- Department of Microbiology and Cell Biology, Indian Institute of Science, Bangalore, India
Most of the human genome, except for a small region that transcribes protein-coding RNAs, was considered junk. With the advent of RNA sequencing technology, we know that much of the genome codes for RNAs with no protein-coding potential. Long non-coding RNAs (lncRNAs) that form a significant proportion are dynamically expressed and play diverse roles in physiological and pathological processes. Precise spatiotemporal control of their expression is essential to carry out various biochemical reactions inside the cell. Intracellular organelles with membrane-bound compartments are known for creating an independent internal environment for carrying out specific functions. The formation of membrane-free ribonucleoprotein condensates resulting in intracellular compartments is documented in recent times to execute specialized tasks such as DNA replication and repair, chromatin remodeling, transcription, and mRNA splicing. These liquid compartments, called membrane-less organelles (MLOs), are formed by liquid–liquid phase separation (LLPS), selectively partitioning a specific set of macromolecules from others. While RNA binding proteins (RBPs) with low complexity regions (LCRs) appear to play an essential role in this process, the role of RNAs is not well-understood. It appears that short nonspecific RNAs keep the RBPs in a soluble state, while longer RNAs with unique secondary structures promote LLPS formation by specifically binding to RBPs. This review will update the current understanding of phase separation, physio-chemical nature and composition of condensates, regulation of phase separation, the role of lncRNA in the phase separation process, and the relevance to cancer development and progression.
Introduction
Human genome sequencing came up with a massive surprise that only less than 2% of the genome is translated and the remaining ∼98% does not encode a protein, creating loopholes in the central dogma. This non-coding part of the genome was considered “junk or dark matter,” with the exceptions of rRNAs, tRNAs, snRNAs, and snoRNAs (Lander et al., 2001). It was unknown that this junk would belong to a new and larger class of transcripts called lncRNAs (long non-coding RNAs). This class represents transcripts of more than 200 nucleotides in length, lacking coding potential; however, some can encode small peptides (Zhang et al., 2018). LncRNAs have unique biochemical properties such as their interaction with DNA, RNA, and protein and their ability to fold into intricate secondary structures that allow them to interact with multiple RBPs (RNA-binding proteins). After the discoveries of gene-regulatory lncRNAs H19 (Brannan et al., 1990) and Xist (X-inactive specific transcript) (Brown et al., 1991) in the 90s, the number of reports of functionally important lncRNAs has increased continuously. From the nucleus to the cytoplasm, they have been linked to the regulation of several cellular processes such as DNA replication and repair, chromatin remodeling, transcription, mRNA splicing, translation, turnover, and signaling pathways. Over the past 2 decades, extensive research has resolved various queries related to lncRNAs. Moreover, a new concept of LLPS (liquid–liquid phase separation) is coming to light, which is believed to answer countless unanswered questions about the cellular world.
Moreover, a bridge between LLPS and lncRNA has been formed, giving a new meaning to the subcellular localization of lncRNA, where it can perform its functions. It has been shown that phase condensates have a dynamic character, and this property can give lncRNAs the ability to perform their regulatory roles rapidly and transiently. Several functional lncRNAs have been known for years, but what makes them unique if they participate in phase condensation will be discussed in this review. It will also be discussed how condensates aid the lncRNAs in playing a pivotal role in fine-tuning many physiological and pathological activities. The content below will also talk about the connection between LLPS and lncRNA in cancer.
Phase separation
The presence of a lipid bilayer helps in compartmentalization and allows eukaryotic cells to organize biochemical reactions in space and time. But one fundamental question remains: how do cells maintain biomolecular interactions and homeostasis in the cytoplasm and nucleus. One way to coordinate spatiotemporal regulation is to control the localization of specific biomolecules at one place while excluding others, thereby creating a heterogeneous environment. Work from past years has shown the existence of such functional membrane-less compartments in the nucleus and cytoplasm. These compartments include the pericentriolar matrix which plays a role in microtubule nucleation (Woodruff et al., 2017), the nucleolus which produces and assembles ribosomes (Boisvert et al., 2007), Cajal bodies for assembling spliceosome machinery (Gall, 2003), and so on. Even though these compartments were discovered decades ago, the involvement of physio-chemical forces and biological contents driving the formation of these bodies is still unfolding. Research has shown that such compartments form through liquid–liquid phase separation (LLPS) or condensation. Because of diversity in their molecular composition, location, and function, “biomolecular condensates” is considered an umbrella term for these membrane-less cellular compartments that form because of condensation (Table 1, Banani et al., 2017; Shin and Brangwynne, 2017).
Physio-chemical nature of condensates
Numerous studies using in vitro and in vivo approaches have provided a solid foundation for understanding the structural and functional aspects of condensates. LLPS can be described as “a thermodynamically driven, reversible, de-mixing process wherein, above a threshold macromolecule concentration, components separate into coexisting dense and dilute liquid phases with different solute concentrations” (Boeynaems et al., 2018; Lyon et al., 2021).
How does the system allow the formation of de-mixed phases? Let us think about the behavior of the multiple copies of two different proteins (X, Y) in a solution. If the interaction between X and Y is energetically more favorable than that between X and X or Y and Y molecules, then the solution will be homogenously mixed, and the system’s entropy will be maximum. However, suppose X and X molecular interaction is energetically favorable over X and Y interaction, in that case, X will get de-mixed from neighboring Y molecules after reaching a critical threshold concentration. Entropy, which generally favors the homogenous distribution of X and Y molecules, gets counteracted by energetically favorable interactions between X and X molecules. The living system also undergoes similar changes where interactions between different molecules lower the energy, driving them to get phase-separated (Mitrea et al., 2018; Hyman et al., 2014; Bhat et al., 2021).
These interactions between molecules such as X and Y can be of both high and low affinity. Most of the high-affinity interactions provide specificity compared to the low-affinity interactions, but multiple weak, cooperative interactions at a higher concentration can also help in the formation of the condensate. These high- and low-affinity interactions include intramolecular and intermolecular interactions such as π–π, π–cation, cation–anion, dipole–dipole, hydrogen–hydrogen, and hydrophobic forces that influence the spatiotemporal arrangement of the molecules in condensates (Figure 1) (Boeynaems et al., 2018; Dignon et al., 2020).
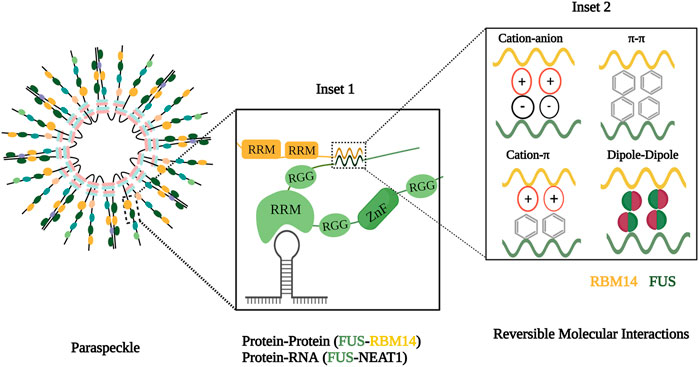
FIGURE 1. Molecular components and forces involved in condensate formation. Inset 1 illustrates the assembly of paraspeckle comprising scaffolds and clients and interactions between these components. Inset 2 depicts molecular forces with in inter and intramolecular interactions with in phase condensate.
Pioneer studies show that P granules and the nucleolus have liquid-like properties typical of phase condensates. These condensates are 1) spherical, due to interfacial tension between two phases; 2) can undergo fission and fusion processes; 3) have diffuse nature, thereby allowing molecular exchange from the nearby environment to support biological function; and 4) can wet other surfaces upon contact (Brangwynne et al., 2009; Brangwynne et al., 2011; Shin and Brangwynne, 2017).
Composition of condensates
In a living cell, forces that favor phase separation are mediated by the cell’s biopolymers, such as proteins and nucleic acids. Condensate components such as protein and RNA can be of two types: scaffolds and clients. Scaffold molecules have higher valency, thereby acting as drivers of phase separation, providing a platform for other proteins. These molecules, abundant in condensates, decide the condensate threshold, and their removal likely prevents condensate assembly. Client molecules are recruited for condensation based on their relative stoichiometry with the scaffold. They perform their function by interacting with scaffolds and other client molecules and are generally dispensable for phase separation. For example, in paraspeckles, a nuclear phase condensate, NEAT1 (nuclear-enriched autosomal transcript 1) lncRNA acts as a scaffold, while the RNA binding protein, FUS (fused in sarcoma), is a client protein (Figure 1) (Banani et al., 2017; Dignon et al., 2020). The central feature of the phase-separated condensate is the presence of multivalent molecules to orchestrate intra- or inter-molecular interactions necessary for phase separation. The multivalency in condensates can be achieved by intrinsically disordered regions (IDRs), RNA-binding domains, and posttranslational modifications (Wiedner and Giudice, 2021).
Protein features for phase separation
Two important features that govern phase separation are the presence of IDR and modular domains. The IDR is a region of amino acids that exhibit low-sequence complexity, are present in a heterogeneous ensemble of conformations, and do not fold into a well-defined three-dimensional structure. They have a higher polar and charged amino acid ratio, including glycine, serine, glutamine, proline, glutamic acid, lysine, and arginine. Even though they have low hydrophobic amino acid content, aromatic amino acids such as tyrosine and phenylalanine are generally interspersed in these regions. These characteristics fulfill the multivalent nature required for phase separation. To phase separate, IDRs can interact with other IDRs of the same protein and/or other proteins. For example, in paraspeckles, RBM14 (RNA binding motif 14) and FUS interact via their IDRs, namely, prion-like domain (Figure 1). These IDRs are present in many proteins such as FUS, YAP (yes-associated protein), and NICD (nephrin intracellular domain) (Banani et al., 2017; Boeynaems et al., 2018; Martin and Holehouse, 2020). Modular domains exhibit discrete secondary and tertiary protein conformations and can interact with other molecules based on their sequence and structure specificity. These domains include RNA recognition motif (RRM), arginine–glycine–glycine motif (RGG), and zinc finger domain. For example, FUS and RBM14 have RRMs, which help them interact with NEAT1 (Figure 1).
RNA features for phase separation
Studies on phase separation reveal the presence and importance of RNA in condensates. RNA can interact with other RNAs and proteins by virtue of its flexible and variable properties. 1) The most fundamental property imparting multivalency is the negative charge of the RNA backbone through which it can mediate electrostatic interactions with other molecules. Also, the single-stranded nature of RNA exposes its bases and phosphate group to interact with multiple positively charged amino acids or molecules. 2) Flexibility also favors attaining multiple confirmations such as G-quadruplexes, hairpin loops, and helix motifs which provide protein or RNA binding sites. RBPs containing RRMs can recognize these structures. 3) Longer lengths of RNAs like long non-coding RNAs (Figure 1) can provide scaffolding property to the condensate as they can offer multiple binding sites. RNA–RNA interactions are important for mediating phase separation, like in the case of paraspeckle and stress granule formation. In addition to promoting phase separation, excessive or high-affinity RNAs can also prevent phase separation. This inhibition can be due to charge repulsion or blocking protein–protein interactions. RNA can regulate condensate assembly by regulating its dynamic nature or acting as a buffer to prevent abnormal aggregations (Roden and Gladfelter, 2021; Su et al., 2021). For example, RNA can prevent the aggregate formation of FUS in the nucleus since it is present in higher concentrations (Maharana et al., 2018).
Regulation of phase separation
Apart from the concentration and multivalency of biopolymers, environmental factors such as pH, temperature, hypoxia, and other stress factors can affect the process of condensation. It can also be regulated by different parameters such as posttranslational modifications (PTMs), the presence of a membrane, molecular chaperones, and other active processes. PTMs affect the physio-chemical nature of amino acids, thereby affecting the strength and multivalency of molecules. Membrane surfaces affect the concentration of molecules by either hampering diffusion or promoting transport or crowding of condensate molecules. Similar to PTMs of proteins, modifications of RNA bases alter essential aspects of RNA functions. These posttranscriptional modifications, such as N6-methyladenosine modification, can also impact condensate assembly. These modifications can influence phase separation in multiple ways. 1) The modifications give the potential for dynamic and adaptive nature to the RNA. 2) It affects the sequence information and has a substantial impact on the structure of RNA molecules. 3) RNA modifications also affect the processing of RNAs. These changes altogether mediate adaptive RNA–RNA and RNA–protein interactions. These factors cumulatively make condensation an adaptive process and only happen upon receiving specific cues from the environment nearby. Thus, the relative stoichiometry of condensate components controls their dwell time within condensate and consequently fine-tunes functions performed by them. (Darino and Schaefer, 2018; Snead and Gladfelter, 2019; Lu et al., 2021; Riback et al., 2017; Roden and Gladfelter, 2021; and Wiedner and Giudice, 2021).
LncRNA, “the anchor” of phase separation
LncRNAs are emerging as important players in the formation of biomolecular condensates. Other RNAs such as mRNAs, rRNAs, and tRNAs have been shown to play important roles in various condensates such as stress granules and P bodies (Protter and Parker, 2016), but decoding the essentiality of lncRNA in phase separation is in its initial stage. LncRNAs do not perform an enzymatic function like a protein nor do they code for a polypeptide like an mRNA, but they have the ability to bring the components together required for a particular biological function, holding them firmly and compartmentalizing them in their contour of action where they can perform their tasks more efficiently. This potential of lncRNAs makes them emerge as anchors of phase separation.
Several features within these anchors have been shown to aid them in organizing a phase condensate. One of these features is their length. LncRNAs being longer than other non-coding RNAs such as miRNA can provide a larger platform for binding RBPs (RNA-binding proteins), as with NORAD lncRNA that has 18 Pumilio binding motifs (Elguindy and Mendell, 2021), providing them better sequestration capacity. They do not contain a specific domain similar to IDRs (Wright and Dyson, 2015) present in proteins forming condensates, but their ability to bind RBPs having IDRs can assist in undergoing phase separation. For example, HNRNPA1 in HSATIII lncRNA nuclear stress bodies has an IDR that mediates protein–protein interaction between numerous HNRNPA1 molecules to cause phase separation (Aly et al., 2019).
The presence of lncRNAs within a condensate makes it less viscous, thus giving a more liquid-like nature compared to the increased protein component, which imparts a solid nature to the condensate (Elbaum-Garfinckle et al., 2015). This protein-to-RNA ratio increase may also prevent condensate formation (Maharana et al., 2018). The fluidity generated in the condensate due to the presence of lncRNAs makes it more dynamic, enhances the rate of molecular exchange, and eases the assembly and disassembly process. Studies on several biomolecular condensates suggest poorly translated mRNAs favor phase separation (Khong et al., 2017). Aptly, lncRNAs, known for their inability to code, hint at their essential role in phase separation.
For any protein or RNA, reaching a threshold concentration for phase separation is necessary (Banani et al., 2017). Therefore, the lncRNAs that form part of condensates are generally upregulated, which may be associated with a cancerous condition such as TNBL (tumor-associated NBL2 transcript) upregulated in colorectal cancer (Dumbovic et al., 2018) or during normal processes such as DIGIT upregulated during endoderm differentiation (Dhaneshvar et al., 2020).
In addition, several reports suggest that inhibiting lncRNAs either by knockdown, antisense oligonucleotides, RNases, RNA pol II inhibitors, or deleting their protein-interacting regions hinders condensate formation, thereby negatively affecting the normal function of the condensate and its components (Pessina et al., 2019; Huo et al., 2020; Lee et al., 2021). This again points toward the importance of lncRNAs in biomolecular condensates and suggests they are the anchors of phase separation. Various other features of lncRNAs may facilitate the compartmentalization process, such as sequence or nucleotide composition, length, secondary structures, and modifications. Some of the important features associated with lncRNAs that form condensates like their subcellular localization, their interacting partners, and the biological functions they assist in are summarized (Table 2).
Some of the lncRNAs reported to be the mainstay in phase separation (Figure 2) are briefly discussed in the sub-sections below.
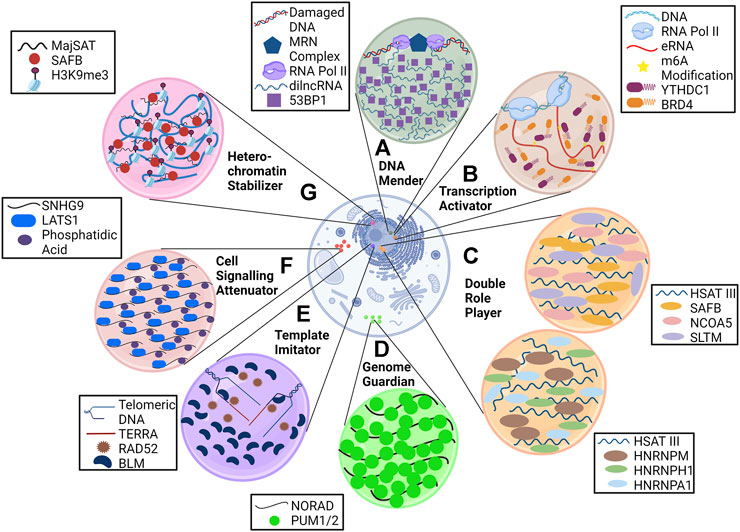
FIGURE 2. LncRNAs are anchors of phase condensates. LncRNAs orchestrate the formation of phase condensates and execute various processes such as (A) DNA repair (dilncRNA), (B) transcription activation (eRNA), (C) pre-mRNA splicing and transcription regulation (HSATIII), (D) genome stabilization (NORAD), (E) alternative lengthening of telomeres (TERRA), and (F) cell signaling (SNHG9).
The heterochromatin stabilizer
MajSAT lncRNA is transcribed from heterochromatin-abundant repetitive elements with which a nuclear matrix (NM)–associated protein, SAFB (scaffold attachment factor B), interacts via its R-/G-rich region. This association forms foci in DAPI-dense regions of the nucleus (heterochromatin region), where SAFB mediates pericentromeric heterochromatin (PCH) stabilization. Downregulation of MajSAT lncRNA with antisense oligonucleotides leads to dissociation of the SAFB condensate, thereby causing weakening of PCH foci as indicated by H3K9me3 staining (Huo et al., 2020).
The transcription activator
Enhancer RNAs (eRNAs), as the name suggests, are long non-coding RNA transcribed from enhancer regions and have a crucial role in transcriptional regulation. They have a heavy deposition of m6A (N6-methyladenosine) modification, as characterized by methylation-inscribed nascent transcript sequencing (MINT-seq) (Lee et al., 2021). These modified eRNAs mark the enhancer regions for the recruitment of m6A reader YTHDC1, forming a phase condensate that facilitates the formation of the BRD4 coactivator condensate, causing transcription activation. Knocking down eRNA or METTL3 and METTL14 (leading to the removal of m6A marks) causes a reduction in YTHDC1 binding to the enhancer, thereby reducing the gene expression. In vitro analysis of YTHDC1/m6A-eRNA suggested that YTHDC1 cannot form the condensate alone.
The cell signaling attenuator
The role of phase condensates in cell signaling is beginning to be deciphered. SNHG9 (small nucleolar RNA host gene 9) lncRNA is now known to regulate the Hippo pathway (Li et al., 2021) by physically interacting with phosphatidic acid (PA) and LATS1 (large tumor suppressor kinase 1) to form a puncta. SNHG9 within this puncta promotes PA-mediated inactivation of LATS1, which causes increased YAP (yes-associated protein) activation and nuclear localization, leading to cancer progression. Knocking down SNHG9 or deleting its PA-interacting region leads to reduced LATS1 puncta formation and sequestration of YAP in the cytoplasm.
The genome guardian
NORAD (non-coding RNA activated by DNA damage) lncRNA is localized in the cytoplasm and sequesters Pumilio proteins (PUM1 and PUM2) in a condensate. Pumilio proteins are RNA-binding proteins that are posttranscriptional repressors and cause translation inhibition and degradation of several mRNAs that include important regulators of mitosis. Negative regulation of PUM proteins by NORAD prevents abnormal mitosis and maintains genomic stability in mammalian cells. NORAD is a perfect example of RNA imparting multivalency in biomolecular condensate as it has 18 PUM response elements (PRE) where PUM proteins bind. A decrease in PREs leads to reduced phase separating ability and requires higher PUM concentration to form condensates (Elguindy and Mendell, 2021). Knockdown of NORAD leads to diffused localization of PUM proteins, significant repression of PUM targets, and higher chromosomal segregation errors.
The template imitator
LncRNAs such as TERRA (telomeric repeat-containing RNA) are known to be a part of ALT-associated pro-myelocytic leukemia bodies (APBs) clustered on the telomeres. Along with TERRA, other DNA replication and repair proteins such as BLM (helicase) and RAD52 (RNA-templated DNA repair) are present in APBs. These bodies mediate telomerase-independent telomere maintenance known as alternative lengthening of telomeres (ALT) in cancer cells, where TERRA acts as a template for telomeric DNA synthesis. Knockdown of associated proteins leads to a reduction in APBs (Min et al., 2019), but the effect of TERRA depletion on it is not known. However, it is known that loss of TERRA results in telomere dysfunction and instability (Chu et al., 2017). Higher expression of TERRA in ALT cells (Episkopou et al., 2014) may have a role in phase separation, but this needs to be further elucidated.
The DNA mender
Double-strand breaks (DSBs) in DNA are sensed by the MRE11-RAD50-NBS1 (MRN) complex, which recruits RNA polymerase II at the damaged sites. This recruitment leads to the synthesis of dilncRNAs (damage-induced long non-coding RNAs) (Sharma et al., 2021), which are further processed into shorter DDR (DNA damage response) RNA. These RNAs form a DNA–RNA hybrid with damaged ends favoring repair by homologous recombination and causing accumulation of DDR factors, such as 53BP1, into foci. Treatment with RNAase A, RNA pol II inhibitors, or antisense oligonucleotides against dilncRNA inhibits this foci formation and even disrupts already formed foci. This, in turn, causes reduced DNA repair efficacy (Pessina et al., 2019).
The double role player
As mentioned earlier, lncRNAs provide binding platforms for various RBPs, but it is not necessary that a particular lncRNA interacts with only a single type of RBP. Various features of lncRNA, especially the repetitive nature, give them the liberty to bind multiple RBPs. One such versatile long non-coding RNA is HSAT III (highly repetitive satellite III) which is now known to provide architecture to two distinct types of nuclear stress bodies under thermal stress. First is nSB-S, in which SAFB associates with HSAT III for the transcription of heat shock proteins. The second is nSB-M, where HNRNPM (heterogeneous nuclear ribonucleoprotein M) interacts with HSAT III and affects pre-mRNA splicing. Knockdown of HSAT III with antisense oligonucleotides causes the disappearance of both types of stress bodies (Aly et al., 2019).
Importance of phase separation in cancer
Over the years, people have been trying to understand the role of cancer driver mutations and their role in cancer progression. Interestingly, it was found that specific mutation that leads to oncogene activation also results in the formation of super-enhancers, which is a type of phase condensates that are formed by a clustered DNA element (Bradner et al., 2017; Cho et al., 2018). Many reports documented the involvement of phase condensation in regulating several functions of cancer cells (Boija et al., 2021). However, further investigation is needed on the mechanistic detail of phase separation involvement in cancer development and progression, which will help improve the therapeutic strategies.
Cancer-associated LncRNAs in phase separation
Long-noncoding RNAs are dysregulated in cancer and have an important role in cancer development and progression. It is challenging to completely understand lncRNA’s function due to its large size, conformational flexibility, and low abundance. However, the specific subcellular localization of lncRNA leads to stoichiometrically measurable effects (Wu et al., 2021). LncRNA has the potential to induce phase separation, but the role of lncRNA on phase separation for cancer development and progression is now at the stage of its infancy.
The NEAT1 lncRNA, a dysregulated lncRNA in cancer, is an architectural component of the paraspeckle nuclear body. In the presence of DNA damage response (DDR), p53 protein is activated, which leads to the NEAT1-mediated paraspeckle formation that attenuates oncogene-dependent activation of p53 (Adriaens et al., 2016). MALAT1 (metastasis-associated lung adenocarcinoma transcript 1) is known to form nuclear speckles comprising pre-mRNA splicing and transcription factors (Hutchinson et al., 2007; Miyagawa et al., 2012). Moreover, MALAT1 has an essential role in metastasis and patient survival in non–small cell lung carcinoma (Ji et al., 2003). Interestingly, another report shows that MALAT1 translocates to a heat-inducible non-coding RNA containing a nuclear body (HiNoCo body) from nuclear speckles in response to heat shock in a heat shock factor 1–independent manner (Onoguchi-Mizutani et al., 2021). They have also shown that the HiNoCo body is a reversible phase condensate and MALAT1 is essential for cell proliferation under heat shock. It has been shown that Hippo signaling pathway is a tumor suppressor pathway by attenuating the YAP nuclear translocation, and most cancer cells are characterized by dysregulation of the Hippo pathway (Pan, 2010; Zhou et al., 2015). However, the lncRNA association with the Hippo signaling pathway is largely unknown. Recently, Li et al., 2006 showed that SNHG9 (small nucleolar RNA host gene 9), a lipid-associated tumor-promoting lncRNA, and phosphatidic acid (PA) facilitate attenuation of LATS1 kinase activity by inducing LLPS, which sequesters LATS1 to promote oncogenic YAP signaling (Li et al., 2021).
Glutamine metabolism is an important source of carbon and nitrogen for several biosynthetic processes. The rate-limiting step of glutaminolysis is the generation of glutamate from glutamine catalyzed by glutaminase-1 (GLS1) (Yoo et al., 2020). Glutamine is the critical nutrient source for many cancer cells (DeBerardinis and Cheng., 2010). The lncRNA GIRGL (glutamine insufficiency regulator of glutaminase lncRNA), which is induced upon glutamine starvation, causes dimerization of CAPRIN1. This complex interacts with GLS1 mRNA, resulting in the formation of phase condensate facilitated by LLPS. In this process, CAPRIN1 suppresses GLS1 mRNA translation which influences cancer cell survival under prolonged glutamine deprivation stress (Wang R. et al., 2021).
Conclusion and future directions
Phase separation provides a framework to understand how an individual cell coordinates specific processes at subcellular levels that are simultaneously robust and adaptive. Like the membranous barrier, phase boundary ensures the controlled localization of biomolecules without the complication of transport through a barrier. The formation of biological condensates is a tightly regulated thermodynamic process that depends on the abundance, sequence, and structure of scaffold and client molecules. Various key mechanisms such as environmental conditions, chaperones, presence of a membrane, and posttranslational modifications can work in concert to affect the physio-chemical property, function, and homeostasis of condensates.
LncRNAs are key molecules in a cell, whether they are part of the phase condensate or not, as they perform various functions in the cell such as gene regulation, including genetic and epigenetic modifications, swimming to different locations within the cell, and having the potential to interact with several biopolymers such as DNA, RNA, proteins, and lipids. The knowledge of LLPS and of lncRNAs being part of phase condensates unfolds whole new possibilities. This efflorescing concept gives a better understanding of how lncRNAs can get concentrated within a particular cell site while interacting with various biopolymers and performing specialized tasks. Further exploration of phase condensation may reveal secrets about the additional functions of known lncRNAs. LLPS can, indeed, be an educator, answering various unsolved questions and providing space to lncRNAs to execute their superpowers. However, a few questions remain to be clarified, like how a few condensates of a particular lncRNA can define its activity at thousands or more locations? Are various effector sites part of a single condensate? While the dynamic nature of phase condensates is well-appreciated, a better understanding of whether this mobility can help simultaneously reach the enormous number of effector sites is needed.
Though phase separation is important for normal cell functioning, some phase-separated condensates are only present in cancer cells, such as FUS-CHOP-mediated phase separation is present in sarcoma (Pérez-Losada et al., 2000). Some condensates can be present in both normal and transformed cells, but the higher expression of proteins involved in phase separation in cancer cells can promote oncogenic signaling. Since phase separation is adaptive, it can respond to oncogenic alterations such as mutations, localization, and degradation of molecules, which regulate the phase separation process either positively or negatively (Gao et al., 2019; Wang B. et al., 2021). Based on the involvement of phase separation in different cancer-related biological processes, altering the expression of critical components through phase separation can be used for targeted therapy. These molecules can include scaffold and client molecules and the environmental conditions involved in phase separation.
The studies on lncRNA-associated liquid–liquid phase separation in cancer are limited. Further investigation is required to improve the understanding of the role of lncRNA for LLPS formation in cancer to improve the therapeutic strategies. In addition, phase condensates may increase the drug efficacy or drug resistance in cancer cells depending on their physicochemical properties to form phase condensates (Klein et al., 2020). It can be hypothesized that phase separation sequesters drugs given to eliminate the cancer cells and lncRNAs may be playing an essential role in it, thereby altering drug efficacy. Where lncRNA is required for the phase condensate, strategies to target lncRNA could be considered. In cases where lncRNA is just a part of phase condensate but is not essential for the architecture itself, methods to inhibit the phase condensate formation may be beneficial. Furthermore, targeting phase separation may form an important therapeutic strategy in the future, especially for undruggable proteins for their intrinsically disordered nature, which is highly abundant in eukaryotes.
Author contributions
KS, BG, NJ, and SJ prepared the manuscript. KS corrected the manuscript as a whole.
Acknowledgments
SJ, BG, and NJ acknowledge DST-NPDF, DST INSPIRE, and DBT for fellowship, respectively. KS acknowledges CEFIPRA DBT, DST, and CSIR (Govt. of India) for research grants. Infrastructure supported by DST, ICMR, DST FIST, DBT-IISc partnership program, and UGC is acknowledged. KS is awarded the J. C. Bose fellowship from DST.
Conflict of interest
The authors declare that the research was conducted in the absence of any commercial or financial relationships that could be construed as a potential conflict of interest.
Publisher’s note
All claims expressed in this article are solely those of the authors and do not necessarily represent those of their affiliated organizations, or those of the publisher, the editors, and the reviewers. Any product that may be evaluated in this article, or claim that may be made by its manufacturer, is not guaranteed or endorsed by the publisher.
Abbreviations
CAPRIN1, Cytoplasmic Activation- And Proliferation-Associated Protein 1; DDR, DNA damage response; GLS1, glutaminase-1; GIRGL, glutamine insufficiency regulator of glutaminase lncRNA; HNRNPA1, heterogeneous nuclear ribonucleoprotein A1; HSATIII, highly repetitive satellite III; LATS1, large tumor suppressor kinase 1; LLPS, Liquid-Liquid Phase Separation; MALAT1, metastasis associated lung adenocarcinoma transcript 1; MINT-seq, methylation-inscribed nascent transcripts sequencing; NICD—Nephrin intracellular domain, NORAD, non-coding RNA activated by DNA damage; NEAT1, Nuclear enriched autosomal transcript 1; FUS, Fused in sarcoma; IDRs, Intrinsically disordered regions; PA, phosphatidic acid; PRE, PUM response elements; PTMs, posttranslational modifications; RBM14, RNA binding motif protein 14; SAFB, scaffold attachment factor B; SNHG9, small nucleolar RNA host gene 9; TERRA, telomeric repeat-containing RNA; TNBL, tumor-associated NBL2 transcript; YAP, Yes-associated protein.
References
Adriaens, C., Standaert, L., Barra, J., Latil, M., Verfaillie, A., Kalev, P., et al. (2016). p53 induces formation of NEAT1 lncRNA-containing paraspeckles that modulate replication stress response and chemosensitivity. Nat Med. 22 (8), 861–868. doi:10.1038/nm.4135
Aguilera-Gomez, A., van Oorschot, M. M., Veenendaal, T., and Rabouille, C. (2016). In vivo vizualisation of mono-ADP-ribosylation by dPARP16 upon amino-acid starvation. eLife 5, e21475. doi:10.7554/eLife.21475
Alenquer, M., Vale-Costa, S., Etibor, T. A., Ferreira, F., Sousa, A. L., Amorim, M. J., et al. (2019). Influenza A virus ribonucleoproteins form liquid organelles at endoplasmic reticulum exit sites. Nat. Commun. 10 (1), 1629. doi:10.1038/s41467-019-09549-4
Altmeyer, M., Neelsen, K. J., Teloni, F., Pozdnyakova, I., Pellegrino, S., Grøfte, M., et al. (2015). Liquid demixing of intrinsically disordered proteins is seeded by poly(ADP-ribose). Nat. Commun. 6, 8088. doi:10.1038/ncomms9088
Aly, M. K., Ninomiya, K., Adachi, S., Natsume, T., and Hirose, T. (2019). Two distinct nuclear stress bodies containing different sets of RNA-binding proteins are formed with HSATIII architectural non-coding RNAs upon thermal stress exposure. Biochem. Biophys. Res. Commun. 516 (2), 419–423. doi:10.1016/j.bbrc.2019.06.061
Audas, T. E., Audas, D. E., Jacob, M. D., Ho, J. D., Khacho, M., Wang, M., et al. (2016). Adaptation to stressors by systemic protein amyloidogenesis. Dev. Cell. 39 (2), 155–168. doi:10.1016/j.devcel.2016.09.002
Banani, S. F., Lee, H. O., Hyman, A. A., and Rosen, M. K. (2017). Biomolecular condensates: Organizers of cellular biochemistry. Nat. Rev. Mol. Cell. Biol. 18 (5), 285–298. doi:10.1038/nrm.2017.7
Banjade, S., and Rosen, M. K. (2014). Phase transitions of multivalent proteins can promote clustering of membrane receptors. eLife 3, e04123. doi:10.7554/eLife.04123
Beutel, O., Maraspini, R., Pombo-García, K., Martin-Lemaitre, C., and Honigmann, A. (2019). Phase separation of zonula occludens proteins drives formation of tight junctions. Cell. 179 (4), 923–936. e11. doi:10.1016/j.cell.2019.10.011
Bhat, P., Honson, D., and Guttman, M. (2021). Nuclear compartmentalization as a mechanism of quantitative control of gene expression. Nat. Rev. Mol. Cell. Biol. 22 (10), 653–670. doi:10.1038/s41580-021-00387-1
Biamonti, G., and Vourc’h, C. (2010). Nuclear stress bodies. Cold Spring Harb. Perspect. Biol. 2 (6), a000695. doi:10.1101/cshperspect.a000695
Boeynaems, S., Alberti, S., Fawzi, N. L., Mittag, T., Polymenidou, M., Rousseau, F., et al. (2018). Protein phase separation: A new phase in cell biology. Trends Cell. Biol. 28 (6), 420–435. doi:10.1016/j.tcb.2018.02.004
Boija, A., Klein, I. A., and Young, R. A. (2021). Biomolecular condensates and cancer. Cancer Cell. 39 (2), 174–192. doi:10.1016/j.ccell.2020.12.003
Boisvert, F. M., van Koningsbruggen, S., Navascués, J., and Lamond, A. I. (2007). The multifunctional nucleolus. Nat. Rev. Mol. Cell. Biol. 8 (7), 574–585. doi:10.1038/nrm2184
Boke, E., Ruer, M., Wühr, M., Coughlin, M., Lemaitre, R., Gygi, S. P., et al. (2016). Amyloid-like self-assembly of a cellular compartment. Cell. 166 (3), 637–650. doi:10.1016/j.cell.2016.06.051
Bradner, J. E., Hnisz, D., and Young, R. A. (2017). Transcriptional addiction in cancer. Cell. 168 (4), 629–643. doi:10.1016/j.cell.2016.12.013
Brangwynne, C. P., Eckmann, C. R., Courson, D. S., Rybarska, A., Hoege, C., Gharakhani, J., et al. (2009). Germline P granules are liquid droplets that localize by controlled dissolution/condensation. Sci. (New York, N.Y.) 324 (5935), 1729–1732. doi:10.1126/science.1172046
Brangwynne, C. P., Mitchison, T. J., and Hyman, A. A. (2011). Active liquid-like behavior of nucleoli determines their size and shape in Xenopus laevis oocytes. Proc. Natl. Acad. Sci. U. S. A. 108 (11), 4334–4339. doi:10.1073/pnas.1017150108
Brannan, C. I., Dees, E. C., Ingram, R. S., and Tilghman, S. M. (1990). The product of the H19 gene may function as an RNA. Mol. Cell. Biol. 10 (1), 28–36. doi:10.1128/mcb.10.1.28
Brown, C. J., Ballabio, A., Rupert, J. L., Lafreniere, R. G., Grompe, M., Tonlorenzi, R., et al. (1991). A gene from the region of the human X inactivation centre is expressed exclusively from the inactive X chromosome. Nature 349 (6304), 38–44. doi:10.1038/349038a0
Cauchi, R. J. (2011). Gem formation upon constitutive Gemin3 overexpression in Drosophila. Cell. Biol. Int. 35 (12), 1233–1238. doi:10.1042/CBI20110147
Cho, W. K., Spille, J. H., Hecht, M., Lee, C., Li, C., Grube, V., et al. (2018). Mediator and RNA polymerase II clusters associate in transcription-dependent condensates. Sci. (New York, N.Y.) 361 (6400), 412–415. doi:10.1126/science.aar4199
Chu, H. P., Cifuentes-Rojas, C., Kesner, B., Aeby, E., Lee, H. G., Wei, C., et al. (2017). TERRA RNA antagonizes ATRX and protects telomeres. Cell. 170 (1), 86–101. e16. doi:10.1016/j.cell.2017.06.017
Clemson, C. M., Hutchinson, J. N., Sara, S. A., Ensminger, A. W., Fox, A. H., Chess, A., et al. (2009). An architectural role for a nuclear non-coding RNA: NEAT1 RNA is essential for the structure of paraspeckles. Mol. Cell. 33 (6), 717–726. doi:10.1016/j.molcel.2009.01.026
Daneshvar, K., Ardehali, M. B., Klein, I. A., Hsieh, F. K., Kratkiewicz, A. J., Mahpour, A., et al. (2020). lncRNA DIGIT and BRD3 protein form phase-separated condensates to regulate endoderm differentiation. Nat. Cell. Biol. 22 (10), 1211–1222. doi:10.1038/s41556-020-0572-2
DeBerardinis, R. J., and Cheng, T. (2010). Q’s next: The diverse functions of glutamine in metabolism, cell biology and cancer. Oncogene 29 (3), 313–324. doi:10.1038/onc.2009.358
Decker, C. J., and Parker, R. (2012). P-Bodies and stress granules: Possible roles in the control of translation and mRNA degradation. Cold Spring Harb. Perspect. Biol. 4 (9), a012286. doi:10.1101/cshperspect.a012286
Dignon, G. L., Best, R. B., and Mittal, J. (2020). Biomolecular phase separation: From molecular driving forces to macroscopic properties. Annu. Rev. Phys. Chem. 71, 53–75. doi:10.1146/annurev-physchem-071819-113553
Drino, A., and Schaefer, M. R. (2018). RNAs, phase separation, and membrane-less organelles: Are post-transcriptional modifications modulating organelle dynamics? Bioessays 40 (12), e1800085. doi:10.1002/bies.201800085
Du, M., and Chen, Z. J. (2018). DNA-induced liquid phase condensation of cGAS activates innate immune signaling. Sci. (New York, N.Y.) 361 (6403), 704–709. doi:10.1126/science.aat1022
Dumbovic, G., Biayna, J., Banús, J., Samuelsson, J., Roth, A., Diederichs, S., et al. (2018). A novel long non-coding RNA from NBL2 pericentromeric macrosatellite forms a perinucleolar aggregate structure in colon cancer. Nucleic Acids Res. 46 (11), 5504–5524. doi:10.1093/nar/gky263
Duronio, R. J., and Marzluff, W. F. (2017). Coordinating cell cycle-regulated histone gene expression through assembly and function of the Histone Locus Body. RNA Biol. 14 (6), 726–738. doi:10.1080/15476286.2016.1265198
Elbaum-Garfinkle, S., Kim, Y., Szczepaniak, K., Chen, C. C., Eckmann, C. R., Myong, S., et al. (2015). The disordered P granule protein LAF-1 drives phase separation into droplets with tunable viscosity and dynamics. Proc. Natl. Acad. Sci. U. S. A. 112 (23), 7189–7194. doi:10.1073/pnas.1504822112
Elguindy, M. M., and Mendell, J. T. (2021). NORAD-induced Pumilio phase separation is required for genome stability. Nature 595 (7866), 303–308. doi:10.1038/s41586-021-03633-w
Episkopou, H., Draskovic, I., Van Beneden, A., Tilman, G., Mattiussi, M., Gobin, M., et al. (2014). Alternative Lengthening of Telomeres is characterized by reduced compaction of telomeric chromatin. Nucleic Acids Res. 42 (7), 4391–4405. doi:10.1093/nar/gku114
Fox, A. H., and Lamond, A. I. (2010). Paraspeckles. Cold Spring Harb. Perspect. Biol. 2 (7), a000687. doi:10.1101/cshperspect.a000687
Gall, J. G. (2003). The centennial of the Cajal body. Nat. Rev. Mol. Cell. Biol. 4 (12), 975–980. doi:10.1038/nrm1262
Gao, Y., Pei, G., Li, D., Li, R., Shao, Y., Zhang, Q. C., et al. (2019). Multivalent m6A motifs promote phase separation of YTHDF proteins. Cell Res. 29 (9), 767–769. doi:10.1038/s41422-019-0210-3
Hall, L. L., Byron, M., Carone, D. M., Whitfield, T. W., Pouliot, G. P., Fischer, A., et al. (2017). Demethylated HSATII DNA and HSATII RNA foci sequester PRC1 and MeCP2 into cancer-specific nuclear bodies. Cell. Rep. 18 (12), 2943–2956. doi:10.1016/j.celrep.2017.02.072
Handwerger, K. E., Cordero, J. A., and Gall, J. G. (2005). Cajal bodies, nucleoli, and speckles in the Xenopus oocyte nucleus have a low-density, sponge-like structure. Mol. Biol. Cell 16 (1), 202–211. doi:10.1091/mbc.e04-08-0742
Harrigan, J. A., Belotserkovskaya, R., Coates, J., Dimitrova, D. S., Polo, S. E., Bradshaw, C. R., et al. (2011). Replication stress induces 53BP1-containing OPT domains in G1 cells. J. Cell. Biol. 193 (1), 97–108. doi:10.1083/jcb.201011083
Heinrich, B. S., Maliga, Z., Stein, D. A., Hyman, A. A., and Whelan, S. (2018). Phase transitions drive the formation of vesicular stomatitis virus replication compartments. mBio 9 (5), e02290–17. doi:10.1128/mBio.02290-17
Hennig, S., Kong, G., Mannen, T., Sadowska, A., Kobelke, S., Blythe, A., et al. (2015). Prion-like domains in RNA binding proteins are essential for building subnuclear paraspeckles. J. Cell. Biol. 210 (4), 529–539. doi:10.1083/jcb.201504117
Hirose, T., Yamazaki, T., and Nakagawa, S. (2019). Molecular anatomy of the architectural NEAT1 non-coding RNA: The domains, interactors, and biogenesis pathway required to build phase-separated nuclear paraspeckles. Wiley Interdiscip. Rev. RNA 10 (6), e1545. doi:10.1002/wrna.1545
Huo, X., Ji, L., Zhang, Y., Lv, P., Cao, X., Wang, Q., et al. (2020). The nuclear matrix protein SAFB cooperates with major satellite RNAs to stabilize heterochromatin architecture partially through phase separation. Mol. Cell. 77 (2), 368–383. e7. doi:10.1016/j.molcel.2019.10.001
Hutchinson, J. N., Ensminger, A. W., Clemson, C. M., Lynch, C. R., Lawrence, J. B., Chess, A., et al. (2007). A screen for nuclear transcripts identifies two linked non-coding RNAs associated with SC35 splicing domains. BMC genomics 8, 39. doi:10.1186/1471-2164-8-39
Hyman, A. A., Weber, C. A., and Jülicher, F. (2014). Liquid-liquid phase separation in biology. Annu. Rev. Cell. Dev. Biol. 30, 39–58. doi:10.1146/annurev-cellbio-100913-013325
Ji, P., Diederichs, S., Wang, W., Böing, S., Metzger, R., Schneider, P. M., et al. (2003). MALAT-1, a novel noncoding RNA, and thymosin beta4 predict metastasis and survival in early-stage non-small cell lung cancer. Oncogene 22 (39), 8031–8041. doi:10.1038/sj.onc.1206928
Jones, N., Blasutig, I. M., Eremina, V., Ruston, J. M., Bladt, F., Li, H., et al. (2006). Nck adaptor proteins link nephrin to the actin cytoskeleton of kidney podocytes. Nature 440 (7085), 818–823. doi:10.1038/nature04662
Khong, A., Matheny, T., Jain, S., Mitchell, S. F., Wheeler, J. R., Parker, R., et al. (2017). The stress granule transcriptome reveals principles of mRNA accumulation in stress granules. Mol. Cell. 68 (4), 808–820. e5. doi:10.1016/j.molcel.2017.10.015
Klein, I. A., Boija, A., Afeyan, L. K., Hawken, S. W., Fan, M., Dall’Agnese, A., et al. (2020). Partitioning of cancer therapeutics in nuclear condensates. Sci. (New York, N.Y.) 368 (6497), 1386–1392. doi:10.1126/science.aaz4427
Lallemand-Breitenbach, V., and de Thé, H. (2010). PML nuclear bodies. Cold Spring Harb. Perspect. Biol. 2 (5), a000661. doi:10.1101/cshperspect.a000661
Lander, E. S., Linton, L. M., Birren, B., Nusbaum, C., Zody, M. C., Baldwin, J., et al. (2001). international human genome sequencing ConsortiumInitial sequencing and analysis of the human genome. Nature 409 (6822), 860–921. doi:10.1038/35057062
Lee, J. H., Wang, R., Xiong, F., Krakowiak, J., Liao, Z., Nguyen, P. T., et al. (2021). Enhancer RNA m6A methylation facilitates transcriptional condensate formation and gene activation. Mol. Cell. 81 (16), 3368–3385.e9. e9. doi:10.1016/j.molcel.2021.07.024
Li, L., Roy, K., Katyal, S., Sun, X., Bléoo, S., Godbout, R., et al. (2006). Dynamic nature of cleavage bodies and their spatial relationship to DDX1 bodies, Cajal bodies, and gems. Mol. Biol. Cell. 17 (3), 1126–1140. doi:10.1091/mbc.e05-08-0768
Li, R. H., Tian, T., Ge, Q. W., He, X. Y., Shi, C. Y., Li, J. H., et al. (2021). A phosphatidic acid-binding lncRNA SNHG9 facilitates LATS1 liquid-liquid phase separation to promote oncogenic YAP signaling. Cell. Res. 31 (10), 1088–1105. doi:10.1038/s41422-021-00530-9
Liu, J. L., and Gall, J. G. (2007). U bodies are cytoplasmic structures that contain uridine-rich small nuclear ribonucleoproteins and associate with P bodies. Proc. Natl. Acad. Sci. U. S. A. 104 (28), 11655–11659. doi:10.1073/pnas.0704977104
Lu, J., Qian, J., Xu, Z., Yin, S., Zhou, L., Zheng, S., et al. (2021). Emerging roles of liquid-liquid phase separation in cancer: From protein aggregation to immune-associated signaling. Front. Cell. Dev. Biol. 9, 631486. doi:10.3389/fcell.2021.631486
Lyon, A. S., Peeples, W. B., and Rosen, M. K. (2021). A framework for understanding the functions of biomolecular condensates across scales. Nat. Rev. Mol. Cell. Biol. 22 (3), 215–235. doi:10.1038/s41580-020-00303-z
Ma, W., and Mayr, C. (2018). A membraneless organelle associated with the endoplasmic reticulum enables 3’utr-mediated protein-protein interactions. Cell. 175 (6), 1492–1506. e19. doi:10.1016/j.cell.2018.10.007
Maharana, S., Wang, J., Papadopoulos, D. K., Richter, D., Pozniakovsky, A., Poser, I., et al. (2018). RNA buffers the phase separation behavior of prion-like RNA binding proteins. Sci. (New York, N.Y.) 360 (6391), 918–921. doi:10.1126/science.aar7366
Mahen, R., and Venkitaraman, A. R. (2012). Pattern formation in centrosome assembly. Curr. Opin. Cell. Biol. 24 (1), 14–23. doi:10.1016/j.ceb.2011.12.012
Marnik, E. A., and Updike, D. L. (2019). Membraneless organelles: P granules in Caenorhabditis elegans. Traffic (Copenhagen, Den. 20 (6), 373–379. doi:10.1111/tra.12644
Martin, E. W., and Holehouse, A. S. (2020). Intrinsically disordered protein regions and phase separation: Sequence determinants of assembly or lack thereof. Emerg. Top. Life Sci. 4 (3), 307–329. doi:10.1042/ETLS20190164
Min, J., Wright, W. E., and Shay, J. W. (2019). Clustered telomeres in phase-separated nuclear condensates engage mitotic DNA synthesis through BLM and RAD52. Genes Dev. 33 (13-14), 814–827. doi:10.1101/gad.324905.119
Mitrea, D. M., Cika, J. A., Stanley, C. B., Nourse, A., Onuchic, P. L., Banerjee, P. R., et al. (2018). Self-interaction of NPM1 modulates multiple mechanisms of liquid-liquid phase separation. Nat. Comm. 9 (1), 842. doi:10.1038/s41467-018-03255-3
Miyagawa, R., Tano, K., Mizuno, R., Nakamura, Y., Ijiri, K., Rakwal, R., et al. (2012). Identification of cis- and trans-acting factors involved in the localization of MALAT-1 non-coding RNA to nuclear speckles. RNA (New York, N.Y.) 18 (4), 738–751. doi:10.1261/rna.028639.111
Morimoto, M., and Boerkoel, C. F. (2013). The role of nuclear bodies in gene expression and disease. Biology (Basel) 2 (3), 976–1033. doi:10.3390/biology2030976
Niemelä, E., Desai, D., Lundsten, E., Rosenholm, J. M., Kankaanpää, P., Eriksson, J. E., et al. (2019). Quantitative bioimage analytics enables measurement of targeted cellular stress response induced by celastrol-loaded nanoparticles. Cell. Stress Chaperones 24 (4), 735–748. doi:10.1007/s12192-019-00999-9
Nizami, Z., Deryusheva, S., and Gall, J. G. (2010). The Cajal body and histone locus body. Cold Spring Harb. Perspect. Biol. 2 (7), a000653. doi:10.1101/cshperspect.a000653
Norton, J. T., and Huang, S. (2013). The perinucleolar compartment: RNA metabolism and cancer. Cancer Treat. Res. 158, 139–152. doi:10.1007/978-3-642-31659-3_6
Onoguchi-Mizutani, R., Kirikae, Y., Ogura, Y., Gutschner, T., Diederichs, S., Akimitsu, N., et al. (2021). Identification of a heat-inducible novel nuclear body containing the long non-coding RNA MALAT1. J. Cell. Sci. 134 (10), jcs253559. doi:10.1242/jcs.253559
Pan, D. (2010). The hippo signaling pathway in development and Cancer. Dev. Cell. 19 (4), 491–505. doi:10.1016/j.devcel.2010.09.011
Pérez-Losada, J., Sánchez-Martín, M., Rodríguez-García, M. A., Pérez-Mancera, P. A., Pintado, B., Flores, T., et al. (2000). Liposarcoma initiated by FUS/TLS-CHOP: the FUS/TLS domain plays a critical role in the pathogenesis of liposarcoma. Oncogene 19 (52), 6015–6022. doi:10.1038/sj.onc.1204018
Pandya-Jones, A., Zheng, S., Guttman, M., Black, D. L., Plath, K., Damianov, A., et al. (2020). A protein assembly mediates Xist localization and gene silencing. Nature 587 (7832), 145–151.doi:10.1038/s41586-020-2703-0
Pessina, F., Giavazzi, F., Yin, Y., Gioia, U., Vitelli, V., Galbiati, A., et al. (2019). Functional transcription promoters at DNA double-strand breaks mediate RNA-driven phase separation of damage-response factors. Nat. Cell. Biol. 21 (10), 1286–1299. doi:10.1038/s41556-019-0392-4
Pirrotta, V., and Li, H. B. (2012). A view of nuclear Polycomb bodies. Curr. Opin. Genet. Dev. 22 (2), 101–109. doi:10.1016/j.gde.2011.11.004
Pollock, C., and Huang, S. (2010). The perinucleolar compartment. Cold Spring Harb. Perspect. Biol. 2 (2), a000679. doi:10.1101/cshperspect.a000679
Prasanth, K. V., Rajendra, T. K., Lal, A. K., and Lakhotia, S. C. (2000). Omega speckles - a novel class of nuclear speckles containing hnRNPs associated with non-coding hsr-omega RNA in Drosophila. J. Cell. Sci. 113 (19), 3485–3497. doi:10.1242/jcs.113.19.3485
Protter, D., and Parker, R. (2016). Principles and properties of stress granules. Trends Cell. Biol. 26 (9), 668–679. doi:10.1016/j.tcb.2016.05.004
Riback, J. A., Katanski, C. D., Kear-Scott, J. L., Pilipenko, E. V., Rojek, A. E., Sosnick, T. R., et al. (2017). Stress-triggered phase separation is an adaptive, evolutionarily tuned response. Cell 168 (6), 1068–1040.e19. doi:10.1016/j.cell.2017.02.027
Roden, C., and Gladfelter, A. S. (2021). RNA contributions to the form and function of biomolecular condensates. Nat. Rev. Mol. Cell. Biol. 22 (3), 183–195. doi:10.1038/s41580-020-0264-6
Schmidt, H. B., and Görlich, D. (2016). Transport selectivity of nuclear pores, phase separation, and membraneless organelles. Trends biochem. Sci. 41 (1), 46–61. doi:10.1016/j.tibs.2015.11.001
Sharma, S., Anand, R., Zhang, X., Francia, S., Michelini, F., Galbiati, A., et al. (2021). MRE11-RAD50-NBS1 complex is sufficient to promote transcription by RNA polymerase II at double-strand breaks by melting DNA ends. Cell. Rep. 34 (1), 108565. doi:10.1016/j.celrep.2020.108565
Sheng, M., and Kim, E. (2011). The postsynaptic organization of synapses. Cold Spring Harb. Perspect. Biol. 3 (12), a005678. doi:10.1101/cshperspect.a005678
Shichino, Y., Yamashita, A., and Yamamoto, M. (2014). Meiotic long non-coding meiRNA accumulates as a dot at its genetic locus facilitated by Mmi1 and plays as a decoy to lure Mmi1. Open Biol. 4 (6), 140022. doi:10.1098/rsob.140022
Shin, Y., and Brangwynne, C. P. (2017). Liquid phase condensation in cell physiology and disease. Sci. (New York, N.Y.) 357 (6357), eaaf4382. doi:10.1126/science.aaf4382
Snead, W. T., and Gladfelter, A. S. (2019). The control centers of biomolecular phase separation: How membrane surfaces, PTMs, and active processes regulate condensation. Mol. Cell. 76 (2), 295–305. doi:10.1016/j.molcel.2019.09.016
So, C., Cheng, S., and Schuh, M. (2021). Phase separation during germline development. Trends Cell. Biol. 31 (4), 254–268. doi:10.1016/j.tcb.2020.12.004
Spector, D. L., and Lamond, A. I. (2011). Nuclear speckles. Cold Spring Harb. Perspect. Biol. 3 (2), a000646. doi:10.1101/cshperspect.a000646
Spector, D. L. (2001). Nuclear domains. J. Cell. Sci. 114 (16), 2891–2893. doi:10.1242/jcs.114.16.2891
Su, X., Ditlev, J. A., Hui, E., Xing, W., Banjade, S., Okrut, J., et al. (2016). Phase separation of signaling molecules promotes T cell receptor signal transduction. Sci. (New York, N.Y.) 352 (6285), 595–599. doi:10.1126/science.aad9964
Su, Y., Maimaitiyiming, Y., Wang, L., Cheng, X., and Hsu, C. H. (2021). Modulation of phase separation by RNA: A glimpse on N6-methyladenosine modification. Front. Cell. Dev. Biol. 9, 786454. doi:10.3389/fcell.2021.786454
Tsalikis, J., Tattoli, I., Ling, A., Sorbara, M. T., Croitoru, D. O., Philpott, D. J., et al. (2015). Intracellular bacterial pathogens trigger the formation of U small nuclear RNA bodies (U bodies) through metabolic stress induction. J. Biol. Chem. 290 (34), 20904–20918. doi:10.1074/jbc.M115.659466
van Leeuwen, W., and Rabouille, C. (2019). Cellular stress leads to the formation of membraneless stress assemblies in eukaryotic cells. Traffic (Copenhagen, Den. 20 (9), 623–638. doi:10.1111/tra.12669
Wang, B., Zhang, L., Dai, T., Qin, Z., Lu, H., Zhang, L., et al. (2021). Liquid-liquid phase separation in human health and diseases. Signal Transduct. Target Ther. 6 (1), 290. doi:10.1038/s41392-021-00678-1
Wang, M., Tao, X., Jacob, M. D., Bennett, C. A., Ho, J., Gonzalgo, M. L., et al. (2018). Stress-induced low complexity RNA activates physiological amyloidogenesis. Cell. Rep. 24 (7), 1713–1721. e4. doi:10.1016/j.celrep.2018.07.040
Wang, R., Cao, L., Thorne, R. F., Zhang, X. D., Li, J., Shao, F., et al. (2021). LncRNA GIRGL drives CAPRIN1-mediated phase separation to suppress glutaminase-1 translation under glutamine deprivation. Sci. Adv. 7 (13), eabe5708. doi:10.1126/sciadv.abe5708
Wiedner, H. J., and Giudice, J. (2021). It’s not just a phase: Function and characteristics of RNA-binding proteins in phase separation. Nat. Struct. Mol. Biol. 28 (6), 465–473. doi:10.1038/s41594-021-00601-w
Woodruff, J. B., Ferreira Gomes, B., Widlund, P. O., Mahamid, J., Honigmann, A., Hyman, A. A., et al. (2017). The centrosome is a selective condensate that nucleates microtubules by concentrating tubulin. Cell. 169 (6), 1066–1077. e10. doi:10.1016/j.cell.2017.05.028
Wright, P. E., and Dyson, H. J. (2015). Intrinsically disordered proteins in cellular signalling and regulation. Nat. Rev. Mol. Cell. Biol. 16 (1), 18–29. doi:10.1038/nrm3920
Wu, M., Yang, L. Z., and Chen, L. L. (2021). Long non-coding RNA and protein abundance in lncRNPs. RNA (New York, N.Y.) 27 (12), 1427–1440. doi:10.1261/rna.078971.121
Yap, K., Mukhina, S., Zhang, G., Tan, J., Ong, H. S., Makeyev, E. V., et al. (2018). A short tandem repeat-enriched RNA assembles a nuclear compartment to control alternative splicing and promote cell survival. Mol. Cell. 72 (3), 525–540. e13. doi:10.1016/j.molcel.2018.08.041
Yoo, H. C., Yu, Y. C., Sung, Y., and Han, J. M. (2020). Glutamine reliance in cell metabolism. Exp. Mol. Med. 52 (9), 1496–1516. doi:10.1038/s12276-020-00504-8
Zacharogianni, M., Aguilera-Gomez, A., Veenendaal, T., Smout, J., and Rabouille, C. (2014). A stress assembly that confers cell viability by preserving ERES components during amino-acid starvation. eLife 3, e04132. doi:10.7554/eLife.04132
Zeng, M., Chen, X., Guan, D., Xu, J., Wu, H., Tong, P., et al. (2018). Reconstituted postsynaptic density as a molecular platform for understanding synapse formation and plasticity. Cell. 174 (5), 1172–1187. e16. doi:10.1016/j.cell.2018.06.047
Zeng, M., Shang, Y., Araki, Y., Guo, T., Huganir, R. L., Zhang, M., et al. (2016). Phase transition in postsynaptic densities underlies formation of synaptic complexes and synaptic plasticity. Cell. 166 (5), 1163–1175. e12. doi:10.1016/j.cell.2016.07.008
Zhang, M., Zhao, K., Xu, X., Yang, Y., Yan, S., Wei, P., et al. (2018). A peptide encoded by circular form of LINC-PINT suppresses oncogenic transcriptional elongation in glioblastoma. Nat. Commun. 9 (1), 4475. doi:10.1038/s41467-018-06862-2
Keywords: lncRNA, phase separation, biomolecular condensates, multivalency, intrinsically disordered region, N6-methylAdenosine (m6A), RNA granules, RNA binding proteins
Citation: Somasundaram K, Gupta B, Jain N and Jana S (2022) LncRNAs divide and rule: The master regulators of phase separation. Front. Genet. 13:930792. doi: 10.3389/fgene.2022.930792
Received: 28 April 2022; Accepted: 07 July 2022;
Published: 10 August 2022.
Edited by:
Prabhu Mathiyalagan, New York University, United StatesReviewed by:
Valeria Specchia, University of Salento, ItalyRobert Kenichi Maeda, Université de Genève, Switzerland
Copyright © 2022 Somasundaram, Gupta, Jain and Jana. This is an open-access article distributed under the terms of the Creative Commons Attribution License (CC BY). The use, distribution or reproduction in other forums is permitted, provided the original author(s) and the copyright owner(s) are credited and that the original publication in this journal is cited, in accordance with accepted academic practice. No use, distribution or reproduction is permitted which does not comply with these terms.
*Correspondence: Kumaravel Somasundaram, skumar1@iisc.ac.in
†These authors have contributed equally to this work