- Brain and Cognitive Sciences Department and McGovern Institute for Brain Research, Massachusetts Institute of Technology, Cambridge, MA, USA
The striatum is composed principally of GABAergic, medium spiny striatal projection neurons (MSNs) that can be categorized based on their gene expression, electrophysiological profiles, and input–output circuits. Major subdivisions of MSN populations include (1) those in ventromedial and dorsolateral striatal regions, (2) those giving rise to the direct and indirect pathways, and (3) those that lie in the striosome and matrix compartments. The first two classificatory schemes have enabled advances in understanding of how basal ganglia circuits contribute to disease. However, despite the large number of molecules that are differentially expressed in the striosomes or the extra-striosomal matrix, and the evidence that these compartments have different input–output connections, our understanding of how this compartmentalization contributes to striatal function is still not clear. A broad view is that the matrix contains the direct and indirect pathway MSNs that form parts of sensorimotor and associative circuits, whereas striosomes contain MSNs that receive input from parts of limbic cortex and project directly or indirectly to the dopamine-containing neurons of the substantia nigra, pars compacta. Striosomes are widely distributed within the striatum and are thought to exert global, as well as local, influences on striatal processing by exchanging information with the surrounding matrix, including through interneurons that send processes into both compartments. It has been suggested that striosomes exert and maintain limbic control over behaviors driven by surrounding sensorimotor and associative parts of the striatal matrix. Consistent with this possibility, imbalances between striosome and matrix functions have been reported in relation to neurological disorders, including Huntington’s disease, L-DOPA-induced dyskinesias, dystonia, and drug addiction. Here, we consider how signaling imbalances between the striosomes and matrix might relate to symptomatology in these disorders.
Introduction
The striatum is the primary input side of the basal ganglia, a set of subcortical brain regions that are important for the control of voluntary movement and our ability to learn patterns of behavior that maximize reward. The striatum integrates incoming information from all regions of the cerebral cortex in order to build motor patterns based on the current environment and desires as well as on past experience (Graybiel, 2008; Figure 1A). Distinct, largely parallel cortico-basal ganglia-thalamo-cortico loops have been proposed to control different aspects of behavior (Alexander et al., 1986) and different stages of behavioral learning (Yin et al., 2008). There are marked changes in activity across the striatum as animals undergo the transition from early-stage, goal-directed motor learning to habitual, goal-independent behaviors (Thorn et al., 2010). Lesion studies suggest that the ventromedial striatum drives the early stages of motor learning and that the dorsolateral striatum is more important for the development of habitual behaviors (Yin et al., 2004; Atallah et al., 2007). Considering its key function in reward-based learning, it is no surprise that the striatum is implicated in a wide variety of behaviors. This breadth of function is strikingly exhibited in the range of clinical disorders for which abnormal functioning of the striatum has been found, including not only classical motor disorders: Parkinson’s disease (PD), Huntington’s disease (HD), and dystonia, but also habit formation and drug addiction, impulsivity and attention-deficit hyperactivity disorder (ADHD), compulsivity and obsessive compulsive disorder, emotional control, and depression (for review, see Graybiel and Mink, 2009).
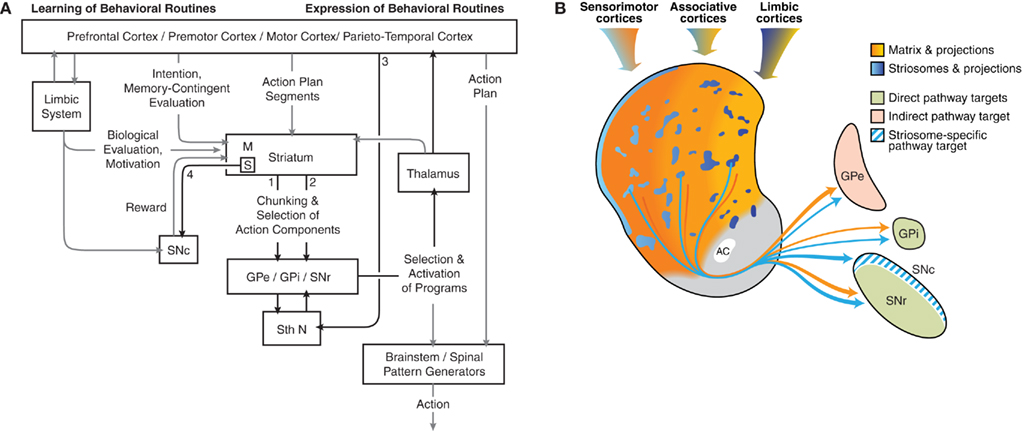
Figure 1. Neuroanatomical connections of the basal ganglia. (A) Schematic diagram of major basal ganglia circuits with highly schematized indications of component functions. The striatum with its matrix (M) and striosomal (S) compartments is centered in the diagram. Four major pathways are emphasized: the direct (1) and indirect (2) pathways, the hyperdirect pathway (3), and the striosomal pathway (4). Reprinted with permission from The Cognitive Neurosciences, 4th Edition (Graybiel and Mink, 2009). (B) Model of the direct, indirect, and striosome-specific striatal projection pathways from the dorsal striatum. The diagram is based on a cross-section through the striatum of an adult rat, immunostained for CalDAG-GEFII. Striosomes are shown in blue, and the extra-striosomal matrix in orange. Shading of the striatum from medial (right) to lateral (left) schematically indicates limbic, associative, and sensorimotor striatal domains. Arrows flowing into the striatum are colored to represent the relative abundance of inputs from limbic cortical regions to striosomes and from sensorimotor and associative regions to the matrix. Arrows exiting the striatum represent GABAergic efferent connections from the medium spiny projection neurons (MSNs) in the striosome and matrix compartments to their respective downstream target nuclei. The nucleus accumbens is shown in gray. GPe, external segment of the globus pallidus; GPi, internal segment of the globus pallidus (entopeduncular nucleus, in rodents); SNr, substantia nigra pars reticulata; SNc, dopamine-containing substantia nigra, pars compacta; AC, anterior commissure; STN, subthalamic nucleus.
Incoming cortical activity is modulated in the striatum by neurochemicals that reflect mood, motivation, and expectation. The striatum is heavily enriched in a number of neurochemical receptor systems including those for dopamine, acetylcholine, endocannabinoids, and endogenous opiates (Graybiel, 1990). Striatal circuits for appropriate behaviors can thus be engaged according to the neurochemical receptors that they express. For example, medium spiny striatal projection neurons (MSNs) that are part of a circuit that promotes movement express the Drd1 (D1) dopamine receptor, which boosts MSN cell excitability. MSNs that are part of a circuit that suppresses movement express the Drd2 (D2) dopamine receptor, which diminishes excitability of this MSN cell-type. Thus, dopamine has a pro-movement effect by simultaneously promoting and disinhibiting movement through the so-called direct and indirect pathways, respectively (Albin et al., 1989; Delong, 1990).
Balanced regulation of the direct and indirect pathways is important for motor control, as evidenced by deficits associated with selective disruption of either the direct pathway (D1-enriched), or the indirect pathway (D2-enriched). The direct pathway MSNs target primarily two nuclei of the basal ganglia – the internal segment of the globus pallidus (GPi), known in rodents as the entopeduncular nucleus (EP), and the substantia nigra pars reticulata (SNr; Figure 1B). The SNr and GPi in turn send their outputs to brainstem nuclei and to components of the motor thalamus, which excite cortical areas related to motor control. The indirect pathway MSNs project to the external pallidal segment (GPe, often known simply as the globus pallidus in rodents), which inversely controls these same thalamo-cortical motor circuits. Support for this model, oversimplified as it undoubtedly is, has flourished with the development of genetically engineered mice and cell-type specific viral vectors in which the direct and indirect pathways can be separately characterized and controlled, including by optogenetic intervention (Valjent et al., 2009; Kravitz and Kreitzer, 2011). Such studies have contributed to our understanding of symptoms and therapies in movement disorders, including PD and HD, in which there is neurodegeneration of basal ganglia nuclei (Albin et al., 1989, 1995).
Studies of how the striatum controls movement have focused heavily on dopamine and the direct and indirect pathways. A less well-studied but equally distinct pair of striatal circuits originates in striatal compartments that are known as the striosomes (or patch) and matrix. These compartments can be defined by their pronounced segregation of numerous neurotransmitter-related signaling molecules (Graybiel, 1990). The striosomal compartment of the dorsal striatum forms a labyrinthine, interconnected structure that is embedded in the surrounding striatal matrix (Pert et al., 1976; Graybiel and Ragsdale, 1978; Herkenham and Pert, 1981; Gerfen, 1984). Whereas the main afferent connections of the matrix are related to associative and sensorimotor regions, striosomes have predominant limbic-related connections (Jimenez-Castellanos and Graybiel, 1987; Gerfen, 1989; Eblen and Graybiel, 1995; Kincaid and Wilson, 1996). Both striosomes and matrix contain MSNs that project to the direct and indirect pathway target nuclei but, in some documented cases, to separate subregions. Most, but not all, of the local connectivity of these MSNs is with other neurons within their own compartment or with interneurons located near the compartment borders (Somogyi et al., 1981; Gerfen, 1984; Walker et al., 1993; Kincaid and Wilson, 1996; Tokuno et al., 2002; Levesque and Parent, 2005; Fujiyama et al., 2006, 2011). The striosome compartment is thought to contain the only striatal neurons that have direct projections to the substantia nigra, pars compacta (SNc), which contains dopamine-producing neurons that project back to the entire dorsal striatum (Gerfen, 1984; Jimenez-Castellanos and Graybiel, 1989; Tokuno et al., 2002; Fujiyama et al., 2011).
The striosome and matrix compartments are typically defined by their gene expression profiles. Upward of 60 genes are reported to be differentially expressed in the striosomes and matrix (Table 1). This review is based on the proposition that these distinct molecular expression patterns are likely to reflect specialized processing by striosome-based circuits of the basal ganglia. As evidence already suggests that striosomes and matrix have differential vulnerability patterns in some basal ganglia-associated disorders, there is a clear need to understand how these compartments influence behavior in health and disease. Until very recently, the functions of striosomes and matrix have been surmised primarily on the basis of their activation profiles, mainly by immediate early gene (IEG) induction assays, and on the basis of their distinct input–output connections. The creation of genetic tools by which these two compartments can be selectively manipulated will certainly shed light on their function. Here, we review differences between the striosomes and matrix at the anatomical and gene expression level and differences between these two compartments in a wide variety of diseases associated with abnormal movement and emotion.
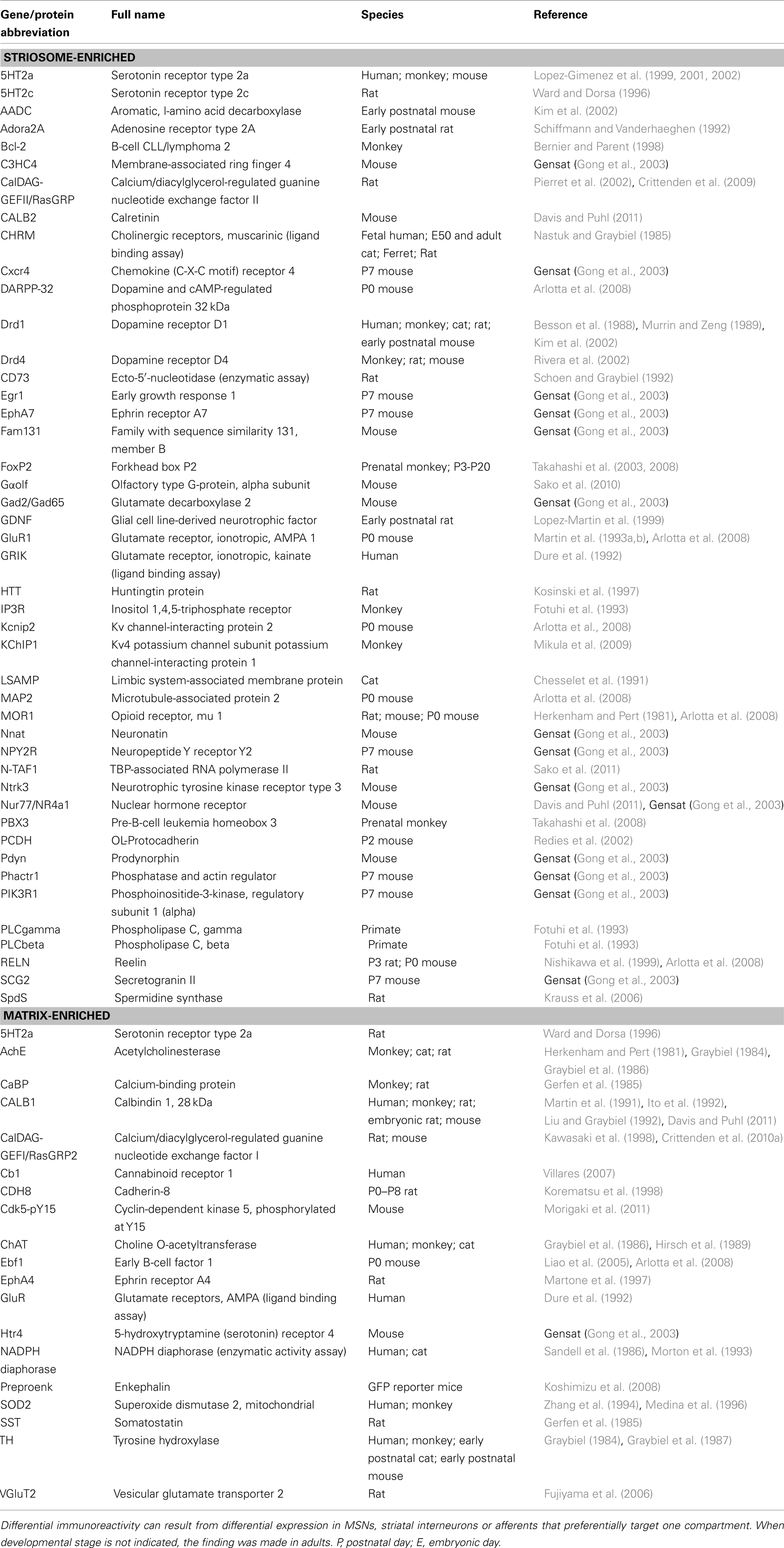
Table 1. Molecules with differential expression between the striosome and matrix compartments of the dorsal striatum.
Distinguishing Features of the Striosome and Matrix Compartments of the Dorsal Striatum
Development of the Striosome and Matrix Compartments
A striosome-substantia nigra-striatum circuit is established relatively early in the developing striatum. The striosomal neurons are born in a discrete time-window, during the time that layer 6 neurons of the neocortex are born (Graybiel and Hickey, 1982), as though forming an ontogenetic unit. They begin to migrate out from the lateral ganglionic eminence earlier in development than most matrix neurons, which are mainly born later in embryogenesis. The developing striosomes correspond to the “dopamine islands” formed by incoming dopamine-containing nigrostriatal fibers (Olson et al., 1972; Graybiel, 1984; Moon Edley and Herkenham, 1984; Fishell and Van Der Kooy, 1987). Anatomical studies suggest that the clusters of MSNs in the proto-striosomes begin to mature just in advance of the dopamine-containing fibers that demarcate the clusters (Newman-Gage and Graybiel, 1988). The dopamine-containing fiber input to striosomes is nevertheless required for the expression of some striosomal markers, including the mu opioid receptor (Van Der Kooy and Fishell, 1992).
The protostriosomal neurons project to developing dopamine-containing neurons in the ventral mesencephalon and secrete Reelin (Nishikawa et al., 1999), a protein that is essential for the lateral migration of dopamine-containing neurons that come to form the SNc (Nishikawa et al., 2003). The neurotrophic factor, GDNF, is also enriched in striosomes, and its expression in the striatum is important for survival of the dopamine-containing neurons (Lopez-Martin et al., 1999; Oo et al., 2005). As later-born matrix neurons migrate out into the striatum, they form caudal-to-rostral and lateral-to-medial gradients of migration, beginning around E20 in rats (Liu and Graybiel, 1992) and at comparable times in cats (Newman-Gage and Graybiel, 1988). Peri-natally, cell-type specific expression of ephrins and their receptors serve to compartmentalize the MSNs into striosome and matrix compartments (Janis et al., 1999; Passante et al., 2008). Finally, extracellular matrix proteins and peri-neuronal nets have dynamic compartmental distributions that may contribute to the consolidation of synaptic circuits and the mature appearance of the striatum by about P20 (Liu and Graybiel, 1992; Lee et al., 2008).
In adulthood, striosomes make up roughly 15% of the volume in the dorsal striatum (Johnston et al., 1990) of humans, monkeys, rats, and mice and they are largest in the rostral and medial parts of the striatum – to be precise, the medial and anterior caudoputamen in rodents and the caudate nucleus and anterior putamen in primates (Graybiel and Ragsdale, 1978). Striosome–matrix compartmentalization is largely, but not entirely, observed by the dendrite and local axon collaterals of striatal neurons. MSNs have axon collaterals that mainly synapse onto neighboring MSNs within the same compartment, and the dendritic trees of MSNs tend to be compartmentally confined (Walker et al., 1993; Kincaid and Wilson, 1996; Yung et al., 1996; Fujiyama et al., 2011). It is thought that differential activation of striosomes and matrix could be modulated, in part, by differentially distributed striatal interneurons, including cholinergic interneurons and somatostatin/nitric oxide synthase-positive interneurons that tend to be located at compartment borders (Graybiel et al., 1986; Kubota and Kawaguchi, 1993; Aosaki et al., 1994; Saka et al., 2002; Miura et al., 2007).
A number of transcription factors, including Ctip2 (Arlotta et al., 2008), RARβ (Liao et al., 2008), and Dlx1/2 (Anderson et al., 1997) are required for the differentiation of MSNs. The compartment-enriched expression of multiple genes has been found to change not only prenatally, but also during the period between birth and maturity, as the compartmental pattern matures. Remarkably, despite the limitation of anatomical marking of post-mortem human brain sections, evidence suggests that the outlines of this developmental pattern are visible in humans. Striosomes have been identified in prenatal striatum by histochemical (acetylcholinesterase) staining methods and a switch in the compartmental staining with this marker occurs during the postnatal period (Graybiel and Ragsdale, 1980). There are also cross-species variations in compartmental gene enrichment. Table 1 summarizes molecules reported to be differentially expressed in striosomes or extra-striosomal matrix, with a bias toward differences reported in adult animals.
Common and Distinct Efferent Connections of the Striosome and Matrix Compartments
Definitive evidence about the connections of striosomal MSNs has been difficult to obtain for technical reasons, but a recent study based on single-neuron labeling now suggests that in adult rats, striosomal MSNs may be unique among striatal MSNs in projecting directly to the SNc, which contains dopamine-producing neurons (Fujiyama et al., 2011). This single-neuron evidence is in accord with earlier work with classical anterograde and retrograde tracing methods (Gerfen, 1985; Jimenez-Castellanos and Graybiel, 1989; Tokuno et al., 2002). One such retrograde labeling experiment indicated that the densocellular zone of the SNc, which exhibits low levels of acetylcholinesterase (AchE) immunoreactivity relative to the surrounding region, is preferentially targeted by striosomes, which are also AchE-poor (Jimenez-Castellanos and Graybiel, 1989). In the rat, the striatonigral fibers have been suggested to form symmetric synapses on both the dendrites and cell bodies of the dopamine-containing neurons (Somogyi et al., 1981; Gerfen, 1984; Tokuno et al., 2002; Fujiyama et al., 2011). A monosynaptic connection between MSNs and dopamine-producing cells of the SNc is also consistent with electrophysiological recording assays in rats (Lee and Tepper, 2009). If confirmed by further work, the direct striosome-SNc projection would parallel a ventral striatal input to the SNc (Somogyi et al., 1981). The striosomes and ventral striatum are in a position to exert global control over dopamine signaling in the dorsal striatum.
Aside from this connection, both striosomes and matrix share in projecting to the main output nuclei of the basal ganglia, but in different proportions. These projections are more abundant from the large matrix compartment (Gerfen and Young, 1988; Jimenez-Castellanos and Graybiel, 1989; Rajakumar et al., 1993; Tokuno et al., 2002; Levesque and Parent, 2005; Fujiyama et al., 2011). Efferents from MSNs in the matrix have consistently been found not to project to the SNc but, rather, to project to the GPe, GPi, and SNr (Gerfen, 1984; Kawaguchi et al., 1990; Gimenez-Amaya and Graybiel, 1991; Levesque and Parent, 2005; Chuhma et al., 2011; Fujiyama et al., 2011). Likewise, a juxtacellular labeling experiment identified six MSNs, all located within the same striosome in the striatum of a squirrel monkey, that had projections to the GPi, GPe, and non-dopaminergic SNr, rather than to the dopamine-containing SNc (Levesque and Parent, 2005). Striosomal MSNs targeting the GPi, GPe, and SNr have also been reported in the rat. Striosomal MSNs that target the GPi/EP are reported to innervate specifically the regions of the GPi that in turn project to the lateral habenula, whereas the matrix MSNs targeting GPi/EP project to regions that are interconnected to motor regions of the thalamus (Rajakumar et al., 1993). This pathway to the habenula is notable, because the lateral habenula, itself interconnected with the prelimbic cortex, can inhibit dopamine cell firing in the SNc (Herkenham and Nauta, 1979; Christoph et al., 1986; Ji and Shepard, 2007; Matsumoto and Hikosaka, 2007; Bromberg-Martin et al., 2010). Thus, the striosomes appear to represent a unique dopamine- and limbic system-related circuit that is situated to influence the associative and sensorimotor regions of the striatum.
Based on these findings, a three-compartment model of striatal efferent connectivity has been proposed, including: (1) the direct pathway targeting the SNr with collaterals in the GPi/EP and GPe, (2) the indirect pathway targeting only the GPe, and (3) the striosome-specific pathway targeting the SNc, with collaterals in the GPi/EP and GPe (Figure 1B; Graybiel et al., 2000; Fujiyama et al., 2011). The apparently unique projection of striosomes to the SNc, if confirmed, would place striosomes in a functionally pivotal position to influence the dopamine system of the midbrain.
Relationship between the Direct–Indirect Pathway Divisions and Striosome–Matrix Compartments
MSNs can be classified according to whether they give rise to either the direct or indirect pathways, and they can also be classified according to whether they are in striosomes or in the extra-striosomal matrix. These are two distinct classificatory schemes. Most of the neurons of direct and indirect pathways lie in the matrix, which makes up approximately 80% of the volume of the striatum. There is evidence that some neurons in striosomes project to direct pathway targets, and others (fewer in number) project to indirect pathway target nuclei. Single-fiber tracing methods have allowed definitive identification of the collaterals of efferent axons of neurons identified as belonging to either striosomes or matrix. Fujiyama et al. (2011) labeled 11 striosomal neurons that were distributed across the striatum and in different rats, and found that 8 projected to the SNc, with collaterals in one or more of the direct pathway targets (SNr, GPi/EP, and GPe) and 3 exhibited an indirect pathway projection pattern, solely to the GPe. Similarly, in the monkey labeling experiment by Parent and colleagues, all six of the labeled striosomal neurons projected to the direct pathway nuclei (Levesque and Parent, 2005). Thus, at least some striosomes have an overabundance of neurons that target GPi/EP and SNr, but they may target specific regions of these nuclei, relative to matrix MSNs. In the matrix, as a whole, the MSNs are equally divided between the direct and indirect pathway projection types. MSNs that target the SNc appear to be restricted to the striosomes and are not found in the matrix.
Direct and indirect pathway MSNs are often identified by their expression of peptides in addition to their expression of dopamine receptors. Direct pathway D1-positive MSNs express substance P and prodynorphin, whereas the indirect pathway D2-positive MSNs express proenkephalin/enkephalin (Gerfen and Young, 1988). In adult rats, an estimated 5% of MSNs in the dorsal striatum express both D1/substance P and D2/enkephalin, whereas co-expression in the ventral striatum may be slightly higher (Surmeier et al., 1996; Wang et al., 2006a; Bertran-Gonzalez et al., 2008; Perreault et al., 2010). D1/D2 heteromers have been discovered and are thought to signal through phospholipase C, a calcium-dependent signaling cascade, rather than through the canonical adenylyl cyclase, cAMP-dependent dopamine receptor signaling cascade (Lee et al., 2004; Perreault et al., 2010). Neurons that co-express substance P and enkephalin have been found in both striosomes and matrix, but may represent a greater proportion of the MSNs in the striosomal compartment than in the matrix (Besson et al., 1990; Wang et al., 2007). Furthermore, co-expression of substance P and enkephalin was abundant in terminals in the SNc, but not in the GPe, GPi, or SNr (Wang et al., 2006a). Thus, it is possible that some of the unique subset of striosomal neurons that target the SNc co-express D1 and D2. This would stand in contrast to the direct pathway neurons of the matrix, which express primarily D1. However, the expression profiles of the striosome-specific pathway MSNs, which target the SNc, have not yet been thoroughly analyzed. Such analysis should take into account that the expression levels of the proteins that are typically used to define the pathways and compartments, including prodynorphin, mu opioid receptor, and dopamine receptors, can change with experience (Moratalla et al., 1996; Cenci et al., 1998; Adams et al., 2003; Jabourian et al., 2005; Perreault et al., 2010).
In summary, data support a model in which MSNs projecting to the SNc are confined to striosomes (striosome-specific pathway), whereas MSNs contributing to the direct and indirect pathway are present mainly in the matrix compartment but also in striosomes. The direct and indirect pathway target nuclei (GPi, SNr, and GPe/EP) receive more inputs from MSNs in the matrix than those in striosomes, because the matrix compartment is much larger than the striosomal compartment. The balance of signaling between the direct and indirect pathways is strongly influenced by dopamine and, therefore, striosomal MSNs are in a position to control this balance through their projection to the dopamine-containing neurons of the SNc. Cholinergic interneurons, which are often located at the border between striosomes and matrix, can also control dopamine release (Threlfell and Cragg, 2011) and the balance of direct vs. indirect pathway activation (Ding et al., 2011).
Amemori et al. (2011) propose a model whereby striosomes and indirect pathway MSNs select modules of previously learned behaviors that are appropriate for the given environmental context. In this model, the direct pathway selects specific actions within each striatal module, whereas the indirect pathway serves to select among modules. Based on contextual prediction errors originating in the limbic system, striosomes provide “responsibility signals” that regulate the activity of adjacent matrix MSNs within their respective modules. This modular regulation is amplified through the indirect pathway by virtue of funneling of representations, which serves to select modules (rather than specific actions) based on contextual information. This model is supported by computational modeling experiments and would support context-dependent learning and faster behavioral adaptation in a fluctuating environment.
Cerebral Cortex Afferents to Striosome and Matrix Compartments
Although a majority of neocortical areas may project to both striosomes and matrix, there are major differences in the strength of the projections to the two compartments. In keeping with the concept that ontogeny recapitulates phylogeny, striosomal MSNs not only begin differentiating before matrix MSNs, but also are innervated by neurons in more evolutionarily conserved regions of the cerebral cortex, especially the limbic medial and lateral margins of the cortical plate (Ragsdale and Graybiel, 1988; Gerfen, 1989). The orbitofrontal, anterior cingulate, and insular cortices preferentially innervate striosomes, whereas projections from the somatosensory, motor, and association cortices terminate mainly in the matrix (Ragsdale and Graybiel, 1990; Flaherty and Graybiel, 1994; Eblen and Graybiel, 1995; Kincaid and Wilson, 1996; Levesque and Parent, 1998). The amygdala, hippocampus, and nucleus accumbens (part of the ventral striatum) are interconnected with the orbitofrontal, anterior cingulate, and insular cortices, supporting the idea that the striosomes are part of a limbic circuit embedded in the sensorimotor and associative striatum (Graybiel, 2008). Indeed, in the cat, part of the amygdala itself also projects to part of the striosomal system (Ragsdale and Graybiel, 1988).
The strong innervation of medial striosomes by limbic neocortical regions that have reduced superficial layers is consistent with the fact that striosomal MSNs receive preferential inputs from deep cortical layer Vb (Gerfen, 1989; Eblen and Graybiel, 1995; Kincaid and Wilson, 1996; Levesque and Parent, 1998). MSNs in the matrix receive input from ontogenically younger, and putatively evolutionarily younger, cortical layers (III–Va). This is consistent with the finding that the somatosensory cortex, which is part of the neocortex that has a well-defined upper layer Va, projects to the matrix and not to the striosomes (Wilson, 1987; Gerfen, 1989). Nevertheless, the correlation between evolutionary age and compartment-specific targeting of corticostriatal neurons is speculative and does not fully represent the types of compartmentalized targeting that have been observed. For example, labeling of efferents from individual cortical regions was found to preferentially fill striosomes dorsally but to avoid striosomes ventrally, within their striatal target zones (Ragsdale and Graybiel, 1990). The striatal target zones themselves progressed dorso-ventrally according to the neighboring cortical regions from which they arose and thus, neighboring striosome–matrix compartments received input from neighboring cortical regions.
Corticostriatal projection neurons send efferents to other brain regions, including the brainstem, thalamus, claustrum, and contralateral cortex (Wilson, 1987; Kincaid and Wilson, 1996; Levesque et al., 1996). Upper layer V is enriched in intra-telencephalic-projecting (IT-type) neurons that can bifurcate to both hemispheres and synapse preferentially onto D1-positive MSNs. By contrast, deep layer V is enriched in pyramidal tract-projecting (PT-type) neurons that send collaterals preferentially to D2-positive MSNs in the ipsilateral striatum (Reiner et al., 2003). Axon terminals from PT-type cortical neurons were found to be larger and more often contacted a perforated post-synaptic density, compared to the IT-type corticostriatal terminals that contacted direct pathway MSNs (Reiner et al., 2010). This is consistent with the preferential activation of IEGs in enkephalin-containing neurons found in response to activation of the motor cortex (Berretta et al., 1997; Parthasarathy and Graybiel, 1997). Based on evidence that afferents to striosomes arise preferentially from deep layers of limbic cortex, one might expect striosomes to receive preferential PT-type inputs. However, the properties of corticostriatal synapses, such as terminal area and post-synaptic density length, were found to be similarly distributed between striosomes and matrix (Fujiyama et al., 2006) and both IT-type and PT-type projections have been detected in striosomes (Donoghue and Herkenham, 1986; Levesque and Parent, 1998; Wang and Pickel, 1998). These findings, together with the evidence that striosomes and matrix both have D1- and D2-dopamine receptor-expressing MSNs, even if not in equal proportion, indicate that each compartment receives input from both IT-type and PT-type cortical neurons. Reiner et al. (2010) point out that BAC mice expressing EGFP in either the PT neurons or the IT neurons will help to resolve this issue of whether there is preferential compartment targeting of one projection type.
In summary, each striatal compartment receives preferential inputs from different cortical regions and individual corticostriatal axons tend to innervate MSNs that are confined to a single compartment (Kincaid and Wilson, 1996). Nevertheless, subregions of the striatum that encompass both striosomes and neighboring clusters of cells in the matrix (matrisomes) are innervated by related cortical regions (Ragsdale and Graybiel, 1990; Flaherty and Graybiel, 1995; Kincaid and Wilson, 1996). Thus, MSNs in striosomes and matrisomes are thought to process related information and to communicate selectively with surrounding regions, likely via interneurons that have dendrites that cross compartmental borders. Such striatal interneurons include NOS/somatostatin-positive interneurons and parvalbumin-positive interneurons, which receive strong input from the cerebral cortex, and cholinergic interneurons, which receive strong input from the thalamus (Graybiel et al., 1986; Sandell et al., 1986; Lapper and Bolam, 1992; Lapper et al., 1992; Kubota and Kawaguchi, 1993; Bennett and Bolam, 1994; Aosaki et al., 1995; Kawaguchi, 1997; Saka et al., 2002; Miura et al., 2008).
Thalamic and Nigral Afferents to Striosome and Matrix Compartments
Thalamic and cortical neurons make excitatory, asymmetric synaptic connections onto MSNs in similar proportions (Doig et al., 2010). These inputs can be distinguished by immunostaining for the vesicular glutamate transporters VGluT1 (present in cortical terminals and also in less abundant amygdaloid terminals) and VGluT2 (present in thalamic terminals), according to evidence from numerous laboratories (Fremeau et al., 2001; Lacey et al., 2005; Raju et al., 2006). With these markers, it was found that inputs from the thalamus terminate on both dendritic spines and dendritic shafts of striatal neurons (Lacey et al., 2005; Raju et al., 2006), but the inputs to shafts are largely confined to the matrix (Fujiyama et al., 2006; Raju et al., 2006). This compartmental selectivity is likely due to the fact that inputs from the parafascicular nucleus, one of the intralaminar nuclei of the thalamus, terminate onto the shafts of cholinergic interneurons (Lapper and Bolam, 1992), which are abundant in the striatal matrix. The parafascicular inputs also contact spines and shafts of MSNs (Lacey et al., 2007). The intralaminar thalamic nuclei are highly interconnected with sensorimotor cortical regions and preferentially target the matrix (Herkenham and Pert, 1981; Ragsdale and Graybiel, 1991; Sadikot et al., 1992). By contrast, the midline paraventricular and rhomboid nuclei of the thalamus, which have strong connections with the nucleus accumbens and the amygdala, have been shown in the cat to target striosomes preferentially (Ragsdale and Graybiel, 1991). This pattern is consistent with striosomes participating in cortico-striato-thalamic circuits with limbic connections and the extra-striosomal matrix participating in cortico-striato-thalamic circuits with sensorimotor connections.
Striosomes and matrix receive dopamine-containing inputs preferentially from distinct groups of neurons in the SNc. The distinct localization of the striosome vs. matrix targeting nigrostriatal neurons has been better documented in rats than in primates (Joel and Weiner, 2000), but differences have been documented in multiple species. The striosomal MSNs are innervated by a group of densely packed dopamine-containing neurons in the ventral tier of the SNc (the A9 cell group) and groups of clustered dopamine-containing neurons that are intermingled with neurons of the SNr (densocellular zone; Gerfen et al., 1987; Jimenez-Castellanos and Graybiel, 1987; Langer and Graybiel, 1989; Prensa and Parent, 2001). These results suggested that there may be reciprocal connections between some of the striosomal system, at least of the so-called subcallosal streak in the lateral striatum, and the densocellular zone of the SNc (Jimenez-Castellanos and Graybiel, 1989; Prensa and Parent, 2001). Matrix neurons are preferentially innervated by dopaminergic neurons in the dorsal tier of the SNc, the retrorubral area (A8 cell group) and the ventral tegmental area (VTA; A10 cell group; Gerfen et al., 1987; Jimenez-Castellanos and Graybiel, 1987; Prensa and Parent, 2001). Nevertheless, single dopamine-containing fibers can innervate both striosomes and matrix (Matsuda et al., 2009) and, for example, ventral tier SNc neurons are reported to innervate the matrix surrounding a target striosome (Prensa and Parent, 2001). The dopamine-containing axons innervating striosomes are reported to be thicker and to have more varicosities than those innervating the matrix, even when the axons are simply different branches from the same innervating neuron (Gerfen et al., 1987; Prensa and Parent, 2001). The ultrastructure of the dopamine-containing afferents is equivalent between compartments, however, and situated so as to modulate both cortical and thalamic excitatory inputs to MSNs (Hanley and Bolam, 1997; Moss and Bolam, 2008). In addition to this compartmental organization of the nigrostriatal system is the well-known gradient of dopamine-containing inputs whereby the medial VTA projects to the ventral striatum and the SNc, located more laterally, projects to the dorsal striatum.
Striosomes, Signaling, and Disease
MSNs in the striosomes and matrix exhibit remarkably similar morphology and baseline electrophysiological profiles despite their divergent expression of numerous signaling molecules (Kawaguchi et al., 1989; Graybiel, 1990; Trytek et al., 1996; Miura et al., 2007). However, drug treatments that engage signaling cascades linked to dopamine, acetylcholine, and opioid receptors evoke strikingly different patterns of IEG induction and electrophysiologically recorded activity in the two compartments (Graybiel et al., 1990b; Krebs et al., 1991; Moratalla et al., 1996; Canales and Graybiel, 2000; Adams et al., 2003; Saka et al., 2004; Miura et al., 2007). Disruption or activation of these same neurotransmitter signaling cascades can lead to neurologic and neuropsychiatric disorders. Hence, the compartmentalized patterning of activity is likely to delineate important distinctions in the operation of the basal ganglia.
The best documented differences in activation of striosomes and matrix are for models of drug addiction and models of L-DOPA-induced dyskinesia (LIDs), two hyper-kinetic disorders in which there is hyper-responsivity of dopamine receptor signaling. In clinical studies, differences in neurodegeneration between striosome and matrix compartments have been reported for HD and dystonia, two additional disorders characterized by hyperactivation of the motor system. Differential degeneration has also been reported in a few cases of multiple system atrophy with predominantly parkinsonian features (MSA-P) and in animal models of PD, diseases characterized by hypo-kinesia. All of these disorders can be accompanied by mood disturbances. Differential degeneration of either the striosomes or the surrounding matrix would be expected to result in an imbalance in the numerous neurotransmitter signaling cascades that are differentially expressed between the two compartments.
It is notable that drug treatments and disease states can also induce differential activation of D1- vs. D2-expressing MSNs. We have reviewed some of these findings here for comparative purposes, but how these observations relate to such compartmentalized activation is still unclear. It is worth keeping in mind evidence that striosomes contain a greater abundance of MSNs that project to the direct pathway nuclei than MSNs projecting to indirect pathway nuclei. However, preferential activation of D1-expressing MSNs cannot always account for predominant striosomal activation because, in some cases, there is wide-spread suppression of the matrix compartment, which does not have an overabundance of D2-expressing MSNs. Furthermore, the identification of SNc-targeting MSNs in the striosomes suggests that some of the striosomal activation is related to this special pathway.
Here, we focus on prominent neuro-molecular aspects of each of these diseases, with an emphasis on extracellular signal-regulated kinases 1 and 2 (ERK1/2) and protein kinase A (PKA) signaling cascades (Figure 2), and we relate these to the striosome/matrix imbalances that have been reported (Table 2; Figure 3).
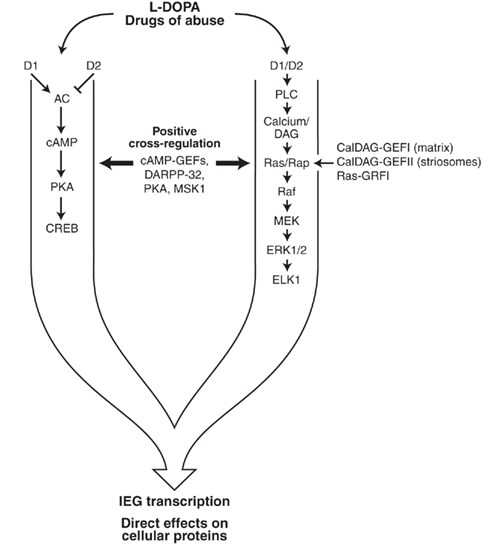
Figure 2. Simplified diagram of the protein kinase A (PKA) and extracellular signal-regulated kinase/mitogen-activated protein kinase (ERK1/2; MAPK) signaling cascades. Both PKA and ERK cascades control neuronal activity and immediate early gene (IEG) expression in medium spiny projection neurons of the striatum. D1 dopamine receptors promote the PKA cascade by activating adenylyl cyclase (AC) whereas D2 dopamine receptors inhibit AC. D1/D2 heterodimers are positively coupled to phospholipase C (PLC). The calcium- and diacylglycerol-regulated guanine nucleotide exchange factors (CalDAG-GEFs), which regulate the ERK1/2 cascade, are differentially expressed in the striosome and matrix compartments. Imbalances in striosome vs. matrix (IEG) transcription are implicated in L-DOPA-induced dyskinesias and drug addiction.
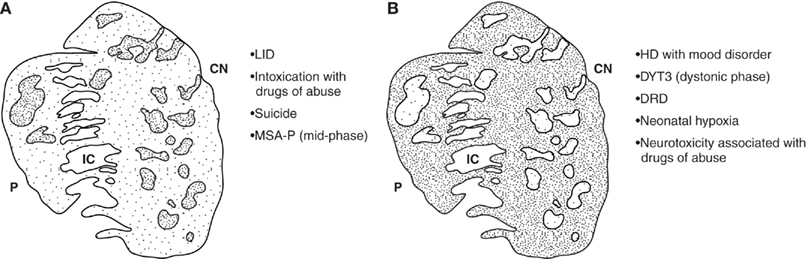
Figure 3. Models of striosome–matrix signaling imbalances in disease. Schematic diagram illustrating relative activity or cell density (dense stipple for high activity, sparse stipple for low activity) found in studies of human brain and brains of animal models. For the diseases or disease models listed, observations were made of either a relative imbalance in MSN cell density, immunomarker expression or IEG induction favoring the striosomes (A) or matrix (B), relative to the opposite compartment. The diagram is based on a cross-section through the striatum of an adult human, immunostained for choline acetyltransferase. Medial is to the right. CN, caudate nucleus; P, putamen; IC, internal capsule; LID, L-DOPA-induced dyskinesia; MSA-P, multiple system atrophy with parkinsonian features; HD, Huntington’s disease; DYT3, X-linked dystonia-parkinsonism; DRD, dopamine-responsive dystonia (DYT5).
Huntington’s Disease
Huntington’s disease is a fatal neurodegenerative disease caused by an expanded CAG repeat in the IT15 gene for Huntingtin protein (Htt; The Huntington’s Disease Collaborative Research Group, 1993). The CAG expansion encodes a polyglutamine tract that contributes to aggregation of the mutant Htt. HD is typified by the death of striatal MSNs and neocortical neurons and by symptoms of emotional disturbances and uncontrollable, choreic motor movements (Bates et al., 2002). HD hyper-kinesia may be linked in part to the preferential degeneration of D2 dopamine receptor-expressing MSNs of the indirect pathway, which normally inhibit movement (Reiner et al., 1988; Delong, 1990; Glass et al., 2000). Experiments regarding selective vulnerability of either striosome or matrix compartments have yielded variable results, however (Table 2).
Early reports indicated that tissue from individuals with early-stage HD have more severe loss of neurons and immunomarkers in the striosomes than in the matrix, whereas tissue from late-stage cases have equivalent neurodegeneration between compartments (Morton et al., 1993; Hedreen and Folstein, 1995). In mouse models, in which death of MSNs is minimal but neurite pathology is evident, there is more Htt aggregate burden and preferential volume loss in striosomes, relative to matrix (Menalled et al., 2002; Lawhorn et al., 2008). These patterns are not universal across individuals or models, and other clinical studies even found preferential loss of matrix markers (Ferrante et al., 1987; Seto-Ohshima et al., 1988). Some clarity to this controversy was provided by a study of 35 HD cases correlating symptomatology and compartmental-specific cell loss analyzed in post-mortem specimens (Tippett et al., 2007). In this study, reduced cell density in striosomes is more frequently observed in striatal sections from the brains of individuals who had presented early on with mood, rather than motor, symptoms. The emotional symptoms in the HD cases included depression, anxiety, and compulsively repetitive behaviors. Furthermore, in a follow-up study, it was shown that cell loss in the anterior cingulate cortex is more severe in post-mortem tissue samples from the individuals that presented with primarily emotional symptoms (Thu et al., 2010). Thus, neurodegeneration of components of the striosome limbic loop is associated with the emotional symptoms that characterize HD. Considering that post-mortem analysis is typically restricted to late-stage HD when nearly the entire brain is affected, longitudinal imaging studies, including of pre-symptomatic individuals, are providing more insight into the correlations between the complex symptomatology and neuronal degeneration patterns that typify HD (Rosas et al., 2008; Politis et al., 2011).
Despite the wide-spread expression of the mutant isoform of Htt in HD, there is disproportionate cell death of striatal MSNs and cortical pyramidal neurons, relative to loss of local interneurons or to neuronal loss in other brain regions. HD leads to a large array of cellular changes, including oxidative stress responses, transcriptional dysregulation, and excitotoxicity. Excitotoxicity is thought to be evoked, at least in part, by an impaired capacity to buffer the calcium rise that follows the stimulation of glutamate and dopamine receptors (Beal, 1998; Bezprozvanny and Hayden, 2004; Tang et al., 2007). Accordingly, disruption of either the cortical (glutamatergic) or nigral (dopamine-containing) afferents to the striatum is protective in the R6/2 mouse model of HD (Stack et al., 2007). Neocortical stimulation, D1 dopamine receptor activation and D2 receptor antagonism can all activate striatal ERK1/2, which exhibits altered activation patterns in HD models (Lievens et al., 2002; Roze et al., 2008). ERK1/2 signaling leads to phosphorylation and activation of the transcription factors ELK1 and CREB (Figure 2) and, thereby, could contribute to, or compensate for, the massive transcriptional dysregulation that occurs in HD (Cha, 2007). Other relevant changes in transcription factors are not discussed here, but even wildtype Htt, as well as mutant Htt, can directly control transcription (Benn et al., 2008).
In view of evidence that there are striosome-predominant and matrix-predominant patterns of cell loss in individual cases of HD, it is of special interest that two striatum-enriched molecules that signal through ERK1/2, CalDAG-GEFI, and CalDAG-GEFII (Figure 2), have strongly compartmentalized patterns of expression. CalDAG-GEFII (aka RasGRP) is expressed especially in striosomes, and CalDAG-GEFI is expressed preferentially in the matrix compartment. The CalDAG-GEFs are severely down-regulated in the R6/2 mouse model of HD and in post-mortem striatal tissue from individuals with HD (Crittenden et al., 2010a,b). This down-regulation is not a function of the preferential loss of D2-positive neurons in HD, considering that CalDAG-GEFI and CalDAG-GEFII are expressed in both prodynorphin and preproenkephalin-positive striatal neurons (Toki et al., 2001). The CalDAG-GEFs contain calcium and diacylglycerol binding domains and signal to the ERK1/2 phosphorylation cascade (Kawasaki et al., 1998) following the activation of cell surface receptors (Dower et al., 2000; Guo et al., 2001; Crittenden et al., 2004). For CalDAG-GEFI, it is known from work in a cortical slice model of HD that knockdown of CalDAG-GEFI expression blocks at least some of the deleterious effects of over-expressing a mutant fragment of the Htt protein (Crittenden et al., 2010a). Thus, the down-regulation of CalDAG-GEFI that is observed in HD may be compensatory. The consequences of CalDAG-GEFII dysregulation in HD models still need to be evaluated. Interestingly, in contrast to CalDAG-GEFI, another matrix-enriched calcium-binding protein, calbindin-D28K, is up-regulated in HD and HD animal models, and this change has been suggested to be protective against calcium-induced excitotoxicity (Huang et al., 1995; Sun et al., 2005). Most striatum-enriched genes are down-regulated in HD however (Desplats et al., 2006), which may be a compensatory response to the differential vulnerability of the MSN cell-type to mutant Htt expression.
Parkinson’s Disease and Parkinsonism
Parkinsonian disorders are characterized by difficulty initiating movements, bradykinesia, muscle rigidity, and postural instability. Idiopathic PD is an age-related neurodegenerative disease second only to Alzheimer’s disease in prevalence. PD is typified by degeneration of dopamine-containing neurons in the SNc, which results in the loss of the dopamine-containing innervation of the striatum, usually with a progression of loss from posterior regions (putamen) that are important for the execution of voluntary movements toward anterior regions (caudate nucleus) that are more associative and limbic in function (Kish et al., 1988). PD is now thought to arise from an interaction between the environment and genetic susceptibility. Exposure to mitochondrial toxins, such as the pesticide rotenone or MPTP-tainted drugs of abuse, are well-documented risk factors (Shulman et al., 2011). A number of genetic mutations can lead to PD, including mutations in SNCA, which encodes alpha-synuclein and for which there is a gene dosage effect that correlates with the severity of disease (Singleton et al., 2003; Chartier-Harlin et al., 2004; Farrer et al., 2004). The pathologic SNc hallmark of PD has long been identified as the large cytoplasmic inclusions known as Lewy bodies, and these are highly enriched in alpha-synuclein protein.
Different groups of dopaminergic neurons within the SNc appear to have different vulnerability to cell death in PD. For example, the loss of neurons within the densely packed cell groups called nigrosomes correlates with disease duration, whereas dopamine-containing neurons in surrounding parts of the SNc are less vulnerable (Damier et al., 1999b). The “nigrosomes” of the substantia nigra share with the striosomes of the dorsal striatum the characteristic of being weakly immunoreactive for calbindin-D28k, relative to other nigral regions (Damier et al., 1999a). A study assessing neuronal loss in a single section through the caudal SNc of 20 PD cases and 36 control cases found preferential loss of pigmented cells in the ventro-lateral SNc in PD, relative to age-related loss of more dorsally located pigmented neurons in controls (Fearnley and Lees, 1991). A second study also reported increased vulnerability of neurons located in the ventro-lateral SNc that are calbindin-D28k-negative (Yamada et al., 1990), but this pattern is not uniform across studies and is likely dependent on the individual and how the SNc subregions are defined (Hirsch et al., 1988; Gibb, 1992). Direct measurements of differential striatal loss of dopamine-containing terminals in striosomes or matrix have not yet been possible because in nearly all PD cases that come to autopsy, the depletion of dopamine is so extensive that markers of the nigrostriatal innervation yield little or no signal.
Differential loss of dopamine terminals in the striosomes, relative to matrix, is observed in a mouse model of dopamine deficiency, weaver, which carries a mutation in the GIRK2/Kir3.2/KCNJ6 gene that encodes a G-protein-activated inwardly rectifying potassium channel (Graybiel et al., 1990c). However, systemic deficits in weaver mice, including in the cerebellum, preclude simple interpretation of motor effects from their striosomal dopamine deficiency. Overall, results from neurotoxin-induced dopamine depletion in animal models of PD have been mixed, with some reporting increased dopamine terminal loss from striosomes and others increased loss from matrix (Table 2). This inconsistency may be related to the fact that the site of intracerebral neurotoxin injection influences the pattern of compartment-selective terminal loss (Zahm, 1991). These studies are further confounded by the fact that the immunomarker typically used to label dopamine terminals, anti-tyrosine hydroxylase (TH) immunoserum, shows higher immunoreactivity in the matrix than in striosomes of normative animals in some species. This differential anti-TH immunoreactivity is observed prominently in primates, including in humans, and weakly in rodents (Graybiel et al., 1987). This mosaic pattern of TH immunostaining was found to be preserved in several post-mortem PD cases, but could only be assessed in the most ventral parts of the caudate nucleus and putamen that were not fully depleted of this marker (Graybiel et al., 1990a). Immunostains for the matrix or striosome MSNs themselves also show grossly normal mosaic staining patterns in striatal sections from individuals with idiopathic PD or in cases of parkinsonism-dementia complex of Guam (Ito et al., 1992, 1993).
In a rare form of parkinsonism, MSA-P, differential matrix vs. striosome MSN degeneration has been reported. In MSA-P, there is degeneration of multiple neuronal types, including MSNs and nigral neurons. Analysis of brain sections through the striatum obtained from individuals that died with MSA-P and control cases showed preferential loss of the matrix marker, calbindin-D28k, and maintenance of a striosome immunomarker in the individuals with MSA-P (Goto and Hirano, 1990; Ito et al., 1992; Sato et al., 2007). It was suggested that, in MSA-P, there is selective loss of matrix MSNs in early stages of disease that progresses to loss of MSNs in both compartments by late stages of disease (Sato et al., 2007). Early loss of matrix signaling in MSA-P is consistent with the course of dopamine terminal loss in more common forms of PD, in which terminal loss progresses forward from the caudal putamen, which is relatively poor in striosomes (Graybiel and Ragsdale, 1978).
Considering the heterogeneity of PD-related disorders, it is tempting to speculate that a consistent compartmentalized pattern of dopamine terminal degeneration might be found if patients were classified according to detailed symptomatology, as has been reported for HD (Tippett et al., 2007; Rosas et al., 2008; Thu et al., 2010). As we discuss in the following section, the best evidence to date for the status of striosomes and matrix in PD brains comes from work on the expression of preproenkephalin opioids in post-mortem sections from PD brains. In these, striosomes are clearly preserved and over-activated in the brains of patients who experienced dyskinesias as side effects of treatment (Henry et al., 2003).
L-DOPA-Induced Dyskinesias
The most widely available treatment for PD is dopamine replacement therapy by oral administration of the dopamine precursor, L-3,4-dihydroxyphenylalanine (L-DOPA). However, as the disease progresses and the dopamine depletion becomes increasingly more severe, L-DOPA treatment often produces debilitating involuntary movements referred to as LIDs. LIDs are likely evoked, at least in part, by hyper-responsivity of MSNs to pulsatile dopamine receptor stimulation during ongoing corticostriatal activation of glutamate receptors (Jenner, 2008). Thus, treatment for these side effects typically requires reducing the L-DOPA dosage to suboptimal levels.
In rodent and non-human primate models, the occurrence of LIDs is correlated with activation of both D1 and D2 dopamine receptors and hyperactivation of the pro-movement direct pathway (Gerfen et al., 1995, 2002, 2008; Jenner, 2008; Berthet et al., 2009; Meurers et al., 2009; Cenci and Konradi, 2010). Stimulation of D1 and glutamate receptors leads to the activation of two key protein kinase cascades, denoted by their central kinase mediators: PKA and ERK1/2 (Figure 2). D1 is known to signal through G-protein coupled receptors to activate adenylyl cyclase for the generation of cAMP. cAMP, in turn, activates PKA, which site-specifically phosphorylates the dopamine and cAMP-regulated phosphoprotein 32 kDa (DARPP-32). DARPP-32 intersects with the ERK1/2 cascade by blocking protein phosphatases that would normally deactivate ERK1/2 (Valjent et al., 2005; Santini et al., 2007). PKA and ERK1/2 control chromatin remodeling and phosphorylate the CREB and ELK transcription factors that converge to drive expression of numerous IEGs, including, for example, c-Fos (Valjent et al., 2005; Santini et al., 2007, 2009). The induction of numerous IEGs has been observed in D1-positive MSNs in normal animals treated with dopamine receptor agonists, and this induction is dramatically increased in the dopamine-depleted striatum of animals treated with L-DOPA (Valjent et al., 2005; Santini et al., 2007, 2009). Protein translation is also activated in D1-positive MSNs of animal models of LIDs, via activation of mammalian target of rapamycin (mTOR) and ERK1/2 (Santini et al., 2009). Several studies indicate that in models of LIDs, there is a switch from PKA- to ERK1/2-mediated signaling in driving IEG expression (Andersson et al., 2001; Gerfen et al., 2002). Nevertheless, inhibition of ERK1/2, PKA, or mTOR in the striatum attenuates the development of LIDs in animal models (Gerfen et al., 2002; Westin et al., 2007; Darmopil et al., 2009; Santini et al., 2009; Lebel et al., 2010). The wide-spread expression and functions of ERK1/2, PKA, and mTOR make them poor targets for therapeutic intervention, however. Evidence from IEG induction assays in human and animal models suggests that there is a striosome to matrix imbalance in signaling cascades that parallels the development of LIDs. Strikingly striosome-predominant increases in the expression of prodynorphin (also called preproenkephalin B) have been documented in post-mortem striatal sections from parkinsonian humans with LIDs (Henry et al., 2003). In monkeys, the IEG Nur77 is dysregulated in striosomes following dopamine depletion with and without dopamine replacement treatments (Mahmoudi et al., 2009). In the anterior caudate nucleus of monkeys with dopamine depletion only, Nur77 is down-regulated in striosomes, relative to levels in control monkeys. By contrast, following dopamine depletion with subsequent L-DOPA treatment, Nur77 is strongly up-regulated in striosomal neurons. The degree of Nur77 up-regulation is inversely correlated with the severity of the LIDs, suggesting that the Nur77 modulation is compensatory. Striosome-predominant IEG induction is also observed in the dopamine-depleted striatum, relative to control striatum, in rat models of LIDs (Cenci et al., 1999; Saka et al., 1999).
This heightened IEG activity in striosomes may be related to activation of the striosome-specific pathway to the SNc (Figure 1B) or to the predominance of D1-positive neurons in striosomes of some species, relative to matrix (Table 1). These possibilities have not been directly assessed, but gene induction studies show increased IEG expression in D1-positive neurons, relative to changes in D2-positive neurons, in rat models of LIDs (Gerfen, 2003; Santini et al., 2009; Bateup et al., 2010). Furthermore, there may be increased signal transduction from D1 receptors in striosomes in LIDs, considering that Gαolf, the G-protein that is coupled to the D1 receptor, is elevated in post-mortem tissue from individuals who died with a diagnosis of PD (Corvol et al., 2004). Gαolf is enriched in striosomes in normal mice (Sako et al., 2010). An imbalance in striosome to matrix activity may also be related to the finding that selective antagonists of mu opioid receptors dramatically reduce LIDs in non-human primate models (Koprich et al., 2011). Mu opioid receptor activation contributes to differential activation of striosomes over matrix (Miura et al., 2007), but whether mu opioid receptor antagonists reduce excessive striosome to matrix activity in LIDs has not yet been reported.
Increasing evidence suggests that ERK1/2-mediated IEG induction in LIDs may involve the striosome-enriched gene, CalDAG-GEFII (Kawasaki et al., 1998; Dower et al., 2000; Pierret et al., 2002; Crittenden et al., 2009). CalDAG-GEFII, an ERK1/2 activator, is strongly up-regulated in a rat model of LIDs, and has a striking striosome-enriched pattern. By contrast, the matrix-enriched gene, CalDAG-GEFI, is down-regulated in the same model. The dysregulation of both of these genes was found to occur in proportion to the severity of LIDs that were expressed premortem in rats (Crittenden et al., 2009). Genetic deletion of another ERK1/2 activator, Ras-GRF1, partially alleviates the development of LIDs in a mouse model, and combined Ras-GRF1 and ERK1/2 knockdown reduces LIDs in a monkey model (Fasano et al., 2010). LIDs are further reduced in the Ras-GRF1 knockout mice by treatment with doses of ERK1/2 antagonists that are too low to be effective in control mice. These results suggest that there are multiple pathways to ERK1/2 activation in LIDs and that Ras-GRF1 and CalDAG-GEFII might, together, fully activate this pathway.
Recent studies highlight evidence that ERK1/2 activation in MSNs is high in the early stages of LIDs induction but decreases with prolonged treatment, despite the maintenance of the dyskinetic behaviors (Santini et al., 2010; Ding et al., 2011). By contrast, striatal DARPP-32 phosphorylation is highest in dopamine-depleted animals that received prolonged L-DOPA treatment. These results led to the proposal that ERK1/2 cascades are important for the priming that constitutes excessive responses of dopamine-depleted animals to the first-ever L-DOPA treatment, whereas cAMP/PKA/DARPP-32 signaling cascades are important for the maintenance of LIDs. However, activated ERK1/2 has been found in cholinergic interneurons of parkinsonian rodent models after prolonged L-DOPA treatment, indicating that ERK1/2 may be critical in MSNs for LID priming and in a sparsely distributed striatal interneuron for LID maintenance (Ding et al., 2011).
Taken together, this evidence strongly suggests that MSNs in striosomes are over-activated, relative to matrix MSNs, in LIDs. This differential activation is likely related to PKA and ERK1/2 activation in striatal MSNs (likely involving the CalDAG-GEFs and Ras-GRF1), and in striatal cholinergic interneurons. Strikingly, increased IEG activation of striosomes relative to matrix is also evoked by over-stimulation of the dopamine system with drugs of abuse. Such over-stimulation elicits repetitive motor and cognitive behaviors that share features with LIDs that are observed in a subpopulation of individuals with PD (Voon et al., 2009). There is a strong and direct correlation between the severity of repetitive behaviors induced by dopamine receptor agonists and the ratio of striosome to matrix IEG up-regulation, further suggesting a link between these different hyper-kinetic states (Canales and Graybiel, 2000; Graybiel et al., 2000).
Dystonia
Dystonia presents as twisting limb movements or sustained abnormal body postures that are attributed to failures in motor control and sensory integration by the cortico-basal ganglia-thalamic loop (Kaji et al., 2005). Electromyographic recordings from the muscles of patients with dystonia have shown that, during a dystonic event, there is co-contraction of opposing muscles or activation of muscle groups neighboring those that are normally used (Cohen and Hallett, 1988; Fahn, 1989). Dystonia can appear in childhood or in adulthood and can involve a single body part (focal), a group of adjacent body parts (segmental) or the whole body (generalized). As with PD, the susceptibility to disease genes and risk factors is variable (Roze et al., 2009; Schmidt et al., 2009). Dystonia can be evoked by a wide variety of phenomena, including repetitive motor tasks, anti-psychotic medications, brain damage, environmental factors, neurological disease (e.g., PD and HD) and genetic mutations. Intense repetition of a specific motor task, such as playing a musical instrument or typing, can predispose individuals to focal dystonias, including writer’s cramp (Byl et al., 1996; Roze et al., 2009). In a review of 158 hemidystonia cases involving stroke, head trauma, or perinatal hypoxia as precipitating factors, 60% were found to have lesions in the contralateral striatum (Chuang et al., 2002). However, disruptions to the striatum, neocortex, GPi, VTA, thalamus, cerebellum, and brain stem can all produce forms of dystonia-like symptoms in animal models (Guehl et al., 2009). Dystonia is also associated with complications of childbirth (Bressman, 2000), presumably because of the hyper-sensitivity of the neonatal basal ganglia to hypoxia. The latency between the precipitating event and the onset of dystonia is often months to years, supporting the hypothesis that aberrant neuroplasticity in the striatum and/or neocortex contributes to dystonia (Byl et al., 1996; Quartarone et al., 2003; Peterson et al., 2010). Disorganization of the somatotopy in the somatosensory and motor cortices and in the basal ganglia has been observed in dystonia (Lenz et al., 1998; Vitek et al., 1999; Quartarone et al., 2008; Delmaire et al., 2009; Tamura et al., 2009). This may bear relevance to the fact that touching a body region near the affected muscle groups can sometimes alleviate symptoms – a long-recognized phenomenon referred to as a “sensory trick.”
Given the heterogeneity in the etiology of dystonia, it is to be expected that there are multiple cellular signaling abnormalities. However, there is a scarcity of reports on signaling mechanisms in dystonia, relative to the many such studies in models of PD, LID, and HD. Dopamine and acetylcholine signaling are known to be important in several forms of dystonia. The clearest example of this is dopamine-responsive dystonia (DRD; also known as Segawa’s disease or DYT5). DRD is an autosomal dominant genetic disorder that is usually produced by loss of function mutations in GTP cyclohydrolase 1 (GCH1; Ichinose et al., 1994; Ludecke et al., 1996; Thony and Blau, 1997, 2006). GCH1 is in the pathway for synthesis of tetrahydrobiopterin (BH4), an essential cofactor for the dopamine-synthesizing protein, TH. Therefore, in DRD, there is likely a deficiency in dopamine, and related biogenic amines, throughout development. Age of onset is variable but often early, and symptoms range from focal to generalized dystonia to developmental delay (Segawa, 2011). Many of the symptoms are alleviated by L-DOPA administration, and LIDs are typically not a complication.
Loss of dopamine in adulthood is also associated with dystonia, as a secondary symptom of PD. In addition, the classical anti-psychotic medications that antagonize D2 receptor signaling precipitate acute or tardive dystonia in a significant proportion of patients and in animal models (Kiriakakis et al., 1998). Moderate abnormalities in D2 receptor binding and expression are observed in cases of focal and generalized dystonia (Tanabe et al., 2009), but whether the defects are in post-synaptic D2 receptors on MSNs, in presynaptic D2 autoreceptors that inhibit dopamine release or in D2-positive striatal interneurons remains controversial (Playford et al., 1993; Naumann et al., 1998; Napolitano et al., 2010).
Dopamine and acetylcholine signaling are tightly interrelated in the striatum and anticholinergic drugs improve dystonic symptoms in some patients and animal models (Bressman, 2000). Clues to the relevance of acetylcholine signaling in dystonia come from animal models of DYT1. DYT1 is produced by a mutation in torsinA (Ozelius et al., 1997), which is expressed widely but is enriched in the cholinergic interneurons of the striatum (thought to correspond mainly to the physiologically identified tonically active neurons, or TANs) and in dopaminergic neurons of the SNc (Shashidharan et al., 2000; Oberlin et al., 2004). D2 is expressed in cholinergic interneurons and can, under certain conditions, inhibit their activation and thereby permit long-term depression (LTD) in MSNs (Wang et al., 2006b). However, in slice recordings from the mouse model of DYT1, the effect is reversed such that D2 agonists activate cholinergic interneurons and MSNs fail to undergo LTD (Pisani et al., 2003). This failure of MSN neuroplasticity is rescued by blocking acetylcholine receptors. Thus, anticholinergics may serve to restore normal plasticity of MSNs in DYT1 models. Because cholinergic interneurons control striosome to matrix activity ratios (Saka et al., 2002), it is possible that anticholinergic therapies change the communication between these striatal compartments as well.
The most direct case made for a striosome to matrix imbalance in some types of dystonia comes from post-mortem analyses of striatal tissue from individuals with X-linked dystonia-parkinsonism (DYT3). DYT3 is caused by mutations in the transcription factor TAF1 and manifests as adult-onset dystonia, often generalized, that progresses to parkinsonism (Makino et al., 2007). Histological analyses of post-mortem striatal sections from five people with DYT3 who died during the dystonic phase and two people who died during the parkinsonian phase led to the discovery of severe striosome degeneration in the individuals with dystonia (Goto et al., 2005). Analyses suggested that gliosis and MSN loss is confined to the striosomal compartment in the individuals in the dystonic phase whereas MSN degeneration spreads to both compartments in the individuals in the parkinsonian phase of the disease. Striatal projections into the GPi, GPe, and substantia nigra all appear to be significantly depleted in a presented DYT3 case. TH staining for dopaminergic neurons in the substantia nigra was patchy, suggesting that there was also ventral midbrain pathology. Thus, DYT3 appears to cause degeneration of MSNs in the striosome compartment in the early phase of the disease and then progresses to cause degeneration of MSNs in the matrix compartment as well. This preferential loss of striosomal MSNs fits with the expression of N-TAF1, the neuron-specific isoform of TAF1 that is implicated as causative in DYT3. In the rat, N-TAF1 expression is strikingly enriched in striosomal MSNs, relative to the low expression in the extra-striosomal matrix (Sako et al., 2011).
Support for the possibility that striosomes are differentially affected in other forms of dystonia comes from a mouse model of an unusual form of DRD. To generate this mouse line, a mutation was introduced into 6-pyruvoyl tetrahydropterin synthase, an enzyme that is required for BH4 synthesis and that was found to be mutated in a human case of DRD (Hanihara et al., 1997). These mutant mice exhibit abnormal hindlimb clasping and slow beam-crossing behavior, suggestive of dystonic and parkinsonian symptoms. A postnatal, striosome-predominant loss of TH protein is observed. The pattern of TH depletion also exhibits similarities to that observed in PD in that it was depleted in a caudal-to-rostral gradient and preserved in the nucleus accumbens (Sato et al., 2008). Unlike in PD however, this DRD mouse model shows preservation of SNc integrity, based on immunostaining of brain sections for markers of dopaminergic neurons. Thus, it appears that 6-pyruvoyl tetrahydropterin synthase is required for TH expression in the terminals of dopaminergic neurons targeting the striosomes, suggesting that preferential dopamine loss in the striosomes may occur in DRD. Clinical studies have yet to test this idea, however.
Drug Addiction
Drug addiction is generally agreed to involve the repeated and escalating use of psychoactive drugs, despite adverse consequences such as loss of employment, friends, and family ties (Diagnostic and Statistical Manual of Mental Disorders IV). Only ∼15% of people and animals who experience a highly addictive drug escalate their use to the point of addiction (Deroche-Gamonet et al., 2004). Susceptibility to drug addiction has environmental components (in particular, repeated drug administration) and genetic components. Twin studies suggest that as much as 50% of the susceptibility may be genetic (Kreek et al., 2005). Relapse after withdrawal is associated, in both humans and animal models, with stress, re-exposure to the drug, or re-exposure to environmental cues associated with the drug (Sinha, 2008). Cues and experiences that promote reinstatement of drug use can activate the same brain regions as the drugs themselves, suggesting that they share neurocircuitry and underlying molecular mechanisms (Childress et al., 1999; Volkow et al., 2006).
It is widely agreed that there are differential effects of drugs of abuse on signaling pathways in the ventromedial and dorsolateral striatum, in the direct and indirect pathways, and in striosome and matrix compartments. Human brain imaging studies have shown that acute drug exposure activates limbic structures including the nucleus accumbens and amygdala and that repeated use expands the region of activation to include the dorsal striatum and neocortex (Breiter et al., 1997; Volkow et al., 2006; Porrino et al., 2007). This changing pattern of activation might be related to the change in self-reported effects of the drug: in the early phases, individuals describe hedonic effects of the drug but in late phases of addiction the effects are more likely to be described as “filling a hole” (Robinson and Berridge, 2008). This contrast fits with the notion that the ventromedial striatum and nucleus accumbens mediate goal-oriented behaviors (e.g., early, hedonia-seeking drug use) whereas the dorsolateral striatum and some neocortical regions mediate habitual behaviors (e.g., late, compulsive drug use; Yin et al., 2004; Barnes et al., 2005; Atallah et al., 2007). Considering that drugs of abuse and associated cues activate the dopamine system (Di Chiara and Imperato, 1988), the transition from ventral to dorsal striatum activity with repeated drug use may be influenced by indirectly recursive corticostriatal circuitry and an apparently progressive connectivity of the striatonigral loop (Haber et al., 2000; Ikemoto, 2007). This possibility has been supported by studies in rats in which physical disruption of this ventral to dorsal striatal connectivity pattern was shown to interfere with the development of habitual responding for drug access (Belin and Everitt, 2008). Thus, the development of a drug-taking habit may rely on the dorsal striatum, and other brain regions, that normally mediate the acquisition of motor and cognitive habits.
Locomotor and highly repetitive and idiosyncratic motor behaviors (stereotypies) are elicited in humans and animals under the influence psychomotor stimulants such as cocaine and amphetamine. These motoric responses to drugs of abuse escalate with repeated treatments, a phenomenon that is termed sensitization and that is thought to be related to drug addiction (Vezina and Leyton, 2009). There is strong evidence that both PKA and ERK1/2 cascades in MSNs mediate acute and sensitized responses to psychomotor stimulants (Ferguson and Robinson, 2004; Shi and Mcginty, 2006; Girault et al., 2007; Russo et al., 2010). Genetic deletion of DARPP-32 (a key effector of the PKA cascade) and inhibitors of MEK1 (an upstream activator of ERK1/2) each block sensitization to drugs of abuse (Valjent et al., 2005). Immunohistochemistry for phosphorylation of activating sites on various members of the PKA and ERK1/2 cascades show that drugs of abuse activate these cascades primarily in D1-expressing neurons (Valjent et al., 2005; Bertran-Gonzalez et al., 2008). Likewise, Delta FosB, an IEG that is downstream of both signaling cascades, is activated preferentially in D1-expressing neurons following drug treatments (Berretta et al., 1992; Hope et al., 1994; Moratalla et al., 1996; Zachariou et al., 2006; Fasano et al., 2009). Activation of D2-positive MSNs is not observed in most reports of MSN activation by drugs of abuse (Bertran-Gonzalez et al., 2008), but this finding may depend on the context in which the drug is taken, given that the activation of D2-positive MSNs is observed when drugs are administered in a novel cage (Badiani et al., 1999; Ferguson and Robinson, 2004; Vezina and Leyton, 2009). Furthermore, it is widely recognized that normal acute and sensitized responses to drugs of abuse are dependent on both D1- and D2-type dopamine receptor activation (Xu et al., 1994; Caine et al., 2002, 2007; Capper-Loup et al., 2002; Barrett et al., 2004; Bateup et al., 2010). Pharmacologic or genetic studies indicating a D2-dependence of drug responses do not typically specify the cell-type of action. Therefore, it may be that D2 activity is required in non-MSN cell types such as cholinergic interneurons, which are sparsely distributed in the striatum. Cholinergic interneurons are implicated in the development of LIDs (Ding et al., 2011) and are required for the increased striosome over matrix activity ratios associated with drugs of abuse (Saka et al., 2002). Psychomotor stimulants have also been shown to signal through D1/D2 heterodimers (Perreault et al., 2010). Thus, D2 expression in MSNs, striatal interneurons, dopamine-producing axon terminals, and D1/D2 heteromers may all be important for particular aspects of drug responsivity. Histochemical examination of post-mortem brains from cocaine addicts who tested positive for cocaine at autopsy, showed that mRNA for dynorphin was selectively elevated in striosomes in the caudate nucleus (Hurd and Herkenham, 1993). In animal models, strong behavioral responses to psychomotor stimulants, administered by intraperitoneal injection in the home cage, is correlated with a profound change in IEG induction in the striosome vs. matrix compartments, favoring increased activation in striosomes (Graybiel et al., 1990b; Canales and Graybiel, 2000; Vanderschuren et al., 2002; Glickstein and Schmauss, 2004; Saka et al., 2004). At very high doses, or with repeated treatments, psychomotor stimulants suppress locomotion in favor of a limited number of spatially confined stereotypies (Lyon and Robbins, 1975). In rats and monkeys, there is a direct correlation between the intensity of drug-induced stereotypy and the increased striosome to matrix ratio of IEG induction in the dorsolateral quadrant of the striatum (Canales and Graybiel, 2000; Saka et al., 2004). Based on this correlation, it was suggested that increased striosome activity and repressed matrix activity in this zone, together, could predict the degree of repetitive behavior expressed. The possibility that increased activity in the striosomes promotes stereotypies whereas activity in the matrix permits flexibility in motor behaviors is consistent with several additional studies. First, rats with a self-stimulation electrode implanted in or near a striosome learn to bar-press more frequently for stimulation than rats in which the stimulating electrode is implanted in the matrix (White and Hiroi, 1998). Second, metabolic labeling studies show that rats moving freely or under gentle restraint in their home cage have preferential activation of MSNs in the matrix compartment (Brown et al., 2002). Third, knockout mice that lack CalDAG-GEFI, an ERK1/2 regulator that is selectively enriched in the matrix compartment, show enhanced stereotypy responses to amphetamine, relative to sibling controls (Crittenden et al., 2006). Importantly, the CalDAG-GEFI knockout mice have normal locomotor responses to low doses of amphetamine, and locomote normally in the absence of drug, suggesting that disruption of matrix function specifically promotes stereotypies induced by high doses of amphetamine. The possibility that differential signaling in the striosomes vs. matrix is related to the switch from goal-oriented behavior to habitual, goal-independent behaviors that compare to drug-induced stereotypy is reviewed by Canales (2005).
Vanderschuren et al. (2002) showed that increased striosome to matrix activity was also observed in adult rats treated with a single high dose, or with repeated low doses, of amphetamine and suggested that the compartmental ratio change was correlated with overall drug response rather than specifically with stereotypies. However, this study did not rate stereotypic behaviors and even low doses of stimulant drug can induce intermittent stereotypies such as sniffing. A single high dose of methylphenidate, an indirect dopamine receptor agonist that is prescribed to children for ADHD, also induces preferential IEG expression in striosomes of adolescent mice (Davis and Puhl, 2011). In follow-up cell culture studies, methylphenidate appeared to be acting through D1 and cAMP-mediated signaling cascades, which are enriched in striosomes (Davis and Puhl, 2011). Likewise, in a study of spontaneous stereotypies in deer mice, it was found that there is an increase in direct over indirect pathway activity, relative to non-stereotypic controls (Tanimura et al., 2011). The stereotypic deer mice do not show changes in striosome to matrix activity, as measured by cytochrome oxidase histochemistry, however. Together, these studies indicate that an increased striosome to matrix activity ratio is correlated with hyper-responsivity to psychomotor stimulants.
Although one study found that deletion of a matrix-enriched signaling molecule (CalDAG-GEFI) is sufficient to promote drug-induced stereotypies (Crittenden et al., 2006), other studies show that an increase in striosome to matrix IEG activity is not necessary to elicit stereotypy. In rats, ablation of cholinergic and nitric oxide synthase-positive interneurons prevents the compartmentalized IEG induction ratio, but not the sensitization of drug-associated stereotypies (Saka et al., 2002). Additionally, mice lacking D2 show increased stereotypy responses to D1-type dopamine receptor agonists but do not exhibit increased striosome to matrix ratios of IEG induction (Glickstein and Schmauss, 2004). By contrast, mice with striosome, but not matrix, overexpression of the negative mGluR1/5 modulator, Homer1a, show enhanced locomotor and stereotypy responses to amphetamine (Tappe and Kuner, 2006). These mice may not have decreased mGluR1/5 signaling however, as they show strong and patchy (presumably striosomal) IEG induction in the striatum. Evidence that there is not a simple correlation between increased striosome function and increased responses to drugs of abuse comes from mice in which rostral striosomes fail to develop (Liao et al., 2008). Mice lacking RARβ, a transcription factor that is required for formation of rostral striosomes, exhibit enhanced repetitive behavioral responses to apomorphine. At a dose of apomorphine that does not affect behaviors of control mice, mice lacking RARβ show increased stereotypic responses. These results could be interpreted to indicate that increased striosome to matrix activity normally serves to dampen stereotypies. Alternatively, any striosome to matrix imbalance, regardless of direction, might predispose to drug-induced stereotypies. In addition, how the selective disruption of striosome or matrix function influences behavioral responses to drugs of abuse could depend on the context of drug exposure and which region of the striatum is activated. This apparent complexity is not surprising considering that different drugs of abuse induce distinct patterns of striosome and matrix IEG induction across the caudal–rostral and medial–lateral gradients of the striatum, and that there are differences in the connectivity and transmitter/modulator expression patterns of the striosomes themselves in different striatal regions (Graybiel et al., 1990b; Hurd and Herkenham, 1993; Wang et al., 1994; Tan et al., 2000; Adams et al., 2001).
Repeated treatment of rats with drugs of abuse also activates IEG expression in the cholinergic interneurons that reside at the compartment borders (Moratalla et al., 1996). This finding is likely related to the observation that prolonged L-DOPA treatment of PD models induces ERK activation in cholinergic interneurons (Ding et al., 2011). Moreover, pharmacological disruption of cholinergic signaling modulates stereotypy responses to drugs of abuse with a dependence on medial–lateral striatal gradients (Ohmori et al., 1995; Aliane et al., 2011). Ablation of cholinergic interneurons disrupts the increased striosome to matrix activity ratio in response to the direct dopamine receptor agonist, apomorphine (Saka et al., 2002). The CalDAG-GEFI knockout mice that have exaggerated drug-induced stereotypies have defective cholinergic signaling as well (Crittenden et al., 2006). Thus, cholinergic modulation of drug responses may occur, in part, through control of striosome to matrix activity ratios.
Studies in animal models suggest that the striosome and matrix compartments are differentially vulnerable to the loss of dopamine terminals that is caused by drug abuse. Treatment of mice with high doses of either methamphetamine or MDMA (“ecstasy”) causes selective degeneration of dopaminergic inputs to the striosomes, relative to the surrounding matrix (Granado et al., 2008, 2010). This selective vulnerability was suggested to be related to the relatively lower striosomal levels of superoxide dismutase 2 (SOD2; Zhang et al., 1994; Medina et al., 1996), which neutralizes the reactive oxygen species that are a byproduct of dopamine metabolism. Another possibility is that the enriched vascularization of striosomes (Breuer et al., 2005) causes them to have increased exposure to injected drugs. Striosomal immunomarkers are selectively depleted in rats treated with excitotoxic drugs or that undergo neonatal hypoxia (Burke and Baimbridge, 1993; Figueredo-Cardenas et al., 1998). The anti-apoptosis factor, Bcl-2, and the neurotrophic factor, GDNF, are enriched in striosomal neurons, suggesting that they may have compensatory anti-neurodegeneration mechanisms in place (Bernier and Parent, 1998; Lopez-Martin et al., 1999; Oo et al., 2005). There remain only a few cases in which brains of drug abusers were processed to detect striosomes, and cell death was not assayed in this study, but the selective striosomal enhancement of the opioid, dynorphin, could relate to striatum-based disruption of limbic-related behaviors in addition to effects attributable to the ventral striatum itself (Hurd and Herkenham, 1993).
In summary, normal and dopamine-depleted animals show a strong correlation between the degree of striosome to matrix ratio of MSN activation and the degree of behavioral response to dopamine agonists or drugs of abuse. New genetic tools that allow the specific activation or repression of genes in defined subsets of striosomal and matrix MSNs will allow us to better understand the meaning of this correlation.
Conclusion
Striosomes are heavily innervated by limbic cortices, which may be related to the preferential degeneration of striosomes and cingulate cortex in individuals with mood disorders associated with HD. Striosomes are also implicated in limbic control of motor behaviors based on findings that drug addicts and suicide victims exhibit preferentially increased striosomal expression of prodynorphin, relative to control individuals. Evidence for imbalance between striosome and matrix functions in PD is primarily associated with L-DOPA treatment that can produce dyskinesias. In some individuals, LIDs are associated with mood disruptions and compulsive behaviors that resemble behaviors seen in drug addicts. Animal models and a few clinical studies support the idea that there are similarities between the excessive dopamine signaling that occurs in MSNs of individuals with LIDs and in drug abuse. Hyperactivation of D1-positive MSNs and increased striosome to matrix activity ratios have been observed in models of both disorders. Inhibitors of PKA and ERK signaling can alleviate LIDs and drug sensitization but more specific targets are needed for therapeutic development. Dystonic symptoms of rigidity can co-occur with the basal ganglia disorders of HD, PD, and LIDs and several small clinical studies indicate differential degeneration of striosome vs. matrix MSNs in hereditary forms of dystonia. The striosome-specific pathway to the SNc may be important for some aspects of HD, LIDs, dystonia, and drug addiction, which have overlapping symptoms of hyperactivation of the motor system and mood disturbances.
Much remains to be determined about the relevance of striosome–matrix architecture to human neurologic and neuropsychiatric disorders. First and foremost, striosomes have so far been below-threshold for reliable detection by human brain imaging methods currently in use, so that evidence is restricted to rare post-mortem specimens. Even in instances in which observations have been made on human brains, they are necessarily incomplete. And importantly, even though there is mounting evidence that some aspects of repetitive behavior may be related to excessive striosomal signaling, there are still no studies for some human disorders in which repetitive behaviors are common symptoms, such as in autism spectrum disorders. Technical advances are close to overcoming some of these limitations, and promise insights into the functions of the highly developed striosomal system of the human striatum.
Conflict of Interest Statement
The authors declare that the research was conducted in the absence of any commercial or financial relationships that could be construed as a potential conflict of interest.
Acknowledgments
We thank Drs Leif G. Gibb, Ledia F. Hernandez, Min Jung Kim, Yasuo Kubota, and Carolyn J. Lacey for comments on the manuscript and Messrs. Alexander McWhinnie and Henry F. Hall for help with the illustrations. Supported by the National Institute of Child Health and Development (R01-HD028341); a Distinguished Investigator Award from NARSAD; the James and Pat Poitras Research Fund; the Grayce B. Kerr Fund; the Stanley H. and Sheila G. Sydney Fund; the Tourette Syndrome Association; the Simons Foundation; the Stanley Center for Psychiatric Research at the Broad Institute, via a grant to Edward Scolnick from the Stanley Medical Research Institute; the MGH/MIT Morris Udall Center of Excellence in Parkinson’s disease research; the National Parkinson Foundation; and the Michael J. Fox Foundation.
References
Adams, D. H., Hanson, G. R., and Keefe, K. A. (2001). Differential effects of cocaine and methamphetamine on neurotensin/neuromedin N and preprotachykinin messenger RNA expression in unique regions of the striatum. Neuroscience 102, 843–851.
Adams, D. H., Hanson, G. R., and Keefe, K. A. (2003). Distinct effects of methamphetamine and cocaine on preprodynorphin messenger RNA in rat striatal patch and matrix. J. Neurochem. 84, 87–93.
Albin, R. L., Young, A. B., and Penney, J. B. (1989). The functional anatomy of basal ganglia disorders. Trends Neurosci. 12, 366–375.
Albin, R. L., Young, A. B., and Penney, J. B. (1995). The functional anatomy of disorders of the basal ganglia. Trends Neurosci. 18, 63–64.
Alexander, G. E., Delong, M. R., and Strick, P. L. (1986). Parallel organization of functionally segregated circuits linking basal ganglia and cortex. Annu. Rev. Neurosci. 9, 357–381.
Aliane, V., Perez, S., Bohren, Y., Deniau, J. M., and Kemel, M. L. (2011). Key role of striatal cholinergic interneurons in processes leading to arrest of motor stereotypies. Brain 134, 110–118.
Amemori, K., Gibb, L. G., and Graybiel, A. M. (2011). Shifting responsibly: the importance of striatal modularity to reinforcement learning in uncertain environments. Front. Hum. Neurosci. 5:47. doi: 10.3389/fnhum.2011.00047
Anderson, S. A., Qiu, M., Bulfone, A., Eisenstat, D. D., Meneses, J., Pedersen, R., and Rubenstein, J. L. (1997). Mutations of the homeobox genes Dlx-1 and Dlx-2 disrupt the striatal subventricular zone and differentiation of late born striatal neurons. Neuron 19, 27–37.
Andersson, M., Konradi, C., and Cenci, M. A. (2001). cAMP response element-binding protein is required for dopamine-dependent gene expression in the intact but not the dopamine-denervated striatum. J. Neurosci. 21, 9930–9943.
Aosaki, T., Kimura, M., and Graybiel, A. M. (1995). Temporal and spatial characteristics of tonically active neurons of the primate’s striatum. J. Neurophysiol. 73, 1234–1252.
Aosaki, T., Tsubokawa, H., Ishida, A., Watanabe, K., Graybiel, A. M., and Kimura, M. (1994). Responses of tonically active neurons in the primate’s striatum undergo systematic changes during behavioral sensorimotor conditioning. J. Neurosci. 14, 3969–3984.
Arlotta, P., Molyneaux, B. J., Jabaudon, D., Yoshida, Y., and Macklis, J. D. (2008). Ctip2 controls the differentiation of medium spiny neurons and the establishment of the cellular architecture of the striatum. J. Neurosci. 28, 622–632.
Atallah, H. E., Lopez-Paniagua, D., Rudy, J. W., and O’reilly, R. C. (2007). Separate neural substrates for skill learning and performance in the ventral and dorsal striatum. Nat. Neurosci. 10, 126–131.
Badiani, A., Oates, M. M., Day, H. E., Watson, S. J., Akil, H., and Robinson, T. E. (1999). Environmental modulation of amphetamine-induced c-fos expression in D1 versus D2 striatal neurons. Behav. Brain Res. 103, 203–209.
Barnes, T. D., Kubota, Y., Hu, D., Jin, D. Z., and Graybiel, A. M. (2005). Activity of striatal neurons reflects dynamic encoding and recoding of procedural memories. Nature 437, 1158–1161.
Barrett, A. C., Miller, J. R., Dohrmann, J. M., and Caine, S. B. (2004). Effects of dopamine indirect agonists and selective D1-like and D2-like agonists and antagonists on cocaine self-administration and food maintained responding in rats. Neuropharmacology 47(Suppl. 1), 256–273.
Bates, G., Harper, P., and Jones, L. (2002). Huntington’s Disease. New York: Oxford University Press.
Bateup, H. S., Santini, E., Shen, W., Birnbaum, S., Valjent, E., Surmeier, D. J., Fisone, G., Nestler, E. J., and Greengard, P. (2010). Distinct subclasses of medium spiny neurons differentially regulate striatal motor behaviors. Proc. Natl. Acad. Sci. U.S.A. 107, 14845–14850.
Beal, M. F. (1998). Mitochondrial dysfunction in neurodegenerative diseases. Biochim. Biophys. Acta 1366, 211–223.
Belin, D., and Everitt, B. J. (2008). Cocaine seeking habits depend upon dopamine-dependent serial connectivity linking the ventral with the dorsal striatum. Neuron 57, 432–441.
Benn, C. L., Sun, T., Sadri-Vakili, G., Mcfarland, K. N., Dirocco, D. P., Yohrling, G. J., Clark, T. W., Bouzou, B., and Cha, J. H. (2008). Huntingtin modulates transcription, occupies gene promoters in vivo, and binds directly to DNA in a polyglutamine-dependent manner. J. Neurosci. 28, 10720–10733.
Bennett, B. D., and Bolam, J. P. (1994). Localisation of parvalbumin-immunoreactive structures in primate caudate-putamen. J. Comp. Neurol. 347, 340–356.
Bernier, P. J., and Parent, A. (1998). The anti-apoptosis bcl-2 proto-oncogene is preferentially expressed in limbic structures of the primate brain. Neuroscience 82, 635–640.
Berretta, S., Parthasarathy, H. B., and Graybiel, A. M. (1997). Local release of GABAergic inhibition in the motor cortex induces immediate-early gene expression in indirect pathway neurons of the striatum. J. Neurosci. 17, 4752–4763.
Berretta, S., Robertson, H. A., and Graybiel, A. M. (1992). Dopamine and glutamate agonists stimulate neuron-specific expression of Fos-like protein in the striatum. J. Neurophysiol. 68, 767–777.
Berthet, A., Porras, G., Doudnikoff, E., Stark, H., Cador, M., Bezard, E., and Bloch, B. (2009). Pharmacological analysis demonstrates dramatic alteration of D1 dopamine receptor neuronal distribution in the rat analog of L-DOPA-induced dyskinesia. J. Neurosci. 29, 4829–4835.
Bertran-Gonzalez, J., Bosch, C., Maroteaux, M., Matamales, M., Herve, D., Valjent, E., and Girault, J. A. (2008). Opposing patterns of signaling activation in dopamine D1 and D2 receptor-expressing striatal neurons in response to cocaine and haloperidol. J. Neurosci. 28, 5671–5685.
Besson, M. J., Graybiel, A. M., and Nastuk, M. A. (1988). [3H]SCH 23390 binding to D1 dopamine receptors in the basal ganglia of the cat and primate: delineation of striosomal compartments and pallidal and nigral subdivisions. Neuroscience 26, 101–119.
Besson, M. J., Graybiel, A. M., and Quinn, B. (1990). Co-expression of neuropeptides in the cat’s striatum: an immunohistochemical study of substance P, dynorphin B and enkephalin. Neuroscience 39, 33–58.
Bezprozvanny, I., and Hayden, M. R. (2004). Deranged neuronal calcium signaling and Huntington disease. Biochem. Biophys. Res. Commun. 322, 1310–1317.
Breiter, H. C., Gollub, R. L., Weisskoff, R. M., Kennedy, D. N., Makris, N., Berke, J. D., Goodman, J. M., Kantor, H. L., Gastfriend, D. R., Riorden, J. P., Mathew, R. T., Rosen, B. R., and Hyman, S. E. (1997). Acute effects of cocaine on human brain activity and emotion. Neuron 19, 591–611.
Breuer, O., Lawhorn, C., Miller, T., Smith, D. M., and Brown, L. L. (2005). Functional architecture of the mammalian striatum: mouse vascular and striosome organization and their anatomic relationships. Neurosci. Lett. 385, 198–203.
Bromberg-Martin, E. S., Matsumoto, M., Nakahara, H., and Hikosaka, O. (2010). Multiple timescales of memory in lateral habenula and dopamine neurons. Neuron 67, 499–510.
Brown, L. L., Feldman, S. M., Smith, D. M., Cavanaugh, J. R., Ackermann, R. F., and Graybiel, A. M. (2002). Differential metabolic activity in the striosome and matrix compartments of the rat striatum during natural behaviors. J. Neurosci. 22, 305–314.
Burke, R. E., and Baimbridge, K. G. (1993). Relative loss of the striatal striosome compartment, defined by calbindin-D28k immunostaining, following developmental hypoxic-ischemic injury. Neuroscience 56, 305–315.
Byl, N. N., Merzenich, M. M., and Jenkins, W. M. (1996). A primate genesis model of focal dystonia and repetitive strain injury: I. Learning-induced dedifferentiation of the representation of the hand in the primary somatosensory cortex in adult monkeys. Neurology 47, 508–520.
Caine, S. B., Negus, S. S., Mello, N. K., Patel, S., Bristow, L., Kulagowski, J., Vallone, D., Saiardi, A., and Borrelli, E. (2002). Role of dopamine D2-like receptors in cocaine self-administration: studies with D2 receptor mutant mice and novel D2 receptor antagonists. J. Neurosci. 22, 2977–2988.
Caine, S. B., Thomsen, M., Gabriel, K. I., Berkowitz, J. S., Gold, L. H., Koob, G. F., Tonegawa, S., Zhang, J., and Xu, M. (2007). Lack of self-administration of cocaine in dopamine D1 receptor knock-out mice. J. Neurosci. 27, 13140–13150.
Canales, J. J. (2005). Stimulant-induced adaptations in neostriatal matrix and striosome systems: transiting from instrumental responding to habitual behavior in drug addiction. Neurobiol. Learn. Mem. 83, 93–103.
Canales, J. J., and Graybiel, A. M. (2000). A measure of striatal function predicts motor stereotypy. Nat. Neurosci. 3, 377–383.
Capper-Loup, C., Canales, J. J., Kadaba, N., and Graybiel, A. M. (2002). Concurrent activation of dopamine D1 and D2 receptors is required to evoke neural and behavioral phenotypes of cocaine sensitization. J. Neurosci. 22, 6218–6227.
Cenci, M. A., and Konradi, C. (2010). Maladaptive striatal plasticity in L-DOPA-induced dyskinesia. Prog. Brain Res. 183, 209–233.
Cenci, M. A., Lee, C. S., and Bjorklund, A. (1998). L-DOPA-induced dyskinesia in the rat is associated with striatal overexpression of prodynorphin- and glutamic acid decarboxylase mRNA. Eur. J. Neurosci. 10, 2694–2706.
Cenci, M. A., Tranberg, A., Andersson, M., and Hilbertson, A. (1999). Changes in the regional and compartmental distribution of FosB- and JunB-like immunoreactivity induced in the dopamine-denervated rat striatum by acute or chronic L-dopa treatment. Neuroscience 94, 515–527.
Cha, J. H. (2007). Transcriptional signatures in Huntington’s disease. Prog. Neurobiol. 83, 228–248.
Chartier-Harlin, M. C., Kachergus, J., Roumier, C., Mouroux, V., Douay, X., Lincoln, S., Levecque, C., Larvor, L., Andrieux, J., Hulihan, M., Waucquier, N., Defebvre, L., Amouyel, P., Farrer, M., and Destee, A. (2004). Alpha-synuclein locus duplication as a cause of familial Parkinson’s disease. Lancet 364, 1167–1169.
Chesselet, M. F., Gonzales, C., and Levitt, P. (1991). Heterogeneous distribution of the limbic system-associated membrane protein in the caudate nucleus and substantia nigra of the cat. Neuroscience 40, 725–733.
Childress, A. R., Mozley, P. D., Mcelgin, W., Fitzgerald, J., Reivich, M., and O’brien, C. P. (1999). Limbic activation during cue-induced cocaine craving. Am. J. Psychiatry 156, 11–18.
Christoph, G. R., Leonzio, R. J., and Wilcox, K. S. (1986). Stimulation of the lateral habenula inhibits dopamine-containing neurons in the substantia nigra and ventral tegmental area of the rat. J. Neurosci. 6, 613–619.
Chuang, C., Fahn, S., and Frucht, S. J. (2002). The natural history and treatment of acquired hemidystonia: report of 33 cases and review of the literature. J. Neurol. Neurosurg. Psychiatr. 72, 59–67.
Chuhma, N., Tanaka, K. F., Hen, R., and Rayport, S. (2011). Functional connectome of the striatal medium spiny neuron. J. Neurosci. 31, 1183–1192.
Cohen, L. G., and Hallett, M. (1988). Hand cramps: clinical features and electromyographic patterns in a focal dystonia. Neurology 38, 1005–1012.
Corvol, J. C., Muriel, M. P., Valjent, E., Feger, J., Hanoun, N., Girault, J. A., Hirsch, E. C., and Herve, D. (2004). Persistent increase in olfactory type G-protein alpha subunit levels may underlie D1 receptor functional hypersensitivity in Parkinson disease. J. Neurosci. 24, 7007–7014.
Crittenden, J. R., Bergmeier, W., Zhang, Y., Piffath, C. L., Liang, Y., Wagner, D. D., Housman, D. E., and Graybiel, A. M. (2004). CalDAG-GEFI integrates signaling for platelet aggregation and thrombus formation. Nat. Med. 10, 982–986.
Crittenden, J. R., Cantuti-Castelvetri, I., Saka, E., Keller-Mcgandy, C. E., Hernandez, L. F., Kett, L. R., Young, A. B., Standaert, D. G., and Graybiel, A. M. (2009). Dysregulation of CalDAG-GEFI and CalDAG-GEFII predicts the severity of motor side-effects induced by anti-parkinsonian therapy. Proc. Natl. Acad. Sci. U.S.A. 106, 2892–2896.
Crittenden, J. R., Dunn, D. E., Merali, F. I., Woodman, B., Yim, M., Borkowska, A. E., Frosch, M. P., Bates, G. P., Housman, D. E., Lo, D. C., and Graybiel, A. M. (2010a). CalDAG-GEFI down-regulation in the striatum as a neuroprotective change in Huntington’s disease. Hum. Mol. Genet. 19, 1756–1765.
Crittenden, J. R., Dunn, D. E., Bowden, H. A., Woodman, B., Yim, M. J., Frosch, M. P., Bates, G. P., Housman, D. E., Lo, D. C., and Graybiel, A. M. (2010b). “Dysregulation of the striatum-enriched signaling molecules, CalDAG-GEFI and CalDAG-GEFII, in Huntington’s disease,” in Poster presentation at the Hereditary Disease Foundation’s HD2010 meeting: “The Milton Wexler Celebration of Life”, Cambridge, MA.
Crittenden, J. R., Picconi, B., Ghiglieri, V., Calabresi, P., Harlan, P., Housman, D. E., and Graybiel, A. M. (2006). “CalDAG-GEFI is required for sensitization to amphetamine-induced stereotypy and corticostriatal LTP, but not for locomotor sensitization and LTD,” in Poster presentation at the Society for Neuroscience Annual Meeting, Atlanta, GA.
Damier, P., Hirsch, E. C., Agid, Y., and Graybiel, A. M. (1999a). The substantia nigra of the human brain. I. Nigrosomes and the nigral matrix, a compartmental organization based on calbindin D(28K) immunohistochemistry. Brain 122 (Pt 8), 1421–1436.
Damier, P., Hirsch, E. C., Agid, Y., and Graybiel, A. M. (1999b). The substantia nigra of the human brain. II. Patterns of loss of dopamine-containing neurons in Parkinson’s disease. Brain 122 (Pt 8), 1437–1448.
Darmopil, S., Martin, A. B., De Diego, I. R., Ares, S., and Moratalla, R. (2009). Genetic inactivation of dopamine D1 but not D2 receptors inhibits L-DOPA-induced dyskinesia and histone activation. Biol. Psychiatry 66, 603–613.
Davis, M. I., and Puhl, H. L. III. (2011). Nr4a1-eGFP is a marker of striosome-matrix architecture, development and activity in the extended striatum. PLoS ONE 6, e16619. doi: 10.1371/journal.pone.0016619
Delmaire, C., Vidailhet, M., Wassermann, D., Descoteaux, M., Valabregue, R., Bourdain, F., Lenglet, C., Sangla, S., Terrier, A., Deriche, R., and Lehericy, S. (2009). Diffusion abnormalities in the primary sensorimotor pathways in writer’s cramp. Arch. Neurol. 66, 502–508.
Delong, M. R. (1990). Primate models of movement disorders of basal ganglia origin. Trends Neurosci. 13, 281–285.
Deroche-Gamonet, V., Belin, D., and Piazza, P. V. (2004). Evidence for addiction-like behavior in the rat. Science 305, 1014–1017.
Desplats, P. A., Kass, K. E., Gilmartin, T., Stanwood, G. D., Woodward, E. L., Head, S. R., Sutcliffe, J. G., and Thomas, E. A. (2006). Selective deficits in the expression of striatal-enriched mRNAs in Huntington’s disease. J. Neurochem. 96, 743–757.
Di Chiara, G., and Imperato, A. (1988). Drugs abused by humans preferentially increase synaptic dopamine concentrations in the mesolimbic system of freely moving rats. Proc. Natl. Acad. Sci. U.S.A. 85, 5274–5278.
Ding, Y., Won, L., Britt, J. P., Lim, S. A., Mcgehee, D. S., and Kang, U. J. (2011). Enhanced striatal cholinergic neuronal activity mediates L-DOPA-induced dyskinesia in parkinsonian mice. Proc. Natl. Acad. Sci. U.S.A. 108, 840–845.
Doig, N. M., Moss, J., and Bolam, J. P. (2010). Cortical and thalamic innervation of direct and indirect pathway medium-sized spiny neurons in mouse striatum. J. Neurosci. 30, 14610–14618.
Donoghue, J. P., and Herkenham, M. (1986). Neostriatal projections from individual cortical fields conform to histochemically distinct striatal compartments in the rat. Brain Res. 365, 397–403.
Dower, N. A., Stang, S. L., Bottorff, D. A., Ebinu, J. O., Dickie, P., Ostergaard, H. L., and Stone, J. C. (2000). RasGRP is essential for mouse thymocyte differentiation and TCR signaling. Nat. Immunol. 1, 317–321.
Dure, L. S. T., Young, A. B., and Penney, J. B. Jr. (1992). Compartmentalization of excitatory amino acid receptors in human striatum. Proc. Natl. Acad. Sci. U.S.A. 89, 7688–7692.
Eblen, F., and Graybiel, A. M. (1995). Highly restricted origin of prefrontal cortical inputs to striosomes in the macaque monkey. J. Neurosci. 15, 5999–6013.
Fahn, S. (1989). Clinical variants of idiopathic torsion dystonia. J. Neurol. Neurosurg. Psychiatry 52(Suppl.), 96–100.
Farrer, M., Kachergus, J., Forno, L., Lincoln, S., Wang, D. S., Hulihan, M., Maraganore, D., Gwinn-Hardy, K., Wszolek, Z., Dickson, D., and Langston, J. W. (2004). Comparison of kindreds with parkinsonism and alpha-synuclein genomic multiplications. Ann. Neurol. 55, 174–179.
Fasano, S., Bezard, E., D’antoni, A., Francardo, V., Indrigo, M., Qin, L., Dovero, S., Cerovic, M., Cenci, M. A., and Brambilla, R. (2010). Inhibition of Ras-guanine nucleotide-releasing factor 1 (Ras-GRF1) signaling in the striatum reverts motor symptoms associated with L-dopa-induced dyskinesia. Proc. Natl. Acad. Sci. U.S.A. 107, 21824–21829.
Fasano, S., D’antoni, A., Orban, P. C., Valjent, E., Putignano, E., Vara, H., Pizzorusso, T., Giustetto, M., Yoon, B., Soloway, P., Maldonado, R., Caboche, J., and Brambilla, R. (2009). Ras-guanine nucleotide-releasing factor 1 (Ras-GRF1) controls activation of extracellular signal-regulated kinase (ERK) signaling in the striatum and long-term behavioral responses to cocaine. Biol. Psychiatry 66, 758–768.
Fearnley, J. M., and Lees, A. J. (1991). Ageing and Parkinson’s disease: substantia nigra regional selectivity. Brain 114(Pt 5), 2283–2301.
Ferguson, S. M., and Robinson, T. E. (2004). Amphetamine-evoked gene expression in striatopallidal neurons: regulation by corticostriatal afferents and the ERK/MAPK signaling cascade. J. Neurochem. 91, 337–348.
Ferrante, R. J., Kowall, N. W., Beal, M. F., Martin, J. B., Bird, E. D., and Richardson, E. P. Jr. (1987). Morphologic and histochemical characteristics of a spared subset of striatal neurons in Huntington’s disease. J. Neuropathol. Exp. Neurol. 46, 12–27.
Figueredo-Cardenas, G., Harris, C. L., Anderson, K. D., and Reiner, A. (1998). Relative resistance of striatal neurons containing calbindin or parvalbumin to quinolinic acid-mediated excitotoxicity compared to other striatal neuron types. Exp. Neurol. 149, 356–372.
Fishell, G., and Van Der Kooy, D. (1987). Pattern formation in the striatum: developmental changes in the distribution of striatonigral neurons. J. Neurosci. 7, 1969–1978.
Flaherty, A. W., and Graybiel, A. M. (1994). Input-output organization of the sensorimotor striatum in the squirrel monkey. J. Neurosci. 14, 599–610.
Flaherty, A. W., and Graybiel, A. M. (1995). Motor and somatosensory corticostriatal projection magnifications in the squirrel monkey. J. Neurophysiol. 74, 2638–2648.
Fotuhi, M., Dawson, T. M., Sharp, A. H., Martin, L. J., Graybiel, A. M., and Snyder, S. H. (1993). Phosphoinositide second messenger system is enriched in striosomes: immunohistochemical demonstration of inositol 1,4,5-trisphosphate receptors and phospholipase C beta and gamma in primate basal ganglia. J. Neurosci. 13, 3300–3308.
Frechilla, D., Cobreros, A., Saldise, L., Moratalla, R., Insausti, R., Luquin, M., and Del Rio, J. (2001). Serotonin 5-HT(1A) receptor expression is selectively enhanced in the striosomal compartment of chronic parkinsonian monkeys. Synapse 39, 288–296.
Fremeau, R. T. Jr., Troyer, M. D., Pahner, I., Nygaard, G. O., Tran, C. H., Reimer, R. J., Bellocchio, E. E., Fortin, D., Storm-Mathisen, J., and Edwards, R. H. (2001). The expression of vesicular glutamate transporters defines two classes of excitatory synapse. Neuron 31, 247–260.
Fujiyama, F., Sohn, J., Nakano, T., Furuta, T., Nakamura, K. C., Matsuda, W., and Kaneko, T. (2011). Exclusive and common targets of neostriatofugal projections of rat striosome neurons: a single neuron-tracing study using a viral vector. Eur. J. Neurosci. 33, 668–677.
Fujiyama, F., Unzai, T., Nakamura, K., Nomura, S., and Kaneko, T. (2006). Difference in organization of corticostriatal and thalamostriatal synapses between patch and matrix compartments of rat neostriatum. Eur. J. Neurosci. 24, 2813–2824.
Gerfen, C. R. (1984). The neostriatal mosaic: compartmentalization of corticostriatal input and striatonigral output systems. Nature 311, 461–464.
Gerfen, C. R. (1985). The neostriatal mosaic. I. Compartmental organization of projections from the striatum to the substantia nigra in the rat. J. Comp. Neurol. 236, 454–476.
Gerfen, C. R. (1989). The neostriatal mosaic: striatal patch-matrix organization is related to cortical lamination. Science 246, 385–388.
Gerfen, C. R. (2003). D1 dopamine receptor supersensitivity in the dopamine-depleted striatum animal model of Parkinson’s disease. Neuroscientist 9, 455–462.
Gerfen, C. R., Baimbridge, K. G., and Miller, J. J. (1985). The neostriatal mosaic: compartmental distribution of calcium-binding protein and parvalbumin in the basal ganglia of the rat and monkey. Proc. Natl. Acad. Sci. U.S.A. 82, 8780–8784.
Gerfen, C. R., Herkenham, M., and Thibault, J. (1987). The neostriatal mosaic: II. Patch- and matrix-directed mesostriatal dopaminergic and non-dopaminergic systems. J. Neurosci. 7, 3915–3934.
Gerfen, C. R., Keefe, K. A., and Gauda, E. B. (1995). D1 and D2 dopamine receptor function in the striatum: coactivation of D1- and D2-dopamine receptors on separate populations of neurons results in potentiated immediate early gene response in D1-containing neurons. J. Neurosci. 15, 8167–8176.
Gerfen, C. R., Miyachi, S., Paletzki, R., and Brown, P. (2002). D1 dopamine receptor supersensitivity in the dopamine-depleted striatum results from a switch in the regulation of ERK1/2/MAP kinase. J. Neurosci. 22, 5042–5054.
Gerfen, C. R., Paletzki, R., and Worley, P. (2008). Differences between dorsal and ventral striatum in Drd1a dopamine receptor coupling of dopamine- and cAMP-regulated phosphoprotein-32 to activation of extracellular signal-regulated kinase. J. Neurosci. 28, 7113–7120.
Gerfen, C. R., and Young, W. S. III. (1988). Distribution of striatonigral and striatopallidal peptidergic neurons in both patch and matrix compartments: an in situ hybridization histochemistry and fluorescent retrograde tracing study. Brain Res. 460, 161–167.
Gibb, W. R. (1992). Melanin, tyrosine hydroxylase, calbindin and substance P in the human midbrain and substantia nigra in relation to nigrostriatal projections and differential neuronal susceptibility in Parkinson’s disease. Brain Res. 581, 283–291.
Gimenez-Amaya, J. M., and Graybiel, A. M. (1991). Modular organization of projection neurons in the matrix compartment of the primate striatum. J. Neurosci. 11, 779–791.
Girault, J. A., Valjent, E., Caboche, J., and Herve, D. (2007). ERK2: a logical AND gate critical for drug-induced plasticity? Curr. Opin. Pharmacol. 7, 77–85.
Glass, M., Dragunow, M., and Faull, R. L. (2000). The pattern of neurodegeneration in Huntington’s disease: a comparative study of cannabinoid, dopamine, adenosine and GABA(A) receptor alterations in the human basal ganglia in Huntington’s disease. Neuroscience 97, 505–519.
Glickstein, S. B., and Schmauss, C. (2004). Focused motor stereotypies do not require enhanced activation of neurons in striosomes. J. Comp. Neurol. 469, 227–238.
Gong, S., Zheng, C., Doughty, M. L., Losos, K., Didkovsky, N., Schambra, U. B., Nowak, N. J., Joyner, A., Leblanc, G., Hatten, M. E., and Heintz, N. (2003). A gene expression atlas of the central nervous system based on bacterial artificial chromosomes. Nature 425, 917–925.
Goto, S., and Hirano, A. (1990). Inhomogeneity of the putaminal lesion in striatonigral degeneration. Acta Neuropathol. 80, 204–207.
Goto, S., Lee, L. V., Munoz, E. L., Tooyama, I., Tamiya, G., Makino, S., Ando, S., Dantes, M. B., Yamada, K., Matsumoto, S., Shimazu, H., Kuratsu, J., Hirano, A., and Kaji, R. (2005). Functional anatomy of the basal ganglia in X-linked recessive dystonia-parkinsonism. Ann. Neurol. 58, 7–17.
Granado, N., Ares-Santos, S., O’shea, E., Vicario-Abejon, C., Colado, M. I., and Moratalla, R. (2010). Selective vulnerability in striosomes and in the nigrostriatal dopaminergic pathway after methamphetamine administration: early loss of TH in striosomes after methamphetamine. Neurotox. Res. 18, 48–58.
Granado, N., Escobedo, I., O’shea, E., Colado, I., and Moratalla, R. (2008). Early loss of dopaminergic terminals in striosomes after MDMA administration to mice. Synapse 62, 80–84.
Graybiel, A. M. (1984). Correspondence between the dopamine islands and striosomes of the mammalian striatum. Neuroscience 13, 1157–1187.
Graybiel, A. M. (1990). Neurotransmitters and neuromodulators in the basal ganglia. Trends Neurosci. 13, 244–254.
Graybiel, A. M. (2008). Habits, rituals, and the evaluative brain. Annu. Rev. Neurosci. 31, 359–387.
Graybiel, A. M., Baughman, R. W., and Eckenstein, F. (1986). Cholinergic neuropil of the striatum observes striosomal boundaries. Nature 323, 625–627.
Graybiel, A. M., Canales, J. J., and Capper-Loup, C. (2000). Levodopa-induced dyskinesias and dopamine-dependent stereotypies: a new hypothesis. Trends Neurosci. 23, S71–S77.
Graybiel, A. M., and Hickey, T. L. (1982). Chemospecificity of ontogenetic units in the striatum: demonstration by combining [3H]thymidine neuronography and histochemical staining. Proc. Natl. Acad. Sci. U.S.A. 79, 198–202.
Graybiel, A. M., Hirsch, E. C., and Agid, Y. (1990a). The nigrostriatal system in Parkinson’s disease. Adv. Neurol. 53, 17–29.
Graybiel, A. M., Moratalla, R., and Robertson, H. A. (1990b). Amphetamine and cocaine induce drug-specific activation of the c-fos gene in striosome-matrix compartments and limbic subdivisions of the striatum. Proc. Natl. Acad. Sci. U.S.A. 87, 6912–6916.
Graybiel, A. M., Ohta, K., and Roffler-Tarlov, S. (1990c). Patterns of cell and fiber vulnerability in the mesostriatal system of the mutant mouse weaver. I. Gradients and compartments. J. Neurosci. 10, 720–733.
Graybiel, A. M., Hirsch, E. C., and Agid, Y. A. (1987). Differences in tyrosine hydroxylase-like immunoreactivity characterize the mesostriatal innervation of striosomes and extrastriosomal matrix at maturity. Proc. Natl. Acad. Sci. U.S.A. 84, 303–307.
Graybiel, A. M., and Ragsdale, C. W. Jr. (1978). Histochemically distinct compartments in the striatum of human, monkeys, and cat demonstrated by acetylthiocholinesterase staining. Proc. Natl. Acad. Sci. U.S.A. 75, 5723–5726.
Graybiel, A. M., and Ragsdale, C. W. Jr. (1980). Clumping of acetylcholinesterase activity in the developing striatum of the human fetus and young infant. Proc. Natl. Acad. Sci. U.S.A. 77, 1214–1218.
Guehl, D., Cuny, E., Ghorayeb, I., Michelet, T., Bioulac, B., and Burbaud, P. (2009). Primate models of dystonia. Prog. Neurobiol. 87, 118–131.
Guo, F. F., Kumahara, E., and Saffen, D. (2001). A CalDAG-GEFI/Rap1/B-Raf cassette couples M(1) muscarinic acetylcholine receptors to the activation of ERK1/2. J. Biol. Chem. 276, 25568–25581.
Haber, S. N., Fudge, J. L., and Mcfarland, N. R. (2000). Striatonigrostriatal pathways in primates form an ascending spiral from the shell to the dorsolateral striatum. J. Neurosci. 20, 2369–2382.
Hanihara, T., Inoue, K., Kawanishi, C., Sugiyama, N., Miyakawa, T., Onishi, H., Yamada, Y., Osaka, H., Kosaka, K., Iwabuchi, K., and Owada, M. (1997). 6-Pyruvoyl-tetrahydropterin synthase deficiency with generalized dystonia and diurnal fluctuation of symptoms: a clinical and molecular study. Mov. Disord. 12, 408–411.
Hanley, J. J., and Bolam, J. P. (1997). Synaptology of the nigrostriatal projection in relation to the compartmental organization of the neostriatum in the rat. Neuroscience 81, 353–370.
Hedreen, J. C., and Folstein, S. E. (1995). Early loss of neostriatal striosome neurons in Huntington’s disease. J. Neuropathol. Exp. Neurol. 54, 105–120.
Henry, B., Duty, S., Fox, S. H., Crossman, A. R., and Brotchie, J. M. (2003). Increased striatal pre-proenkephalin B expression is associated with dyskinesia in Parkinson’s disease. Exp. Neurol. 183, 458–468.
Herkenham, M., and Nauta, W. J. (1979). Efferent connections of the habenular nuclei in the rat. J. Comp. Neurol. 187, 19–47.
Herkenham, M., and Pert, C. B. (1981). Mosaic distribution of opiate receptors, parafascicular projections and acetylcholinesterase in rat striatum. Nature 291, 415–418.
Hirsch, E., Graybiel, A. M., and Agid, Y. A. (1988). Melanized dopaminergic neurons are differentially susceptible to degeneration in Parkinson’s disease. Nature 334, 345–348.
Hirsch, E. C., Graybiel, A. M., Hersh, L. B., Duyckaerts, C., and Agid, Y. (1989). Striosomes and extrastriosomal matrix contain different amounts of immunoreactive choline acetyltransferase in the human striatum. Neurosci. Lett. 96, 145–150.
Hope, B. T., Nye, H. E., Kelz, M. B., Self, D. W., Iadarola, M. J., Nakabeppu, Y., Duman, R. S., and Nestler, E. J. (1994). Induction of a long-lasting AP-1 complex composed of altered Fos-like proteins in brain by chronic cocaine and other chronic treatments. Neuron 13, 1235–1244.
Huang, Q., Zhou, D., Sapp, E., Aizawa, H., Ge, P., Bird, E. D., Vonsattel, J. P., and Difiglia, M. (1995). Quinolinic acid-induced increases in calbindin D28k immunoreactivity in rat striatal neurons in vivo and in vitro mimic the pattern seen in Huntington’s disease. Neuroscience 65, 397–407.
Hurd, Y. L., and Herkenham, M. (1993). Molecular alterations in the neostriatum of human cocaine addicts. Synapse 13, 357–369.
Hurd, Y. L., Herman, M. M., Hyde, T. M., Bigelow, L. B., Weinberger, D. R., and Kleinman, J. E. (1997). Prodynorphin mRNA expression is increased in the patch vs matrix compartment of the caudate nucleus in suicide subjects. Mol. Psychiatry 2, 495–500.
Ichinose, H., Ohye, T., Takahashi, E., Seki, N., Hori, T., Segawa, M., Nomura, Y., Endo, K., Tanaka, H., Tsuji, S., Fujita, K., and Nagatsu, T. (1994). Hereditary progressive dystonia with marked diurnal fluctuation caused by mutations in the GTP cyclohydrolase I gene. Nat. Genet. 8, 236–242.
Ikemoto, S. (2007). Dopamine reward circuitry: two projection systems from the ventral midbrain to the nucleus accumbens-olfactory tubercle complex. Brain Res. Rev. 56, 27–78.
Iravani, M. M., Syed, E., Jackson, M. J., Johnston, L. C., Smith, L. A., and Jenner, P. (2005). A modified MPTP treatment regime produces reproducible partial nigrostriatal lesions in common marmosets. Eur. J. Neurosci. 21, 841–854.
Ito, H., Goto, S., Sakamoto, S., and Hirano, A. (1992). Calbindin-D28k in the basal ganglia of patients with parkinsonism. Ann. Neurol. 32, 543–550.
Ito, H., Goto, S., Sakamoto, S., and Hirano, A. (1993). Striosomal arrangement of met-enkephalin and substance P expression in parkinsonism-dementia complex on Guam. Acta Neuropathol. 85, 390–393.
Jabourian, M., Venance, L., Bourgoin, S., Ozon, S., Perez, S., Godeheu, G., Glowinski, J., and Kemel, M. L. (2005). Functional mu opioid receptors are expressed in cholinergic interneurons of the rat dorsal striatum: territorial specificity and diurnal variation. Eur. J. Neurosci. 21, 3301–3309.
Janis, L. S., Cassidy, R. M., and Kromer, L. F. (1999). Ephrin-A binding and EphA receptor expression delineate the matrix compartment of the striatum. J. Neurosci. 19, 4962–4971.
Jenner, P. (2008). Molecular mechanisms of L-DOPA-induced dyskinesia. Nat. Rev. Neurosci. 9, 665–677.
Ji, H., and Shepard, P. D. (2007). Lateral habenula stimulation inhibits rat midbrain dopamine neurons through a GABA(A) receptor-mediated mechanism. J. Neurosci. 27, 6923–6930.
Jimenez-Castellanos, J., and Graybiel, A. M. (1987). Subdivisions of the dopamine-containing A8-A9-A10 complex identified by their differential mesostriatal innervation of striosomes and extrastriosomal matrix. Neuroscience 23, 223–242.
Jimenez-Castellanos, J., and Graybiel, A. M. (1989). Compartmental origins of striatal efferent projections in the cat. Neuroscience 32, 297–321.
Joel, D., and Weiner, I. (2000). The connections of the dopaminergic system with the striatum in rats and primates: an analysis with respect to the functional and compartmental organization of the striatum. Neuroscience 96, 451–474.
Johnston, J. G., Gerfen, C. R., Haber, S. N., and Van Der Kooy, D. (1990). Mechanisms of striatal pattern formation: conservation of mammalian compartmentalization. Brain Res. Dev. Brain Res. 57, 93–102.
Kaji, R., Urushihara, R., Murase, N., Shimazu, H., and Goto, S. (2005). Abnormal sensory gating in basal ganglia disorders. J. Neurol. 252(Suppl. 4), IV13–IV16.
Kawaguchi, Y., Wilson, C. J., and Emson, P. C. (1989). Intracellular recording of identified neostriatal patch and matrix spiny cells in a slice preparation preserving cortical inputs. J. Neurophysiol. 62, 1052–1068.
Kawaguchi, Y., Wilson, C. J., and Emson, P. C. (1990). Projection subtypes of rat neostriatal matrix cells revealed by intracellular injection of biocytin. J. Neurosci. 10, 3421–3438.
Kawasaki, H., Springett, G. M., Toki, S., Canales, J. J., Harlan, P., Blumenstiel, J. P., Chen, E. J., Bany, I. A., Mochizuki, N., Ashbacher, A., Matsuda, M., Housman, D. E., and Graybiel, A. M. (1998). A Rap guanine nucleotide exchange factor enriched highly in the basal ganglia. Proc. Natl. Acad. Sci. U.S.A. 95, 13278–13283.
Kim, D. S., Froelick, G. J., and Palmiter, R. D. (2002). Dopamine-dependent desensitization of dopaminergic signaling in the developing mouse striatum. J. Neurosci. 22, 9841–9849.
Kincaid, A. E., and Wilson, C. J. (1996). Corticostriatal innervation of the patch and matrix in the rat neostriatum. J. Comp. Neurol. 374, 578–592.
Kiriakakis, V., Bhatia, K. P., Quinn, N. P., and Marsden, C. D. (1998). The natural history of tardive dystonia. A long-term follow-up study of 107 cases. Brain 121(Pt 11), 2053–2066.
Kish, S. J., Shannak, K., and Hornykiewicz, O. (1988). Uneven pattern of dopamine loss in the striatum of patients with idiopathic Parkinson’s disease. Pathophysiologic and clinical implications. N. Engl. J. Med. 318, 876–880.
Koprich, J. B., Fox, S. H., Johnston, T. H., Goodman, A., Le Bourdonnec, B., Dolle, R. E., Dehaven, R. N., Dehaven-Hudkins, D. L., Little, P. J., and Brotchie, J. M. (2011). The selective mu-opioid receptor antagonist adl5510 reduces levodopa-induced dyskinesia without affecting antiparkinsonian action in mptp-lesioned macaque model of Parkinson’s disease. Mov. Disord. 26, 1225–1233.
Korematsu, K., Goto, S., Okamura, A., and Ushio, Y. (1998). Heterogeneity of cadherin-8 expression in the neonatal rat striatum: comparison with striatal compartments. Exp. Neurol. 154, 531–536.
Koshimizu, Y., Wu, S. X., Unzai, T., Hioki, H., Sonomura, T., Nakamura, K. C., Fujiyama, F., and Kaneko, T. (2008). Paucity of enkephalin production in neostriatal striosomal neurons: analysis with preproenkephalin-green fluorescent protein transgenic mice. Eur. J. Neurosci. 28, 2053–2064.
Kosinski, C. M., Cha, J. H., Young, A. B., Persichetti, F., Macdonald, M., Gusella, J. F., Penney, J. B. Jr., and Standaert, D. G. (1997). Huntingtin immunoreactivity in the rat neostriatum: differential accumulation in projection and interneurons. Exp. Neurol. 144, 239–247.
Krauss, M., Langnaese, K., Richter, K., Brunk, I., Wieske, M., Ahnert-Hilger, G., Veh, R. W., and Laube, G. (2006). Spermidine synthase is prominently expressed in the striatal patch compartment and in putative interneurones of the matrix compartment. J. Neurochem. 97, 174–189.
Kravitz, A. V., and Kreitzer, A. C. (2011). Optogenetic manipulation of neural circuitry in vivo. Curr. Opin. Neurobiol. 21, 433–439.
Krebs, M. O., Trovero, F., Desban, M., Gauchy, C., Glowinski, J., and Kemel, M. L. (1991). Distinct presynaptic regulation of dopamine release through NMDA receptors in striosome- and matrix-enriched areas of the rat striatum. J. Neurosci. 11, 1256–1262.
Kreek, M. J., Nielsen, D. A., Butelman, E. R., and Laforge, K. S. (2005). Genetic influences on impulsivity, risk taking, stress responsivity and vulnerability to drug abuse and addiction. Nat. Neurosci. 8, 1450–1457.
Kubota, Y., and Kawaguchi, Y. (1993). Spatial distributions of chemically identified intrinsic neurons in relation to patch and matrix compartments of rat neostriatum. J. Comp. Neurol. 332, 499–513.
Lacey, C. J., Bolam, J. P., and Magill, P. J. (2007). Novel and distinct operational principles of intralaminar thalamic neurons and their striatal projections. J. Neurosci. 27, 4374–4384.
Lacey, C. J., Boyes, J., Gerlach, O., Chen, L., Magill, P. J., and Bolam, J. P. (2005). GABA(B) receptors at glutamatergic synapses in the rat striatum. Neuroscience 136, 1083–1095.
Langer, L. F., and Graybiel, A. M. (1989). Distinct nigrostriatal projection systems innervate striosomes and matrix in the primate striatum. Brain Res. 498, 344–350.
Lapper, S. R., and Bolam, J. P. (1992). Input from the frontal cortex and the parafascicular nucleus to cholinergic interneurons in the dorsal striatum of the rat. Neuroscience 51, 533–545.
Lapper, S. R., Smith, Y., Sadikot, A. F., Parent, A., and Bolam, J. P. (1992). Cortical input to parvalbumin-immunoreactive neurones in the putamen of the squirrel monkey. Brain Res. 580, 215–224.
Lawhorn, C., Smith, D. M., and Brown, L. L. (2008). Striosome-matrix pathology and motor deficits in the YAC128 mouse model of Huntington’s disease. Neurobiol. Dis. 32, 471–478.
Lebel, M., Chagniel, L., Bureau, G., and Cyr, M. (2010). Striatal inhibition of PKA prevents levodopa-induced behavioural and molecular changes in the hemiparkinsonian rat. Neurobiol. Dis. 38, 59–67.
Lee, C. R., and Tepper, J. M. (2009). Basal ganglia control of substantia nigra dopaminergic neurons. J. Neural Transm. Suppl. 73, 71–90.
Lee, H., Leamey, C. A., and Sawatari, A. (2008). Rapid reversal of chondroitin sulfate proteoglycan associated staining in subcompartments of mouse neostriatum during the emergence of behaviour. PLoS ONE 3, e3020. doi: 10.1371/journal.pone.0003020
Lee, S. P., So, C. H., Rashid, A. J., Varghese, G., Cheng, R., Lanca, A. J., O’dowd, B. F., and George, S. R. (2004). Dopamine D1 and D2 receptor Co-activation generates a novel phospholipase C-mediated calcium signal. J. Biol. Chem. 279, 35671–35678.
Lenz, F. A., Suarez, J. I., Metman, L. V., Reich, S. G., Karp, B. I., Hallett, M., Rowland, L. H., and Dougherty, P. M. (1998). Pallidal activity during dystonia: somatosensory reorganisation and changes with severity. J. Neurol. Neurosurg. Psychiatr. 65, 767–770.
Levesque, M., Charara, A., Gagnon, S., Parent, A., and Deschenes, M. (1996). Corticostriatal projections from layer V cells in rat are collaterals of long-range corticofugal axons. Brain Res. 709, 311–315.
Levesque, M., and Parent, A. (1998). Axonal arborization of corticostriatal and corticothalamic fibers arising from prelimbic cortex in the rat. Cereb. Cortex 8, 602–613.
Levesque, M., and Parent, A. (2005). The striatofugal fiber system in primates: a reevaluation of its organization based on single-axon tracing studies. Proc. Natl. Acad. Sci. U.S.A. 102, 11888–11893.
Liao, W. L., Tsai, H. C., Wang, H. F., Chang, J., Lu, K. M., Wu, H. L., Lee, Y. C., Tsai, T. F., Takahashi, H., Wagner, M., Ghyselinck, N. B., Chambon, P., and Liu, F. C. (2008). Modular patterning of structure and function of the striatum by retinoid receptor signaling. Proc. Natl. Acad. Sci. U.S.A. 105, 6765–6770.
Liao, W. L., Tsai, H. C., Wu, C. Y., and Liu, F. C. (2005). Differential expression of RARbeta isoforms in the mouse striatum during development: a gradient of RARbeta2 expression along the rostrocaudal axis. Dev. Dyn. 233, 584–594.
Lievens, J. C., Woodman, B., Mahal, A., and Bates, G. P. (2002). Abnormal phosphorylation of synapsin I predicts a neuronal transmission impairment in the R6/2 Huntington’s disease transgenic mice. Mol. Cell. Neurosci. 20, 638–648.
Liu, F. C., and Graybiel, A. M. (1992). Heterogeneous development of calbindin-D28K expression in the striatal matrix. J. Comp. Neurol. 320, 304–322.
Lopez-Gimenez, J. F., Mengod, G., Palacios, J. M., and Vilaro, M. T. (1999). Human striosomes are enriched in 5-HT2A receptors: autoradiographical visualization with [3H]MDL100,907,[125I](±)DOI and [3H]ketanserin. Eur. J. Neurosci. 11, 3761–3765.
Lopez-Gimenez, J. F., Tecott, L. H., Palacios, J. M., Mengod, G., and Vilaro, M. T. (2002). Serotonin 5- HT (2C) receptor knockout mice: autoradiographic analysis of multiple serotonin receptors. J. Neurosci. Res. 67, 69–85.
Lopez-Gimenez, J. F., Vilaro, M. T., Palacios, J. M., and Mengod, G. (2001). Mapping of 5-HT2A receptors and their mRNA in monkey brain: [3H]MDL100,907 autoradiography and in situ hybridization studies. J. Comp. Neurol. 429, 571–589.
Lopez-Martin, E., Caruncho, H. J., Rodriguez-Pallares, J., Guerra, M. J., and Labandeira-Garcia, J. L. (1999). Striatal dopaminergic afferents concentrate in GDNF-positive patches during development and in developing intrastriatal striatal grafts. J. Comp. Neurol. 406, 199–206.
Ludecke, B., Knappskog, P. M., Clayton, P. T., Surtees, R. A., Clelland, J. D., Heales, S. J., Brand, M. P., Bartholome, K., and Flatmark, T. (1996). Recessively inherited L-DOPA-responsive parkinsonism in infancy caused by a point mutation (L205P) in the tyrosine hydroxylase gene. Hum. Mol. Genet. 5, 1023–1028.
Lyon, M., and Robbins, T. W. (1975). The Action of Central Nervous System Stimulant Drugs: A General Theory Concerning Amphetamine Effects. New York, NY: Spectrum.
Mahmoudi, S., Samadi, P., Gilbert, F., Ouattara, B., Morissette, M., Gregoire, L., Rouillard, C., Di Paolo, T., and Levesque, D. (2009). Nur77 mRNA levels and L-Dopa-induced dyskinesias in MPTP monkeys treated with docosahexaenoic acid. Neurobiol. Dis. 36, 213–222.
Makino, S., Kaji, R., Ando, S., Tomizawa, M., Yasuno, K., Goto, S., Matsumoto, S., Tabuena, M. D., Maranon, E., Dantes, M., Lee, L. V., Ogasawara, K., Tooyama, I., Akatsu, H., Nishimura, M., and Tamiya, G. (2007). Reduced neuron-specific expression of the TAF1 gene is associated with X-linked dystonia-parkinsonism. Am. J. Hum. Genet. 80, 393–406.
Martin, L. J., Blackstone, C. D., Levey, A. I., Huganir, R. L., and Price, D. L. (1993a). AMPA glutamate receptor subunits are differentially distributed in rat brain. Neuroscience 53, 327–358.
Martin, L. J., Blackstone, C. D., Levey, A. I., Huganir, R. L., and Price, D. L. (1993b). Cellular localizations of AMPA glutamate receptors within the basal forebrain magnocellular complex of rat and monkey. J. Neurosci. 13, 2249–2263.
Martin, L. J., Hadfield, M. G., Dellovade, T. L., and Price, D. L. (1991). The striatal mosaic in primates: patterns of neuropeptide immunoreactivity differentiate the ventral striatum from the dorsal striatum. Neuroscience 43, 397–417.
Martone, M. E., Holash, J. A., Bayardo, A., Pasquale, E. B., and Ellisman, M. H. (1997). Immunolocalization of the receptor tyrosine kinase EphA4 in the adult rat central nervous system. Brain Res. 771, 238–250.
Matsuda, W., Furuta, T., Nakamura, K. C., Hioki, H., Fujiyama, F., Arai, R., and Kaneko, T. (2009). Single nigrostriatal dopaminergic neurons form widely spread and highly dense axonal arborizations in the neostriatum. J. Neurosci. 29, 444–453.
Matsumoto, M., and Hikosaka, O. (2007). Lateral habenula as a source of negative reward signals in dopamine neurons. Nature 447, 1111–1115.
Medina, L., Figueredo-Cardenas, G., and Reiner, A. (1996). Differential abundance of superoxide dismutase in interneurons versus projection neurons and in matrix versus striosome neurons in monkey striatum. Brain Res. 708, 59–70.
Menalled, L. B., Sison, J. D., Wu, Y., Olivieri, M., Li, X. J., Li, H., Zeitlin, S., and Chesselet, M. F. (2002). Early motor dysfunction and striosomal distribution of huntingtin microaggregates in Huntington’s disease knock-in mice. J. Neurosci. 22, 8266–8276.
Meurers, B. H., Dziewczapolski, G., Shi, T., Bittner, A., Kamme, F., and Shults, C. W. (2009). Dopamine depletion induces distinct compensatory gene expression changes in DARPP-32 signal transduction cascades of striatonigral and striatopallidal neurons. J. Neurosci. 29, 6828–6839.
Mikula, S., Parrish, S. K., Trimmer, J. S., and Jones, E. G. (2009). Complete 3D visualization of primate striosomes by KChIP1 immunostaining. J. Comp. Neurol. 514, 507–517.
Miura, M., Masuda, M., and Aosaki, T. (2008). Roles of micro-opioid receptors in GABAergic synaptic transmission in the striosome and matrix compartments of the striatum. Mol. Neurobiol. 37, 104–115.
Miura, M., Saino-Saito, S., Masuda, M., Kobayashi, K., and Aosaki, T. (2007). Compartment-specific modulation of GABAergic synaptic transmission by mu-opioid receptor in the mouse striatum with green fluorescent protein-expressing dopamine islands. J. Neurosci. 27, 9721–9728.
Moon Edley, S., and Herkenham, M. (1984). Comparative development of striatal opiate receptors and dopamine revealed by autoradiography and histofluorescence. Brain Res. 305, 27–42.
Moratalla, R., Elibol, B., Vallejo, M., and Graybiel, A. M. (1996). Network-level changes in expression of inducible Fos-Jun proteins in the striatum during chronic cocaine treatment and withdrawal. Neuron 17, 147–156.
Moratalla, R., Quinn, B., Delanney, L. E., Irwin, I., Langston, J. W., and Graybiel, A. M. (1992). Differential vulnerability of primate caudate-putamen and striosome-matrix dopamine systems to the neurotoxic effects of 1-methyl-4-phenyl-1,2,3,6-tetrahydropyridine. Proc. Natl. Acad. Sci. U.S.A. 89, 3859–3863.
Morigaki, R., Sako, W., Okita, S., Kasahara, J., Yokoyama, H., Nagahiro, S., Kaji, R., and Goto, S. (2011). Cyclin-dependent kinase 5 with phosphorylation of serine 159 residue is enriched in striatal matrix compartment in adult mice. Neuroscience 189, 25–31.
Morton, A. J., Nicholson, L. F., and Faull, R. L. (1993). Compartmental loss of NADPH diaphorase in the neuropil of the human striatum in Huntington’s disease. Neuroscience 53, 159–168.
Moss, J., and Bolam, J. P. (2008). A dopaminergic axon lattice in the striatum and its relationship with cortical and thalamic terminals. J. Neurosci. 28, 11221–11230.
Murrin, L. C., and Zeng, W. Y. (1989). Dopamine D1 receptor development in the rat striatum: early localization in striosomes. Brain Res. 480, 170–177.
Napolitano, F., Pasqualetti, M., Usiello, A., Santini, E., Pacini, G., Sciamanna, G., Errico, F., Tassone, A., Di Dato, V., Martella, G., Cuomo, D., Fisone, G., Bernardi, G., Mandolesi, G., Mercuri, N. B., Standaert, D. G., and Pisani, A. (2010). Dopamine D2 receptor dysfunction is rescued by adenosine A2A receptor antagonism in a model of DYT1 dystonia. Neurobiol. Dis. 38, 434–445.
Nastuk, M. A., and Graybiel, A. M. (1985). Patterns of muscarinic cholinergic binding in the striatum and their relation to dopamine islands and striosomes. J. Comp. Neurol. 237, 176–194.
Naumann, M., Pirker, W., Reiners, K., Lange, K. W., Becker, G., and Brucke, T. (1998). Imaging the pre- and postsynaptic side of striatal dopaminergic synapses in idiopathic cervical dystonia: a SPECT study using [123I] epidepride and [123I] beta-CIT. Mov. Disord. 13, 319–323.
Newman-Gage, H., and Graybiel, A. M. (1988). Expression of calcium/calmodulin-dependent protein kinase in relation to dopamine islands and synaptic maturation in the cat striatum. J. Neurosci. 8, 3360–3375.
Nishikawa, S., Goto, S., Hamasaki, T., Ogawa, M., and Ushio, Y. (1999). Transient and compartmental expression of the reeler gene product reelin in the developing rat striatum. Brain Res. 850, 244–248.
Nishikawa, S., Goto, S., Yamada, K., Hamasaki, T., and Ushio, Y. (2003). Lack of Reelin causes malpositioning of nigral dopaminergic neurons: evidence from comparison of normal and Reln(rl) mutant mice. J. Comp. Neurol. 461, 166–173.
Oberlin, S. R., Konakova, M., Pulst, S., and Chesselet, M. F. (2004). Development and anatomic localization of torsinA. Adv. Neurol. 94, 61–65.
Ohmori, T., Abekawa, T., and Koyama, T. (1995). Scopolamine prevents augmentation of stereotypy induced by chronic methamphetamine treatment. Psychopharmacology (Berl.) 121, 158–163.
Olson, L., Seiger, A., and Fuxe, K. (1972). Heterogeneity of striatal and limbic dopamine innervation: highly fluorescent islands in developing and adult rats. Brain Res. 44, 283–288.
Oo, T. F., Ries, V., Cho, J., Kholodilov, N., and Burke, R. E. (2005). Anatomical basis of glial cell line-derived neurotrophic factor expression in the striatum and related basal ganglia during postnatal development of the rat. J. Comp. Neurol. 484, 57–67.
Ozelius, L. J., Hewett, J. W., Page, C. E., Bressman, S. B., Kramer, P. L., Shalish, C., De Leon, D., Brin, M. F., Raymond, D., Corey, D. P., Fahn, S., Risch, N. J., Buckler, A. J., Gusella, J. F., and Breakefield, X. O. (1997). The early-onset torsion dystonia gene (DYT1) encodes an ATP-binding protein. Nat. Genet. 17, 40–48.
Parthasarathy, H. B., and Graybiel, A. M. (1997). Cortically driven immediate-early gene expression reflects modular influence of sensorimotor cortex on identified striatal neurons in the squirrel monkey. J. Neurosci. 17, 2477–2491.
Passante, L., Gaspard, N., Degraeve, M., Frisen, J., Kullander, K., De Maertelaer, V., and Vanderhaeghen, P. (2008). Temporal regulation of ephrin/Eph signalling is required for the spatial patterning of the mammalian striatum. Development 135, 3281–3290.
Perreault, M. L., Hasbi, A., Alijaniaram, M., Fan, T., Varghese, G., Fletcher, P. J., Seeman, P., O’dowd, B. F., and George, S. R. (2010). The dopamine D1-D2 receptor heteromer localizes in dynorphin/enkephalin neurons: increased high affinity state following amphetamine and in schizophrenia. J. Biol. Chem. 285, 36625–36634.
Pert, C. B., Kuhar, M. J., and Snyder, S. H. (1976). Opiate receptor: autoradiographic localization in rat brain. Proc. Natl. Acad. Sci. U.S.A. 73, 3729–3733.
Peterson, D. A., Sejnowski, T. J., and Poizner, H. (2010). Convergent evidence for abnormal striatal synaptic plasticity in dystonia. Neurobiol. Dis. 37, 558–573.
Pierret, P., Mechawar, N., Vallee, A., Patel, J., Priestley, J. V., Dunn, R. J., Dower, N. A., Stone, J. C., and Richardson, P. M. (2002). Presence of Ras guanyl nucleotide-releasing protein in striosomes of the mature and developing rat. Neuroscience 111, 83–94.
Pisani, A., Bonsi, P., Centonze, D., Gubellini, P., Bernardi, G., and Calabresi, P. (2003). Targeting striatal cholinergic interneurons in Parkinson’s disease: focus on metabotropic glutamate receptors. Neuropharmacology 45, 45–56.
Playford, E. D., Fletcher, N. A., Sawle, G. V., Marsden, C. D., and Brooks, D. J. (1993). Striatal [18F]dopa uptake in familial idiopathic dystonia. Brain 116 (Pt 5), 1191–1199.
Politis, M., Pavese, N., Tai, Y. F., Kiferle, L., Mason, S. L., Brooks, D. J., Tabrizi, S. J., Barker, R. A., and Piccini, P. (2011). Microglial activation in regions related to cognitive function predicts disease onset in Huntington’s disease: a multimodal imaging study. Hum. Brain Mapp. 32, 258–270.
Porrino, L. J., Smith, H. R., Nader, M. A., and Beveridge, T. J. (2007). The effects of cocaine: a shifting target over the course of addiction. Prog. Neuropsychopharmacol. Biol. Psychiatry 31, 1593–1600.
Prensa, L., and Parent, A. (2001). The nigrostriatal pathway in the rat: a single-axon study of the relationship between dorsal and ventral tier nigral neurons and the striosome/matrix striatal compartments. J. Neurosci. 21, 7247–7260.
Quartarone, A., Bagnato, S., Rizzo, V., Siebner, H. R., Dattola, V., Scalfari, A., Morgante, F., Battaglia, F., Romano, M., and Girlanda, P. (2003). Abnormal associative plasticity of the human motor cortex in writer’s cramp. Brain 126, 2586–2596.
Quartarone, A., Morgante, F., Sant’angelo, A., Rizzo, V., Bagnato, S., Terranova, C., Siebner, H. R., Berardelli, A., and Girlanda, P. (2008). Abnormal plasticity of sensorimotor circuits extends beyond the affected body part in focal dystonia. J. Neurol. Neurosurg. Psychiatr. 79, 985–990.
Ragsdale, C. W. Jr., and Graybiel, A. M. (1988). Fibers from the basolateral nucleus of the amygdala selectively innervate striosomes in the caudate nucleus of the cat. J. Comp. Neurol. 269, 506–522.
Ragsdale, C. W. Jr., and Graybiel, A. M. (1990). A simple ordering of neocortical areas established by the compartmental organization of their striatal projections. Proc. Natl. Acad. Sci. U.S.A. 87, 6196–6199.
Ragsdale, C. W. Jr., and Graybiel, A. M. (1991). Compartmental organization of the thalamostriatal connection in the cat. J. Comp. Neurol. 311, 134–167.
Rajakumar, N., Elisevich, K., and Flumerfelt, B. A. (1993). Compartmental origin of the striato-entopeduncular projection in the rat. J. Comp. Neurol. 331, 286–296.
Raju, D. V., Shah, D. J., Wright, T. M., Hall, R. A., and Smith, Y. (2006). Differential synaptology of vGluT2-containing thalamostriatal afferents between the patch and matrix compartments in rats. J. Comp. Neurol. 499, 231–243.
Redies, C., Kovjanic, D., Heyers, D., Medina, L., Hirano, S., Suzuki, S. T., and Puelles, L. (2002). Patch/matrix patterns of gray matter differentiation in the telencephalon of chicken and mouse. Brain Res. Bull. 57, 489–493.
Reiner, A., Albin, R. L., Anderson, K. D., D’amato, C. J., Penney, J. B., and Young, A. B. (1988). Differential loss of striatal projection neurons in Huntington disease. Proc. Natl. Acad. Sci. U.S.A. 85, 5733–5737.
Reiner, A., Hart, N. M., Lei, W., and Deng, Y. (2010). Corticostriatal projection neurons – dichotomous types and dichotomous functions. Front. Neuroanat. 4:142. doi: 10.3389/fnana.2010.00142
Reiner, A., Jiao, Y., Del Mar, N., Laverghetta, A. V., and Lei, W. L. (2003). Differential morphology of pyramidal tract-type and intratelencephalically projecting-type corticostriatal neurons and their intrastriatal terminals in rats. J. Comp. Neurol. 457, 420–440.
Rivera, A., Cuellar, B., Giron, F. J., Grandy, D. K., De La Calle, A., and Moratalla, R. (2002). Dopamine D4 receptors are heterogeneously distributed in the striosomes/matrix compartments of the striatum. J. Neurochem. 80, 219–229.
Roberts, R. C., Roche, J. K., and Conley, R. R. (2005). Synaptic differences in the patch matrix compartments of subjects with schizophrenia: a postmortem ultrastructural study of the striatum. Neurobiol. Dis. 20, 324–335.
Robinson, T. E., and Berridge, K. C. (2008). Review. The incentive sensitization theory of addiction: some current issues. Philos. Trans. R. Soc. Lond. B Biol. Sci. 363, 3137–3146.
Rosas, H. D., Salat, D. H., Lee, S. Y., Zaleta, A. K., Hevelone, N., and Hersch, S. M. (2008). Complexity and heterogeneity: what drives the ever-changing brain in Huntington’s disease? Ann. N. Y. Acad. Sci. 1147, 196–205.
Roze, E., Betuing, S., Deyts, C., Marcon, E., Brami-Cherrier, K., Pages, C., Humbert, S., Merienne, K., and Caboche, J. (2008). Mitogen- and stress-activated protein kinase-1 deficiency is involved in expanded-huntingtin-induced transcriptional dysregulation and striatal death. FASEB J. 22, 1083–1093.
Roze, E., Soumare, A., Pironneau, I., Sangla, S., De Cock, V. C., Teixeira, A., Astorquiza, A., Bonnet, C., Bleton, J. P., Vidailhet, M., and Elbaz, A. (2009). Case-control study of writer’s cramp. Brain 132, 756–764.
Russo, S. J., Dietz, D. M., Dumitriu, D., Morrison, J. H., Malenka, R. C., and Nestler, E. J. (2010). The addicted synapse: mechanisms of synaptic and structural plasticity in nucleus accumbens. Trends Neurosci. 33, 267–276.
Sadikot, A. F., Parent, A., Smith, Y., and Bolam, J. P. (1992). Efferent connections of the centromedian and parafascicular thalamic nuclei in the squirrel monkey: a light and electron microscopic study of the thalamostriatal projection in relation to striatal heterogeneity. J. Comp. Neurol. 320, 228–242.
Saka, E., Elibol, B., Erdem, S., and Dalkara, T. (1999). Compartmental changes in expression of c-Fos and FosB proteins in intact and dopamine-depleted striatum after chronic apomorphine treatment. Brain Res. 825, 104–114.
Saka, E., Goodrich, C., Harlan, P., Madras, B. K., and Graybiel, A. M. (2004). Repetitive behaviors in monkeys are linked to specific striatal activation patterns. J. Neurosci. 24, 7557–7565.
Saka, E., Iadarola, M., Fitzgerald, D. J., and Graybiel, A. M. (2002). Local circuit neurons in the striatum regulate neural and behavioral responses to dopaminergic stimulation. Proc. Natl. Acad. Sci. U.S.A. 99, 9004–9009.
Sako, W., Morigaki, R., Kaji, R., Tooyama, I., Okita, S., Kitazato, K., Nagahiro, S., Graybiel, A. M., and Goto, S. (2011). Identification and localization of neuron-specific isoform of TAF1 in rat brain: implications for neuropathology of DYT3 dystonia. Neuroscience 189, 100–107.
Sako, W., Morigaki, R., Nagahiro, S., Kaji, R., and Goto, S. (2010). Olfactory type G-protein alpha subunit in striosome-matrix dopamine systems in adult mice. Neuroscience 170, 497–502.
Sandell, J. H., Graybiel, A. M., and Chesselet, M. F. (1986). A new enzyme marker for striatal compartmentalization: NADPH diaphorase activity in the caudate nucleus and putamen of the cat. J. Comp. Neurol. 243, 326–334.
Santini, E., Alcacer, C., Cacciatore, S., Heiman, M., Herve, D., Greengard, P., Girault, J. A., Valjent, E., and Fisone, G. (2009). L-DOPA activates ERK signaling and phosphorylates histone H3 in the striatonigral medium spiny neurons of hemiparkinsonian mice. J. Neurochem. 108, 621–633.
Santini, E., Sgambato-Faure, V., Li, Q., Savasta, M., Dovero, S., Fisone, G., and Bezard, E. (2010). Distinct changes in cAMP and extracellular signal-regulated protein kinase signalling in L-DOPA-induced dyskinesia. PLoS ONE 5, e12322. doi: 10.1371/journal.pone.0012322
Santini, E., Valjent, E., Usiello, A., Carta, M., Borgkvist, A., Girault, J. A., Herve, D., Greengard, P., and Fisone, G. (2007). Critical involvement of cAMP/DARPP-32 and extracellular signal-regulated protein kinase signaling in L-DOPA-induced dyskinesia. J. Neurosci. 27, 6995–7005.
Sato, K., Kaji, R., Matsumoto, S., Nagahiro, S., and Goto, S. (2007). Compartmental loss of striatal medium spiny neurons in multiple system atrophy of parkinsonian type. Mov. Disord. 22, 2365–2370.
Sato, K., Sumi-Ichinose, C., Kaji, R., Ikemoto, K., Nomura, T., Nagatsu, I., Ichinose, H., Ito, M., Sako, W., Nagahiro, S., Graybiel, A. M., and Goto, S. (2008). Differential involvement of striosome and matrix dopamine systems in a transgenic model of dopa-responsive dystonia. Proc. Natl. Acad. Sci. U.S.A. 105, 12551–12556.
Schiffmann, S. N., and Vanderhaeghen, J. J. (1992). Ontogeny of gene expression of adenosine A2 receptor in the striatum: early localization in the patch compartment. J. Comp. Neurol. 317, 117–128.
Schmidt, A., Jabusch, H. C., Altenmuller, E., Hagenah, J., Bruggemann, N., Lohmann, K., Enders, L., Kramer, P. L., Saunders-Pullman, R., Bressman, S. B., Munchau, A., and Klein, C. (2009). Etiology of musician’s dystonia: familial or environmental? Neurology 72, 1248–1254.
Schoen, S. W., and Graybiel, A. M. (1992). 5′-nucleotidase: a new marker for striosomal organization in the rat caudoputamen. J. Comp. Neurol. 322, 566–576.
Segawa, M. (2011). Hereditary progressive dystonia with marked diurnal fluctuation. Brain Dev. 33, 195–201.
Seto-Ohshima, A., Emson, P. C., Lawson, E., Mountjoy, C. Q., and Carrasco, L. H. (1988). Loss of matrix calcium-binding protein-containing neurons in Huntington’s disease. Lancet 1, 1252–1255.
Shashidharan, P., Kramer, B. C., Walker, R. H., Olanow, C. W., and Brin, M. F. (2000). Immunohistochemical localization and distribution of torsinA in normal human and rat brain. Brain Res. 853, 197–206.
Shi, X., and Mcginty, J. F. (2006). Extracellular signal-regulated mitogen-activated protein kinase inhibitors decrease amphetamine-induced behavior and neuropeptide gene expression in the striatum. Neuroscience 138, 1289–1298.
Shulman, J. M., De Jager, P. L., and Feany, M. B. (2011). Parkinson’s disease: genetics and pathogenesis. Annu. Rev. Pathol. 6, 193–222.
Singleton, A. B., Farrer, M., Johnson, J., Singleton, A., Hague, S., Kachergus, J., Hulihan, M., Peuralinna, T., Dutra, A., Nussbaum, R., Lincoln, S., Crawley, A., Hanson, M., Maraganore, D., Adler, C., Cookson, M. R., Muenter, M., Baptista, M., Miller, D., Blancato, J., Hardy, J., and Gwinn-Hardy, K. (2003). alpha-Synuclein locus triplication causes Parkinson’s disease. Science 302, 841.
Sinha, R. (2008). Chronic stress, drug use, and vulnerability to addiction. Ann. N. Y. Acad. Sci. 1141, 105–130.
Somogyi, P., Bolam, J. P., Totterdell, S., and Smith, A. D. (1981). Monosynaptic input from the nucleus accumbens – ventral striatum region to retrogradely labelled nigrostriatal neurones. Brain Res. 217, 245–263.
Stack, E. C., Dedeoglu, A., Smith, K. M., Cormier, K., Kubilus, J. K., Bogdanov, M., Matson, W. R., Yang, L., Jenkins, B. G., Luthi-Carter, R., Kowall, N. W., Hersch, S. M., Beal, M. F., and Ferrante, R. J. (2007). Neuroprotective effects of synaptic modulation in Huntington’s disease R6/2 mice. J. Neurosci. 27, 12908–12915.
Sun, Z., Wang, H. B., Deng, Y. P., Lei, W. L., Xie, J. P., Meade, C. A., Del Mar, N., Goldowitz, D., and Reiner, A. (2005). Increased calbindin-D28k immunoreactivity in striatal projection neurons of R6/2 Huntington’s disease transgenic mice. Neurobiol. Dis. 20, 907–917.
Surmeier, D. J., Song, W. J., and Yan, Z. (1996). Coordinated expression of dopamine receptors in neostriatal medium spiny neurons. J. Neurosci. 16, 6579–6591.
Takahashi, K., Liu, F. C., Hirokawa, K., and Takahashi, H. (2003). Expression of Foxp2, a gene involved in speech and language, in the developing and adult striatum. J. Neurosci. Res. 73, 61–72.
Takahashi, K., Liu, F. C., Oishi, T., Mori, T., Higo, N., Hayashi, M., Hirokawa, K., and Takahashi, H. (2008). Expression of FOXP2 in the developing monkey forebrain: comparison with the expression of the genes FOXP1, PBX3, and MEIS2. J. Comp. Neurol. 509, 180–189.
Tamura, Y., Ueki, Y., Lin, P., Vorbach, S., Mima, T., Kakigi, R., and Hallett, M. (2009). Disordered plasticity in the primary somatosensory cortex in focal hand dystonia. Brain 132, 749–755.
Tan, A., Moratalla, R., Lyford, G. L., Worley, P., and Graybiel, A. M. (2000). The activity-regulated cytoskeletal-associated protein arc is expressed in different striosome-matrix patterns following exposure to amphetamine and cocaine. J. Neurochem. 74, 2074–2078.
Tanabe, L. M., Kim, C. E., Alagem, N., and Dauer, W. T. (2009). Primary dystonia: molecules and mechanisms. Nat. Rev. Neurol. 5, 598–609.
Tang, T. S., Chen, X., Liu, J., and Bezprozvanny, I. (2007). Dopaminergic signaling and striatal neurodegeneration in Huntington’s disease. J. Neurosci. 27, 7899–7910.
Tanimura, Y., King, M. A., Williams, D. K., and Lewis, M. H. (2011). Development of repetitive behavior in a mouse model: roles of indirect and striosomal basal ganglia pathways. Int. J. Dev. Neurosci. 29, 461–467.
Tappe, A., and Kuner, R. (2006). Regulation of motor performance and striatal function by synaptic scaffolding proteins of the Homer1 family. Proc. Natl. Acad. Sci. U.S.A. 103, 774–779.
The Huntington’s Disease Collaborative Research Group. (1993). A novel gene containing a trinucleotide repeat that is expanded and unstable on Huntington’s disease chromosomes. The Huntington’s Disease Collaborative Research Group. Cell 72, 971–983.
Thony, B., and Blau, N. (1997). Mutations in the GTP cyclohydrolase I and 6-pyruvoyl-tetrahydropterin synthase genes. Hum. Mutat. 10, 11–20.
Thony, B., and Blau, N. (2006). Mutations in the BH4-metabolizing genes GTP cyclohydrolase I, 6-pyruvoyl-tetrahydropterin synthase, sepiapterin reductase, carbinolamine-4a-dehydratase, and dihydropteridine reductase. Hum. Mutat. 27, 870–878.
Thorn, C. A., Atallah, H., Howe, M., and Graybiel, A. M. (2010). Differential dynamics of activity changes in dorsolateral and dorsomedial striatal loops during learning. Neuron 66, 781–795.
Threlfell, S., and Cragg, S. J. (2011). Dopamine signaling in dorsal versus ventral striatum: the dynamic role of cholinergic interneurons. Front. Syst. Neurosci. 5:11. doi: 10.3389/fnsys.2011.00011
Thu, D. C., Oorschot, D. E., Tippett, L. J., Nana, A. L., Hogg, V. M., Synek, B. J., Luthi-Carter, R., Waldvogel, H. J., and Faull, R. L. (2010). Cell loss in the motor and cingulate cortex correlates with symptomatology in Huntington’s disease. Brain 133, 1094–1110.
Tippett, L. J., Waldvogel, H. J., Thomas, S. J., Hogg, V. M., Van Roon-Mom, W., Synek, B. J., Graybiel, A. M., and Faull, R. L. (2007). Striosomes and mood dysfunction in Huntington’s disease. Brain 130, 206–221.
Toki, S., Kawasaki, H., Tashiro, N., Housman, D. E., and Graybiel, A. M. (2001). Guanine nucleotide exchange factors CalDAG-GEFI and CalDAG-GEFII are colocalized in striatal projection neurons. J. Comp. Neurol. 437, 398–407.
Tokuno, H., Chiken, S., Kametani, K., and Moriizumi, T. (2002). Efferent projections from the striatal patch compartment: anterograde degeneration after selective ablation of neurons expressing mu-opioid receptor in rats. Neurosci. Lett. 332, 5–8.
Trytek, E. S., White, I. M., Schroeder, D. M., Heidenreich, B. A., and Rebec, G. V. (1996). Localization of motor- and nonmotor-related neurons within the matrix-striosome organization of rat striatum. Brain Res. 707, 221–227.
Turner, B. H., Wilson, J. S., Mckenzie, J. C., and Richtand, N. (1988). MPTP produces a pattern of nigrostriatal degeneration which coincides with the mosaic organization of the caudate nucleus. Brain Res. 473, 60–64.
Valjent, E., Bertran-Gonzalez, J., Herve, D., Fisone, G., and Girault, J. A. (2009). Looking BAC at striatal signaling: cell-specific analysis in new transgenic mice. Trends Neurosci. 32, 538–547.
Valjent, E., Pascoli, V., Svenningsson, P., Paul, S., Enslen, H., Corvol, J. C., Stipanovich, A., Caboche, J., Lombroso, P. J., Nairn, A. C., Greengard, P., Herve, D., and Girault, J. A. (2005). Regulation of a protein phosphatase cascade allows convergent dopamine and glutamate signals to activate ERK in the striatum. Proc. Natl. Acad. Sci. U.S.A. 102, 491–496.
Van Der Kooy, D., and Fishell, G. (1992). Embryonic lesions of the substantia nigra prevent the patchy expression of opiate receptors, but not the segregation of patch and matrix compartment neurons, in the developing rat striatum. Brain Res. Dev. Brain Res. 66, 141–145.
Vanderschuren, L. J., Schoffelmeer, A. N., Van Leeuwen, S. D., Hof, L., Jonker, A. J., and Voorn, P. (2002). Compartment-specific changes in striatal neuronal activity during expression of amphetamine sensitization are the result of drug hypersensitivity. Eur. J. Neurosci. 16, 2462–2468.
Vezina, P., and Leyton, M. (2009). Conditioned cues and the expression of stimulant sensitization in animals and humans. Neuropharmacology 56(Suppl. 1), 160–168.
Villares, J. (2007). Chronic use of marijuana decreases cannabinoid receptor binding and mRNA expression in the human brain. Neuroscience 145, 323–334.
Vitek, J. L., Chockkan, V., Zhang, J. Y., Kaneoke, Y., Evatt, M., Delong, M. R., Triche, S., Mewes, K., Hashimoto, T., and Bakay, R. A. (1999). Neuronal activity in the basal ganglia in patients with generalized dystonia and hemiballismus. Ann. Neurol. 46, 22–35.
Volkow, N. D., Wang, G. J., Telang, F., Fowler, J. S., Logan, J., Childress, A. R., Jayne, M., Ma, Y., and Wong, C. (2006). Cocaine cues and dopamine in dorsal striatum: mechanism of craving in cocaine addiction. J. Neurosci. 26, 6583–6588.
Voon, V., Fernagut, P. O., Wickens, J., Baunez, C., Rodriguez, M., Pavon, N., Juncos, J. L., Obeso, J. A., and Bezard, E. (2009). Chronic dopaminergic stimulation in Parkinson’s disease: from dyskinesias to impulse control disorders. Lancet Neurol. 8, 1140–1149.
Walker, R. H., Arbuthnott, G. W., Baughman, R. W., and Graybiel, A. M. (1993). Dendritic domains of medium spiny neurons in the primate striatum: relationships to striosomal borders. J. Comp. Neurol. 337, 614–628.
Wang, H., and Pickel, V. M. (1998). Dendritic spines containing mu-opioid receptors in rat striatal patches receive asymmetric synapses from prefrontal corticostriatal afferents. J. Comp. Neurol. 396, 223–237.
Wang, H. B., Deng, Y. P., and Reiner, A. (2007). In situ hybridization histochemical and immunohistochemical evidence that striatal projection neurons co-containing substance P and enkephalin are overrepresented in the striosomal compartment of striatum in rats. Neurosci. Lett. 425, 195–199.
Wang, H. B., Laverghetta, A. V., Foehring, R., Deng, Y. P., Sun, Z., Yamamoto, K., Lei, W. L., Jiao, Y., and Reiner, A. (2006a). Single-cell RT-PCR, in situ hybridization histochemical, and immunohistochemical studies of substance P and enkephalin co-occurrence in striatal projection neurons in rats. J. Chem. Neuroanat. 31, 178–199.
Wang, Z., Kai, L., Day, M., Ronesi, J., Yin, H. H., Ding, J., Tkatch, T., Lovinger, D. M., and Surmeier, D. J. (2006b). Dopaminergic control of corticostriatal long-term synaptic depression in medium spiny neurons is mediated by cholinergic interneurons. Neuron 50, 443–452.
Wang, J. Q., Daunais, J. B., and Mcginty, J. F. (1994). NMDA receptors mediate amphetamine-induced upregulation of zif/268 and preprodynorphin mRNA expression in rat striatum. Synapse 18, 343–353.
Ward, R. P., and Dorsa, D. M. (1996). Colocalization of serotonin receptor subtypes 5-HT2A, 5-HT2C, and 5-HT6 with neuropeptides in rat striatum. J. Comp. Neurol. 370, 405–414.
Westin, J. E., Vercammen, L., Strome, E. M., Konradi, C., and Cenci, M. A. (2007). Spatiotemporal pattern of striatal ERK1/2 phosphorylation in a rat model of L-DOPA-induced dyskinesia and the role of dopamine D1 receptors. Biol. Psychiatry 62, 800–810.
White, N. M., and Hiroi, N. (1998). Preferential localization of self-stimulation sites in striosomes/patches in the rat striatum. Proc. Natl. Acad. Sci. U.S.A. 95, 6486–6491.
Wilson, C. J. (1987). Morphology and synaptic connections of crossed corticostriatal neurons in the rat. J. Comp. Neurol. 263, 567–580.
Xu, M., Moratalla, R., Gold, L. H., Hiroi, N., Koob, G. F., Graybiel, A. M., and Tonegawa, S. (1994). Dopamine D1 receptor mutant mice are deficient in striatal expression of dynorphin and in dopamine-mediated behavioral responses. Cell 79, 729–742.
Yamada, T., Mcgeer, P. L., Baimbridge, K. G., and Mcgeer, E. G. (1990). Relative sparing in Parkinson’s disease of substantia nigra dopamine neurons containing calbindin-D28K. Brain Res. 526, 303–307.
Yin, H. H., Knowlton, B. J., and Balleine, B. W. (2004). Lesions of dorsolateral striatum preserve outcome expectancy but disrupt habit formation in instrumental learning. Eur. J. Neurosci. 19, 181–189.
Yin, H. H., Ostlund, S. B., and Balleine, B. W. (2008). Reward-guided learning beyond dopamine in the nucleus accumbens: the integrative functions of cortico-basal ganglia networks. Eur. J. Neurosci. 28, 1437–1448.
Yung, K. K., Smith, A. D., Levey, A. I., and Bolam, J. P. (1996). Synaptic connections between spiny neurons of the direct and indirect pathways in the neostriatum of the rat: evidence from dopamine receptor and neuropeptide immunostaining. Eur. J. Neurosci. 8, 861–869.
Zachariou, V., Sgambato-Faure, V., Sasaki, T., Svenningsson, P., Berton, O., Fienberg, A. A., Nairn, A. C., Greengard, P., and Nestler, E. J. (2006). Phosphorylation of DARPP-32 at Threonine-34 is required for cocaine action. Neuropsychopharmacology 31, 555–562.
Zahm, D. S. (1991). Compartments in rat dorsal and ventral striatum revealed following injection of 6-hydroxydopamine into the ventral mesencephalon. Brain Res. 552, 164–169.
Keywords: striatum, medium spiny neuron, Huntington’s disease, Parkinson’s disease, dystonia, dyskinesia, substantia nigra, CalDAG-GEF
Citation: Crittenden JR and Graybiel AM (2011) Basal ganglia disorders associated with imbalances in the striatal striosome and matrix compartments. Front. Neuroanat. 5:59. doi: 10.3389/fnana.2011.00059
Received: 14 June 2011; Paper pending published: 11 July 2011;
Accepted: 18 August 2011; Published online: 07 September 2011.
Edited by:
Emmanuel Valjent, Institut de Génomique Fonctionelle, INSERM U661, UMR 5203 CNRS, Universite Montpellier 1 & 2, FranceReviewed by:
Ricardo Insausti, University of Castilla la Mancha, SpainJames M. Tepper, Rutgers The State University of New Jersey, USA
Copyright: © 2011 Crittenden and Graybiel. This is an open-access article subject to a non-exclusive license between the authors and Frontiers Media SA, which permits use, distribution and reproduction in other forums, provided the original authors and source are credited and other Frontiers conditions are complied with.
*Correspondence: Jill R. Crittenden, McGovern Institute for Brain Research, Massachusetts Institute of Technology, 46-6133, 77 Massachusetts Avenue, Cambridge, MA 02139, USA e-mail:anJjQG1pdC5lZHU=