- Botany Department, University of Wyoming, Laramie, WY, United States
The isotopic composition of xylem water is frequently measured to identify sources of plant water uptake and evaluate the ecosystem water budget. The most common approach to sample xylem water is cryogenic vacuum distillation (CVD). However, the water recovered by CVD is total xylem water from the complex xylem tissue, including living xylem parenchyma cells, embolized tracheary conduits, and small or disconnected conduits that may have a different isotopic composition from water conducted through conduits of the dominant flow from roots to leaves. The isotopic composition of water in the dominant flow network is likely more representative of the isotopic composition of daily transpiration whereas the total xylem water likely integrates water with a longer residence time that may undergo exchange with organic compounds. An alternative extraction method using a pressure chamber (PC) can capture predominantly the transpiration-stream water through the dominant flow network. We compared the offsets in the isotopic composition of water recovered using CVD and PC from eight conifer species that vary in xylem anatomical and functional traits. The PC method accessed a significantly distinct isotopic domain of stem xylem water compared to the total xylem water accessed by CVD (δ2H, p = 0.012; δ18O, p = 0.028). The difference between δ2H of stem water extracted by PC and CVD methods (Δ2Hstem) was significantly correlated with stem water content (p = 0.048) and the mean Δ2Hstem for each species had a significant relationship with species-specific xylem vulnerability to cavitation (i.e., ψ50) from literature values (p = 0.030). We found a significant positive relationship between Δ2Hstem and Δ18Ostem across all trees sampled (p = <0.001). These results support the existence of isotopically heterogeneous water pools, but we cannot exclude potential CVD artifacts contributing to a portion of the Δ2Hstem offsets. Our data suggest additional mechanisms of incomplete mixing and variable residence time in xylem conduits may contribute to isotopic heterogeneity proposed by previous work. Future work should consider using the PC method for assessing the isotopic composition of daily scale transpiration and determining species-specific xylem anatomical properties that could explain isotopic differences between various xylem water pools.
Introduction
Transpiration is the dominant component of evapotranspiration returning water to the atmosphere over most landscapes on Earth (Schlesinger and Jasechko, 2014). Increases in transpiration with compensating reductions in streamflow and groundwater storage have already been linked to rising temperatures related to climate change in mountain ecosystems (Mastrotheodoros et al., 2020). Many areas may face increased water scarcity over the coming decades as temperatures warm and precipitation becomes more uncertain and variable (IPPC, 2014). Therefore, the tools we use to quantify components of the water budget and estimate water movement in terrestrial environments need to accurately and precisely reflect processes used in ecohydrologic models.
Water stable isotope measurements have been used extensively to track water movement through the soil-plant-atmosphere continuum (Penna et al., 2013; Sprenger et al., 2016), identify plant water sources (Ehleringer and Dawson, 1992; Barbeta et al., 2019), and validate estimates of evaporation and plant transpiration (Williams et al., 2004; Bowen et al., 2019). However, the extraction methods used to recover water from plant and soil samples for isotopic analysis have come under intense scrutiny because of unresolved questions about the proportion of total water recovered by each method and the possible isotope fractionations expressed during storage and extraction (Orlowski et al., 2016a; Newberry et al., 2017; Tsuruta et al., 2019; Chen et al., 2020). These questions have prompted many inter-method and inter-laboratory soil and plant water extraction comparisons (Orlowski et al., 2016b, 2018; Millar et al., 2018; Fischer et al., 2019).
The most common and widely accepted method for extracting total water from soil and plant samples for isotopic analysis is cryogenic vacuum distillation (CVD). Other methods for collecting water from plant and soil samples are available, such as direct vapor equilibration, centrifugation, high pressure mechanical squeezing, microwave extraction, and a newly developed cavitron method (Barbeta et al., 2022), but all have been less frequently employed than CVD. Thus far, the consensus from the comparisons of extraction methods for soil samples, as well as studies that have used a stepwise application and/or combination of extraction methods (Bowers et al., 2020; Orlowski and Breuer, 2020), is that each method can access various proportions of fast- and slow-flow soil water domains (Sprenger and Allen, 2020). Furthermore, these fast and slow domains can be isotopically distinct due to incomplete mixing and isotopic fractionations related to evaporation, soil particle wettability, and interactions with soil minerals, dissolved ions and organic matter content (Meißner et al., 2014; Oerter et al., 2014; Chen et al., 2016; Gaj et al., 2017, 2019; Gaj and McDonnell, 2019; Sprenger et al., 2019; Bowers et al., 2020; Orlowski and Breuer, 2020). Isotopic heterogeneity and exchange processes affecting bulk and component isotopic values of water in the transpiration stream in plant tissues has received much less attention (Knighton et al., 2020). This is primarily because of a longstanding assumption that water is unaltered and well-mixed during its transit from absorption surfaces in roots and up through the stem, such that the isotopic composition of the total water in the stem is representative of plant source water. Consequentially, the comparisons of plant water extraction methods thus far have primarily focused on non-woody species and differences in cost, difficulty, potential for co-extracted and spectroscopically interfering organic compounds, and the extraction apparatus and protocols rather than addressing the potential for isotopically heterogeneous plant water and variable removal of total plant water using each method (Millar et al., 2018; Fischer et al., 2019).
Millar et al. (2018) compared six extraction methods (i.e., direct vapor equilibration, centrifugation, two versions of CVD, high-pressure mechanical squeezing, and microwave extraction) for four plant portions (i.e., inflorescence, leaf, stem, and root) of spring wheat (Triticum aestivum). Notably, they discussed that high-pressure mechanical squeezing, centrifugation and CVD are all capable of accessing the total plant water pool whereas direct vapor equilibration is the most appropriate for investigating plant water sources and therefore more representative of transpiration. The total plant water pool is potentially different from the transpiration-stream plant water pool in that it could contain water from living parenchyma cells within the xylem. These parenchyma cells are not directly in the transpiration-stream and their primary role is carbohydrate and water storage, with possible additional roles in fungal defense (Morris et al., 2016a) and embolism recovery (Secchi et al., 2017). Since they are not primarily conducting water, they may have different isotopic water compositions due to fractionations associated with cellular metabolic activity, long residence time integrating previous plant water sources, and variable and limited exchange with conducting cells. In addition, the total plant water could contain water from tracheary elements that are embolized or downstream (i.e., closer to leaves) of an embolism and either not conducting water currently to the leaves or conducting to a lesser extent than the predominant flow paths from roots to leaves. Similarly, small tracheary elements could be simply conducting at a much lesser extent due to smaller conduit diameters as has been supported mathematically by the Hagen-Poiseuille equation (Hacke et al., 2017). Other than direct vapor equilibration and new cavitron method, sampling xylem sap using a Scholander-type pressure chamber (PC) is the only other method reported in the literature for acquiring transpiration-stream water from plant samples for isotopic analysis (Ellsworth and Williams, 2007; Penna et al., 2013; Geißler et al., 2019; Zuecco et al., 2020). While the Scholander-type pressure chamber (PC) has primarily been used for ecophysiological studies aiming to understand plant water stress by measuring pre-dawn and midday leaf water potentials (Tyree and Hammel, 1972; Carrière et al., 2020), it has the methodological advantage of sampling water primarily from the functional tracheary elements. Previous work using the PC method for isotopic analysis of xylem sap has demonstrated that the transpiration-stream water recovered from the PC method can be isotopically distinct from the total plant water recovered by CVD (Ellsworth and Williams, 2007; Zuecco et al., 2020). However, previous work has not investigated the role of xylem anatomy and functionality on these differences. For this study, we used published data on parenchyma content as a proxy of the influence of living cells in the complex woody tissue. Additionally, we used published data on vulnerability to cavitation (i.e., ψ50) as this parameter has been shown to integrate complex tradeoffs in anatomical structure and function of xylem tissue (Pratt and Jacobsen, 2017; Mrad et al., 2018). Lastly, recent work has raised concerns that hydrogen atoms of water molecules exchange with plant organic compounds and cause artifacts in the isotope composition of waters recovered by CVD (Millar et al., 2018; Chen et al., 2020). These proposed artifacts associated with the CVD method correspond to changes of δ2H values but not of δ18O values. Chen et al. (2020) concluded their differences in δ2H values between CVD and transpiration-stream water with the addition of a rehydration experiment failed to support the existence of isotopically heterogenous xylem water. However, recent work has suggested alternative surface- and aquaporin-mediated mechanisms that could produce similar changes to δ2H values before water is extracted by CVD and therefore in support of isotopic heterogeneity of xylem water (Zhao et al., 2016; Barbeta et al., 2020). It is also worth noting that previous work supporting CVD artifacts have not used mature trees. Millar et al. (2018) used wheat and Chen et al. (2020) used 2- to 4-year old saplings which both have a much smaller capacity for internal storage compared to mature trees that variably rely on internal storage (Cermak et al., 2007; Matheny et al., 2015). Thus, using mature trees and observing a covariation of H and O isotopes recovered by different methods may reflect the presence of isotopically heterogeneous pools of xylem water solely or in addition to the proposed CVD artifacts. If not properly quantified, the differences between the isotopic composition of total tissue water and that of the transpiration-stream water could greatly affect the estimation of sources of water used by trees and add significant errors to estimates of the ecosystem water budget.
This study proposes to quantify the degree that total water collected from stems of coniferous trees native to the central Rocky Mountains differs in stable isotope composition from that collected directly from the transpiration-stream and compare these differences to stem anatomical and hydraulic traits. The relative proportion of living cells was considered during the evaluation of each species by using previously reported data on woody tissue composition in conifers. In addition to the parenchyma content, stem water content and species-specific hydraulic vulnerabilities to cavitation, which both integrate many complex wood anatomical differences between species, were used to assess whether isotopic heterogeneity was related to hydraulic characteristics of each species. Specifically, our research aimed to: (1) quantify whether transpiration-stream and total plant xylem water were consistently and significantly different across coniferous tree species, (2) assess if these differences were related to stem water content as well as species-specific parenchyma cell content and xylem vulnerability to cavitation, and (3) assess with mature trees if these differences support isotopically heterogenous xylem water storage rather than simply method specific isotopic artifacts.
Materials and Methods
Study Areas and Time
This study presents findings from samples taken from trees at two locations in southeast Wyoming, USA. The first location where naturally occurring trees were sampled was a forested hillslope within the No Name Watershed of the Medicine Bow National Forest, located roughly 50 km west of Laramie, WY (henceforth referred to as “Med Bow”). The Med Bow location is roughly 2,950 meters above sea level and receives about 900 mm of precipitation annually, predominantly as snow. Sampling took place at the Med Bow location on September 2nd and 4th of 2020. The second location where artificially planted trees were sampled was on the main campus of the University of Wyoming, located in Laramie, WY (henceforth referred to as “Campus”). The Campus location is roughly 2190 meters above sea level and is well-watered by irrigation and receives additional precipitation of roughly 280 mm per year, predominantly as snowfall. Sampling took place at the Campus location September 14th, 16th, 18th, and 19th of 2020. On each sampling day and at each site, samples were collected on clear weather mornings while plants were active from 8:00 a.m. to 12:00 p.m.
Study Species and Plant Selection
Med Bow location included three native species of Lodgepole pine (Pinus contorta), Engelmann spruce (Picea engelmannia), and Sub-alpine fir (Abies laziocarpa). The Campus location included five more species native to the central Rocky Mountains that were planted by the University of Wyoming: Blue Spruce (Picea pungens), White fir (Abies concolor), Ponderosa pine (Pinus ponderosa), Pinyon pine (Pinus edulis), and Rocky Mountain juniper (Juniperus scopulorum). Three individuals of each species were sampled at each location. Individuals were chosen at each site for this study by having similar sunlight exposure, canopy dominance, and diameter at breast height.
Stem Sampling
Small terminal branches were taken from the southern aspect of each individual tree at about 3–5 meters off the ground with a long reach pruner. However, some of the Lodgepole pine samples at the Med Bow location were outside the reach of the long reach pruner and were retrieved using a 12-gauge shotgun. All needles were removed from samples immediately following removal from the tree. Small twigs connected to the main stem of collected branch samples were removed to isolate 15-cm long stem segments. Then phloem and bark were removed from about 5 cm of the proximal end of the stem (Figure 1A). Next, a sub-sample of roughly 1 cm of the woody xylem at the proximal end of the stem was removed and placed in a glass vial to later collect total plant water by CVD (Figure 1B), henceforth referred to as “Stem-CVD” samples. The remaining segment of woody xylem was wrapped in Teflon to facilitate an airtight seal in the Scholander-type pressure chamber gasket for PC extraction (Figure 1B), henceforth referred to as “Stem-PC” samples. We collected and combined water from five consecutively sampled stems for each tree to make Stem-PC composite samples (see below for details on PC water extractions). Stem-CVD samples also were composite samples consisting of trimmings from the same five stems of a tree to permit a direct comparison between CVD and PC extraction methods.
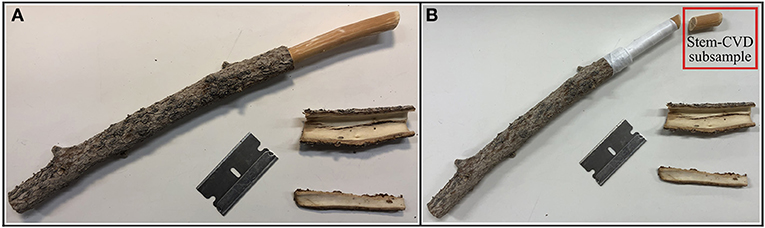
Figure 1. Example of preparation of stem sample immediately after pruning (A) and following the subsampling of roughly 1 cm cut section for cryogenic vacuum distillation (CVD) extraction for Stem-CVD composite subsamples and Teflon wrapping of remaining stem sample before Scholander-type pressure chamber (PC) water extraction for Stem-PC composite samples (B). Figure includes an example stem of Blue Spruce at Campus location for visualization and this particular stem was not actually used for extractions.
Trunk Sampling
To strengthen the comparisons of water extraction methods with previous studies, core samples from the tree trunk of each sampled plant were taken to extract water using CVD. Each individual tree trunk was sampled on the same day that stems were sampled. Cores from tree trunks were sampled with a threaded, 4.3-mm diameter increment borer. Phloem and bark were immediately removed from xylem core tissue with tweezers once each core was removed from borer by the extractor and only 3 cm of the most distal (youngest) xylem was kept and placed in a glass sample vial. Excess (older) xylem was removed from the desired portion with a pair of scissors while holding the 3-cm core portion with tweezers. To integrate variation within a given tree, four core samples were taken from the four cardinal directions on the trunk and placed in a single glass vial to make a composite trunk sample for each tree. Composite samples were parafilmed, transported in a cooler from the field, and stored in a −20°C freezer until water was extracted by CVD for isotopic analysis, henceforth referred to as “Trunk-CVD” samples (see below for details on CVD extractions).
PC Water Extraction
The remaining stem segment wrapped in Teflon (mentioned above in Stem Sampling) was immediately placed into a PMS instruments Model 1000 pressure chamber instrument (PMS Instruments Company, Albany, OR, USA) after subsampling the stem for Stem-CVD composite sample and wrapping in Teflon (Figure 1B). We used the 3/8-inch gasket and insert with the 1/2-inch compression gland base from PMS Instruments Company to accommodate larger diameter stems than normally used for leaf water potential measurements. The chamber was pressurized using nitrogen gas up to 30 bars for pine samples and 35 bars for all other tree samples, at which point sap started to elute from the tip of the stem samples exposed outside the chamber. Sap was collected by dabbing and resting plastic Eppendorf tubes with glass wool on the exposed stem, and the chamber was commonly tilted on its side (Figure 2). Sap was collected for 3 min from each of the five stems that make up the composite Stem-PC samples. This timeframe was chosen to reduce the exposure to the atmosphere and limit the potential for evaporative enrichment.
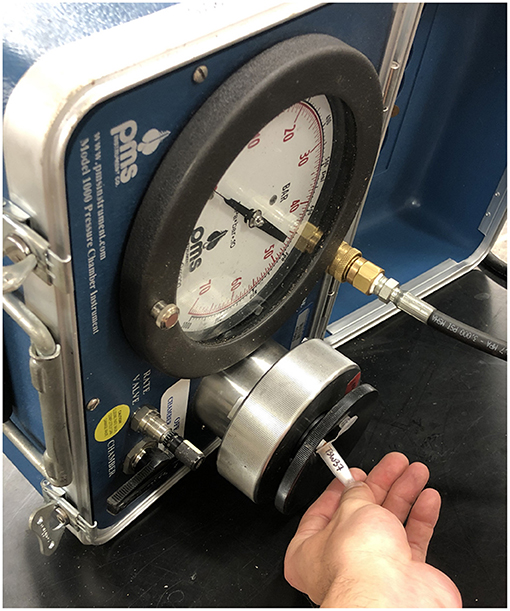
Figure 2. Example of collecting Stem-PC sample into Eppendorf tube filled with glass wool and the PMS instruments Model 1000 pressure chamber instrument on its side so that gravity helps facilitate the collection of sap.
Prior to collection of sap, all Eppendorf tubes were prepared by filling roughly 1 gram of glass wool into each tube and labeling with a sharpie. Tubes were then oven dried at 105°C for 24 h and all vials were placed in a desiccator immediately following removal from the oven. Eppendorf tubes were removed from the desiccator on sampling days and stored in two plastic bags on site and during transportation. Control samples were taken on each sampling day to verify whether evaporative enrichment was altering sample values. To do this we applied 0.75 ml of tap water with known stable isotopic water values to separate control Eppendorf vials containing glass wool and treated as above. After either sap was collected, or control water was applied, all samples were parafilmed and transported in a cooler to the lab where samples were stored at −4°C until water was later extracted from Stem-PC and Control samples using CVD. We opted to also extract water from Stem-PC tubes additionally by CVD rather than mechanical squeezing so that there was a more direct comparison between total plant water and PC sampled water and because it had been done in like manner previously (Ellsworth and Williams, 2007).
CVD Water Extraction
All collected samples (Controls and Stem-PC samples in Eppendorf tubes and Stem-CVD and Trunk-CVD samples in glass vials) underwent water extraction by CVD on the glass water extraction line of the University of Wyoming Stable Isotope Facility. We performed the CVD procedure for 2 h at 102°C and at a starting vacuum pressure of <0.1–2.7 Pa. Temperature and pressure were controlled and monitored using heating coils, thermistors, and vacuum gauges. All samples were weighed prior to and post extraction and then placed in an oven for 24 h and re-weighed to determine extraction efficiency. More than 99% of water was removed from all samples during the CVD procedure.
Stable Isotope Analysis
The stable isotope composition of water is expressed as δ values in units of per mill (‰) and calculated using the isotopic ratios of 2H/1H and 18O/16O for each sample (Rsample) and the international standard (Rstandard) (Equation 1). The international standard Vienna Standard Mean Oceanic Water (VSMOW) was used in Equation 1 and all measurements were corrected to the VSMOW scale using working reference waters calibrated to VSMOW and the Standard Light Antarctic Precipitation (SLAP) reference waters obtained from the International Atomic Energy Agency (IAEA).
The isotopic composition of all water samples was analyzed at the University of Wyoming Stable Isotope Facility using a Delta V isotope ratio mass spectrometer (IRMS) with a temperature conversion/elemental analyzer (TC/EA) interface from Thermo Scientific (Thermo Scientific Corporation, Bremen, Germany). For this study, the measurement precision for the IRMS was 0.95 ‰ for δ2H and 0.31 ‰ for δ18O.
Wood Metrics
Water content was calculated for each Stem-CVD as the weight of water per dry weight of stems (g/g). Weight of water for each Stem-CVD sample was calculated as the difference between sample weight before CVD extraction and after 24 h in the oven post CVD extraction.
Since conifers primarily have ray parenchyma, we used values of ray parenchyma (RP) content of stems for the species in this study that were available in the Global Wood Parenchyma Database (Morris et al., 2016b). Out of the eight species in this study, only four species (i.e., Picea engelmannia, Pinus contorta, Pinus ponderosa, and Abies concolor) had RP data in the Global Wood Parenchyma Database and available data for each species was from Myer (1922).
We used hydraulic vulnerability to cavitation data from Choat et al. (2012) because it was a recent synthesis of data from the literature and had xylem ψ50 values for six of our eight study species (i.e., Pinus contorta, Picea engelmannia, Abies laziocarpa, Abies concolor, Pinus ponderosa, and Pinus edulis). ψ50 is the point along the hydraulic vulnerability to cavitation curve at which the plant has lost fifty percent of its hydraulic conductance capacity. ψ50 was used since it has been shown to integrate other wood metrics that were hard to find for our study species, such as conduit diameter (Pratt and Jacobsen, 2017).
Statistical and Data Analyses
The comparison of Stem-PC to Stem-CVD sample types was facilitated by calculating a Δstem in per mill for both Hydrogen and Oxygen as shown in Equation (2), where δStem−PC and δStem−CVD would both be the δ2H values for Stem-PC and Stem-CVD from the same individual to calculate a Δ2H for that individual tree. Directionality of subtraction was chosen to compare Δstem values from the δ data with water content data similar to Chen et al. (2020). In addition, mean Δstem values for each isotope (2H and 18O) were calculated for each species, location, and overall.
Control samples (see PC water extraction section above) taken on each day of sampling were compared to the original tap water sample by doing similar subtraction as shown in Equation (3), where δtapwater was the known stable water isotopic signature (δ2H = −128 ‰, δ18O = −17.2 ‰) of tap water from the University of Wyoming campus and δcontrol was the respective isotopic ratio value, δ2H or δ18O, of each control (δcontrol). Due to a glass tube breaking during CVD extraction of one control sample, only five of the six control samples were used. Mean Δcontrol values in per mill for each isotope (2H and 18O) were calculated to compare to mean Δstem values.
In addition to using control samples to investigate effects of evaporative enrichment for Stem-PC samples compared to Stem-CVD samples, we calculated a line-conditioned excess (lc-excess) in per mill for each sample to test for evaporative enrichment from the relative distance in dual isotope space away from the local meteoric water line (LMWL) (Landwehr and Coplen, 2006). Lc-excess for each sample was calculated with Equation (4) by using the LMWL for the region reported by Mercer et al. (2020). Linear regressions with isotopic ratio values of plant water (i.e., δ2H ~ δ18O) were performed for each sample type (e.g., Stem-PC, Stem-CVD, Trunk-CVD) to determine presence of significant correlations (p < 0.05) and compare slopes and intercepts to those of the LMWL.
Plant xylem water stable isotope data (δ2H, δ18O, and lc-excess) were tested for normality using quantile-quantile plots, density plots, and the Shapiro-Wilk normality test (Shapiro and Wilk, 1965; Ghasemi and Zahediasl, 2012). Plant xylem water stable isotope data were non-normally distributed for δ2H, δ18O, and lc-excess values. Therefore, three non-parametric Kruskal-Wallis tests [one-way analysis of variance on ranks (ANOVA)] were used to determine if the population of medians for each sample type (i.e., Stem-PC, Stem-CVD, and Trunk-CVD) were statistically similar for δ2H, δ18O, and lc-excess values (Kruskal and Wallis, 1952). Afterwards, a Dunn's test for each Kruskal-Wallis test was performed using the Benjamini-Hochberg adjustment to control false discovery rates and adjust p-values for determining if the sample types represented significantly different pools of water from the plants xylems (p ≤ 0.05) (Dunn, 1964; Benjamini and Hochberg, 1995). There was a bimodal distribution of plant xylem water stable isotope data reflecting the two locations (i.e., Campus and Med Bow), but we could not test the effects of location and/or species with sample-type due to the Kruskal-Wallis test only permitting one independent variable.
The Δ2Hstem and Δ18Ostem values were tested for normality using the same methods applied to plant water isotope data and were found to be normal. Linear regressions were used to determine if there were significant relationships (p ≤ 0.05) between either water content in stems, RP content for species, or ψ50 for species and the Δ2Hstem and Δ18Ostem values per individual tree. Additional linear regressions were used to determine if there was a significant relationship (p ≤ 0.05) between ψ50 for species and the mean Δ2Hstem or Δ18Ostem values per species. Lastly, linear regressions were used to determine if the covariation of H and O isotopes recovered by different methods had a significant relationship.
Data analyses were conducted using the statistical software R v. 4.0.3 (R Core Team, 2020). Data manipulation, summarization, and visualization were performed with the tidyverse package (Wickham et al., 2019). Density plots and quantile-quantile plots were constructed with the ggpubr package (Kassambara, 2020). Shapiro-Wilks normality tests, non-parametric Kruskal-Wallis tests, and Dunn's tests were performed using the rstatix package (Kassambara, 2021). Linear regressions were performed with the stats package (R Core Team, 2020). All code and data is available in online repository (Bowers, 2022).
Results
Table 1 summarizes the descriptive statistics of plant water stable isotope data for each species by sample type. The plant water stable isotope data is presented in dual isotope space in Figure 3 as well as by three boxplots for δ2H, δ18O, and lc-excess separated by sample type and species in Figure 4. Δ2Hstem and Δ18Ostem values for each species are depicted in Figure 5 and summary statistics are detailed in Table 2. By location, Med Bow trees had a mean Δ2Hstem value of −12.6 ‰ and a standard deviation of 3.6 ‰ which was comparable to the mean Δ2Hstem value of −11.9 ‰ and standard deviation of 2.8 ‰ of Campus trees. Med Bow trees had a mean Δ18Ostem value of −1.53 ‰ and standard deviation of 0.71 ‰, and Campus trees had a mean Δ18Ostem value of −1.21 ‰ and standard deviation of 0.65 ‰. These Δ2Hstem and Δ18Ostem values for both sampling locations were greater than the mean and standard deviations of Δcontrol values, which were −1 ± 2.5 ‰ for mean and standard deviation of Δ2Hcontrol and −0.82 ± 0.72 ‰ for mean and standard deviation of Δ18Ocontrol.
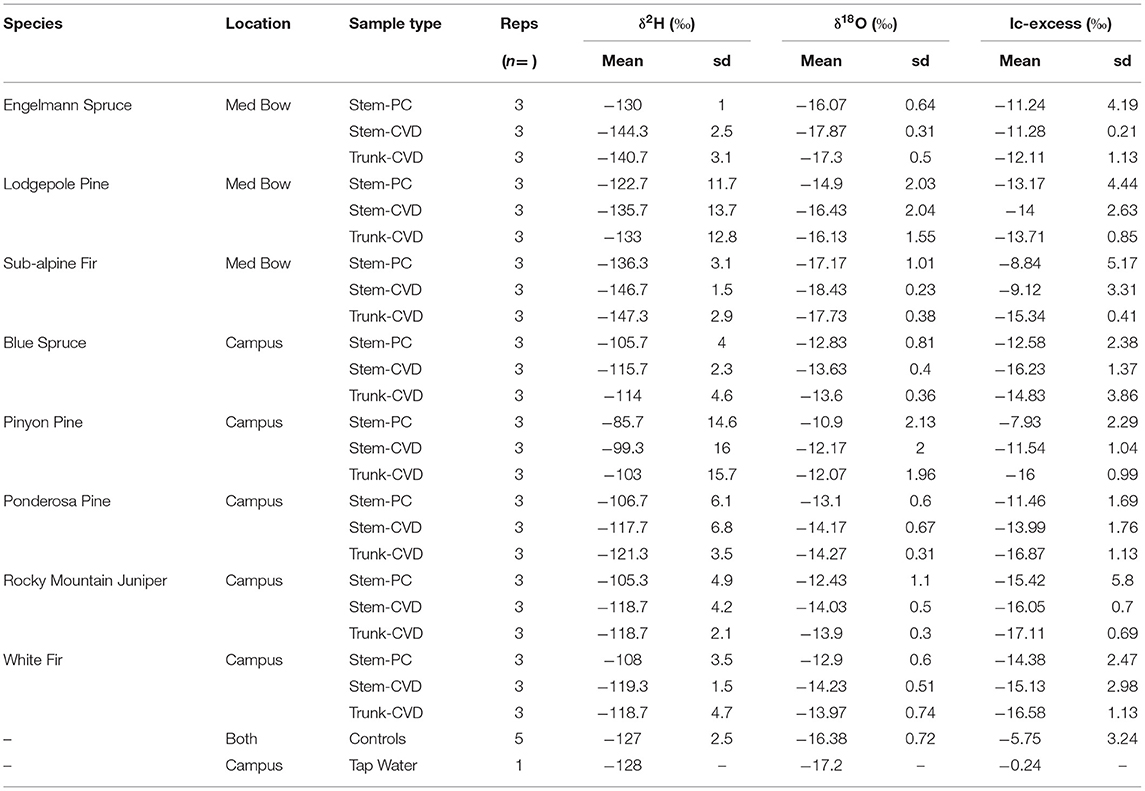
Table 1. Summary table of mean δ2H, δ18O, and lc-excess values and standard deviation (sd) of δ2H, δ18O, and lc-excess values separated by sample type and species.
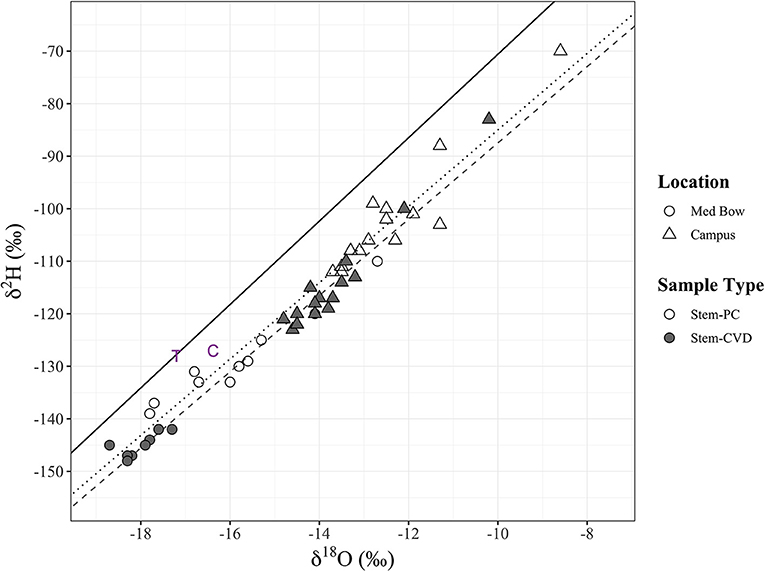
Figure 3. All stem xylem water stable isotope samples (n = 48) in dual isotope space (δ2H and δ18O). Sample type and sampling location are denoted by fill and shape, respectively: Stem-PC (no fill), Stem-CVD (gray fill), Med Bow (circles), and Campus (triangles). Plot includes the LMWL (black solid line) and two additional data points of tap water sample (purple “T”) used for controls and the mean for control samples (purple “C”). Significant positive relationships were found between δ2H and δ18O for each sample type with trend lines included in plot: Stem-PC linear model (dotted black line) and Stem- CVD linear model (dashed gray line) (see end of results section for formulas).
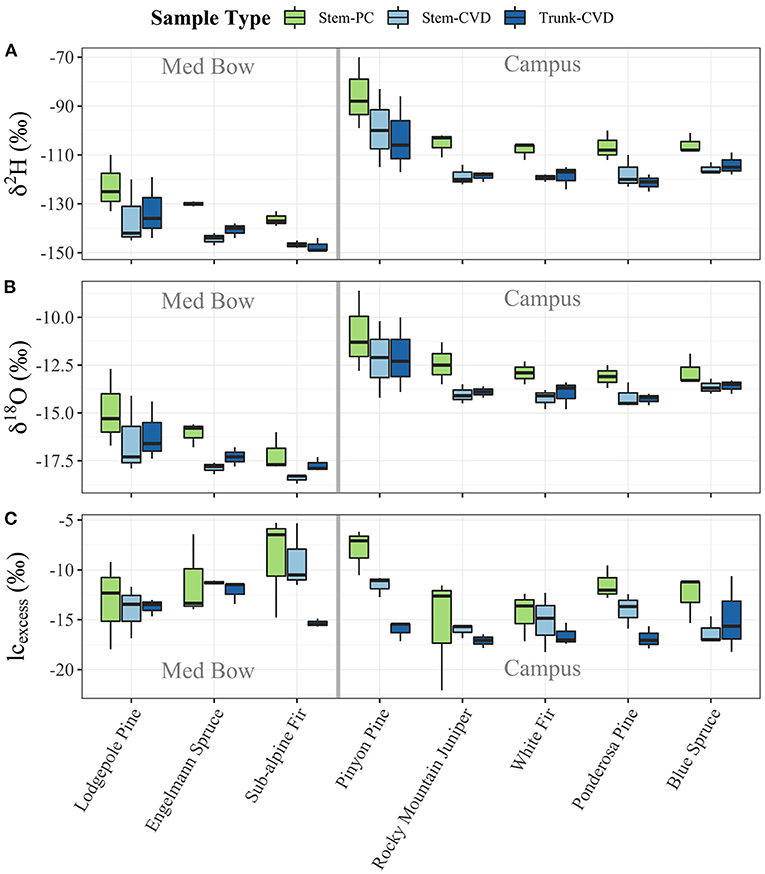
Figure 4. Boxplots of plant water stable isotope data separated by sample type and species: (A) δ2H, (B) δ18O, and (C) lc-excess. Gray bars in center of plots help visually separate samples by location.
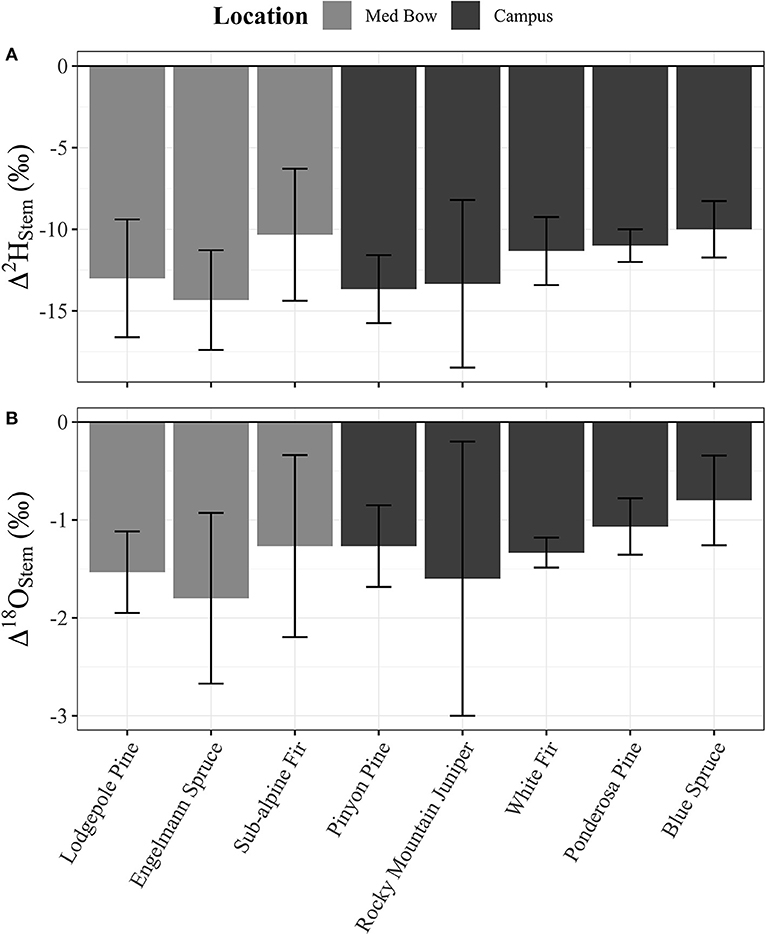
Figure 5. (A) Δ2Hstem and (B) Δ18Ostem column plots for each species with n = 3 per species. Plot panels share the same x-axis and legend.
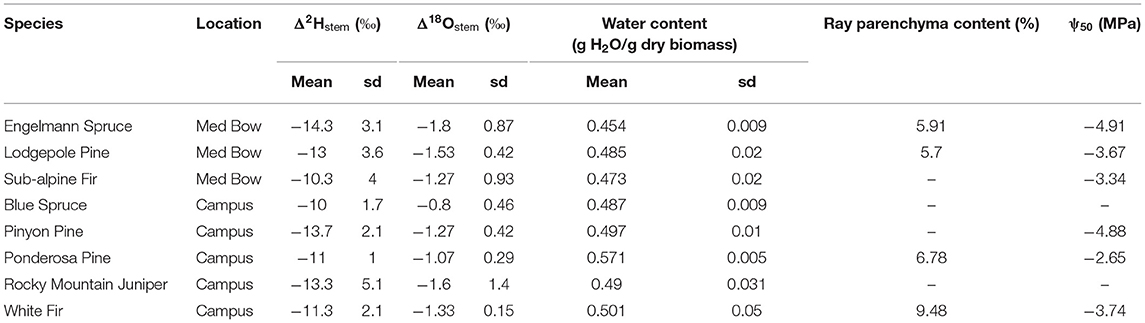
Table 2. Summary statistics of Δ2Hstem, Δ18Ostem, water content values as well as values of ray parenchyma content (RP) and ψ50 from literature for each species (see wood metrics section of methods for literature sources).
ANOVAs of Sample Types
All pairwise comparisons are reported in Table 3. For δ2H data, Stem-PC samples were significantly different from both Stem-CVD samples (p = 0.012) and Trunk-CVD samples (p = 0.012). For δ18O data, Stem-PC samples were significantly different from Stem-CVD samples (p = 0.028), but Stem-PC were not significantly different from Trunk-CVD samples (p = 0.051). A different pattern arose for lc-excess data with Stem-PC and Stem-CVD samples not significantly different from one another (p = 0.18), but both significantly different from Trunk-CVD samples in their respective pairwise comparisons (p = 0.001 and 0.037, respectively). Trunk-CVD samples had the lowest mean value of lc-excess for all sample types and across both locations with a mean and standard deviation of −15.3 ± 2.1 ‰ compared to Stem-PC and Stem-CVD (−11.9 ± 4.0 ‰ and −13.4 ± 3.0 ‰ mean and standard deviation, respectively).
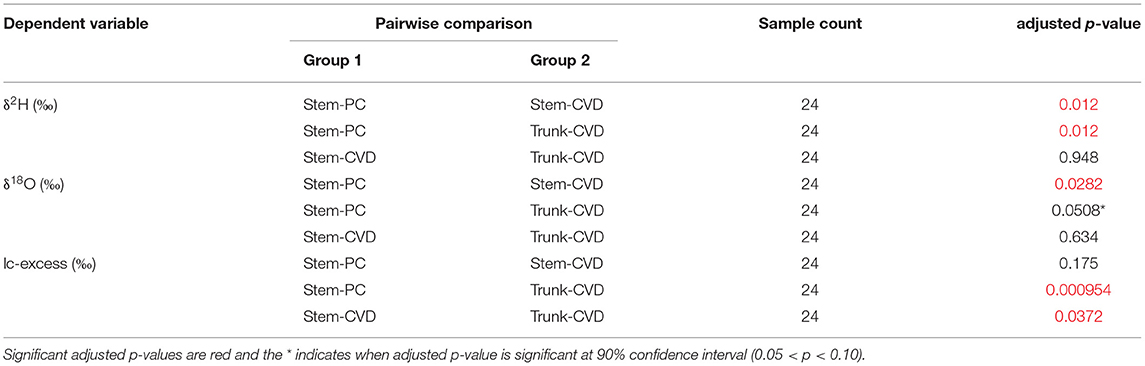
Table 3. Summary table of results from Dunn's test of Kruskal-Wallace ANOVA test with adjusted p-values using the Benjamini-Hochberg adjustment method.
Linear Regressions
Water content of stems had a significant positive relationship with Δ2Hstem values (y = 0.3252x – 28.21, R2 = 0.17, p = 0.048), but there was no significant relationship with Δ18Ostem values (p = 0.092) even though the trend direction was similar (Figure 6). We analyzed the Δstem and ψ50 data in two different ways with either Δstem values from each tree or mean Δstem values for each species. There was not a significant relationship between either Δ2Hstem values (p = 0.056) or Δ18Ostem values (p = 0.25) and ψ50 values for each species. However, there was a significant positive relationship (y = 1.56x – 6.27, R2 = 0.73, p = 0.030) of mean Δ2Hstem for each species (n = 3 individuals/species) and ψ50 values for each species (Figure 7). Mean Δ18Ostem values and ψ50 values did not have a significant relationship (p = 0.16). Finally, there was not a significant relationship between RP and either the Δ2Hstem values (p = 0.28) or Δ18Ostem values (p = 0.47) for each individual tree that had RP species data available. There was a significant positive relationship between Δ2Hstem and Δ18Ostem for each individual tree (y = 3.91x – 6.92, R2 = 0.76, p = <0.001) (Figure 8).
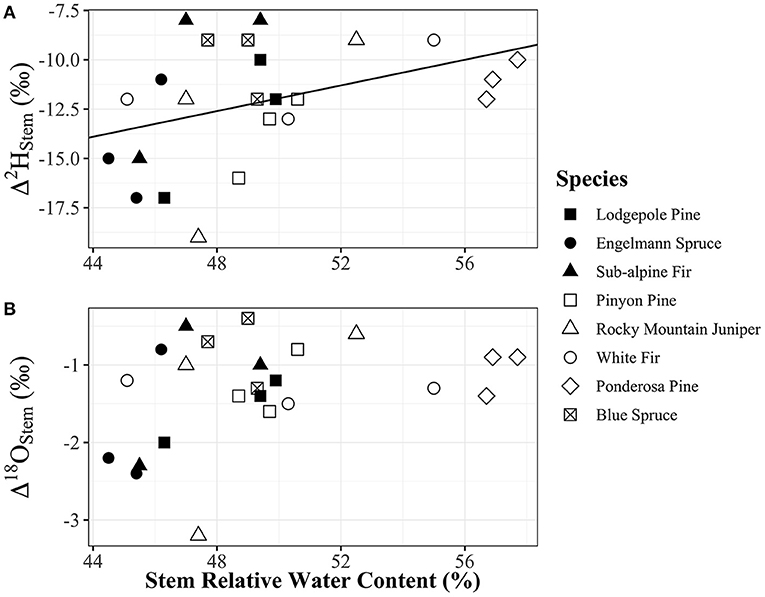
Figure 6. (A) Δ2Hstem and (B) Δ18Ostem vs. relative water content of stems [(g water/g dry mass) × 100]. Relative water content of stems had a significant positive relationship with Δ2Hstem values (y = 0.3252x – 28.21, p = 0.048, R2 = 0.17), but not with Δ18Ostem values (p = 0.092) which also had a similar positive trend in data. Plot panels share the same x-axis and legend. Points shapes are different for each species with filled in point shapes from the Med Bow location and remaining point shapes from the Campus location.
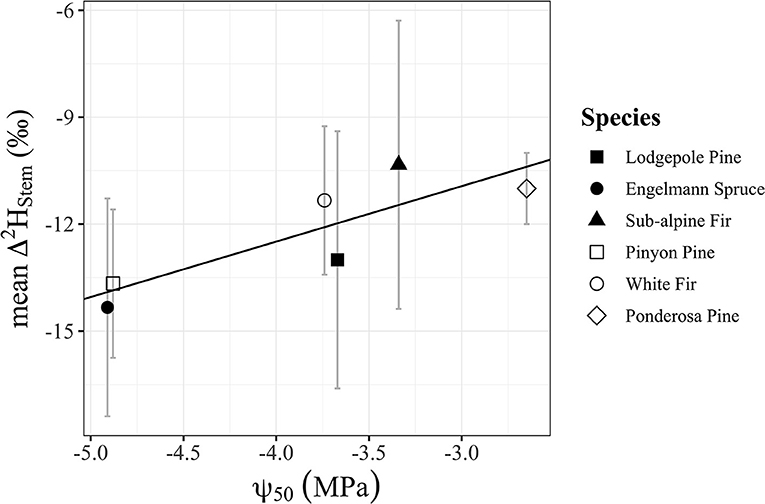
Figure 7. Significant positive relationship (p = 0.030, R2 = 0.73) of mean Δ2Hstem for each species (n = 3 individuals/species) and ψ50 values for each species from Choat et al. (2012). Points shapes are different for each species with filled in point shapes from the Med Bow location and remaining point shapes from the Campus location.
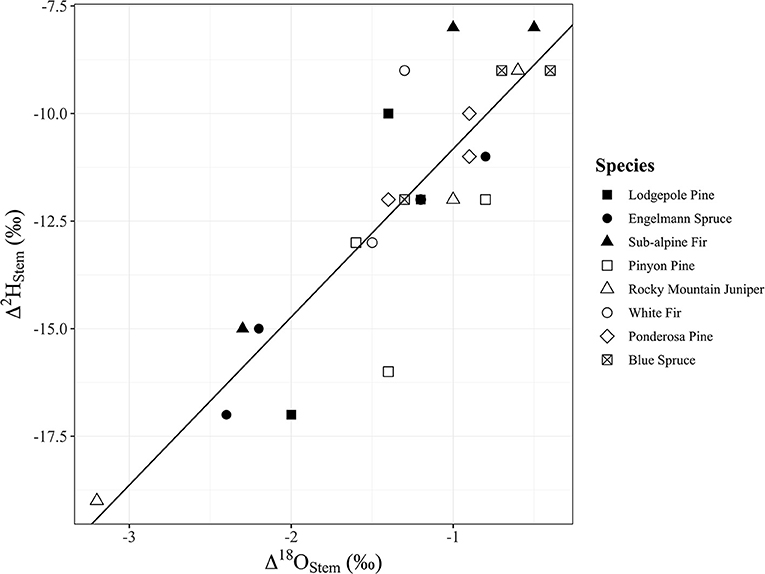
Figure 8. Significant positive relationship between Δ2Hstem and Δ18Ostem for each individual tree (y = 3.91x – 6.92, p = <0.001, R2 = 0.76). Points shapes are different for each species with filled in point shapes from the Med Bow location and remaining point shapes from the Campus location.
Linear regressions between δ2H and δ18O for each sample type all had a significant relationship. δ2H and δ18O for Stem-PC had a linear model of δ2H = 7.27 x δ18O −12.29 (R2 = 0.95, p < 0.00001) (Figure 3). δ2H and δ18O for Stem-CVD had a linear model of δ2H = 7.26 × δ18O −14.87 (R2 = 0.98, p < 0.00001) (Figure 3). δ2H and δ18O for Trunk-CVD had a linear model of δ2H = 7.47 × δ18O −13.48 (R2 = 0.98, p < 0.00001).
Discussion
Our study with conifers native to the Central Rocky Mountains of North America demonstrates that total plant xylem water can be incompletely mixed isotopically. Additionally, the different pools of water recovered from the same stems of trees by the two extraction methods in our study (e.g., PC and CVD) had significantly different isotopic ratio values based on our ANOVA results (Table 3; Figure 4). These differences in the isotopic value of stem water extracted using CVD and PC methods were significantly related to species-specific wood metrics; Δ2Hstem as a function of water content of stems (p = 0.048, R2 = 0.17) and species mean Δ2Hstem as a function of species ψ50 values from the literature (p = 0.030, R2 = 0.73). Furthermore, we are confident that our observations were not influenced by potential methodological artifacts since the control samples used to verify the method demonstrated minimal difference to the known reference water used (Δcontrol values) while also being smaller than the difference in isotopic ratio values between extraction methods (Δstem values).
Previous comparisons of the PC and CVD xylem water extraction methods for analysis of stable isotope values has been limited, but the majority of published work found patterns comparable to ours suggesting isotopically distinct plant water pools are accessed by the two methods (Ellsworth and Williams, 2007; Zuecco et al., 2020). Conversely, Geißler et al. (2019) did not find a significant difference between six stem water samples of Acacia mellifera shrubs extracted by the PC and CVD methods, but they only compared δ18O data between extraction methods. Furthermore, while the PC method employed in the Geißler et al. (2019) study was similar to our protocol, a description of the plant tissues used for the CVD extraction was not provided, which has been shown to be an important criteria for comparisons (Zhao et al., 2016; Zuecco et al., 2020). Our study was unique from past studies in that we had a direct comparison for both extraction methods of xylem tissue from the same stems of individual trees in composite samples. We are confident that our samples had minimal contributions of co-extracted organic compounds because our samples were visibly clear. The presence of organic compounds is particularly of interest when analyzing water samples with laser-based spectroscopy methods as organic compounds have been shown to affect isotopic values (Brian Leen et al., 2012; Millar et al., 2018), but we analyzed all our samples with IRMS to reduce the possibility of these effects. Interestingly, Barbeta et al. (2022) recently highlighted that similar results can be produced from both laser-based spectroscopy and IRMS methods when analyzing differences between bulk stem water and sap water. With respect to our data, we are confident that Stem-PC samples had minimal contributions from phloem water because the phloem was removed near the collection surface during PC extractions and the samples were visibly clear with the lowest average lc-excess values of all sample types for each species (Table 1). Together with our low average Δcontrol values and the slope of linear model of Stem-PC samples being similar to the LMWL, these low lc-excess values support that our Stem-PC samples were influenced minimally by evaporative enrichment during extractions and transport from field.
In addition to tissue type sampled for CVD, the other nuances of source water and internal plant storage may provide some insight to the differences between our results and those of Geißler et al. (2019). The Geißler et al. (2019) study species was a shrub and their site received precipitation primarily as rain which likely has a smaller range in isotopic values throughout the year compared to the range in isotopic values of snow and rain at our study sites. Therefore, compared to our study sites and species, Geißler et al. (2019) may have had a reduced range of isotopic values of their root water sources and a smaller reservoir of internal storage which collectively affected the observed differences in isotopic value of water recovered by PC and CVD. We did not take precipitation samples in this study, but our Stem-PC isotopic values more closely resembled summer rainfall for the region whereas the Stem-CVD and Trunk-CVD isotopic values resembled a mixture of recent rainwater and of snowmelt for the region (Mercer et al., 2020; Miller et al., 2021). This suggests that the trees in our study may be filling internal storage reservoirs within their complex woody tissue when soil conditions are favorable after snowmelt. Then, some of this storage later contributes to transpiration and subsequently is refilled depending on future climate and soil moisture conditions. A fluctuating tree water storage and variable contribution of tree water storage to transpiration has been documented to be species-specific with Red Maple and Red Oak (Matheny et al., 2015). Aside from some of the differences with a previous study that used the PC method, our observations are consistent with previous work showing that total plant xylem water recovered using CVD had more depleted δ2H values than that of transpiration-stream xylem water (Chen et al., 2020), but as highlighted above the range in source water could be important to consider in this context as well as the size of the tree in regards to internal storage.
Other work has highlighted differences in δ2H of xylem water in the context of fractionation during root water uptake (Lin and da SL Sternberg, 1993; Ellsworth and Williams, 2007; Zhao et al., 2016; Barbeta et al., 2019), during plant water storage via organic hydrogen exchange (Millar et al., 2018), during extraction by CVD (Chen et al., 2020), and from aquaporin- or surface-mediated isotopic heterogeneity within complex woody tissue (Zhao et al., 2016; Barbeta et al., 2020, 2022). In regards to fractionation during root water uptake, it is hard to directly compare our study results since our study design focused on possible heterogeneity within the xylem of multiple plants (i.e., transpiration-stream water compared to bulk xylem water) and source waters were not sampled. Whereas, most studies focusing on possible root water fractionation included bulk stem water compared to a source water. Therefore, if we assume our Stem-PC samples are representative of source water, then we can cautiously consider the differences observed in root water uptake fractionation studies and how they relate to our Δstem values. With this is mind, significant differences in δ2H of bulk xylem water extracted via CVD compared to source water have been repeatedly documented and often associated with salt and drought tolerant species (Lin and da SL Sternberg, 1993; Ellsworth and Williams, 2007; Zhao et al., 2016; Poca et al., 2019). However, while many of these studies found differences in δ18O of bulk xylem water extracted via CVD compared to source water, most of the time these differences were not significant (Lin and da SL Sternberg, 1993; Zhao et al., 2016). Ellsworth and Williams (2007) found a significant difference in δ18O of bulk xylem stem water and source water for P. velutina plants, but these differences were attributed to evaporation from the stem of immature saplings with incompletely suberized stems. Poca et al. (2019) only found significant differences in δ18O for their arbuscular mycorrhizal treatment plants. Barbeta et al. (2019) found that xylem δ18O could be explained by a mixture of source water isotopic signatures but xylem δ2H was more depleted than any possible source. However, they did find an interesting relationship where more dominant trees had more depleted xylem water than non-dominant trees for both δ18O and δ2H (Barbeta et al., 2019). Out of the many tissue types sampled, Zhao et al. (2016) found that only core samples near the ground had significantly different δ18O values compared to the primary source of groundwater. Barbeta et al. (2020) found offsets between soil and plant water for both isotopes but their Δ18O were not always significant even though they were proportional to Δ2H. Furthermore, their results supported that the differences were not from fractionation at the root-soil interface, but rather from either isotopic heterogeneities in soil pores or within complex woody tissue (Barbeta et al., 2020). This explanation has been supported further by a similar study that included sap water extracted with a novel cavitron method (Barbeta et al., 2022).
In regards to exchangeable organic hydrogens or potential CVD artifacts, Millar et al. (2018) found that both CVD versions, one following a low temperature protocol (Orlowski et al., 2013) and another following a high temperature protocol (Koeniger et al., 2011), recovered water that was depleted in 2H relative to the other extraction methods. Millar et al. (2018) concluded that this was likely because the bulk water accessed by CVD contains a small proportion of water that had exchanged hydrogens with plant organic compounds before extraction. However, more recent work by Chen et al. (2020) presented this hydrogen exchange as a unique effect imparted on the plant water during the CVD extraction because the δ2H changes during the evaporative extraction process unlike other non-evaporative extraction methods, effectively changing the exchange rate of hydrogens during extraction. The effect of the fractionation is also proposed to be increasingly buffered with increasing stem water content prior to extraction. Chen et al. (2020) supported this hypothesis with a steady-state transpiration chamber experiment and subsequent rehydration experiment of stem samples where their δ2H offset values (similar to our Δ2Hstem values) for each experiment had a significant positive relationship with stem water content. While our Δ2Hstem values have a similar positive relationship with stem water content (Figure 6), our results differ from theirs in that we found more pronounced differences with δ18O values (i.e., Δ18Ostem values) between extraction methods and a similar trend in Δ18Ostem values and stem water content. Recent work by Barbeta et al. (2022) presented a similar positive relationship with their Δ2H offset values and stem relative water content and like ours, it was greater in magnitude compared to Chen et al. (2020), but also greater in magnitude compared to ours (slopes: 0.144 vs. 0.325 vs. 0.745). However, our relationship explained the least amount of data (R2 = 0.17) compared to previous relationships that had higher R2 values. Additionally, we found a significant positive relationship between Δ2Hstem and Δ18Ostem values among all trees sampled (Figure 8), demonstrating a covariation of H and O isotopes between extraction methods which would not be predicted from H exchange with organic compounds during CVD. Therefore, our greater slope and covariation of H and O isotopes compared to previous work suggests there could be other or additional mechanisms affecting the Δstem values than the proposed CVD artifacts (Barbeta et al., 2022), such as incomplete mixing of total plant xylem water, since both isotopes presented significant differences. An additional rehydration experiment similar to previous work would help further confirm that additional mechanisms to the proposed CVD artifacts may be affecting the Δ2Hstem values (Barbeta et al., 2022) and we encourage future studies using the PC method to implement such an experiment. Granted, we cannot confirm that the water recovered by the PC method in our study is exclusively representative of transpiration-stream water, more specifically the water under suction by the primary conduit flow network on its way from roots to leaves prior to sampling (Venturas et al., 2017). Previous work comparing isotopic composition of transpiration-stream water to bulk xylem water has included an independent verification with a controlled experiment and known source waters (Chen et al., 2020; Barbeta et al., 2022). Our goal was primarily to assess presence of isotopic heterogeneity in mature trees and whether it related to xylem vulnerability and anatomical properties. Moving forward it will be necessary to conduct a controlled experiment with the PC method to confirm its ability to recover primarily transpiration-stream water. The future controlled experiment could consider including a paired use of a steady-state chamber like that of Chen et al. (2020) and Stem-PC samples like in our study. Another option could include using cryogenic scanning electron microscopy as well as X-ray computed microtomography imaging before and after using the PC method (Yazaki et al., 2020). Each of these options could improve our understanding of the mechanisms at play as well as potentially validate the more cost effective and field-site friendly PC method.
Correlations With Wood Metrics
We demonstrate that the differences in isotopic ratio values between extraction methods (Δstem values) are related to species-specific wood anatomical metrics that support previous hypotheses of variable residence time and incomplete mixing of water in complex woody tissue (Knighton et al., 2020). The significant positive relationship (p = 0.030, R2 = 0.73) of mean Δ2Hstem for each species (n = 3 individuals/species) and ψ50 values for each species (Figure 7) is particularly notable in this context. The species less vulnerable to cavitation (lower ψ50) may possess incompletely mixed xylem conduit flow networks compared to their more vulnerable counterparts. We propose that this is because the taxa that have lower ψ50 may be able to maintain adequate hydraulic function with some cavitated conduits creating xylem regions with poor connectivity to downstream or neighboring tracheary elements in the transpiration-stream. The significant positive relationship between stem water content and Δ2Hstem values (Figure 6) could be simply related to the amount of exchangeable hydrogens which happens to support both the proposed isotopic heterogeneity of xylem water and the proposed CVD artifacts that are currently hard to separate from one another as mentioned by recent work (Barbeta et al., 2022). Alternatively, we hypothesize that xylem anatomical properties (i.e., xylem density, conduit density, mean and/or range of conduit diameters, pit membrane density) are playing a role in this relationship that we are not able to disentangle with our dataset.
While our dataset was limited, the role of parenchyma content in affecting presence of multiple plant water pools with distinct isotopic ratio values cannot be fully ruled out since parenchyma content in gymnosperms is a much lower fraction than in angiosperms (Morris et al., 2016b; Pratt and Jacobsen, 2017). Recent work has supported this with two angiosperms species having significantly greater Δ2H compared to a gymnosperm species (Barbeta et al., 2022). Future work should consider using more direct measurements of ray and axial parenchyma content, conduit diameters, inter-conduit pit density as well as xylem vulnerability to cavitation of sampled trees for direct comparisons and evaluations. We acknowledge that ψ50 is an arbitrary parameter; there are many efforts to examine the functional significance of other points along the vulnerability curve (i.e., ψ88) as well as the slope of the curve and the methods used to produce a curve (Venturas et al., 2017). All of these xylem anatomical and physiological properties could be important as we seek to understand the mechanism(s) causing differences in isotopic composition of transpiration-stream xylem water and bulk xylem water. Once the mechanism(s) causing differences become(s) more fully understood, then stable isotopes as a tool could inform our overall understanding of the functional and structural tradeoffs of xylem anatomy.
Isotopic Heterogeneity of Xylem Water
As mentioned previously, our data suggest that within-stem isotopic heterogeneity (as proposed by Zhao et al., 2016; Barbeta et al., 2019) is responsible, at least in part if not fully, for the significant Δ2Hstem and Δ18Ostem values observed, but CVD artifacts (as proposed by Chen et al., 2020) cannot be excluded as contributing to the observed Δ2Hstem offsets. Thus far, isotopic heterogeneity of xylem water has focused on either incomplete mixing and variable residence times of xylem water or fractionation during transportation and/or storage of water within complex woody tissue compartments, including the water associated with cell walls and fibers (Zhao et al., 2016; Barbeta et al., 2019, 2020, 2022). Regarding incomplete mixing and variable residence times of xylem water, discussions have mainly centered on poor connectivity between capillary xylem water in dead conducting cells and living symplastic cells. However, since our offsets were correlated with ψ50 and a covariation of both isotopes was observed, we propose that there could also be incomplete mixing and variable residence times within dead conduit cells in the context of the isotopic heterogeneity hypothesis. This includes water in the tapered end of cavitated conduits (Yazaki et al., 2020) and we propose it also includes water in smaller conduits that may be inaccessible by the PC method. In fact, when attempting to use the PC method on Quercus macrocarpa specimens on Campus, we had limited success in retrieving water beyond the largest vessels because once evacuated the large vessels became the path of least resistance for the pressurized gas which effectively reduced the applied pressure to remaining filled conduits (data not shown). The gymnosperms used in this study did not present this observable issue during extraction via PC most likely due to their lack of vessels. Recent work has also shown that not all tracheary elements capable of conducting water in an excised stem participate in conducting water in vivo (Bouda et al., 2019). Therefore, differences observed in both isotopes could reflect different timing of replenishment or turnover between conduits. It is worth noting here that Barbeta et al. (2022) found significant differences between δ18O and δ2H of sap water and bulk xylem water for their field samples that would support this proposition. Our results suggest that studies aiming to identify the isotopic signature of transpiration-stream water need to consider the presence of multiple plant water domains with variable residence times in complex woody tissue that can be impacted by species-specific wood metrics and site-specific seasonal variability in the isotopic composition of precipitation.
Data Availability Statement
The datasets presented in this study can be found in online repositories. The names of the repository/repositories and accession number(s) can be found below: https://doi.org/10.17605/OSF.IO/VCZR3.
Author Contributions
WB and DW contributed to developing the methods, study design, and manuscript preparation. WB primarily preformed the field and laboratory work with the help of a lab technician and other assistance and preformed the data analysis. Both authors contributed to the article and approved the submitted version.
Funding
Support for this work was provided the Wyoming EPSCoR Microbial Ecology Collaborative, with funding from NSF grant number #EPS-1655726 and by a grant to DW from the Faculty Grant-in-Aid program at the University of Wyoming.
Conflict of Interest
The authors declare that the research was conducted in the absence of any commercial or financial relationships that could be construed as a potential conflict of interest.
Publisher's Note
All claims expressed in this article are solely those of the authors and do not necessarily represent those of their affiliated organizations, or those of the publisher, the editors and the reviewers. Any product that may be evaluated in this article, or claim that may be made by its manufacturer, is not guaranteed or endorsed by the publisher.
Acknowledgments
We would like to thank Matthew Tetrick, Erin Bowers, and Rob Bowers for their help with field work. In addition, we would like to thank the Stable Isotope Facility (SIF) of the University of Wyoming for providing a CVD water extraction line and analyzing our stable isotope data.
References
Barbeta, A., Burlett, R., Martín-Gómez, P., Fréjaville, B., Devert, N., Wingate, L., et al. (2022). Evidence for distinct isotopic compositions of sap and tissue water in tree stems: consequences for plant water source identification. New Phytol. 233, 1121–1132. doi: 10.1111/nph.17857
Barbeta, A., Gimeno, T. E., Clavé, L., Fréjaville, B., Jones, S. P., Delvigne, C., et al. (2020). An explanation for the isotopic offset between soil and stem water in a temperate tree species. New Phytol. 227, 766–779. doi: 10.1111/nph.16564
Barbeta, A., Jones, S. P., Clavé, L., Wingate, L., Gimeno, T. E., Fréjaville, B., et al. (2019). Unexplained hydrogen isotope offsets complicate the identification and quantification of tree water sources in a riparian forest. Hydrol. Earth Syst. Sci. 23, 129–2146. doi: 10.5194/hess-23-2129-2019
Benjamini, Y., and Hochberg, Y. (1995). Controlling the false discovery rate: a practical and powerful approach to multiple testing. J. R. Stat. Soc. Ser. B 57, 289–300. doi: 10.1111/j.2517-6161.1995.tb02031.x
Bouda, M., Windt, C. W., McElrone, A. J., and Brodersen, C. R. (2019). In vivo pressure gradient heterogeneity increases flow contribution of small diameter vessels in grapevine. Nat. Commun. 10, 5645. doi: 10.1038/s41467-019-13673-6
Bowen, G. J., Cai, Z., Fiorella, R. P., and Putman, A. L. (2019). Isotopes in the water cycle: regional- to global-scale patterns and applications. Annu. Rev. Earth Planet. Sci. 47, 453–479. doi: 10.1146/annurev-earth-053018-060220
Bowers, W. H.. (2022). Isotopic heterogeneity of stem water in conifers is correlated to xylem hydraulic traits and supports multiple residence times [data files and R code]. Open Sci. Framework. doi: 10.17605/OSF.IO/VCZR3
Bowers, W. H., Mercer, J. J., Pleasants, M. S., and Williams, D. G. (2020). A combination of soil water extraction methods quantifies the isotopic mixing of waters held at separate tensions in soil. Hydrol. Earth Syst. Sci. 24, 4045–4060. doi: 10.5194/hess-24-4045-2020
Brian Leen, J., Berman, E. S. F., Liebson, L., and Gupta, M. (2012). Spectral contaminant identifier for off-axis integrated cavity output spectroscopy measurements of liquid water isotopes. Rev. Sci. Instrum. 83, 044305. doi: 10.1063/1.4704843
Carrière, S. D., Martin-StPaul, N. K., Cakpo, C. B., Patris, N., Gillon, M., Chalikakis, K., et al. (2020). The role of deep vadose zone water in tree transpiration during drought periods in karst settings – Insights from isotopic tracing and leaf water potential. Sci. Total Environ. 699, 134332. doi: 10.1016/j.scitotenv.2019.134332
Cermak, J., Kucera, J., Bauerle, W. L., Phillips, N., and Hinckley, T. M. (2007). Tree water storage and its diurnal dynamics related to sap flow and changes in stem volume in old-growth Douglas-fir trees. Tree Physiol. 27, 181–198. doi: 10.1093/treephys/27.2.181
Chen, G., Auerswald, K., and Schnyder, H. (2016). 2H and 18O depletion of water close to organic surfaces. Biogeosciences 13, 3175–3186. doi: 10.5194/bg-13-3175-2016
Chen, Y., Helliker, B. R., Tang, X., Li, F., Zhou, Y., and Song, X. (2020). Stem water cryogenic extraction biases estimation in deuterium isotope composition of plant source water. Proc. Natl. Acad. Sci. U. S. A. 117, 33345–33350. doi: 10.1073/pnas.2014422117
Choat, B., Jansen, S., Brodribb, T. J., Cochard, H., Delzon, S., Bhaskar, R., et al. (2012). Global convergence in the vulnerability of forests to drought. Nature 491, 752–755. doi: 10.1038/nature11688
Dunn, O. J.. (1964). Multiple comparisons using rank sums. Technometrics 6, 241. doi: 10.1080/00401706.1964.10490181
Ehleringer, J. R., and Dawson, T. E. (1992). Water uptake by plants: perspectives from stable isotope composition. Plant. Cell Environ. 15, 1073–1082. doi: 10.1111/j.1365-3040.1992.tb01657.x
Ellsworth, P. Z., and Williams, D. G. (2007). Hydrogen isotope fractionation during water uptake by woody xerophytes. Plant Soil 291, 93–107. doi: 10.1007/s11104-006-9177-1
Fischer, B. M. C., Frentress, J., Manzoni, S., Cousins, S. A. O., Hugelius, G., Greger, M., et al. (2019). Mojito, anyone? An exploration of low-tech plant water extraction methods for isotopic analysis using locally-sourced materials. Front. Earth Sci. 7, 1–11. doi: 10.3389/feart.2019.00150
Gaj, M., Kaufhold, S., Koeniger, P., Beyer, M., Weiler, M., and Himmelsbach, T. (2017). Mineral mediated isotope fractionation of soil water. Rapid Commun. Mass Spectrom. 31, 269–280. doi: 10.1002/rcm.7787
Gaj, M., Lamparter, A., Woche, S. K., Bachmann, J., McDonnell, J. J., and Stange, C. F. (2019). The role of matric potential, solid interfacial chemistry, and wettability on isotopic equilibrium fractionation. Vadose Zone J. 18, 1–11. doi: 10.2136/vzj2018.04.0083
Gaj, M., and McDonnell, J. J. (2019). Possible soil tension controls on the isotopic equilibrium fractionation factor for evaporation from soil. Hydrol. Process. 33, 1629–1634. doi: 10.1002/hyp.13418
Geißler, K., Heblack, J., Uugulu, S., Wanke, H., and Blaum, N. (2019). Partitioning of water between differently sized shrubs and potential groundwater recharge in a Semiarid Savanna in Namibia. Front. Plant Sci. 10, 1–13. doi: 10.3389/fpls.2019.01411
Ghasemi, A., and Zahediasl, S. (2012). Normality tests for statistical analysis: a guide for non-statisticians. Int. J. Endocrinol. Metab. 10, 486–489. doi: 10.5812/ijem.3505
Hacke, U. G., Spicer, R., Schreiber, S. G., and Plavcová, L. (2017). An ecophysiological and developmental perspective on variation in vessel diameter. Plant. Cell Environ. 40, 831–845. doi: 10.1111/pce.12777
IPCC (2014). Climate Change 2014: Synthesis Report. Contribution of Working Groups I, II and III to the Fifth Assessment Report of the Intergovernmental Panel on Climate Change, eds Core Writing Team R. K. Pachauri, and L. A. Meyer (Geneva: IPCC), 151.
Kassambara, A.. (2020). ggpubr: ‘ggplot2' Based Publication Ready Plots. R Package Version 0.4.0. Available online at: https://CRAN.Rproject.org/package=ggpubr
Kassambara, A.. (2021). rstatix: Pipe-Friendly Framework for Basic Statistical Tests. R package version 0.7.0. Available online at: https://CRAN.Rproject.org/package=rstatix
Knighton, J., Kuppel, S., Smith, A., Soulsby, C., Sprenger, M., and Tetzlaff, D. (2020). Using isotopes to incorporate tree water storage and mixing dynamics into a distributed ecohydrologic modelling framework. Ecohydrology 13, e2201. doi: 10.1002/eco.2201
Koeniger, P., Marshall, J. D., Link, T., and Mulch, A. (2011). An inexpensive, fast, and reliable method for vacuum extraction of soil and plant water for stable isotope analyses by mass spectrometry. Rapid Commun. Mass Spectrom. 25, 3041–3048. doi: 10.1002/rcm.5198
Kruskal, W. H., and Wallis, W. A. (1952). Use of ranks in one-criterion variance analysis. J. Am. Stat. Assoc. 47, 583–621. doi: 10.1080/01621459.1952.10483441
Landwehr, J., and Coplen, T. B. (2006). Line-Conditioned Excess: A New Method for Characterizing Stable Hydrogen and Oxygen Isotope Ratios in Hydrologic Systems. International Atomic Energy Agency.
Lin, G., and da SL Sternberg, L. (1993). “Hydrogen isotopic fractionation by plant roots during water uptake in coastal wetland plants,” in Stable Isotopes and Plant Carbon-water Relations, eds J. R. Ehleringer, A. E. Hall, and G.D. Farguhar (San Diego, CA: Academic Press Inc.), 497–510.
Mastrotheodoros, T., Pappas, C., Molnar, P., Burlando, P., Manoli, G., Parajka, J., et al. (2020). More green and less blue water in the Alps during warmer summers. Nat. Clim. Change 10, 155–161. doi: 10.1038/s41558-019-0676-5
Matheny, A. M., Bohrer, G., Garrity, S. R., Morin, T. H., Howard, C. J., and Vogel, C. S. (2015). Observations of stem water storage in trees of opposing hydraulic strategies. Ecosphere 6, art165. doi: 10.1890/ES15-00170.1
Meißner, M., Köhler, M., Schwendenmann, L., Hölscher, D., and Dyckmans, J. (2014). Soil water uptake by trees using water stable isotopes (δ2H and δ18O)–a method test regarding soil moisture, texture and carbonate. Plant Soil 376, 327–335. doi: 10.1007/s11104-013-1970-z
Mercer, J. J., Liefert, D. T., and Williams, D. G. (2020). Atmospheric vapour and precipitation are not in isotopic equilibrium in a continental mountain environment. Hydrol. Process. 34, 3078–3101. doi: 10.1002/hyp.13775
Millar, C., Pratt, D., Schneider, D. J., and McDonnell, J. J. (2018). A comparison of extraction systems for plant water stable isotope analysis. Rapid Commun. Mass Spectrom. 32, 1031–1044. doi: 10.1002/rcm.8136
Miller, S. A., Mercer, J. J., Lyon, S. W., Williams, D. G., and Miller, S. N. (2021). Stable isotopes of water and specific conductance reveal complimentary information on streamflow generation in snowmelt-dominated, seasonally arid watersheds. J. Hydrol. 596, 126075. doi: 10.1016/j.jhydrol.2021.126075
Morris, H., Brodersen, C., Schwarze, F. W. M. R., and Jansen, S. (2016a). The parenchyma of secondary xylem and its critical role in tree defense against fungal decay in relation to the CODIT model. Front. Plant Sci. 7, 1–18. doi: 10.3389/fpls.2016.01665
Morris, H., Plavcová, L., Cvecko, P., Fichtler, E., Gillingham, M. A. F., Martínez-Cabrera, H. I., et al. (2016b). A global analysis of parenchyma tissue fractions in secondary xylem of seed plants. New Phytol. 209, 1553–1565. doi: 10.1111/nph.13737
Mrad, A., Domec, J., Huang, C., Lens, F., and Katul, G. (2018). A network model links wood anatomy to xylem tissue hydraulic behaviour and vulnerability to cavitation. Plant. Cell Environ. 41, 2718–2730. doi: 10.1111/pce.13415
Myer, J. E.. (1922). Ray volumes of the commercial woods of the United States and their significance. J. Forest. 20, 337–351.
Newberry, S. L., Nelson, D. B., and Kahmen, A. (2017). Cryogenic vacuum artifacts do not affect plant water-uptake studies using stable isotope analysis. Ecohydrology 10, 1–10. doi: 10.1002/eco.1892
Oerter, E., Finstad, K., Schaefer, J., Goldsmith, G. R., Dawson, T., and Amundson, R. (2014). Oxygen isotope fractionation effects in soil water via interaction with cations (Mg, Ca, K, Na) adsorbed to phyllosilicate clay minerals. J. Hydrol. 515, 1–9. doi: 10.1016/j.jhydrol.2014.04.029
Orlowski, N., and Breuer, L. (2020). Sampling soil water along the pF curve for δ2H and δ18O analysis. Hydrol. Process. 34, 4959–4972. doi: 10.1002/hyp.13916
Orlowski, N., Breuer, L., Angeli, N., Boeckx, P., Brumbt, C., Cook, C., et al. (2018). Inter-laboratory comparison of cryogenic water extraction systems for stable isotope analysis of soil water. Hydrol. Earth Syst. Sci. Discuss. 22, 3619–3637. doi: 10.5194/hess-22-3619-2018
Orlowski, N., Breuer, L., and Mcdonnell, J. J. (2016a). Critical issues with cryogenic extraction of soil water for stable isotope analysis. Ecohydrology 9, 3–10. doi: 10.1002/eco.1722
Orlowski, N., Frede, H.-G., Brüggemann, N., and Breuer, L. (2013). Validation and application of a cryogenic vacuum extraction system for soil and plant water extraction for isotope analysis. J. Sensors Sens. Syst. 2, 179–193. doi: 10.5194/jsss-2-179-2013
Orlowski, N., Pratt, D. L., and McDonnell, J. J. (2016b). Intercomparison of soil pore water extraction methods for stable isotope analysis. Hydrol. Process. 30, 3434–3449. doi: 10.1002/hyp.10870
Penna, D., Oliviero, O., Assendelft, R., Zuecco, G., van Meerveld, I. H., Anfodillo, T., et al. (2013). Tracing the water sources of trees and streams: isotopic analysis in a small pre-alpine catchment. Proc. Environ. Sci. 19, 106–112. doi: 10.1016/j.proenv.2013.06.012
Poca, M., Coomans, O., Urcelay, C., Zeballos, S. R., Bod,é, S., and Boeckx, P. (2019). Isotope fractionation during root water uptake by Acacia caven is enhanced by arbuscular mycorrhizas. Plant Soil 441, 485–497. doi: 10.1007/s11104-019-04139-1
Pratt, R. B., and Jacobsen, A. L. (2017). Conflicting demands on angiosperm xylem: Tradeoffs among storage, transport and biomechanics. Plant Cell Environ. 40, 897–913. doi: 10.1111/pce.12862
R Core Team (2020). R: A Language and Environment for Statistical Computing. Available online at: https://www.r-project.org/ (accessed October 10, 2020).
Schlesinger, W. H., and Jasechko, S. (2014). Transpiration in the global water cycle. Agric. For. Meteorol. 189–190, 115–117. doi: 10.1016/j.agrformet.2014.01.011
Secchi, F., Pagliarani, C., and Zwieniecki, M. A. (2017). The functional role of xylem parenchyma cells and aquaporins during recovery from severe water stress. Plant Cell Environ. 40, 858–871. doi: 10.1111/pce.12831
Shapiro, S. S., and Wilk, M. B. (1965). An analysis of variance test for normality (complete samples). Biometrika 52, 591–611. doi: 10.1093/biomet/52.3-4.591
Sprenger, M., and Allen, S. T. (2020). What ecohydrologic separation is and where we can go with it. Water Resour. Res. 56, 1–7. doi: 10.1029/2020WR027238
Sprenger, M., Leistert, H., Gimbel, K., and Weiler, M. (2016). Illuminating hydrological processes at the soil-vegetation-atmosphere interface with water stable isotopes. Rev. Geophys. 54, 674–704. doi: 10.1002/2015RG000515
Sprenger, M., Llorens, P., Cayuela, C., Gallart, F., and Latron, J. (2019). Mechanisms of consistently disconnected soil water pools over (pore)space and time. Hydrol. Earth Syst. Sci. Discuss. 23, 2751–2762. doi: 10.5194/hess-2019-143
Tsuruta, K., Yamamoto, H., Katsuyama, M., Kosugi, Y., Okumura, M., and Matsuo, N. (2019). Effects of cryogenic vacuum distillation on the stable isotope ratios of soil water. Hydrol. Res. Lett. 13, 1–6. doi: 10.3178/hrl.13.1
Tyree, M. T., and Hammel, H. T. (1972). The measurement of the turgor pressure and the water relations of plants by the pressure-bomb technique. J. Exp. Bot. 23, 267–282. doi: 10.1093/jxb/23.1.267
Venturas, M. D., Sperry, J. S., and Hacke, U. G. (2017). Plant xylem hydraulics: what we understand, current research, and future challenges. J. Integr. Plant Biol. 59, 356–389. doi: 10.1111/jipb.12534
Wickham, H., Averick, M., Bryan, J., Chang, W., McGowan, L., François, R., et al. (2019). Welcome to the tidyverse. J. Open Source Softw. 4, 1686. doi: 10.21105/joss.01686
Williams, D. G., Cable, W., Hultine, K., Hoedjes, J. C. B., Yepez, E. A., Simonneaux, V., et al. (2004). Evapotranspiration components determined by stable isotope, sap flow and eddy covariance techniques. Agric. Forest Meteorol. 125, 241–258. doi: 10.1016/j.agrformet.2004.04.008
Yazaki, K., Levia, D. F., Takenouchi, A., Watanabe, M., Kabeya, D., Miki, N. H., et al. (2020). Imperforate tracheary elements and vessels alleviate xylem tension under severe dehydration: insights from water release curves for excised twigs of three tree species. Am. J. Bot. 107, 1122–1135. doi: 10.1002/ajb2.1518
Zhao, L., Wang, L., Cernusak, L. A., Liu, X., Xiao, H., Zhou, M., et al. (2016). Significant difference in hydrogen isotope composition between xylem and tissue water in populus euphratica. Plant Cell Environ. 39, 1848–1857. doi: 10.1111/pce.12753
Zuecco, G., Amin, A., Frentress, J., Engel, M., Marchina, C., Anfodillo, T., et al. (2020). A comparative study of plant water extraction methods for isotopic analyses: Scholander-type pressure chamber vs. cryogenic vacuum distillation. Hydrol. Earth Syst. Sci. Discuss. doi: 10.5194/hess-2020-446. [Epub ahead of print].
Keywords: xylem water, stable isotopes, ecohydrology, gymnosperms, cryogenic vacuum distillation, pressure chamber, water extraction
Citation: Bowers WH and Williams DG (2022) Isotopic Heterogeneity of Stem Water in Conifers Is Correlated to Xylem Hydraulic Traits and Supports Multiple Residence Times. Front. Water 4:861590. doi: 10.3389/frwa.2022.861590
Received: 24 January 2022; Accepted: 14 March 2022;
Published: 08 April 2022.
Edited by:
María Poca, CONICET Institute of Applied Mathematics San Luis (IMASL), ArgentinaCopyright © 2022 Bowers and Williams. This is an open-access article distributed under the terms of the Creative Commons Attribution License (CC BY). The use, distribution or reproduction in other forums is permitted, provided the original author(s) and the copyright owner(s) are credited and that the original publication in this journal is cited, in accordance with accepted academic practice. No use, distribution or reproduction is permitted which does not comply with these terms.
*Correspondence: William H. Bowers, willhbowers@gmail.com