- Centro de Investigación en Medicina Traslacional Severo Amuchastegui (CIMETSA), Instituto Universitario de Ciencias Biomédicas de Córdoba (IUCBC), CONICET, Córdoba, Argentina
The genome tridimensional (3D) organization and its role towards the regulation of key cell processes such as transcription is currently a main question in biology. Interphase chromosomes are spatially segregated into “territories,” epigenetically-defined large domains of chromatin that interact to form “compartments” with common transcriptional status, and insulator-flanked domains called “topologically associating domains” (TADs). Moreover, chromatin organizes around nuclear structures such as lamina, speckles, or the nucleolus to acquire a higher-order genome organization. Due to recent technological advances, the different hierarchies are being solved. Particularly, advances in microscopy technologies are shedding light on the genome structure at multiple levels. Intriguingly, more and more reports point to high variability and stochasticity at the single-cell level. However, the functional consequences of such variability in genome conformation are still unsolved. Here, I will discuss the implication of the cell-to-cell heterogeneity at the different scales in the context of newly developed imaging approaches, particularly multiplexed Fluorescence in situ hybridization methods that enabled “chromatin tracing.” Extensions of these methods are now combining spatial information of dozens to thousands of genomic loci with the localization of nuclear features such as the nucleolus, nuclear speckles, or even histone modifications, creating the fast-moving field of “spatial genomics.” As our view of genome organization shifts the focus from ensemble to single-cell, new insights to fundamental questions begin to emerge.
Introduction
In eukaryotes, DNA is arranged in a three-dimensional (3D) packaging within the nucleus. The genome hierarchical 3D organization conforms a key regulatory layer of gene expression and cell fate control (Bonev and Cavalli, 2016). Individual chromosomes are spatially partitioned into discrete “chromosome territories” (Cremer and Cremer, 2001; Bolzer et al., 2005; Cremer et al., 2006). Down from the chromosomal scale, the genome is partitioned into two types of structural units. On the one hand, active (A) and inactive (B) compartments are genomic regions spanning several mega-base pairs (Mb) which tend to engage in homotypic (A-A or B-B) rather than heterotypic contacts. On the other hand, topologically associating domains (TADs) are defined as regions at the sub-Mb scale displaying higher intra-domain interactions and relatively insulated from neighboring domains.
The segregation of active and repressed chromatin was observed for the first time by Emil Heitz, who in 1928 suggested the terms “heterochromatin” and “euchromatin” (Passarge, 1979). A great deal about chromatin spatial organization has been learned thanks to the development of biochemical methods called chromatin conformation capture (3C) and 3C derivatives (Goel and Hansen, 2021; Jerkovic and Cavalli, 2021). 3C-based techniques rely on DNA crosslinking to fix the interacting sequences and nuclease fragmentation to retrieve the contact frequency of pairs of genomic positions. In particular, genome-wide maps of chromatin interaction have been obtained by sequencing-based high-throughput chromosome conformation capture techniques (Hi-C). Through initial Hi-C maps, it was found that domains sharing biochemical properties such as epigenetic marks and transcriptional status tend to interact with domains of the same type, to form A/B compartments (size ∼ 1–3 Mb), which resemble euchromatin and heterochromatin, respectively (Lieberman-Aiden et al., 2009).
The other genome “structural unit,” TADs, were discovered due to an increased genomic resolution of 3C-based methods (Dixon et al., 2012; Nora et al., 2012; Sexton et al., 2012), with an average size between 185–900 kb in mammals (Dixon et al., 2012; Rao et al., 2014; Bonev et al., 2017) and 100–150 kb in Drosophila (Ulianov et al., 2016; Wang et al., 2018). TADs organization is, for the most part, stable between cell types or through differentiation (i.e., most TAD borders are invariant) (Dixon et al., 2015; Dixon et al., 2016). Furthermore, TADs borders coincide to a high degree with replication domain boundaries (Pope et al., 2014; Dixon et al., 2016; Ulianov et al., 2016). Even more importantly, cis-regulatory elements that direct transcription are mostly restricted to interactions within a TAD (Lupiáñez et al., 2015; Dixon et al., 2016). All in all, this points to a role of TADs as conserved genome “units of regulation” or even thought as physical globular domains present in most cells of a population. As we will see from single-cell techniques, the latter is an oversimplification.
Finally, the spatial compartmentalization of nuclear events is evidenced by the spatially defined localization of processes. The existence of diverse nuclear bodies, membraneless compartments with specific tasks, is a key aspect of the nuclear organization (Misteli, 2005; Mao et al., 2011). For example, nuclear speckles are subnuclear bodies that contain mRNA processing and splicing factors (Galganski et al., 2017). It has been shown that highly transcribed Pol II regions organize around nuclear speckles, whereas inactive genomic regions are frequently associated with the nuclear periphery (Guelen et al., 2008) or the nucleolus (Quinodoz et al., 2018). Inter-chromosomal contacts are organized around nuclear bodies to create a higher-order genome organization. Additionally, another principle of non-random nuclear architecture is the radial organization model where euchromatic regions (A compartment) organize centrally with respect to nuclear lamina whereas heterochromatin (B compartment) is associated with the nuclear periphery and perinucleolar regions (Buchwalter et al., 2019; Crosetto and Bienko, 2020). More importantly, the non-random organization of the genome has meaningful effects on its function and activity. As technology develops, both imaging- and sequencing-based, there is a notorious shift in paradigm: ensemble measurements are just simply not enough to understand the structure-function relationship. Here I will discuss the microscopy improvements that lead to new insights into the stochasticity in genome organization and its influence on the mechanisms involved.
Introducing “Spatial Genomics”
Microscopy methods enable the visualization of genomic features in single cells (Xie and Liu, 2021). Fluorescence in situ hybridization (FISH) detects the physical position of targeted sequences by the annealing of labeled DNA or RNA probes. As genome-wide methods started to be widely used across many laboratories, single-cell 3D-DNA FISH was used as an orthogonal method to validate observations (Nora et al., 2012). Therefore, selected pairs of loci were used to measure physical distances and compare them with 3C contact frequencies (Giorgetti and Heard, 2016).
Two major FISH limitations can be identified when it comes to extending its throughput. The first is the probe design and production. Traditionally, FISH probes are derived from molecular cloning to vectors such as bacterial artificial chromosomes (BACs) (Roohi et al., 2008) and PCR-based methods like HD-FISH (Bienko et al., 2013). These methods are laborious and time-consuming, especially to produce multiple probes. Due to advances in high-throughput parallel chemical synthesis, it is now possible to construct FISH probes from oligonucleotides (oligos), termed Oligopaints (Beliveau et al., 2012; Beliveau et al., 2015). Oligo-based probes are selected bioinformatically and allow for great flexibility in terms of experimental design, targeting from a few kilobases (kb) to Mbs (Beliveau et al., 2018).
The other limitation is the color channels available to imaging, restricting FISH to 2–3 loci per experiment. An initial effort using a sequential color code trace a whole chromosomal arm (Lowenstein et al., 2004) although it has remained challenging to unambiguously identify multiple loci. Xiaowei Zhuang’s lab developed the concept of sequential imaging of target loci combining the flexibility of Oligopaints with microfluidics in a regular widefield fluorescence microscope to accomplish the multiplexed detection of FISH probes (Wang et al., 2016).
The idea is to use a set of oligos (hereafter “barcode”), targeting a specific locus, that shares the same overhang region that is then recognized by a fluorescently labeled secondary oligo. After hybridizing primary probes to all target regions, barcode-specific secondary probes are injected to then imaged across multiple fields of view, photobleach and start a new hybridization cycle (Figure 1A). In each cycle, the barcodes appear as fluorescent spots whose centroid position is determined with nanometric precision (Boettiger and Murphy, 2020). Therefore, the method enables a direct tracing of the chromatin path with a genomic coverage and resolution according to the design of the Oligopaint probes (i.e., size of the barcoded regions and the distance between barcodes).
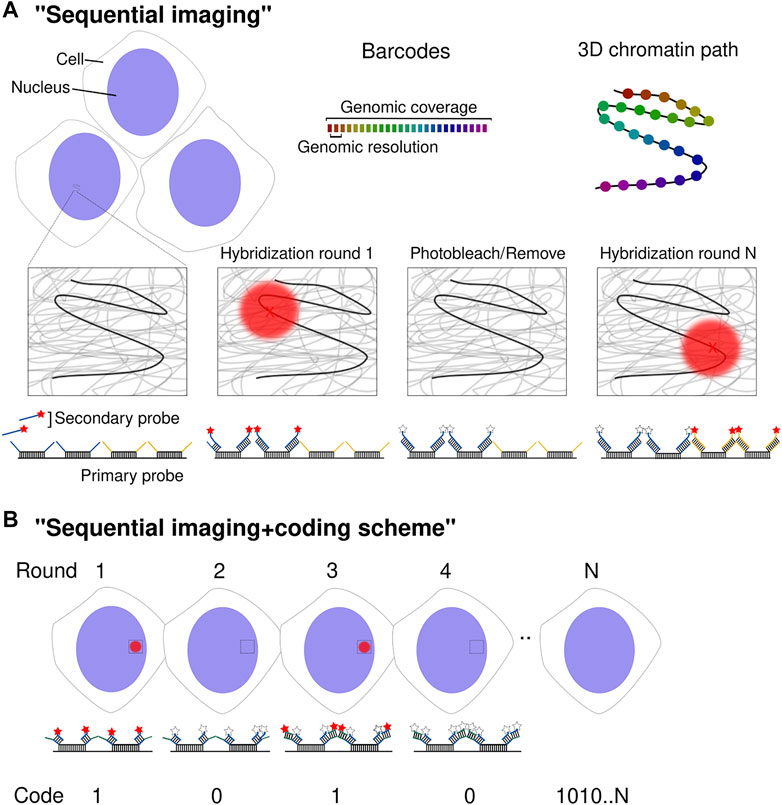
FIGURE 1. Spatial genomics approaches. (A) Schematic diagram of multiplexed DNA barcode detection. DNA loci are detected sequentially through secondary readout probes complementary to barcode-specific overhang sequences. The centroid of diffraction-limited spots (red X) is determined with nanometric precision. After each hybridization and imaging round, fluorophores are either removed or photobleached before starting a new cycle. Upon completion of N rounds, the chromatin path is determined in individual chromosomes across thousands of cells. (B) Schematic diagram of the implementation of a coding scheme using N sequential cycles. Although the procedure to determine the chromatin path is the same as in (A), a coding scheme is implemented. Every barcode is detected by more than one readout probe (two in this case) by the use of multiple overhang sequences per barcode. This leads to the detection of the same barcode in several imaging rounds. Detection is read as a “1” whereas no detection as “0”. Post-signal processing allows decoding the position of 2N barcodes.
The initial approach managed to image ∼30 genomic loci covering a whole human chromosome with a genomic resolution around the Mb and revealing that at this scale there is a strong correlation between mean spatial distance with Hi-C contact frequency (Wang et al., 2016). Following this study, three papers appeared within a 6-months window, further developing the multiplexed methods using “chromatin tracing” (Bintu et al., 2018), “Hi-M” (Cardozo Gizzi et al., 2019) and “optical reconstruction of chromatin architecture” (ORCA) (Mateo et al., 2019) and achieved a resolution of 2–30 kb at the sub-TAD scale to cover ∼20–70 regions. At this scale, it was found that TADs, discovered by 3C-based methods, indeed appeared when averaging the population chromatin spatial conformation (see below Stochasticity in Genome Organization). Furthermore, it was then possible to establish, in the same cells, the transcriptional status by imaging RNA species (Cardozo Gizzi et al., 2019; Mateo et al., 2019). In Drosophila, it was shown that active transcription is associated with the unfolding of the gene-containing TAD at the ensemble level (Cardozo Gizzi et al., 2019). In eukaryotic cells, transcription is controlled by cis-regulatory elements (CREs) such as enhancers, silencers and promoters. By using contiguous barcodes to achieve a resolution of ∼2 kb, it was possible to study CRE hubs that regulate gene expression. It was found that enhancer-promoter (E-P) distance was only a weak predictor of transcription (Mateo et al., 2019) and that distal CRE hubs are formed before gene activation (or even TADs) and may reinforce transcriptional repression (Espinola et al., 2021). Additionally, the simultaneous detection of RNA can also be used as a proxy to perform cell-type specific studies (Mateo et al., 2019; Liu et al., 2020; Espinola et al., 2021).
The “coding scheme” concept was later introduced to deliver throughput orders of magnitude higher. It was adapted from multiplexed error-robust FISH (MERFISH) (Chen et al., 2015) or sequential FISH (seqFISH) (Lubeck et al., 2014; Shah et al., 2018; Eng et al., 2019), initially developed for RNA in situ detection. Xiaowei Zhuang’s DNA-MERFISH and Long Cai’s seqFISH+ were developed in parallel and consist of embedding a particular barcode with more than one class of readout sequence, constituting a binary code. In other words, two to five different overhang sequences are added in each barcode, that will be then detected with multiple readout fluorescent oligos (Figure 1B). The “1” or “0” value of each bit corresponds to the presence or not of a particular barcode in a hybridization round. This allows for 2N genomic positions to be imaged in N rounds of hybridization. The vast majority of possible encoded barcodes are not used to implement an error detection and correction scheme. Su et al. (2020) employed 100-binary barcodes with two “1” bits and 98 “0” bits to image 1,041 genomic loci employing 50 hybridization rounds in two channels. In this study, a particular genomic locus would be decoded after being detected (on or “1”) in a particular spatial localization in two out of 100 different hybridization cycles. In Takei et al. (2021a) 2,460 genomic loci were imaged using 80 hybridization rounds in two channels.
A different spatial genomics approach is the combination of microscopy and sequencing by adapting and improving fluorescence RNA in situ sequencing (IGS) or FISSEQ technology (Lee et al., 2014). Recent developments of IGS have permitted both targeted (Oligo-FISSEQ) (Nguyen et al., 2020) or untargeted approaches (Payne et al., 2021). Oligo-FISSEQ uses barcoded Oligopaints targeting multiple genomic regions that are sequenced in situ whereas untargeted IGS uses Tn5 transposase to randomly incorporate DNA sequencing adaptors into fixed DNA, achieving a resolution of ∼1 Mb genome-wide. Finally, combining chromating tracing with multimodal RNA- and immuno-labeling (Liu et al., 2020; Su et al., 2020; Payne et al., 2021; Takei et al., 2021a) enables the profiling of genome conformation, nuclear bodies, gene expression and epigenetic status in the same cell.
In the last 3 years, this revolution kickstarted a new field. These very recent developments put us within range of genome-wide spatial maps of chromatin organization, complementing the best of genomics and microscopy fields. More and more labs are developing and implementing “spatial genomics” approaches even if at the present the methodology employs custom-made setups and requires an in-house knowledge of automated image analysis. From these approaches, the different contributions of heterogeneity to chromosome architecture at different scales are being sorted out.
Stochasticity in Genome Organization
Genome organization has a large degree of variability at the single-cell level (Finn and Misteli, 2019) and the 3D segregation of chromosomes shows a clear variability between cells. Accordingly, the relative position of a particular chromosome to each other is not “predefined” yet the “chromosome territories” are physical structures present in all cells within a population. This is not the case for A/B compartments or TADs that arise from averaging multiple cell conformations in mammalian cells. In other words, they are statistical features of genome organization not necessarily present from cell to cell. Here I will discuss the evidence supporting this claim, mainly obtained from spatial genomics techniques unless stated otherwise.
The segregation of active and inactive chromatin by the preferred contacts between chromatin of the same class is observed in single cells, that display their chromosomes in a “polarized fashion” in interphase human fibroblasts (Wang et al., 2016; Nir et al., 2018) and C. elegans embryos (Sawh et al., 2020). This indicates that compartments, or regions of active/inactive chromatin, are localized side-by-side with various degrees of intermixing. Consistent with genome-wide studies, chromatin tracing experiments found a spatial correlation between nucleoli and nuclear lamina with B-compartment regions (Liu et al., 2020; Su et al., 2020) or between speckle with A-compartment regions (Liu et al., 2020). Furthermore, the degree of segregation between compartments showed a gradual establishment during the cell cycle, increasing from G1 to G2/S phase (Su et al., 2020) as previously seen by Hi-C (Abramo et al., 2019). However, individual chromosomes display a high level of variation, from the extreme complete segregation of A- and B-clusters to a highly intermingling configuration (Liu et al., 2020; Su et al., 2020).
Microscopy reports determined a low contact probability (1–10%) of long-range associations between any pair of loci and a modest two-fold increase within TADs (Cattoni et al., 2017; Finn et al., 2019). The single-cell contact maps frequently exhibit TAD-like structures, as seen in multiple chromatin tracing studies (Bintu et al., 2018; Mateo et al., 2019; Su et al., 2020; Payne et al., 2021; Takei et al., 2021b). These are local physical domains of enhanced contact that are well separated from one another. The physical properties of domains, such as size or degree of insulation, displayed a large heterogeneity (Boettiger et al., 2016; Nir et al., 2018; Szabo et al., 2018; Luppino et al., 2020; Szabo et al., 2020). This is consistent with the high variability in TAD formation observed in single-cell sequencing-based biochemical methods (reviewed in Ulianov et al., 2017; Galitsyna and Gelfand, 2021), such as Hi-C (Flyamer et al., 2017; Nagano et al., 2017; Stevens et al., 2017; Tan et al., 2018), ChIA-Drop (Zheng et al., 2019) or scSPRITE (Arrastia et al., 2021). Consistently, the boundaries of such domains do not necessarily correspond to ensemble-averaged TADs (eTADs; Bohrer and Larson, 2021) (Figure 2).
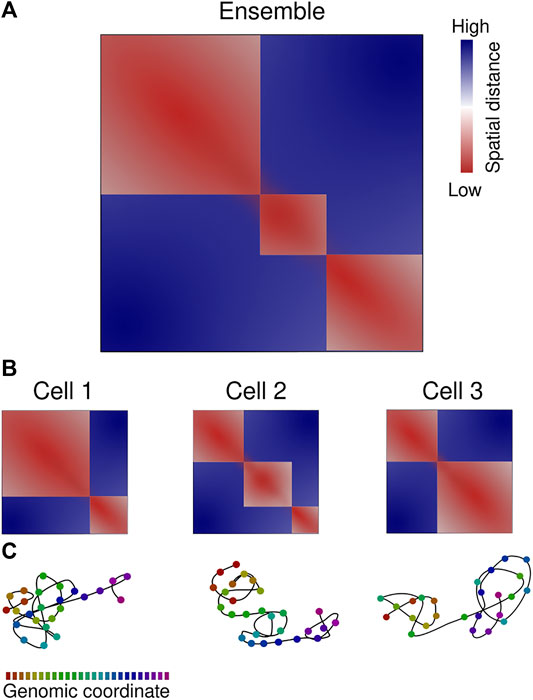
FIGURE 2. Chromatin organization is variable between cells. (A) Ensemble-averaged median spatial distance map, color-code from red to blue according to the scale bar. Three eTADs are clearly visible. (B) Single-cell spatial distance maps indicate the heterogeneity in chromatin 3D architecture. (C) Chromatin path representation of the single-cell distance maps, color-coded according to the genomic coordinate scale bar. Representation based on spatial genomic approaches.
In mammals, Hi-C-defined eTADs are frequently flanked by pairs of CTCF binding sites in convergent orientation and serve as anchors for chromatin loops (Nora et al., 2012; Rao et al., 2014). TAD-like domain boundaries were preferentially positioned at CTCF and cohesin binding sites, belonging to eTADs boundaries, peaking at ∼15% probability. However, all other genomic loci within a TAD shared a boundary probability of ∼5–7% (Bintu et al., 2018; Su et al., 2020). In contrast, Drosophila TADs, whose borders are not enriched in CTCF, are much more stable from cell-to-cell, observed both by microscopy (Szabo et al., 2018) and single-cell Hi-C (Ulianov et al., 2021); although the reasons are under investigation (Ulianov and Razin, 2021).
A very graphical example of TAD architecture at the single-cell level is this is the organization of the inactivated X chromosome, used as a model for chromosome organization (Galupa and Heard, 2018). In mammalian females, the two copies of X chromosomes display a very different transcriptional and epigenetic landscape. At the ensemble level, the inactivated X chromosome (Xi) displays only two mega domains with the boundary located at macrosatellite DXZ4. Strikingly, both the active X chromosome and Xi show TAD-like domains at the single-cell level (Cheng et al., 2021; Takei et al., 2021b).
The role of TADs in transcription regulation is still an open question, but evidence supports both a role on facilitating CREs communication within the TAD and on blocking enhancer-promoter contacts between TADs (Furlong and Levine, 2018; Cavalheiro et al., 2021). However, the stochastic nature of TADs (and compartments) questions the real influence of TADs on transcription modulation (Bohrer and Larson, 2021). The timescales involved in chromosome organization and transcription is a dimension that needs to be considered, and that is not being addressed by FISH or sequencing-based methods in fixed cells (Nollmann et al., 2021). The live-cell tracking of loci gives information on the dynamic nature of regulatory DNA contacts such as E-P interactions (Brandão et al., 2021), and thus can bring understanding into the role of 3D genome organization in CREs regulation (Schoenfelder and Fraser, 2019).
Cell-to-cell variability within a phenotypically indistinguishable population has also been found in the transcriptome and epigenome (DNA methylation and histone modification profile) (Golov et al., 2016). For example, results by microscopy indicate that mRNA levels of targeted genes fluctuate from cell to cell due to the intrinsically stochastic, infrequent events of gene activation (Raj et al., 2006). Transcriptional activation can reposition genes in space (Zink et al., 2004), possibly by the action of RNA polymerases (Heinz et al., 2018; Brandão et al., 2019). Moreover, chromatin marks exhibit high variability between cells (Takei et al., 2021a), such as the intensities of H3K4me3 histone mark at different gene bodies (Woodworth et al., 2021), that at some point may regulate chromatin compartmentalization (Wang et al., 2019) (see below). Moreover, H3K4me3 histone mark intensities at different gene bodies show great heterogeneity (Woodworth et al., 2021) or that chromatin marks exhibit high variability in embryonic stem cells (Takei et al., 2021a). Considering that transcriptional activation can reposition genes in space (Zink et al., 2004) by the action of RNA polymerases (Heinz et al., 2018; Brandão et al., 2019) or that histone modifications may regulate chromatin compartmentalization (Wang et al., 2019) (see below). Therefore, the variability in gene expression and/or epigenetic status could have a direct effect on the observed stochasticity in genome conformation at the compartment and TAD levels (Ulianov and Razin, 2021). The influence and interdependence between genome organization, epigenomics and transcription is a central question in cellular biology. In the next section, I will address this by dissecting the current knowledge on the cellular processes directing them.
Molecular Mechanisms of Spatial Organization
The two types of 3D organization found in mammalian chromosomes form by independent mechanisms (Schwarzer et al., 2017; Nuebler et al., 2018). In contrast to what was once thought, there is no hierarchy between compartments and TADs, but rather a competition between two different organization modes. The self-organization principle of the genome (Misteli, 2020) indicates that chromatin of the same type tends to interact in the space and this is directly related to the polymeric nature of the genome, although the mechanism remains elusive. Polymer physics has modeled the genome as consecutive blocks of alternating active/inactive chromatin (block copolymers), that assemble to generate the observed compartmentalization (Jost et al., 2014; Hildebrand and Dekker, 2020). It has been proposed that such compartments can arise through polymer phase separation mediated by associations of chromatin domains of similar epigenetic and/or transcriptional state (Rowley et al., 2017; Cook and Marenduzzo, 2018; Erdel and Rippe, 2018). Furthermore, a recent Hi-C study of outstanding sequencing deep revealed that median size of A/B compartments intervals is only 12.5 kb, and that even kilobase-sized domains show enhanced interactions with regions of the same class (Gu et al., 2021). However, the molecular bases of these associations are unknown.
Although spatial genetics approaches established that interactions between compartments vary from cell to cell, B-B domain contact frequencies were higher than A-A domains at distances below 75 Mb but not at higher genomic distances (Su et al., 2020), consistent with Hi-C studies in mammalian (Abramo et al., 2019) or Drosophila cells (Ulianov et al., 2021). The latter indicates that the mechanism of compartment segregation differs according to chromatin type. Accordingly, different players have been proposed, such as HP1alpha-mediated heterochromatin phase segregation (Larson et al., 2017; Strom et al., 2017) or clustering of active transcription sites (Hilbert et al., 2021). Based on polymer simulations, it was proposed that interactions between heterochromatin regions control compartmentalization over euchromatin contacts or the interaction of heterochromatin with the nuclear lamina (Falk et al., 2019). Recently, the role of homotypic repetitive elements and their RNA products has also been suggested as a mechanism of chromatin organization (Lu et al., 2021).
One spatial genomics study was able to establish a “chromatin profile” based on the multiplexed detection of several histone marks at specific DNA locus that matched ChIP-seq (Shen et al., 2012) or SPRITE measurements (Quinodoz et al., 2018) at 1-Mb resolution, but in this case with single-cell information. This analysis found “fixed” loci that, despite the variability in genome organization, are consistently associated with particular hallmarks (e.g., nuclear speckles, H3K9me3 mark, etc.) in most of the cells (Takei et al., 2021b). The existence of such “anchoring” points on each chromosome restricts their possible conformations. Because the spatial organization of nuclear bodies is cell-type dependent, they postulate that the nuclear architecture arises from the interaction between fixed or dominant loci with them. Moreover, related cell types have similar A/B compartment organization but very different nucleolar and lamina associations (Liu et al., 2020).
The loop-extrusion mechanism (Alipour and Marko, 2012) is to date the most accepted model of TAD formation in mammalian genomes (Nuebler et al., 2018). It postulates that the ring-shaped cohesin complex acts as a molecular motor actively extruding DNA and forming increasingly long chromatin loops that are stalled at convergent CTCF sites (Sanborn et al., 2015; Fudenberg et al., 2016; Fudenberg et al., 2017). Once bound to chromatin, the cohesin ring stochastically detaches from it, giving rise to highly dynamic structures (Hansen et al., 2017). This paradigm explains the Hi-C data showing the existence of chromatin loops between eTAD boundaries that present CTCF and cohesin complexes (Rao et al., 2014; Bonev et al., 2017). Moreover, when cohesin-loading factor Nipbl is removed from mouse cells, eTAD organization is lost (Schwarzer et al., 2017). However, chromatin tracing indicates that in single cells pairs of eTADs boundaries do not show a smaller physical distance distribution compared to control loci (Su et al., 2020) but rather there is a progressive looping anchored at the stronger CTCF binding site that progresses to more and more downstream loci (Beckwith et al., 2021). More strikingly, TAD-like domains persist upon cohesin depletion, although the boundary positions are randomized (Bintu et al., 2018). In line with this, even genomic regions that do now display eTADs form domain-like structures indicating that the folding of chromatin into this architecture is an intrinsic characteristic and that loop extrusion is a regulator of this process.
The process of compartmentalization and TAD formation shapes the genome architecture and changes the chromatin accessibility of genes and regulatory elements, modulating the functional output of genomes (Rowley and Corces, 2018). Among different cell types, the general principles of single-cell genome organization delineated above are conserved. However, cell-type specific spatial configurations delineate the functional differences (Liu et al., 2020; Takei et al., 2021b). Based on microscopy observations, we have proposed through the concept of “modulated stochasticity” that subtle changes in interaction frequencies give rise to measurable differences in genome architecture and could have a meaningful role in gene regulation (Cattoni et al., 2017). Complementary, nuclear structures such as speckles, which in practice act as chromatin scaffolds, might define different cell types and states. The stochasticity of genome architecture is a consequence of its polymeric nature, and it is modulated by several mechanisms mediated by proteins that interact through the sequence information. These mechanisms include, but are not restricted to, the processes of compartmentalization and loop extrusion. In general, sequences encode information for specific protein binding whose abundance and action will generate/regulate contacts between genomic loci.
Conclusion and Future Perspectives
In this review, I have summarized the technological improvements and recent discoveries of spatial genomic approaches. These advances, together with single-cell sequencing methods, are shifting the focus from ensemble measurements to the organization of genomes at the single-cell level to account for the observed high degree of stochasticity and heterogeneity.
Genome organization is shaped from its polymeric nature together with biological processes such as loop-extrusion, which are both stochastic in nature. The question that emerges is what is the biological relevance of such variable organization. In other words, how the genome architecture shapes transcription: the structure/function conundrum. Maybe the important point here is it not anymore whether genome conformation is cause or consequence of genome function but rather what is the relationship between them. Furthermore, epigenetics and gene expression display a high degree of cell-to-cell variability. In order to reveal the contribution of each aspect to the function of genomes, new technologies capable of simultaneous detection of transcriptional output, epigenetic state and 3D conformation in the same cell will have to emerge. Undoubtedly, live-cell measurements, currently limited in scope, will also be necessary to understand the temporal aspects of genome organization. More importantly, despite the efforts, the function and activity of TADs and nuclear compartments continue to be unresolved. How are the specific genomic interactions generated if such heterogeneity is present? Moreover, how stochasticity is modulated to allow for precise spatio-temporal regulation of gene expression? Further developments in microscopy, genome-wide approaches and polymer simulations hold promise for the understanding of these key questions.
Author Contributions
The author confirms being the sole contributor of this work and has approved it for publication.
Funding
The work in Cardozo Gizzi’s lab is funded by the Agencia Nacional de Promoción Científica y Tecnológica (ANPCYT) of Argentina (grant PICT-2019-00291) and Ministerio de Ciencia y Tecnología, Provincia de Córdoba (Res 79/18, 2018). AMCG is an investigator of Consejo Nacional de Investigaciones Científicas y Técnicas (CONICET), Argentina.
Conflict of Interest
The author declares that the research was conducted in the absence of any commercial or financial relationships that could be construed as a potential conflict of interest.
Publisher’s Note
All claims expressed in this article are solely those of the authors and do not necessarily represent those of their affiliated organizations, or those of the publisher, the editors and the reviewers. Any product that may be evaluated in this article, or claim that may be made by its manufacturer, is not guaranteed or endorsed by the publisher.
Acknowledgments
I thank Ana Lis Moyano, Gonzalo Guendulain and Carlos Wilson for the critical reading of the manuscript.
References
Abramo, K., Valton, A.-L., Venev, S. V., Ozadam, H., Fox, A. N., and Dekker, J. (2019). A Chromosome Folding Intermediate at the Condensin-To-Cohesin Transition during Telophase. Nat. Cell Biol. 21, 1393–1402. doi:10.1038/s41556-019-0406-2
Alipour, E., and Marko, J. F. (2012). Self-Organization of Domain Structures by DNA-Loop-Extruding Enzymes. Nucleic Acids Res. 40, 11202–11212. doi:10.1093/nar/gks925
Arrastia, M. V., Jachowicz, J. W., Ollikainen, N., Curtis, M. S., Lai, C., Quinodoz, S. A., et al. (2021). Single-Cell Measurement of Higher-Order 3D Genome Organization with scSPRITE. Nat. Biotechnol. doi:10.1038/s41587-021-00998-1
Beckwith, K. S., Ødegård-Fougner, Ø., Morero, N. R., Barton, C., Schueder, F., Alexander, S., et al. (2021). Visualization of Loop Extrusion by DNA Nanoscale Tracing in Single Human Cells. bioRxiv. doi:10.1101/2021.04.12.439407
Beliveau, B. J., Boettiger, A. N., Avendaño, M. S., Jungmann, R., McCole, R. B., Joyce, E. F., et al. (2015). Single-Molecule Super-Resolution Imaging of Chromosomes and In Situ Haplotype Visualization Using Oligopaint FISH Probes. Nat. Commun. 6, 7147. doi:10.1038/ncomms8147
Beliveau, B. J., Joyce, E. F., Apostolopoulos, N., Yilmaz, F., Fonseka, C. Y., McCole, R. B., et al. (2012). Versatile Design and Synthesis Platform for Visualizing Genomes with Oligopaint FISH Probes. Proc. Natl. Acad. Sci. 109, 21301–21306. doi:10.1073/pnas.1213818110
Beliveau, B. J., Kishi, J. Y., Nir, G., Sasaki, H. M., Saka, S. K., Nguyen, S. C., et al. (2018). OligoMiner Provides a Rapid, Flexible Environment for the Design of Genome-Scale Oligonucleotide In Situ Hybridization Probes. Proc. Natl. Acad. Sci. USA 115, E2183–E2192. doi:10.1073/pnas.1714530115
Bienko, M., Crosetto, N., Teytelman, L., Klemm, S., Itzkovitz, S., and van Oudenaarden, A. (2013). A Versatile Genome-Scale PCR-Based Pipeline for High-Definition DNA FISH. Nat. Methods 10, 122–124. doi:10.1038/nmeth.2306
Bintu, B., Mateo, L. J., Su, J.-H., Sinnott-Armstrong, N. A., Parker, M., Kinrot, S., et al. (2018). Super-Resolution Chromatin Tracing Reveals Domains and Cooperative Interactions in Single Cells. Science 362, eaau1783. doi:10.1126/science.aau1783
Boettiger, A., and Murphy, S. (2020). Advances in Chromatin Imaging at Kilobase-Scale Resolution. Trends Genet. 36, 273–287. doi:10.1016/j.tig.2019.12.010
Boettiger, A. N., Bintu, B., Moffitt, J. R., Wang, S., Beliveau, B. J., Fudenberg, G., et al. (2016). Super-Resolution Imaging Reveals Distinct Chromatin Folding for Different Epigenetic States. Nature 529, 418–422. doi:10.1038/nature16496
Bohrer, C. H., and Larson, D. R. (2021). The Stochastic Genome and its Role in Gene Expression. Cold Spring Harb. Perspect. Biol. 13, a040386. doi:10.1101/cshperspect.a040386
Bolzer, A., Kreth, G., Solovei, I., Koehler, D., Saracoglu, K., Fauth, C., et al. (2005). Three-Dimensional Maps of All Chromosomes in Human Male Fibroblast Nuclei and Prometaphase Rosettes. PLoS Biol. 3, e157. doi:10.1371/journal.pbio.0030157
Bonev, B., and Cavalli, G. (2016). Organization and Function of the 3D Genome. Nat. Rev. Genet. 17, 661–678. doi:10.1038/nrg.2016.112
Bonev, B., Mendelson Cohen, N., Szabo, Q., Fritsch, L., Papadopoulos, G. L., Lubling, Y., et al. (2017). Multiscale 3D Genome Rewiring during Mouse Neural Development. Cell 171, 557–572.e24. doi:10.1016/j.cell.2017.09.043
Brandão, H. B., Gabriele, M., and Hansen, A. S. (2021). Tracking and Interpreting Long-Range Chromatin Interactions with Super-Resolution Live-Cell Imaging. Curr. Opin. Cell Biol. 70, 18–26. doi:10.1016/j.ceb.2020.11.002
Brandão, H. B., Paul, P., van den Berg, A. A., Rudner, D. Z., Wang, X., and Mirny, L. A. (2019). RNA Polymerases as Moving Barriers to Condensin Loop Extrusion. Proc. Natl. Acad. Sci. USA 116, 20489–20499. doi:10.1073/pnas.1907009116
Buchwalter, A., Kaneshiro, J. M., and Hetzer, M. W. (2019). Coaching from the Sidelines: the Nuclear Periphery in Genome Regulation. Nat. Rev. Genet. 20, 39–50. doi:10.1038/s41576-018-0063-5
Cardozo Gizzi, A. M., Cattoni, D. I., Fiche, J.-B., Espinola, S. M., Gurgo, J., Messina, O., et al. (2019). Microscopy-Based Chromosome Conformation Capture Enables Simultaneous Visualization of Genome Organization and Transcription in Intact Organisms. Mol. Cell 74, 212–222.e5. doi:10.1016/j.molcel.2019.01.011
Cattoni, D. I., Cardozo Gizzi, A. M., Georgieva, M., Di Stefano, M., Valeri, A., Chamousset, D., et al. (2017). Single-Cell Absolute Contact Probability Detection Reveals Chromosomes Are Organized by Multiple Low-Frequency yet Specific Interactions. Nat. Commun. 8, 1753. doi:10.1038/s41467-017-01962-x
Cavalheiro, G. R., Pollex, T., and Furlong, E. E. (2021). To Loop or Not to Loop: what Is the Role of TADs in Enhancer Function and Gene Regulation? Curr. Opin. Genet. Develop. 67, 119–129. doi:10.1016/j.gde.2020.12.015
Chen, K. H., Boettiger, A. N., Moffitt, J. R., Wang, S., and Zhuang, X. (2015). RNA Imaging. Spatially Resolved, Highly Multiplexed RNA Profiling in Single Cells. Science 348, aaa6090. doi:10.1126/science.aaa6090
Cheng, Y., Liu, M., Hu, M., and Wang, S. (2021). TAD-Like Single-Cell Domain Structures Exist on Both Active and Inactive X Chromosomes and Persist under Epigenetic Perturbations. Genome Biol. 22, 309. doi:10.1186/s13059-021-02523-8
Cook, P. R., and Marenduzzo, D. (2018). Transcription-Driven Genome Organization: a Model for Chromosome Structure and the Regulation of Gene Expression Tested through Simulations. Nucleic Acids Res. 46, 9895–9906. doi:10.1093/nar/gky763
Cremer, T., and Cremer, C. (2001). Chromosome Territories, Nuclear Architecture and Gene Regulation in Mammalian Cells. Nat. Rev. Genet. 2, 292–301. doi:10.1038/35066075
Cremer, T., Cremer, M., Dietzel, S., Müller, S., Solovei, I., and Fakan, S. (2006). Chromosome Territories - a Functional Nuclear Landscape. Curr. Opin. Cell Biol. 18, 307–316. doi:10.1016/j.ceb.2006.04.007
Crosetto, N., and Bienko, M. (2020). Radial Organization in the Mammalian Nucleus. Front. Genet. 11. doi:10.3389/fgene.2020.00033
Dixon, J. R., Gorkin, D. U., and Ren, B. (2016). Chromatin Domains: The Unit of Chromosome Organization. Mol. Cell 62, 668–680. doi:10.1016/j.molcel.2016.05.018
Dixon, J. R., Jung, I., Selvaraj, S., Shen, Y., Antosiewicz-Bourget, J. E., Lee, A. Y., et al. (2015). Chromatin Architecture Reorganization during Stem Cell Differentiation. Nature 518, 331–336. doi:10.1038/nature14222
Dixon, J. R., Selvaraj, S., Yue, F., Kim, A., Li, Y., Shen, Y., et al. (2012). Topological Domains in Mammalian Genomes Identified by Analysis of Chromatin Interactions. Nature 485, 376–380. doi:10.1038/nature11082
Eng, C.-H. L., Lawson, M., Zhu, Q., Dries, R., Koulena, N., Takei, Y., et al. (2019). Transcriptome-Scale Super-Resolved Imaging in Tissues by RNA seqFISH+. Nature 568, 235–239. doi:10.1038/s41586-019-1049-y
Erdel, F., and Rippe, K. (2018). Formation of Chromatin Subcompartments by Phase Separation. Biophysical J. 114, 2262–2270. doi:10.1016/j.bpj.2018.03.011
Espinola, S. M., Götz, M., Bellec, M., Messina, O., Fiche, J.-B., Houbron, C., et al. (2021). Cis-Regulatory Chromatin Loops Arise before TADs and Gene Activation, and Are Independent of Cell Fate during Early Drosophila Development. Nat. Genet. 53, 477–486. doi:10.1038/s41588-021-00816-z
Falk, M., Feodorova, Y., Naumova, N., Imakaev, M., Lajoie, B. R., Leonhardt, H., et al. (2019). Heterochromatin Drives Compartmentalization of Inverted and Conventional Nuclei. Nature 570, 395–399. doi:10.1038/s41586-019-1275-3
Finn, E. H., and Misteli, T. (2019). Molecular Basis and Biological Function of Variability in Spatial Genome Organization. Science 365. doi:10.1126/science.aaw9498
Finn, E. H., Pegoraro, G., Brandão, H. B., Valton, A.-L., Oomen, M. E., Dekker, J., et al. (2019). Extensive Heterogeneity and Intrinsic Variation in Spatial Genome Organization. Cell 176, 1502–1515.e10. doi:10.1016/j.cell.2019.01.020
Flyamer, I. M., Gassler, J., Imakaev, M., Brandão, H. B., Ulianov, S. V., Abdennur, N., et al. (2017). Single-Nucleus Hi-C Reveals Unique Chromatin Reorganization at Oocyte-To-Zygote Transition. Nature 544, 110–114. doi:10.1038/nature21711
Fudenberg, G., Abdennur, N., Imakaev, M., Goloborodko, A., and Mirny, L. A. (2017). Emerging Evidence of Chromosome Folding by Loop Extrusion. Cold Spring Harb. Symp. Quant. Biol. 82, 45–55. doi:10.1101/sqb.2017.82.034710
Fudenberg, G., Imakaev, M., Lu, C., Goloborodko, A., Abdennur, N., and Mirny, L. A. (2016). Formation of Chromosomal Domains by Loop Extrusion. Cell Rep. 15, 2038–2049. doi:10.1016/j.celrep.2016.04.085
Furlong, E. E. M., and Levine, M. (2018). Developmental Enhancers and Chromosome Topology. Science 361, 1341–1345. doi:10.1126/science.aau0320
Galganski, L., Urbanek, M. O., and Krzyzosiak, W. J. (2017). Nuclear Speckles: Molecular Organization, Biological Function and Role in Disease. Nucleic Acids Res. 45, 10350–10368. doi:10.1093/nar/gkx759
Galitsyna, A. A., and Gelfand, M. S. (2021). Single-Cell Hi-C Data Analysis: Safety in Numbers. Brief. Bioinform. doi:10.1093/bib/bbab316
Galupa, R., and Heard, E. (2018). X-Chromosome Inactivation: A Crossroads between Chromosome Architecture and Gene Regulation. Annu. Rev. Genet. 52, 535–566. doi:10.1146/annurev-genet-120116-024611
Giorgetti, L., and Heard, E. (2016). Closing the Loop: 3C versus DNA FISH. Genome Biol. 17, 215. doi:10.1186/s13059-016-1081-2
Goel, V. Y., and Hansen, A. S. (2021). The Macro and Micro of Chromosome Conformation Capture. Wiley Interdiscip. Rev. Dev. Biol. 10, e395. doi:10.1002/wdev.395
Golov, A. K., Razin, S. V., and Gavrilov, A. A. (2016). Single-Cell Genome-Wide Studies Give New Insight into Nongenetic Cell-To-Cell Variability in Animals. Histochem. Cell Biol. 146, 239–254. doi:10.1007/s00418-016-1466-z
Gu, H., Harris, H., Olshansky, M., Eliaz, Y., Krishna, A., Kalluchi, A., et al. (2021). Fine-Mapping of Nuclear Compartments Using Ultra-Deep Hi-C Shows that Active Promoter and Enhancer Elements Localize in the Active A Compartment Even when Adjacent Sequences Do Not. bioRxiv, 462599. doi:10.0310.1101/2021.10.03.462599
Guelen, L., Pagie, L., Brasset, E., Meuleman, W., Faza, M. B., Talhout, W., et al. (2008). Domain Organization of Human Chromosomes Revealed by Mapping of Nuclear Lamina Interactions. Nature 453, 948–951. doi:10.1038/nature06947
Hansen, A. S., Pustova, I., Cattoglio, C., Tjian, R., and Darzacq, X. (2017). CTCF and Cohesin Regulate Chromatin Loop Stability with Distinct Dynamics. Elife 6. doi:10.7554/eLife.25776
Heinz, S., Texari, L., Hayes, M. G. B., Urbanowski, M., Chang, M. W., Givarkes, N., et al. (2018). Transcription Elongation Can Affect Genome 3D Structure. Cell 174, 1522–1536.e22. doi:10.1016/j.cell.2018.07.047
Hilbert, L., Sato, Y., Kuznetsova, K., Bianucci, T., Kimura, H., Jülicher, F., et al. (2021). Transcription Organizes Euchromatin via Microphase Separation. Nat. Commun. 12, 1–12. doi:10.1038/s41467-021-21589-3
Hildebrand, E. M., and Dekker, J. (2020). Mechanisms and Functions of Chromosome Compartmentalization. Trends Biochem. Sci. 45, 385–396. doi:10.1016/j.tibs.2020.01.002
Jerkovic´, I., and Cavalli, G. (2021). Understanding 3D Genome Organization by Multidisciplinary Methods. Nat. Rev. Mol. Cell Biol. 22, 511–528. doi:10.1038/s41580-021-00362-w
Jost, D., Carrivain, P., Cavalli, G., and Vaillant, C. (2014). Modeling Epigenome Folding: Formation and Dynamics of Topologically Associated Chromatin Domains. Nucleic Acids Res. 42, 9553–9561. doi:10.1093/nar/gku698
Larson, A. G., Elnatan, D., Keenen, M. M., Trnka, M. J., Johnston, J. B., Burlingame, A. L., et al. (2017). Liquid Droplet Formation by HP1α Suggests a Role for Phase Separation in Heterochromatin. Nature 547, 236–240. doi:10.1038/nature22822
Lee, J. H., Daugharthy, E. R., Scheiman, J., Kalhor, R., Yang, J. L., Ferrante, T. C., et al. (2014). Highly Multiplexed Subcellular RNA Sequencing In Situ. Science 343, 1360–1363. doi:10.1126/science.1250212
Lieberman-Aiden, E., van Berkum, N. L., Williams, L., Imakaev, M., Ragoczy, T., Telling, A., et al. (2009). Comprehensive Mapping of Long-Range Interactions Reveals Folding Principles of the Human Genome. Science 326, 289–293. doi:10.1126/science.1181369
Liu, M., Lu, Y., Yang, B., Chen, Y., Radda, J. S. D., Hu, M., et al. (2020). Multiplexed Imaging of Nucleome Architectures in Single Cells of Mammalian Tissue. Nat. Commun. 11, 2907. doi:10.1038/s41467-020-16732-5
Lowenstein, M. G., Goddard, T. D., and Sedat, J. W. (2004). Long-Range Interphase Chromosome Organization in Drosophila: A Study Using Color Barcoded Fluorescence In Situ Hybridization and Structural Clustering Analysis. MBoC 15, 5678–5692. doi:10.1091/mbc.e04-04-0289
Lu, J. Y., Chang, L., Li, T., Wang, T., Yin, Y., Zhan, G., et al. (2021). Homotypic Clustering of L1 and B1/Alu Repeats Compartmentalizes the 3D Genome. Cell Res 31, 613–630. doi:10.1038/s41422-020-00466-6
Lubeck, E., Coskun, A. F., Zhiyentayev, T., Ahmad, M., and Cai, L. (2014). Single-Cell In Situ RNA Profiling by Sequential Hybridization. Nat. Methods 11, 360–361. doi:10.1038/nmeth.2892
Lupiáñez, D. G., Kraft, K., Heinrich, V., Krawitz, P., Brancati, F., Klopocki, E., et al. (2015). Disruptions of Topological Chromatin Domains Cause Pathogenic Rewiring of Gene-Enhancer Interactions. Cell 161, 1012–1025. doi:10.1016/j.cell.2015.04.004
Luppino, J. M., Park, D. S., Nguyen, S. C., Lan, Y., Xu, Z., Yunker, R., et al. (2020). Cohesin Promotes Stochastic Domain Intermingling to Ensure Proper Regulation of Boundary-Proximal Genes. Nat. Genet. 52, 840–848. doi:10.1038/s41588-020-0647-9
Mao, Y. S., Zhang, B., and Spector, D. L. (2011). Biogenesis and Function of Nuclear Bodies. Trends Genet. 27, 295–306. doi:10.1016/j.tig.2011.05.006
Mateo, L. J., Murphy, S. E., Hafner, A., Cinquini, I. S., Walker, C. A., and Boettiger, A. N. (2019). Visualizing DNA Folding and RNA in Embryos at Single-Cell Resolution. Nature 568, 49–54. doi:10.1038/s41586-019-1035-4
Misteli, T. (2020). The Self-Organizing Genome: Principles of Genome Architecture and Function. Cell 183, 28–45. doi:10.1016/j.cell.2020.09.014
Nagano, T., Lubling, Y., Várnai, C., Dudley, C., Leung, W., Baran, Y., et al. (2017). Cell-Cycle Dynamics of Chromosomal Organization at Single-Cell Resolution. Nature 547, 61–67. doi:10.1038/nature23001
Nguyen, H. Q., Chattoraj, S., Castillo, D., Nguyen, S. C., Nir, G., Lioutas, A., et al. (2020). 3D Mapping and Accelerated Super-Resolution Imaging of the Human Genome Using In Situ Sequencing. Nat. Methods 17, 822–832. doi:10.1038/s41592-020-0890-0
Nir, G., Farabella, I., Pérez Estrada, C., Ebeling, C. G., Beliveau, B. J., Sasaki, H. M., et al. (2018). Walking along Chromosomes with Super-Resolution Imaging, Contact Maps, and Integrative Modeling. PLoS Genet. 14, e1007872. doi:10.1371/journal.pgen.1007872
Nollmann, M., Bennabi, I., Götz, M., and Gregor, T. (2021). The Impact of Space and Time on the Functional Output of the Genome. Cold Spring Harb. Perspect. Biol., a040378. doi:10.1101/cshperspect.a040378
Nora, E. P., Lajoie, B. R., Schulz, E. G., Giorgetti, L., Okamoto, I., Servant, N., et al. (2012). Spatial Partitioning of the Regulatory Landscape of the X-Inactivation centre. Nature 485, 381–385. doi:10.1038/nature11049
Nuebler, J., Fudenberg, G., Imakaev, M., Abdennur, N., and Mirny, L. A. (2018). Chromatin Organization by an Interplay of Loop Extrusion and Compartmental Segregation. Proc. Natl. Acad. Sci. USA 115, E6697–E6706. doi:10.1073/pnas.1717730115
Passarge, E. (1979). Emil Heitz and the Concept of Heterochromatin: Longitudinal Chromosome Differentiation Was Recognized Fifty Years Ago. Am. J. Hum. Genet. 31, 106–115.
Payne, A. C., Chiang, Z. D., Reginato, P. L., Mangiameli, S. M., Murray, E. M., Yao, C.-C., et al. (2021). In Situ genome Sequencing Resolves DNA Sequence and Structure in Intact Biological Samples. Science 371, eaay3446. doi:10.1126/science.aay3446
Pope, B. D., Ryba, T., Dileep, V., Yue, F., Wu, W., Denas, O., et al. (2014). Topologically Associating Domains Are Stable Units of Replication-Timing Regulation. Nature 515, 402–405. doi:10.1038/nature13986
Quinodoz, S. A., Ollikainen, N., Tabak, B., Palla, A., Schmidt, J. M., Detmar, E., et al. (2018). Higher-Order Inter-Chromosomal Hubs Shape 3D Genome Organization in the Nucleus. Cell 174, 744–757.e24. doi:10.1016/j.cell.2018.05.024
Raj, A., Peskin, C. S., Tranchina, D., Vargas, D. Y., and Tyagi, S. (2006). Stochastic mRNA Synthesis in Mammalian Cells. PLoS Biol. 4, e309. doi:10.1371/journal.pbio.0040309
Rao, S. S. P., Huntley, M. H., Durand, N. C., Stamenova, E. K., Bochkov, I. D., Robinson, J. T., et al. (2014). A 3D Map of the Human Genome at Kilobase Resolution Reveals Principles of Chromatin Looping. Cell 159, 1665–1680. doi:10.1016/j.cell.2014.11.021
Roohi, J., Cammer, M., Montagna, C., and Hatchwell, E. (2008). An Improved Method for Generating BAC DNA Suitable for FISH. Cytogenet. Genome Res. 121, 7–9. doi:10.1159/000124374
Rowley, M. J., and Corces, V. G. (2018). Organizational Principles of 3D Genome Architecture. Nat. Rev. Genet. 19, 789–800. doi:10.1038/s41576-018-0060-8
Rowley, M. J., Nichols, M. H., Lyu, X., Ando-Kuri, M., Rivera, I. S. M., Hermetz, K., et al. (2017). Evolutionarily Conserved Principles Predict 3D Chromatin Organization. Mol. Cell 67, 837–852.e7. doi:10.1016/j.molcel.2017.07.022
Sanborn, A. L., Rao, S. S. P., Huang, S.-C., Durand, N. C., Huntley, M. H., Jewett, A. I., et al. (2015). Chromatin Extrusion Explains Key Features of Loop and Domain Formation in Wild-Type and Engineered Genomes. Proc. Natl. Acad. Sci. USA 112, E6456–E6465. doi:10.1073/pnas.1518552112
Sawh, A. N., Shafer, M. E. R., Su, J.-H., Zhuang, X., Wang, S., and Mango, S. E. (2020). Lamina-Dependent Stretching and Unconventional Chromosome Compartments in Early C. elegans Embryos. Mol. Cell 78, 96–111.e6. doi:10.1016/j.molcel.2020.02.006
Schoenfelder, S., and Fraser, P. (2019). Long-Range Enhancer-Promoter Contacts in Gene Expression Control. Nat. Rev. Genet. 20, 437–455. doi:10.1038/s41576-019-0128-0
Schwarzer, W., Abdennur, N., Goloborodko, A., Pekowska, A., Fudenberg, G., Loe-Mie, Y., et al. (2017). Two Independent Modes of Chromatin Organization Revealed by Cohesin Removal. Nature 551, 51–56. doi:10.1038/nature24281
Sexton, T., Yaffe, E., Kenigsberg, E., Bantignies, F., Leblanc, B., Hoichman, M., et al. (2012). Three-Dimensional Folding and Functional Organization Principles of the Drosophila Genome. Cell 148, 458–472. doi:10.1016/j.cell.2012.01.010
Shah, S., Takei, Y., Zhou, W., Lubeck, E., Yun, J., Eng, C.-H. L., et al. (2018). Dynamics and Spatial Genomics of the Nascent Transcriptome by Intron seqFISH. Cell 174, 363–376.e16. doi:10.1016/j.cell.2018.05.035
Shen, Y., Yue, F., McCleary, D. F., Ye, Z., Edsall, L., Kuan, S., et al. (2012). A Map of the Cis-Regulatory Sequences in the Mouse Genome. Nature 488, 116–120. doi:10.1038/nature11243
Stevens, T. J., Lando, D., Basu, S., Atkinson, L. P., Cao, Y., Lee, S. F., et al. (2017). 3D Structures of Individual Mammalian Genomes Studied by Single-Cell Hi-C. Nature 544, 59–64. doi:10.1038/nature21429
Strom, A. R., Emelyanov, A. V., Mir, M., Fyodorov, D. V., Darzacq, X., and Karpen, G. H. (2017). Phase Separation Drives Heterochromatin Domain Formation. Nature 547, 241–245. doi:10.1038/nature22989
Su, J.-H., Zheng, P., Kinrot, S. S., Bintu, B., and Zhuang, X. (2020). Genome-Scale Imaging of the 3D Organization and Transcriptional Activity of Chromatin. Cell 182, 1641–1659.e26. doi:10.1016/j.cell.2020.07.032
Szabo, Q., Jost, D., Chang, J. M., Cattoni, D. I., Papadopoulos, G. L., Bonev, B., et al. (2018). TADs Are 3D Structural Units of Higher-Order Chromosome Organization in Drosophila. Sci. Adv. 4, eaar8082. doi:10.1126/sciadv.aar8082
Szabo, Q., Donjon, A., Jerković, I., Papadopoulos, G. L., Cheutin, T., Bonev, B., et al. (2020). Regulation of Single-Cell Genome Organization into TADs and Chromatin Nanodomains. Nat. Genet. 52, 1151–1157. doi:10.1038/s41588-020-00716-8
Takei, Y., Yun, J., Zheng, S., Ollikainen, N., Pierson, N., White, J., et al. (2021a). Integrated Spatial Genomics Reveals Global Architecture of Single Nuclei. Nature 590, 344–350. doi:10.1038/s41586-020-03126-2
Takei, Y., Zheng, S., Yun, J., Shah, S., Pierson, N., White, J., et al. (2021b). Single-Cell Nuclear Architecture across Cell Types in the Mouse Brain. Science, eabj1966. doi:10.1126/science.abj1966
Tan, L., Xing, D., Chang, C.-H., Li, H., and Xie, X. S. (2018). Three-Dimensional Genome Structures of Single Diploid Human Cells. Science 361, 924–928. doi:10.1126/science.aat5641
Ulianov, S. V., Khrameeva, E. E., Gavrilov, A. A., Flyamer, I. M., Kos, P., Mikhaleva, E. A., et al. (2016). Active Chromatin and Transcription Play a Key Role in Chromosome Partitioning into Topologically Associating Domains. Genome Res. 26, 70–84. doi:10.1101/gr.196006.115
Ulianov, S. V., and Razin, S. V. (2021). The Two Waves in Single-Cell 3D Genomics. Semin. Cell Dev. Biol. doi:10.1016/j.semcdb.2021.05.021
Ulianov, S. V., Tachibana-Konwalski, K., and Razin, S. V. (2017). Single-Cell Hi-C Bridges Microscopy and Genome-Wide Sequencing Approaches to Study 3D Chromatin Organization. Bioessays 39, 1700104. doi:10.1002/bies.201700104
Ulianov, S. V., Zakharova, V. V., Galitsyna, A. A., Kos, P. I., Polovnikov, K. E., Flyamer, I. M., et al. (2021). Order and Stochasticity in the Folding of Individual Drosophila Genomes. Nat. Commun. 12, 41. doi:10.1038/s41467-020-20292-z
Wang, L., Gao, Y., Zheng, X., Liu, C., Dong, S., Li, R., et al. (2019). Histone Modifications Regulate Chromatin Compartmentalization by Contributing to a Phase Separation Mechanism. Mol. Cell 76, 646–659.e6. doi:10.1016/j.molcel.2019.08.019
Wang, Q., Sun, Q., Czajkowsky, D. M., and Shao, Z. (2018). Sub-kb Hi-C in D. melanogaster Reveals Conserved Characteristics of TADs between Insect and Mammalian Cells. Nat. Commun. 9, 188–8. doi:10.1038/s41467-017-02526-9
Wang, S., Su, J.-H., Beliveau, B. J., Bintu, B., Moffitt, J. R., Wu, C.-T., et al. (2016). Spatial Organization of Chromatin Domains and Compartments in Single Chromosomes. Science 353, 598–602. doi:10.1126/science.aaf8084
Woodworth, M. A., Ng, K. K. H., Halpern, A. R., Pease, N. A., Nguyen, P. H. B., Kueh, H. Y., et al. (2021). Multiplexed Single-Cell Profiling of Chromatin States at Genomic Loci by Expansion Microscopy. Nucleic Acids Res. 49, e82. doi:10.1093/nar/gkab423
Xie, L., and Liu, Z. (2021). Single-Cell Imaging of Genome Organization and Dynamics. Mol. Syst. Biol. 17, e9653. doi:10.15252/msb.20209653
Zheng, M., Tian, S. Z., Capurso, D., Kim, M., Maurya, R., Lee, B., et al. (2019). Multiplex Chromatin Interactions with Single-Molecule Precision. Nature 566, 558–562. doi:10.1038/s41586-019-0949-1
Keywords: chromatin 3D architecture, chromosome conformation, topologically associated domain (TAD), fluorescence in situ cell hybridization (FISH), oligopaint, transcriptional regulation, genome organization, stochasticity
Citation: Cardozo Gizzi AM (2021) A Shift in Paradigms: Spatial Genomics Approaches to Reveal Single-Cell Principles of Genome Organization. Front. Genet. 12:780822. doi: 10.3389/fgene.2021.780822
Received: 21 September 2021; Accepted: 03 November 2021;
Published: 19 November 2021.
Edited by:
Veniamin Fishman, Institute of Cytology and Genetics (RAS), RussiaReviewed by:
Krishna Mohan Parsi, University of Massachusetts Medical School, United StatesAleksandra Galitsyna, Skolkovo Institute of Science and Technology, Russia
Ilya M. Flyamer, Medical Research Council (MRC) Institute of Genetics and Molecular Medicine (IGMM), United Kingdom
Copyright © 2021 Cardozo Gizzi. This is an open-access article distributed under the terms of the Creative Commons Attribution License (CC BY). The use, distribution or reproduction in other forums is permitted, provided the original author(s) and the copyright owner(s) are credited and that the original publication in this journal is cited, in accordance with accepted academic practice. No use, distribution or reproduction is permitted which does not comply with these terms.
*Correspondence: Andres M. Cardozo Gizzi, andres.cardozo@iucbc.edu.ar