G., Patel B., Thakore S., Liu J. (2017). Primary ovarian insufficiency: Current concepts. South. Med. J. Mar 110, 147–153. Epub 2017/03/04. doi:10.14423/SMJ.0000000000000611
Corfe B. M. (2012). Hypothesis: Butyrate is not an HDAC inhibitor, but a product inhibitor of deacetylation. Mol. Biosyst. Jun 8, 1609–1612. Epub 2012/03/27. doi:10.1039/c2mb25028d
Cotton A. M., Lam L., Affleck J. G., Wilson I. M., Peñaherrera M. S., McFadden D. E., et al. (2011). Chromosome-wide DNA methylation analysis predicts human tissue-specific X inactivation. Hum. Genet. 130, 187–201. Epub 2011/05/21. doi:10.1007/s00439-011-1007-8
Coulam C. B., Adamson S. C., Annegers J. F. (1986). Incidence of premature ovarian failure. Obstetrics Gynecol. 67, 604–606. Epub 1986/04/01.
Dai H. Q., Wang B. A., Yang L., Chen J. J., Zhu G. C., Sun M. L., et al. (2016). TET-mediated DNA demethylation controls gastrulation by regulating Lefty-Nodal signalling. Nature 538, 528–532. Epub 2016/10/28. doi:10.1038/nature20095
Dang Y., Wang X., Hao Y., Zhang X., Zhao S., Ma J., et al. (2018). MicroRNA-379-5p is associate with biochemical premature ovarian insufficiency through PARP1 and XRCC6. Cell death Dis. 9, 9106. Epub 2018/01/26. doi:10.1038/s41419-017-0163-8
Dang Y., Zhao S., Qin Y., Han T., Li W., Chen Z. J. (2015). MicroRNA-22-3p is down-regulated in the plasma of Han Chinese patients with premature ovarian failure. Fertil. Steril. 103, 802–807. e801Epub 2015/01/15. doi:10.1016/j.fertnstert.2014.12.106
Das R., Hampton D. D., Jirtle R. L. (2009). Imprinting evolution and human health. Mammalian genome official J. Int. Mammalian Genome Soc. 20, 563–572. Epub 2009/10/16. doi:10.1007/s00335-009-9229-y
de Ruijter A. J., van Gennip A. H., Caron H. N., Kemp S., van Kuilenburg A. B. (2003). Histone deacetylases (HDACs): Characterization of the classical HDAC family. Biochem. J. 370, 737–749. Epub 2002/11/14. doi:10.1042/BJ20021321
Deaton A. M., Bird A. (2011). CpG islands and the regulation of transcription. Genes & Dev. 25, 1010–1022. Epub 2011/05/18. doi:10.1101/gad.2037511
Donadeu F. X., Mohammed B. T., Ioannidis J. (2017). A miRNA target network putatively involved in follicular atresia. Domest. Anim. Endocrinol. 58, 76–83. Epub 2016/09/25. doi:10.1016/j.domaniend.2016.08.002
Duncan C. G., Grimm S. A., Morgan D. L., Bushel P. R., Bennett B. D., Roberts J. D., et al. (2018). Dosage compensation and DNA methylation landscape of the X chromosome in mouse liver. Sci. Rep. 8 (8), 10138. Epub 2018/07/06. doi:10.1038/s41598-018-28356-3
Eiras M. C., Pinheiro D. P., Romcy K. A. M., Ferriani R. A., Reis R. M. D., Furtado C. L. M. (2022). Polycystic ovary syndrome: The epigenetics behind the disease. Reprdo Sci. 29, 680–694. Epub 2021/04/08. doi:10.1007/s43032-021-00516-3
El-Derany M. O., Said R. S., El-Demerdash E. (2021). Bone marrow-derived mesenchymal stem cells reverse radiotherapy-induced premature ovarian failure: Emphasis on signal integration of TGF-β, wnt/β-catenin and Hippo pathways. Stem Cell Rev. Rep. 17, 1429–1445. Epub 2021/02/18. doi:10.1007/s12015-021-10135-9
Eslami H., Eslami A., Favaedi R., Asadpour U., Zari Moradi S., Eftekhari-Yazdi P., et al. (2018). Epigenetic aberration of FMR1 gene in infertile women with diminished ovarian reserve. Cell J. 20, 78–83. Epub 2018/01/09. doi:10.22074/cellj.2018.4398
Esteller M. (2011). Non-coding RNAs in human disease. Nat. Rev. Genet. 18 (12), 861–874. Epub 2011/11/19. doi:10.1038/nrg3074
Ferreira S. I., Matoso E., Pinto M., Almeida J., Liehr T., Melo J. B., et al. (2010). X-chromosome terminal deletion in a female with premature ovarian failure: Haploinsufficiency of X-linked genes as a possible explanation. Mol. Cytogenet. 3, 14. Epub 2010/07/22. doi:10.1186/1755-8166-3-14
Filkowski J. N., Ilnytskyy Y., Tamminga J., Koturbash I., Golubov A., Bagnyukova T., et al. (2010). Hypomethylation and genome instability in the germline of exposed parents and their progeny is associated with altered miRNA expression. Carcinogenesis 31, 1110–1115. Epub 2009/12/05. doi:10.1093/carcin/bgp300
Finnegan E. J., Kovac K. A. (2000). Plant DNA methyltransferases. Plant Mol. Biol. 43, 189–201. Epub 2000/09/22. doi:10.1023/a:1006427226972
Fu X., He Y., Wang X., Peng D., Chen X., Li X., et al. (2017). Overexpression of miR-21 in stem cells improves ovarian structure and function in rats with chemotherapy-induced ovarian damage by targeting PDCD4 and PTEN to inhibit granulosa cell apoptosis. Stem Cell Res. Ther. 8, 187. Epub 2017/08/16. doi:10.1186/s13287-017-0641-z
Gao Y. Y., Chen L., Wang T., Nie Z. W., Zhang X., Miao Y. L. (2016). Oocyte aging-induced Neuronatin (NNAT) hypermethylation affects oocyte quality by impairing glucose transport in porcine. Sci. Rep. 6, 36008. Epub 2016/10/27. doi:10.1038/srep36008
Ge W., Li L., Dyce P. W., De Felici M., Shen W. (2019). Establishment and depletion of the ovarian reserve: Physiology and impact of environmental chemicals. Cell. Mol. life Sci. CMLS 76, 1729–1746. Epub 2019/02/28. doi:10.1007/s00018-019-03028-1
Georges R. O., Sepulveda H., Angel J. C., Johnson E., Palomino S., Nowak R. B., et al. (2022). Acute deletion of TET enzymes results in aneuploidy in mouse embryonic stem cells through decreased expression of Khdc3. Nat. Commun. 13, 6230. Epub 2022/10/21. doi:10.1038/s41467-022-33742-7
Grindler N. M., Allsworth J. E., Macones G. A., Kannan K., Roehl K. A., Cooper A. R. (2015). Persistent organic pollutants and early menopause in U.S. women. PloS one 10, e0116057. Epub 2015/01/30. doi:10.1371/journal.pone.0116057
Gu L., Wang Q., Sun Q. Y. (2010). Histone modifications during mammalian oocyte maturation: Dynamics, regulation and functions. Cell cycleGeorget. Tex) 9, 1942–1950. Epub 2010/05/04. doi:10.4161/cc.9.10.11599
Guglielmino M. R., Santonocito M., Vento M., Ragusa M., Barbagallo D., Borzì P., et al. (2011). TAp73 is downregulated in oocytes from women of advanced reproductive age. Cell cycleGeorget. Tex) 10, 3253–3256. Epub 2011/09/29. doi:10.4161/cc.10.19.17585
Halimi M., Asghari S. M., Sariri R., Moslemi D., Parsian H. (2012). Cellular response to ionizing radiation: A MicroRNA story. Int. J. Mol. Cell. Med. Fall 1, 178–184. Epub 2012/10/01.
He M., Zhang T., Zhu Z., Qin S., Wang H., Zhao L., et al. (2020). LSD1 contributes to programmed oocyte death by regulating the transcription of autophagy adaptor SQSTM1/p62. Aging Cell. Mar. 19, e13102. Epub 2020/02/20. doi:10.1111/acel.13102
Hoang P. H., Landi M. T. (2022). DNA methylation in lung cancer: Mechanisms and associations with histological subtypes, molecular alterations, and major epidemiological factors. Cancers 14, 961. Epub 2022/02/26. doi:10.3390/cancers14040961
Inoue T., Nakamura A., Iwahashi-Odano M., Tanase-Nakao K., Matsubara K., Nishioka J., et al. (2020). Contribution of gene mutations to silver-russell syndrome phenotype: Multigene sequencing analysis in 92 etiology-unknown patients. Clin. epigenetics 12, 86. Epub 2020/06/18. doi:10.1186/s13148-020-00865-x
Jiao X., Ke H., Qin Y., Chen Z. J. (2018). Molecular genetics of premature ovarian insufficiency. Trends Endocrinol. metabolism TEM 29, 795–807. Epub 2018/08/07. doi:10.1016/j.tem.2018.07.002
Kamalidehghan B., Habibi M., Afjeh S. S., Shoai M., Alidoost S., Almasi Ghale R., et al. (2020). The importance of small non-coding RNAs in human reproduction: A review article. Appl. Clin. Genet. 13, 1–11. Epub 2020/02/06. doi:10.2147/TACG.S207491
Kawaguchi Y., Kovacs J. J., McLaurin A., Vance J. M., Ito A., Yao T. P. (2003). The deacetylase HDAC6 regulates aggresome formation and cell viability in response to misfolded protein stress. Cell 115, 727–738. Epub 2003/12/17. doi:10.1016/s0092-8674(03)00939-5
Kline J., Kinney A., Levin B., Kelly A., Yu C. Y., Brown S., et al. (2006). X-chromosome inactivation and ovarian age during the reproductive years. Fertil. Steril. 85, 1488–1495. Epub 2006/05/02. doi:10.1016/j.fertnstert.2005.10.059
Klose R. J., Bird A. P. (2006). Genomic DNA methylation: The mark and its mediators. Trends Biochem. Sci. 31, 89–97. Epub 2006/01/13. doi:10.1016/j.tibs.2005.12.008
Kordowitzki P., Haghani A., Zoller J. A., Li C. Z., Raj K., Spangler M. L., et al. (2021). Epigenetic clock and methylation study of oocytes from a bovine model of reproductive aging. Aging Cell 20, e13349. Epub 2021/04/03. doi:10.1111/acel.13349
Kuang H., Han D., Xie J., Yan Y., Li J., Ge P. (2014). Profiling of differentially expressed microRNAs in premature ovarian failure in an animal model. Gynecol. Endocrinol. official J. Int. Soc. Gynecol. Endocrinol. 30, 57–61. Epub 2013/11/06. doi:10.3109/09513590.2013.850659
Kumar R., Horikoshi N., Singh M., Gupta A., Misra H. S., Albuquerque K., et al. (2012). Chromatin modifications and the DNA damage response to ionizing radiation. Front. Oncol. 2, 214. Epub 2013/01/25. doi:10.3389/fonc.2012.00214
Lee H. J., Hore T. A., Reik W. (2014). Reprogramming the methylome: Erasing memory and creating diversity. Cell stem Cell 14, 710–719. Epub 2014/06/07. doi:10.1016/j.stem.2014.05.008
Lee T. I., Young R. A. (2013). Transcriptional regulation and its misregulation in disease. Cell. Mar. 14 (152), 1237–1251. Epub 2013/03/19. doi:10.1016/j.cell.2013.02.014
Li Y., Shin D., Kwon S. H. (2013). Histone deacetylase 6 plays a role as a distinct regulator of diverse cellular processes. FEBS J. 280, 775–793. Epub 2012/11/28. doi:10.1111/febs.12079
Li L., Zhang T., Qin X. S., Ge W., Ma H. G., Sun L. L., et al. (2014). Exposure to diethylhexyl phthalate (DEHP) results in a heritable modification of imprint genes DNA methylation in mouse oocytes. Mol. Biol. Rep 41, 1227–1235. Epub 2014/01/07. doi:10.1007/s11033-013-2967-7
Li M., Peng J., Zeng Z. (2020a). Overexpression of long non-coding RNA nuclear enriched abundant transcript 1 inhibits the expression of p53 and improves premature ovarian failure. Exp. Ther. Med 20, 69. Epub 2020/09/24. doi:10.3892/etm.2020.9197
Li X., Xie J., Wang Q., Cai H., Xie C., Fu X. (2020b). miR-21 and pellino-1 expression profiling in autoimmune premature ovarian insufficiency. J. Immunol. Res. 2020, 3582648. Epub 2020/05/01. doi:10.1155/2020/3582648
Li C. J., Lin L. T., Tsai H. W., Chern C. U., Wen Z. H., Wang P. H., et al. (2021a). The molecular regulation in the pathophysiology in ovarian aging. Aging Dis 12, 934–949. Epub 2021/06/08. doi:10.14336/AD.2020.1113
Li Y., Chen X., Lu C. (2021b). The interplay between DNA and histone methylation: Molecular mechanisms and disease implications. EMBO Rep. 22, e51803. Epub 2021/04/13. doi:10.15252/embr.202051803
Liehr T., Ziegler M., Löhmer S., Weise A. (2018). Assessing skewed X-chromosome inactivation. Curr. Protoc. Hum. Genet. 10, e66. Jul Epub 2018/07/11. doi:10.1002/cphg.66
Ling C., Rönn T. (2019). Epigenetics in human obesity and type 2 diabetes. Cell metab. 29, 1028–1044. Epub 2019/04/16. doi:10.1016/j.cmet.2019.03.009
Liu J., Yao W., Yao Y., Du X., Zhou J., Ma B., et al. (2014). MiR-92a inhibits porcine ovarian granulosa cell apoptosis by targeting Smad7 gene. FEBS Lett. 588 (588), 4497–4503. Epub 2014/12/03. doi:10.1016/j.febslet.2014.10.021
Liu J., Tu F., Yao W., Li X., Xie Z., Liu H., et al. (2016). Conserved miR-26b enhances ovarian granulosa cell apoptosis through HAS2-HA-CD44-Caspase-3 pathway by targeting HAS2. Sci. Rep. 6 (6), 21197. Epub 2016/02/19. doi:10.1038/srep21197
Liu J., Li X., Yao Y., Li Q., Pan Z., Li Q (2018a). miR-1275 controls granulosa cell apoptosis and estradiol synthesis by impairing LRH-1/CYP19A1 axis. Biochimica et biophysica acta Gene regulatory mechanisms. 246–257. Epub 2018/01/30.
Liu J., Zhang W., Wu Z., Dai L., Koji T. (2018b). Changes in DNA methylation of oocytes and granulosa cells assessed by HELMET during folliculogenesis in mouse ovary. Acta Histochem. Cytochem. 51, 93–100. Epub 2018/06/06. doi:10.1267/ahc.17039
Liu X., Nie Z. W., Gao Y. Y., Chen L., Yin S. Y., Zhang X., et al. (2018c). Sodium fluoride disturbs DNA methylation of NNAT and declines oocyte quality by impairing glucose transport in porcine oocytes. Environ. Mol. Mutagen. 59, 223–233. Epub 2017/12/30. doi:10.1002/em.22165
Liu C., Li Q., Yang Y. (2019a). Effects of the modified bazhen decoction in the treatment of premature ovarian failure in rats. Ann. Clin. laboratory Sci. Jan 49, 16–22. doi:10.1016/j.cmet.2019.03.009 Epub 2019/03/01.
Liu T., Liu Y., Huang Y., Chen J., Yu Z., Chen C., et al. (2019b). miR-15b induces premature ovarian failure in mice via inhibition of α-Klotho expression in ovarian granulosa cells. Free Radic. Biol. Med. 141, 383–392. Epub 2019/07/17. doi:10.1016/j.freeradbiomed.2019.07.010
Liu L., Wang H., Xu G. L., Liu L. (2021a). Tet1 deficiency leads to premature ovarian failure. Front. Cell Dev. Biol. 9, 644135. Epub 2021/04/10. doi:10.3389/fcell.2021.644135
Liu T., Jing F., Huang P., Geng Z., Xu J., Li J., et al. (2021b). Thymopentin alleviates premature ovarian failure in mice by activating YY2/Lin28A and inhibiting the expression of let-7 family microRNAs. Cell Prolif. 54, e13089. Epub 2021/06/29. doi:10.1111/cpr.13089
Liu T., Lin J., Chen C., Nie X., Dou F., Chen J., et al. (2021c). MicroRNA-146b-5p overexpression attenuates premature ovarian failure in mice by inhibiting the Dab2ip/Ask1/p38-Mapk pathway and γH2A.X phosphorylation. Cell Prolif. 54, e12954. Epub 2020/11/10. doi:10.1111/cpr.12954
Lyko F. (2018). The DNA methyltransferase family: A versatile toolkit for epigenetic regulation. Nat. Rev. Genet. Feb 19, 81–92. Epub 2017/10/17. doi:10.1038/nrg.2017.80
Ma M., Zhang J., Gao X., Yao W., Li Q., Pan Z. (2020). miR-361-5p mediates SMAD4 to promote porcine granulosa cell apoptosis through VEGFA. Biomolecules 10, 1281. Epub 2020/09/10. doi:10.3390/biom10091281
Ma P., Schultz R. M. (2016). HDAC1 and HDAC2 in mouse oocytes and preimplantation embryos: Specificity versus compensation. Cell death Differ. 23, 1119–1127. Epub 2016/04/16. doi:10.1038/cdd.2016.31
Maegawa S., Hinkal G., Kim H. S., Shen L., Zhang L., Zhang J., et al. (2010). Widespread and tissue specific age-related DNA methylation changes in mice. Genome Res. 20, 332–340. Epub 2010/01/29. doi:10.1101/gr.096826.109
Manosalva I., González A. (2009). Aging alters histone H4 acetylation and CDC2A in mouse germinal vesicle stage oocytes. Biol. reproduction 81, 1164–1171. Epub 2009/07/31. doi:10.1095/biolreprod.109.078386
Manosalva I., González A., Gonzalez A. (2010). Aging changes the chromatin configuration and histone methylation of mouse oocytes at germinal vesicle stage. Theriogenology 74, 1539–1547. Epub 2010/08/24. doi:10.1016/j.theriogenology.2010.06.024
Marshall K. L., Rivera R. M. (2018). The effects of superovulation and reproductive aging on the epigenome of the oocyte and embryo. Mol. reproduction Dev. 85, 90–105. Epub 2017/12/28. doi:10.1002/mrd.22951
Marshall K. L., Wang J., Ji T., Rivera R. M. (2018). The effects of biological aging on global DNA methylation, histone modification, and epigenetic modifiers in the mouse germinal vesicle stage oocyte. Anim. Reprod. 15, 1253–1267. Epub 2018/12/05. doi:10.21451/1984-3143-AR2018-0087
Matoba S., Liu Y., Lu F., Iwabuchi K. A., Shen L., Inoue A., et al. (2014). Embryonic development following somatic cell nuclear transfer impeded by persisting histone methylation. Cell 6 (159), 884–895. Epub 2014/11/25. doi:10.1016/j.cell.2014.09.055
Matthews B. G., Bowden N. A., Wong-Brown M. W. (2021). Epigenetic mechanisms and therapeutic targets in chemoresistant high-grade serous ovarian cancer. Cancers 29, 5993. Epub 2021/12/11. doi:10.3390/cancers13235993
Mattiroli F., Penengo L. (2021). Histone ubiquitination: An integrative signaling platform in genome stability. Trends Genet. TIG 37, 566–581. Epub 2021/01/25. doi:10.1016/j.tig.2020.12.005
Metheetrairut C., Slack F. J. (2013). MicroRNAs in the ionizing radiation response and in radiotherapy. Curr. Opin. Genet. Dev. 23, 12–19. Epub 2013/03/05. doi:10.1016/j.gde.2013.01.002
Mezzanzanica D., Canevari S., Cecco L. D., Bagnoli M. (2011). miRNA control of apoptotic programs: Focus on ovarian cancer. Expert Rev. Mol. diagnostics 11, 277–286. Epub 2011/04/06. doi:10.1586/erm.11.1
Miousse I. R., Kutanzi K. R., Koturbash I. (2017). Effects of ionizing radiation on DNA methylation: From experimental biology to clinical applications. Int. J. Radiat. Biol. 93, 457–469. Epub 2017/01/31. doi:10.1080/09553002.2017.1287454
Moolhuijsen L. M. E., Visser J. A. (2020). Anti-müllerian hormone and ovarian reserve: Update on assessing ovarian function. J. Clin. Endocrinol. metabolism 105, 3361–3373. Epub 2020/08/10. doi:10.1210/clinem/dgaa513
Nebbioso A., Tambaro F. P., Dell'Aversana C., Altucci L. (2018). Cancer epigenetics: Moving forward. PLoS Genet. 14, e1007362. Epub 2018/06/08. doi:10.1371/journal.pgen.1007362
Nervi C., De Marinis E., Codacci-Pisanelli G. (2015). Epigenetic treatment of solid tumours: A review of clinical trials. Clin. epigenetics 7, 127. Epub 2015/12/23. doi:10.1186/s13148-015-0157-2
Nilsson E., Klukovich R., Sadler-Riggleman I., Beck D., Xie Y., Yan W., et al. (2018). Environmental toxicant induced epigenetic transgenerational inheritance of ovarian pathology and granulosa cell epigenome and transcriptome alterations: Ancestral origins of polycystic ovarian syndrome and primary ovarian insufiency. Epigenetics 13, 875–895. Epub 2018/09/13. doi:10.1080/15592294.2018.1521223
Nobile V., Pucci C., Chiurazzi P., Neri G., Tabolacci E. (2021). DNA methylation, mechanisms of FMR1 inactivation and therapeutic perspectives for fragile X syndrome. Biomolecules 11, 296. Epub 2021/03/07. doi:10.3390/biom11020296
Pan H., Ma P., Zhu W., Schultz R. M. (2008). Age-associated increase in aneuploidy and changes in gene expression in mouse eggs. Dev. Biol. 316, 397–407. Epub 2008/03/18. doi:10.1016/j.ydbio.2008.01.048
Pasyukova E. G., Symonenko A. V., Rybina O. Y., Vaiserman A. M. (2021). Epigenetic enzymes: A role in aging and prospects for pharmacological targeting. Ageing Res. Rev. 67, 101312. Epub 2021/03/04. doi:10.1016/j.arr.2021.101312
Peñaherrera M. S., Barrett I. J., Brown C. J., Langlois S., Yong S. L., Lewis S., et al. (2000). An association between skewed X-chromosome inactivation and abnormal outcome in mosaic trisomy 16 confined predominantly to the placenta. Clin. Genet. 58, 436–446. Epub 2001/01/10. doi:10.1034/j.1399-0004.2000.580603.x
Petrussa L., Van de Velde H., De Rycke M. (2014). Dynamic regulation of DNA methyltransferases in human oocytes and preimplantation embryos after assisted reproductive technologies. Mol. Hum. Reprod. 20, 861–874. Epub 2014/07/06. doi:10.1093/molehr/gau049
Pogribny I., Koturbash I., Tryndyak V., Hudson D., Stevenson S. M., Sedelnikova O., et al. (2005). Fractionated low-dose radiation exposure leads to accumulation of DNA damage and profound alterations in DNA and histone methylation in the murine thymus. Mol. cancer Res. MCR 3, 553–561. Epub 2005/10/29. doi:10.1158/1541-7786.MCR-05-0074
Prachayasittikul V., Prathipati P., Pratiwi R., Phanus-Umporn C., Malik A. A., Schaduangrat N., et al. (2017). Exploring the epigenetic drug discovery landscape. Expert Opin. drug Discov. 12, 345–362. Epub 2017/03/10. doi:10.1080/17460441.2017.1295954
Pu D., Wu J., Liu J. (2010). Skewed X chromosome inactivation may be not associated with premature ovarian failure. Gynecol. Endocrinol. official J. Int. Soc. Gynecol. Endocrinol. 26, 423–428. Epub 2010/02/23. doi:10.3109/09513591003632217
Qian Y., Tu J., Tang N. L., Kong G. W., Chung J. P., Chan W. Y., et al. (2015). Dynamic changes of DNA epigenetic marks in mouse oocytes during natural and accelerated aging. Int. J. Biochem. Cell Biol. 67, 121–127. Epub 2015/05/20. doi:10.1016/j.biocel.2015.05.005
Qin Y., Jiao X., Simpson J. L., Chen Z. J. (2015). Genetics of primary ovarian insufficiency: New developments and opportunities. Hum. Reprod. Update 21, 787–808. Epub 2015/08/06. doi:10.1093/humupd/dmv036
Qiu J., Sun Y., Sun W., Wang Y., Fan T., Yu J. (2020). Neonatal exposure to bisphenol A advances pubertal development in female rats. Mol. reproduction Dev. 87, 503–511. Epub 2020/02/29. doi:10.1002/mrd.23329
Quan N., Harris L. R., Halder R., Trinidad C. V., Johnson B. W., Horton S., et al. (2020). Differential sensitivity of inbred mouse strains to ovarian damage in response to low-dose total body irradiation. Biol. reproduction 102, 133–144. Epub 2019/08/23. doi:10.1093/biolre/ioz164
Rah H., Jeon Y. J., Shim S. H., Cha S. H., Choi D. H., Kwon H., et al. (2013). Association of miR-146aC>G, miR-196a2T>C, and miR-499A>G polymorphisms with risk of premature ovarian failure in Korean women. Repord Sci. 20, 60–68. Epub 2012/08/09. doi:10.1177/1933719112450341
Reddy P., Zheng W., Liu K. (2010). Mechanisms maintaining the dormancy and survival of mammalian primordial follicles. Trends Endocrinol. metabolism TEM 21, 96–103. Epub 2009/11/17. doi:10.1016/j.tem.2009.10.001
Rhon-Calderón E. A., Galarza R. A., Lomniczi A., Faletti A. G. (2016). The systemic and gonadal toxicity of 3-methylcholanthrene is prevented by daily administration of α-naphthoflavone. Toxicology 353, 58–69. Epub 2016/05/11.
Rhon-Calderón E. A., Toro C. A., Lomniczi A., Galarza R. A., Faletti A. G. (2018). Changes in the expression of genes involved in the ovarian function of rats caused by daily exposure to 3-methylcholanthrene and their prevention by α-naphthoflavone. Archives Toxicol. 92, 907–919. Epub 2017/11/03. doi:10.1007/s00204-017-2096-5
Richardson M. C., Guo M., Fauser B. C., Macklon N. S. (2014). Environmental and developmental origins of ovarian reserve. Hum. Reprod. Update 20, 353–369. Epub 2013/11/30. doi:10.1093/humupd/dmt057
Rinaldi L., Datta D., Serrat J., Morey L., Solanas G., Avgustinova A., et al. (2016). Dnmt3a and Dnmt3b associate with enhancers to regulate human epidermal stem cell homeostasis. Cell stem Cell 19, 491–501. Epub 2016/08/02. doi:10.1016/j.stem.2016.06.020
Safi-Stibler S., Gabory A. (2020). Epigenetics and the Developmental Origins of Health and Disease: Parental environment signalling to the epigenome, critical time windows and sculpting the adult phenotype. Seminars Cell & Dev. Biol. 97, 172–180. Epub 2019/10/08. doi:10.1016/j.semcdb.2019.09.008
Sato K., Uehara S., Hashiyada M., Nabeshima H., Sugawara J., Terada Y., et al. (2004). Genetic significance of skewed X-chromosome inactivation in premature ovarian failure. Am. J. Med. Genet. Part A 130a, 240–244. Epub 2004/09/21. doi:10.1002/ajmg.a.30256
Shao G. B., Wang J., Zhang L. P., Wu C. Y., Jin J., Sang J. R., et al. (2015). Aging alters histone H3 lysine 4 methylation in mouse germinal vesicle stage oocytes. Reproduction, Fertil. Dev. 27, 419–426. Epub 2014/01/05. doi:10.1071/RD13293
Skvortsova K., Iovino N., Bogdanović O. (2018). Functions and mechanisms of epigenetic inheritance in animals. Nat. Rev. Mol. Cell Biol. 19, 774–790. Epub 2018/11/15. doi:10.1038/s41580-018-0074-2
Spath M. A., Nillesen W. N., Smits A. P., Feuth T. B., Braat D. D., van Kessel A. G., et al. (2010). X chromosome inactivation does not define the development of premature ovarian failure in fragile X premutation carriers. Am. J. Med. Genet. Part A 152a, 387–393. Epub 2010/01/27. doi:10.1002/ajmg.a.33243
Sullivan S. D., Sarrel P. M., Nelson L. M. (2016). Hormone replacement therapy in young women with primary ovarian insufficiency and early menopause. Fertil. Steril. 106, 1588–1599. Epub 2016/12/04. doi:10.1016/j.fertnstert.2016.09.046
Sun B., Ma Y., Wang F., Hu L., Sun Y. (2019). miR-644-5p carried by bone mesenchymal stem cell-derived exosomes targets regulation of p53 to inhibit ovarian granulosa cell apoptosis. Stem Cell Res. Ther. 29 (10), 360. Epub 2019/12/01. doi:10.1186/s13287-019-1442-3
Sun Y. C., Sun X. F., Dyce P. W., Shen W., Chen H. (2017). The role of germ cell loss during primordial follicle assembly: A review of current advances. Int. J. Biol. Sci. 13, 449–457. Epub 2017/05/23. doi:10.7150/ijbs.18836
Sun Y. C., Wang Y. Y., Sun X. F., Cheng S. F., Li L., Zhao Y., et al. (2018). The role of autophagy during murine primordial follicle assembly. Aging 510, 197–211. Epub 2018/02/08. doi:10.18632/aging.101376
Suo L., Meng Q. G., Pei Y., Yan C. L., Fu X. W., Bunch T. D., et al. (2010). Changes in acetylation on lysine 12 of histone H4 (acH4K12) of murine oocytes during maternal aging may affect fertilization and subsequent embryo development. Fertil. Steril. 93, 945–951. Epub 2009/03/17. doi:10.1016/j.fertnstert.2008.12.128
Swain J. E., Ding J., Brautigan D. L., Villa-Moruzzi E., Smith G. D. (2007). Proper chromatin condensation and maintenance of histone H3 phosphorylation during mouse oocyte meiosis requires protein phosphatase activity. Biol. reproduction 76, 628–638. Epub 2006/12/22. doi:10.1095/biolreprod.106.055798
Tesfaye D., Gebremedhn S., Salilew-Wondim D., Hailay T., Hoelker M., Grosse-Brinkhaus C., et al. (2018). MicroRNAs: Tiny molecules with a significant role in mammalian follicular and oocyte development. Reprod. Camb. Engl. 155, R121-R135–r135. Epub 2017/11/25. doi:10.1530/REP-17-0428
Tomizawa S., Kobayashi H., Watanabe T., Andrews S., Hata K., Kelsey G., et al. (2011). Dynamic stage-specific changes in imprinted differentially methylated regions during early mammalian development and prevalence of non-CpG methylation in oocytes. Development. 138, 811–820. Epub 2011/01/21. doi:10.1242/dev.061416
Tüysüz B., Güneş N., Geyik F., Yeşil G., Celkan T., Vural M. (2021). Investigation of (epi)genotype causes and follow-up manifestations in the patients with classical and atypical phenotype of Beckwith-Wiedemann spectrum. Am. J. Med. Genet. Part A 185, 1721–1731. Epub 2021/03/12. doi:10.1002/ajmg.a.62158
Uysal F., Ozturk S., Akkoyunlu G. (2017). DNMT1, DNMT3A and DNMT3B proteins are differently expressed in mouse oocytes and early embryos. J. Mol. histology 48, 417–426. Epub 2017/10/14. doi:10.1007/s10735-017-9739-y
Vabre P., Gatimel N., Moreau J., Gayrard V., Picard-Hagen N., Parinaud J., et al. (2017). Environmental pollutants, a possible etiology for premature ovarian insufficiency: A narrative review of animal and human data. Environ. health a Glob. access Sci. source 16, 37. Epub 2017/04/09. doi:10.1186/s12940-017-0242-4
van den Berg I. M., Eleveld C., van der Hoeven M., Birnie E., Steegers E. A., Galjaard R. J., et al. (2011). Defective deacetylation of histone 4 K12 in human oocytes is associated with advanced maternal age and chromosome misalignment. Hum. Reprod. Oxf. Engl. 26, 1181–1190. Epub 2011/02/26. doi:10.1093/humrep/der030
Vaquero A., Scher M., Erdjument-Bromage H., Tempst P., Serrano L., Reinberg D. (2007). SIRT1 regulates the histone methyl-transferase SUV39H1 during heterochromatin formation. Nature 15 (450), 440–444. Epub 2007/11/16. doi:10.1038/nature06268
Verdel A., Curtet S., Brocard M. P., Rousseaux S., Lemercier C., Yoshida M., et al. (2000). Active maintenance of mHDA2/mHDAC6 histone-deacetylase in the cytoplasm. Curr. Biol. CB 10, 747–749. Epub 2000/06/30. doi:10.1016/s0960-9822(00)00542-x
Verdone L., Caserta M., Di Mauro E. (2005). Role of histone acetylation in the control of gene expression. Biochem. Cell Biol. 83, 344–353. Epub 2005/06/17. doi:10.1139/o05-041
Vujovic S. (2009). Aetiology of premature ovarian failure. Menopause Int. 15, 72–75. Epub 2009/05/26. doi:10.1258/mi.2009.009020
Wang C., Li D., Zhang S., Xing Y., Gao Y., Wu J. (2016). MicroRNA-125a-5p induces mouse granulosa cell apoptosis by targeting signal transducer and activator of transcription 3. Menopause 23, 100–107. Epub 2015/07/15. doi:10.1097/GME.0000000000000507
Wang H., Cai H., Wang X., Zhang M., Liu B., Chen Z., et al. (2019). HDAC3 maintains oocyte meiosis arrest by repressing amphiregulin expression before the LH surge. Nat. Commun. 10, 5719. Epub 2019/12/18. doi:10.1038/s41467-019-13671-8
Wang H., Liu L., Gou M., Huang G., Tian C., Yang J., et al. (2021a). Roles of Tet2 in meiosis, fertility and reproductive aging. Protein & Cell 12, 578–585. Epub 2020/11/21. doi:10.1007/s13238-020-00805-8
Wang J., Liu W., Yu D., Yang Z., Li S., Sun X. (2021b). Research progress on the treatment of premature ovarian failure using mesenchymal stem cells: A literature review. Front. Cell Dev. Biol. 9, 749822. Epub 2021/12/31. doi:10.3389/fcell.2021.749822
Webber L., Davies M., Anderson R., Bartlett J., Braat D., Cartwright B., et al. (2016). ESHRE guideline: Management of women with premature ovarian insufficiency. Hum. Reprod. Oxf. Engl. 31, 926–937. Epub 2016/03/25.
Wu X., Zhang Y. (2017). TET-Mediated active DNA demethylation: Mechanism, function and beyond. Nat. Rev. Genet. 18, 517–534. Epub 2017/05/31. doi:10.1038/nrg.2017.33
Xiao G. Y., Cheng C. C., Chiang Y. S., Cheng W. T., Liu I. H., Wu S. C. (2016). Exosomal miR-10a derived from amniotic fluid stem cells preserves ovarian follicles after chemotherapy. Sci. Rep. 6 (6), 23120. Epub 2016/03/17. doi:10.1038/srep23120
Xing M., Chen X., Li X., Yang Y., Wang X., Cao X., et al. (2018). Histone deacetylase inhibitor stimulates E2 and P4 secretion in sika deer ovarian granulosa cells at a moderate dose. Cell Biol. Int. 42, 324–333. Epub 2017/10/25. doi:10.1002/cbin.10899
Xiong F., Hu L., Zhang Y., Xiao X., Xiao J. (2016). miR-22 inhibits mouse ovarian granulosa cell apoptosis by targeting SIRT1. Biol. open 5, 367–371. Epub 2016/02/26. doi:10.1242/bio.016907
Xiong Y., Liu T., Wang S., Chi H., Chen C., Zheng J. (2017). Cyclophosphamide promotes the proliferation inhibition of mouse ovarian granulosa cells and premature ovarian failure by activating the lncRNA-Meg3-p53-p66Shc pathway. Gene 5, 1–8. Epub 2016/10/13. doi:10.1016/j.gene.2016.10.011
Xu H., Yu H., Jin R., Wu X., Chen H. (2021). Genetic and epigenetic targeting therapy for pediatric acute lymphoblastic leukemia. Cells 10, 3349. Epub 2021/12/25. doi:10.3390/cells10123349
Yamaguchi S., Hong K., Liu R., Shen L., Inoue A., Diep D., et al. (2012). Tet1 controls meiosis by regulating meiotic gene expression. Nature 492, 443–447. Epub 2012/11/16. doi:10.1038/nature11709
Yang F., Baumann C., Viveiros M. M., De La Fuente R. (2012a). Histone hyperacetylation during meiosis interferes with large-scale chromatin remodeling, axial chromatid condensation and sister chromatid separation in the mammalian oocyte. Int. J. Dev. Biol. 56, 889–899. Epub 2013/02/19. doi:10.1387/ijdb.120246rd
Yang X., Zhou Y., Peng S., Wu L., Lin H. Y., Wang S., et al. (2012b). Differentially expressed plasma microRNAs in premature ovarian failure patients and the potential regulatory function of mir-23a in granulosa cell apoptosis. Reprod. Camb. Engl. 144, 235–244. Epub 2012/06/02. doi:10.1530/REP-11-0371
Yang X., Gao L., Guo X., Shi X., Wu H., Song F., et al. (2014). A network based method for analysis of lncRNA-disease associations and prediction of lncRNAs implicated in diseases. PloS one 9, e87797. Epub 2014/02/06. doi:10.1371/journal.pone.0087797
Yang S., Ding S., He S., He L., Gao K., Peng S., et al. (2019). Differentiation of primordial germ cells from premature ovarian insufficiency-derived induced pluripotent stem cells. Stem Cell Res. Ther. 10, 156. Epub 2019/06/04. doi:10.1186/s13287-019-1261-6
Yang M., Lin L., Sha C., Li T., Zhao D., Wei H., et al. (2020). Bone marrow mesenchymal stem cell-derived exosomal miR-144-5p improves rat ovarian function after chemotherapy-induced ovarian failure by targeting PTEN. Lab. Invest. 100, 342–352. Epub 2019/09/21. doi:10.1038/s41374-019-0321-y
Yang Y., Huang W., Yuan L. (2021). Effects of environment and lifestyle factors on premature ovarian failure. Adv. Exp. Med. Biol. 1300, 63–111. Epub 2021/02/02. doi:10.1007/978-981-33-4187-6_4
Yao W., Pan Z., Du X., Zhang J., Liu H., Li Q. (2021). NORHA, a novel follicular atresia-related lncRNA, promotes porcine granulosa cell apoptosis via the miR-183-96-182 cluster and FoxO1 axis. J. animal Sci. Biotechnol. 12, 103. Epub 2021/10/08. doi:10.1186/s40104-021-00626-7
Ye Q., Zeng X., Wang S., Zeng X., Yang G., Ye C., et al. (2021). Butyrate drives the acetylation of histone H3K9 to activate steroidogenesis through PPARγ and PGC1α pathways in ovarian granulosa cells. FASEB J. 35, e21316. Epub 2021/01/13. doi:10.1096/fj.202000444R
Yin Y., Morgunova E., Jolma A., Kaasinen E., Sahu B., Khund-Sayeed S., et al. (2017). Impact of cytosine methylation on DNA binding specificities of human transcription factors. Science 356, eaaj2239. Epub 2017/05/06. doi:10.1126/science.aaj2239
Yoon S. H., Choi Y. M., Hong M. A., Kang B. M., Kim J. J., Min E. G., et al. (2008). X chromosome inactivation patterns in patients with idiopathic premature ovarian failure. HumRepord. 23, 688–692. Epub 2008/01/10. doi:10.1093/humrep/dem415
Yu B., Dong X., Gravina S., Kartal Ö., Schimmel T., Cohen J., et al. (2017). Genome-wide, single-cell DNA methylomics reveals increased non-CpG methylation during human oocyte maturation. Stem Cell Rep. 9, 397–407. Epub 2017/06/27. doi:10.1016/j.stemcr.2017.05.026
Yu B., Russanova V. R., Gravina S., Hartley S., Mullikin J. C., Ignezweski A., et al. (2015). DNA methylome and transcriptome sequencing in human ovarian granulosa cells links age-related changes in gene expression to gene body methylation and 3'-end GC density. Oncotarget 28 (6), 3627–3643. Epub 2015/02/17. doi:10.18632/oncotarget.2875
Yu Y., Zhang Q., Sun K., Xiu Y., Wang X., Wang K., et al. (2022). Long non-coding RNA BBOX1 antisense RNA 1 increases the apoptosis of granulosa cells in premature ovarian failure by sponging miR-146b. Bioengineered 13, 6092–6099. Epub 2022/02/22. doi:10.1080/21655979.2022.2031675
Yue M. X., Fu X. W., Zhou G. B., Hou Y. P., Du M., Wang L., et al. (2012). Abnormal DNA methylation in oocytes could be associated with a decrease in reproductive potential in old mice. J. assisted reproduction Genet. 29, 643–650. Epub 2012/05/24. doi:10.1007/s10815-012-9780-4
Zama A. M., Uzumcu M. (2009). Fetal and neonatal exposure to the endocrine disruptor methoxychlor causes epigenetic alterations in adult ovarian genes. Endocrinol. Oct. 150, 4681–4691. Epub 2009/07/11. doi:10.1210/en.2009-0499
Zhang X., Yuan Z., Zhang Y., Yong S., Salas-Burgos A., Koomen J., et al. (2012). HDAC6 modulates cell motility by altering the acetylation level of cortactin. Mol. Cell 27, 197–213. Epub 2007/07/24. doi:10.1016/j.molcel.2007
Zhang X. F., Zhang L. J., Feng Y. N., Chen B., Feng Y. M., Liang G. J., et al. (2012). Bisphenol A exposure modifies DNA methylation of imprint genes in mouse fetal germ cells. Mol. Biol. Rep. 39, 8621–8628. Epub 2012/06/16. doi:10.1007/s11033-012-1716-7
Zhang Q., Sun H., Jiang Y., Ding L., Wu S., Fang T., et al. (2013). MicroRNA-181a suppresses mouse granulosa cell proliferation by targeting activin receptor IIA. PloS one 8, e59667. Epub 2013/03/26. doi:10.1371/journal.pone.0059667
Zhang L., Hou X., Ma R., Moley K., Schedl T., Wang Q. (2014). Sirt2 functions in spindle organization and chromosome alignment in mouse oocyte meiosis. FASEB J. official Publ. Fed. Am. Soc. Exp. Biol. 28, 1435–1445. Epub 2013/12/18. doi:10.1096/fj.13-244111
Zhang X., Yang J., Wang H., Guo R., Yin Y., Zhang D., et al. (2017). Overexpression of Hdac6 extends reproductive lifespan in mice. Protein & Cell 8, 360–364. Epub 2017/03/03. doi:10.1007/s13238-017-0375-9
Zhang C., Shen J., Kong S., Zhang M., Zhang Q., Zhou J., et al. (2019a). MicroRNA-181a promotes follicular granulosa cell apoptosis via sphingosine-1-phosphate receptor 1 expression downregulation. Biol. reproduction 101 (101), 975–985. Epub 2019/07/31. doi:10.1093/biolre/ioz135
Zhang J., Xu Y., Liu H., Pan Z. (2019b). MicroRNAs in ovarian follicular atresia and granulosa cell apoptosis. Reproductive Biol. Endocrinol. RB&E 17, 9. Epub 2019/01/12. doi:10.1186/s12958-018-0450-y
Zhang T., Du X., Zhao L., He M., Lin L., Guo C., et al. (2019c). SIRT1 facilitates primordial follicle recruitment independent of deacetylase activity through directly modulating Akt1 and mTOR transcription. FASEB J. official Publ. Fed. Am. Soc. Exp. Biol. 33, 14703–14716. Epub 2019/11/07. doi:10.1096/fj.201900782R
Zhang X., Dang Y., Liu R., Zhao S., Ma J., Qin Y. (2020). MicroRNA-127-5p impairs function of granulosa cells via HMGB2 gene in premature ovarian insufficiency. J. Cell. physiology 235, 8826–8838. Epub 2020/05/12. doi:10.1002/jcp.29725
Zhang T., He M., Zhao L., Qin S., Zhu Z., Du X., et al. (2021). HDAC6 regulates primordial follicle activation through mTOR signaling pathway. Cell death Dis. 12, 559. Epub 2021/05/31. doi:10.1038/s41419-021-03842-1
Zhang L., Mao B., Zhao X., Yuan Y., Wang W., Lin S. (2022). Translation regulatory long non-coding RNA 1 (TRERNA1) sponges microRNA-23a to suppress granulosa cell apoptosis in premature ovarian failure. Bioengineered 13, 2173–2180. Epub 2022/01/18. doi:10.1080/21655979.2021.2023802
Zhao G., Yan G., Cheng J., Zhou X., Fang T., Sun H., et al. (2015). Hyaluronic acid prevents immunosuppressive drug-induced ovarian damage via up-regulating PGRMC1 expression. Sci. Rep. 5 (5), 7647. Epub 2015/01/07. doi:10.1038/srep07647
Zhao G., Zhou X., Fang T., Hou Y., Hu Y. (2014). Hyaluronic acid promotes the expression of progesterone receptor membrane component 1 via epigenetic silencing of miR-139-5p in human and rat granulosa cells. Biol. reproduction 91, 116. Epub 2014/09/19. doi:10.1095/biolreprod.114.120295
Zhao W., Dong L. (2018). Long non-coding RNA HOTAIR overexpression improves premature ovarian failure by upregulating Notch-1 expression. Exp. Ther. Med. 16, 4791–4795. Epub 2018/12/14. doi:10.3892/etm.2018.6750
Zheng C., Liu S., Qin Z., Zhang X., Song Y. (2021). LncRNA DLEU1 is overexpressed in premature ovarian failure and sponges miR-146b-5p to increase granulosa cell apoptosis. J. ovarian Res. 14, 14151. Epub 2021/11/07. doi:10.1186/s13048-021-00905-x
Zhou J., Liu J., Pan Z., Du X., Li X., Ma B., et al. (2015). The let-7g microRNA promotes follicular granulosa cell apoptosis by targeting transforming growth factor-β type 1 receptor. Mol. Cell. Endocrinol. 409, 103–112. Epub 2015/03/31. doi:10.1016/j.mce.2015.03.012
Zhu D., Zhang Y., Wang S. (2021). Histone citrullination: A new target for tumors. Mol. cancer 20, 90. Epub 2021/06/13. doi:10.1186/s12943-021-01373-z
Keywords: premature ovarian failure, epigenetics, DNA methylation, histone modifications, non-coding RNA
Citation: Wang J, Sun X, Yang Z, Li S, Wang Y, Ren R, Liu Z and Yu D (2023) Epigenetic regulation in premature ovarian failure: A literature review. Front. Physiol. 13:998424. doi: 10.3389/fphys.2022.998424
Received: 01 August 2022; Accepted: 14 December 2022;
Published: 04 January 2023.
Edited by:
Elisabeth Pinart, University of Girona, SpainReviewed by:
Haicui Wu, Affiliated Hospital of Shandong University of Traditional Chinese Medicine, ChinaHong Zan, The University of Texas Health Science Center at San Antonio, United States
Copyright © 2023 Wang, Sun, Yang, Li, Wang, Ren, Liu and Yu. This is an open-access article distributed under the terms of the Creative Commons Attribution License (CC BY). The use, distribution or reproduction in other forums is permitted, provided the original author(s) and the copyright owner(s) are credited and that the original publication in this journal is cited, in accordance with accepted academic practice. No use, distribution or reproduction is permitted which does not comply with these terms.
*Correspondence: Dehai Yu, yudehai@jlu.edu.cn