- Neuroscience Program, Department of Psychology, University of Michigan, Ann Arbor, MI, USA
Striatal fast-spiking interneurons (FSIs) have a major influence over behavioral output, and a deficit in these cells has been observed in dystonia and Tourette syndrome. FSIs receive cortical input, are coupled together by gap junctions, and make perisomatic GABAergic synapses onto many nearby projection neurons. Despite being critical components of striatal microcircuits, until recently little was known about FSI activity in behaving animals. Striatal FSIs are near-continuously active in awake rodents, but even neighboring FSIs show uncorrelated activity most of the time. A coordinated “pulse” of increased FSI firing occurs throughout striatum when rats initiate one chosen action while suppressing a highly trained alternative. This pulse coincides with a drop in globus pallidus population activity, suggesting that pallidostriatal disinhibition may have a important role in timing or coordinating action execution. In addition to changes in firing rate, FSIs show behavior-linked modulation of spike timing. The variability of inter-spike intervals decreases markedly following instruction cues, and FSIs also participate in fast striatal oscillations that are linked to rewarding events and dopaminergic drugs. These studies have revealed novel and unexpected properties of FSIs, that should help inform new models of striatal information processing in both normal and aberrant conditions.
Introduction
The striatum is the largest component of basal ganglia circuitry, and the primary location at which information is passed into those circuits from cortex and thalamus. Alterations in striatal physiology are implicated in a wide range of neurological and psychiatric disorders from Parkinson’s Disease to drug addiction. Although controversies continue concerning the precise normal behavioral functions of striatum (and basal ganglia more generally; Turner and Desmurget, 2010), most investigators think that it is critical for adaptive decision-making: the refinement, selection, and energization of behaviors through trial-and-error learning (Berke, 2009b). Understanding exactly what computational algorithms are achieved by striatum – and how – is a major ongoing challenge in systems neuroscience. Fast-spiking interneurons (FSIs) are relatively sparse elements of striatal networks that nonetheless seem to be critical nodes governing and orchestrating striatal output. In this brief review I first summarize key anatomical and physiological properties of these cells that are thought to be key to their functional properties, before considering some of the quite surprising observations from a series of recent studies examining their firing patterns in awake, behaving animals.
FSI Anatomy and Connectivity
Striatal FSIs are GABAergic cells that express the calcium-binding protein parvalbumin, and in most respects are similar to FSIs in other structures such as cortex. They comprise roughly 1% of striatal neurons (Luk and Sadikot, 2001), scattered throughout the structure but with a strong lateral > medial gradient (Gerfen et al., 1985). This distribution is not found for other interneuron types and may indicate a particularly important role for FSIs in sensorimotor (lateral) striatum. Compared to nearby medium-spiny projection neurons (MSNs), FSIs receive convergent inputs from a wider range of distinct cortical regions, suggestive of a role in sensorimotor integration (Ramanathan et al., 2002). However, while MSNs receive very few synapses from each of a large number of different corticostriatal axons (Kincaid et al., 1998), FSIs receive more numerous inputs from each of a smaller number of afferents (Bennett and Bolam, 1994). An important unresolved question is whether the cortical cells that project to FSIs are somehow distinct to other corticostriatal neurons. FSIs appear to be more sensitive to cortical inputs than MSNs – for example, focal stimulation of cortex provokes an immediate-early gene response in FSIs over a broader expanse of striatum than MSNs (Parthasarathy and Graybiel, 1997). FSIs connect to each other via both chemical synapses and gap junctions on their dendrites (Kita et al., 1990; Fukuda, 2009), with the resulting electrical meshwork presumably coordinating FSI activity in some fashion. FSIs each make GABAA-mediated synapses onto hundreds of surrounding MSNs, largely onto the somatic region where they can have a powerful influence over MSN spike initiation. By contrast, MSN–MSN GABAergic synapses tend to be distal to the cell body and individually weaker (Koos et al., 2004; Gustafson et al., 2006), albeit larger in number, and MSNs do not provide reciprocal connections onto FSIs (Chuhma et al., 2011). These anatomical features quite reasonably led to the view that FSIs serve a broad, coordinated function, rather than participating in the rich details of striatal information processing (e.g., Plenz, 2003). Finally, FSIs receive an unusual back-projection from globus pallidus (GP; Bevan et al., 1998), that is quite divergent – i.e., small portions of pallidum can affect large areas of striatum (Rajakumar et al., 1994; Spooren et al., 1996).
FSI Physiology in Culture and Slice Preparations
Fast-spiking interneurons have similar properties throughout striatum, including nucleus accumbens (Taverna et al., 2007). As is the case for cortical FSIs, striatal FSIs have distinctive very brief waveforms (Kawaguchi, 1993) and can fire spikes at substantially higher rates than MSNs (e.g., Plenz and Kitai, 1998). Even with steady input they can fire in a variety of patterns, including both single spiking and irregular high-frequency bursts (“stuttering”). Such bursts are typically preceded by gamma-band fluctuations in membrane potential, and reflect intrinsic resonance of FSIs at gamma frequencies (Bracci et al., 2003). This is potentially important, as in neocortical and hippocampal circuits FSI-mediated gamma frequency inhibition is critical for organizing projection cells into functional cell assemblies (Buzsáki, 2006). Although it is not yet clear if similar mechanisms operate in striatum (see below), in vitro FSIs seem to be especially likely to participate in striatal cell assemblies (Carrillo-Reid et al., 2008), that arise even without patterned afferent input.
Important advances by Koos and colleagues verified that FSIs form functional electrical connections with each other, and that even single FSI spikes can potently delay or abolish MSN spiking evoked by current injection (Koos and Tepper, 1999). FSIs appear to influence somewhere between 25 and 75% of MSNs within several hundred microns of their cell body, and to preferentially contact striatonigral (D1+) MSNs (Gittis et al., 2010), although striatopallidal (D2+) MSNs are also targets (Planert et al., 2010). Based on a detailed examination of MSN membrane voltage, Wilson (2009) has argued that FSI inputs are likely responsible for rapid fluctuations that determine the precise time course of MSN spiking.
FSI activity in slices is affected by major neuromodulatory systems, acting through both pre- and post-synaptic receptors. Dopamine increases FSI activity by depolarization (mediated via D5 receptors) while also reducing GABAergic input onto these cells (via pre-synaptic D2 receptors; Centonze et al., 2003). Serotonin also appears to excite FSIs (Blomeley and Bracci, 2009). Acetylcholine seems to exert a more mixed effect, directly depolarizing FSIs (via nicotinic receptors) while reducing their influence on MSNs (via pre-synaptic muscarinic receptors on FSI terminals; Koos and Tepper, 2002). FSIs can show synaptic plasticity at multiple time scales, including strong short-term depression of their synapses onto MSNs (Plenz and Kitai, 1998; Koos et al., 2004; Gittis et al., 2010; Planert et al., 2010) and spike-timing-dependent LTP/LTD at their own inputs (e.g., Fino et al., 2008). FSI-mediated control of MSN spike timing likely also has a strong influence over synaptic change at MSN inputs – differences in GABAergic transmission may be a key factor behind discrepant observations of Hebbian and anti-Hebbian corticostriatal plasticity (Fino et al., 2005; Shen et al., 2008).
FSI Physiology in Anesthetized Animals
Studies in urethane-anesthetized rats have provided an important bridge between in vitro investigations of FSIs and behavioral experiments. Using a juxtacellular labeling approach, Mallet et al. (2005, 2006) confirmed that all extracellularly recorded neurons with very brief waveforms are parvalbumin-positive, and that these cells can readily respond to cortical stimulation by firing bursts with very short inter-spike intervals (2–3 ms). This FSI response appears to mediate powerful feed-forward inhibition, that can constrain the temporal response of MSNs to cortical input. For reasons that are not yet fully clear, such shaping of MSN spike timing was altered following dopamine depletion – even though the spontaneous firing rate of FSIs was not altered, striatopallidal MSNs tended to have less precise evoked responses. In both normal and dopamine-depleted animals, MSNs were more spontaneously active when the cortex was undergoing slow-wave oscillations compared to tail pinch-induced cortical desynchronization, while FSIs showed the opposite pattern – evidence of a broadly reciprocal relationship between MSN and FSI activity, at least on relatively slow timescales.
Identifying FSIs in Freely Moving Animals
There are inherent uncertainties in trying to distinguish different (chemically defined) subpopulations of neurons using extracellular recording alone, and historically studies of striatum in behaving animals have not attempted to do so. The main exception has been monkey studies that contrast “phasically active” (presumed MSNs) with “tonically active” neurons (TANs). TANs are thought to be cholinergic interneurons as they show regular firing and long-duration waveforms, which are properties of rat cholinergic interneurons studied under anesthesia (Wilson et al., 1990). Beginning in 2004 my colleagues and I reported (Berke et al., 2004) that there are also clearly distinguishable subgroups of rat striatal neurons, including a set of neurons that are near-continuously (i.e., tonically) active. However, rather than resembling TANs these active cells had very brief waveforms, fired high-frequency bursts during natural sleep or high-voltage spindle oscillations, and showed a graded distribution, being more prevalent in lateral striatum. Taken together these properties provide strong evidence that these cells are the parvalbumin-positive FSIs. The basic distinction between a larger population of presumed MSNs that are typically silent most of the time, and a smaller population of presumed FSIs that have very brief waveforms and are near-continuously active is also clear in our subsequent studies using different data sets in rats (e.g., Gage et al., 2010; Wiltschko et al., 2010), or mice (Gittis et al., submitted) and in the work of other groups (e.g., Schmitzer-Torbert and Redish, 2008; Lansink et al., 2010; Morra et al., 2010). Not all rodent striatal cells easily fall into these two classes – for example, some active cells seem to have a characteristic intermediate-duration waveform and consistent behavioral responses (Berke, 2008) and may be another class of GABAergic interneuron (perhaps the somatostatin-positive cells). Also, a somewhat puzzling observation is that a very low proportion of rat striatal cells resemble monkey TANs based on waveform and firing pattern. The actual proportion of cholinergic neurons is not known to be substantially different between the two species, although there are differences in cholinergic cell shape (Yelnick et al., 1993). Conversely there have been no descriptions as yet of FSIs in primate extracellular recordings, and this seems to reflect experimental methods more than real species differences (Wu and Parent, 2000). Nonetheless, the results of recent investigations lead to some recommendations. Firstly, given that FSIs are tonically active in awake animals, the term “TANs” in monkeys is a confusing misnomer (as has been pointed out before, for other reasons – Bennett and Wilson, 2003) and should perhaps be dropped in favor of “presumed cholinergic interneurons.” Secondly, the strong tendency of presumed FSIs to transition to high-frequency bursting during natural slow-wave sleep seems to be a particularly useful criterion for distinguishing them (Berke, 2008), and so routinely recording during sleep is highly valuable. These FSI bursts, which resemble the intrinsic stuttering mode of FSIs (Golomb et al. 2007, Klaus et al. 2011), do not occur on every cycle of cortical slow-waves, and are not usually synchronized between neighboring cells (Berke et al. 2004).
FSI Activity during Awake and Drug-Induced Behavioral States
Overall, FSIs tend to have higher firing rates in awake animals (typically 10–30 Hz) than during natural slow-wave sleep, while the reverse tends to be true for MSNs (unpublished observations). This crude FSI/MSN reciprocity corresponds well to the prior studies under anesthesia discussed above. By contrast, at least two major FSI properties expected from the prior literature were not observed. Despite their gap-junction connectivity FSIs do not seem to show millisecond-scale synchronization in awake animals (Berke, 2008) – even when neighboring FSIs are recorded from the same tetrode. A subsequent modeling study of the striatal FSI network (Hjorth et al., 2009) found that the dominant effect of gap junctions is to lower firing rates by shunting current between cells, and it is only under conditions of very high input synchrony that gap junctions promote synchrony of spike outputs. It appears that FSI inputs are not normally sufficiently synchronous in awake animals, with the possible exception of intermittent states like high-voltage spindles. In addition, we found no evidence for rapid FSI inhibition of MSNs, using cross-correlograms to plot MSN spike probability around FSI spike times (Gage et al., 2010). Although cross-correlograms are not an highly sensitive tool, especially with low spike counts, in the same experiments we were readily able to see rapid FSI inhibition of nearby projection cells in motor cortex. This difference was unexpected given the strong brain slice evidence that striatal FSIs control MSN spike times, and clearly needs to be investigated further. As it stands, it appears that not to be the case that each FSI spike provokes synchronous moments of silence in the surrounding volume of MSNs. One factor that is likely relevant is that in awake animals MSNs usually have relatively depolarized membrane potentials (Mahon et al., 2006) that are close to the chloride reversal potential – although FSI GABA release should still tend to reduce MSN spike probability under these conditions. Another potential factor is that the recent spiking history of MSNs and FSIs can alter synaptic actions – for example through short-term depression – leading to strong interactions only under specific behavioral conditions (Fujisawa et al., 2008). Certainly it was anticipated that FSI action on MSNs would be more complex than simple inhibition (Plenz, 2003; Bracci and Panzeri, 2006), and this has now been observed in large-scale striatal network simulations in which removing FSIs actually results in lowered overall MSN firing (Humphries et al., 2009).
Some major classes of psychoactive drugs achieve their behavioral effects largely through actions in striatum, and so administering these drugs in striatal recording studies offers both a means of manipulating striatal circuitry, and possible insights into how such drugs operate at the circuit level. Wiltschko et al. (2010) gave rats systemic injections of either the stimulant amphetamine or a D2 antagonist (an antipsychotic), and compared the effects on MSNs and FSIs. As seen in previous investigations in behaving animals (Rebec, 2006) the effects on MSNs were highly variable. Even though the two drugs respectively stimulated, and suppressed, psychomotor activation, in each case many MSNs increased firing rate compared to control saline injections, and many decreased. The story for FSIs was very different – all FSIs were suppressed by the D2 antagonist, while following amphetamine most FSIs increased firing rate (and none decreased activity). This far more uniform response confirms that the presumed FSIs are a functionally distinct population of striatal neurons, and shows that their influence over striatal output is not reflected simply by reciprocal firing to the overall MSN population. Interestingly, FSIs also have distinct drug responses to MSNs at the level of nuclear signaling – amphetamine causes phosphorylation of the transcriptional regulation MeCP2 selectively in ventral striatal FSIs, and the level of phosphorylation correlates with behavioral sensitization (Deng et al., 2010). The specific mechanisms leading to drug-altered FSI activity are not trivial to determine (see discussion in Wiltschko et al., 2010). Perhaps the most straightforward possibilities are that amphetamine-induced dopamine increases produce depolarization of FSIs via D1-type receptors, while the D2 antagonist reduces FSI spiking by increasing GABAergic transmission onto FSIs, due to a combination of enhanced pallidostriatal activity (Billings and Marshall, 2003) and reduced autoreceptor function at the corresponding GABAergic terminals in striatum. In a related pharmacological/recording study Morra et al. (2010) gave the stimulant methamphetamine, in combination with cannabinoid CB1 receptors antagonists in the nucleus accumbens. Once again they found that FSIs (in this case in ventral striatum) were more likely than MSNs to have firing rates positively correlated with drug-induced changes in locomotor activity. Taken together these results indicate that FSIs tend to act in a similar way on slow time scales, and may be very important for mediating psychoactive drug actions on behavior, yet do so without producing dramatic changes in overall striatal output.
FSI Activity during Behavioral Tasks
So far only a few studies have examined the correlations between FSI firing and more specific behaviors, so some interpretations remain preliminary. Nonetheless there are some clear initial conclusions that can be drawn. Given that FSI firing rates show quite consistent positive correlations with simple measures of locomotor activity, one might expect that these cells would also show consistent activity patterns relative to behavioral events. In general, this is not the case. In rats performing maze tasks FSIs were shown to have strong event-related changes in firing rate, but with complex time courses that were highly idiosyncratic (Berke, 2008; Schmitzer-Torbert and Redish, 2008). There was little sign of an overall FSI population response – even at times when MSN population activity was changing – and simultaneously recorded, neighboring FSIs that would be expected to contact highly overlapping sets of MSNs had apparently unrelated time courses. This was quite surprising, and not readily compatible with the idea that FSIs simply provide a coordinated broad inhibition of MSNs. If it is indeed the case that individual MSNs are receiving very distinct patterns of perisomatic input from each of a handful of FSIs (∼4–27; Koos and Tepper, 1999), this could be responsible for the “noisy” high-frequency component of MSN membrane voltage (Mahon et al., 2006; Wilson, 2009) but it is not clear what functional role this could achieve. It may be that a network-level, rather than single-cell, perspective will be informative here (see below).
In a subsequent study we examined single units in striatum, GP, and motor cortex in an operant task designed to help separate out processes of movement inhibition, preparation, and execution (Gage et al., 2010). Rats had to maintain their noses in a central hole before receiving first an instruction tone indicating that a left or right movement would be required, then after a delay another sound telling them to proceed. Once again we observed that FSIs had highly idiosyncratic firing rate time courses, with (for example) no systematic preference for contralateral or ipsilateral movements. However, in this task we found that FSI firing rates did show a specific moment of transient coordination, with FSIs throughout striatum increasing firing rate just as the rat initiated his chosen left or right movement. We suggested that the need to engage one action while suppressing another highly trained alternative might be responsible for this brief yet broad FSI “pulse,” which was not seen around other movements in the task. This is an interesting possibility in light of observations that humans with Tourette Syndrome – characterized by difficulties in suppressing overtrained “tics” – have a deficit in striatal FSIs (Kalanithi et al., 2005; Kataoka et al., 2010).
The coordinated FSI population activity increase was accompanied by a matching decrease in overall GP activity, a striking finding that suggests that disinhibition in the pallidostriatal pathway is driving the FSI pulse. The pallidostriatal pathway allows for a wide range of influences over striatal processing, since GP is a central hub receiving input from all over the basal ganglia. It is also a particularly good candidate for driving distributed, coordinated changes in FSI firing given that the pallidostriatal pathway is more divergent than striatopallidal projections. This idea is quite different to previous concepts of broadly coordinated FSI activity conveying feed-forward inhibition from cortex (see above). Although FSIs obviously get cortical inputs and certainly can provide rapid feed-forward inhibition to MSNs, it has so far proven hard to observe evidence of this process occurring under natural conditions. In part this is likely because normal information processing generally involves much weaker, less abrupt signals than electrical cortical stimulation. But it is also because cortical inputs appear to actually drive complex, idiosyncratic activity patterns in FSIs that are usually uncoordinated and cause subtle shifts in MSN spike timing rather than broad changes in firing rate.
FSIs and the Dynamic Organization of Striatal Microcircuits
So far I have focused on changes in FSI firing rate, but there is intriguing evidence that behavior-linked changes in FSI spike timing may be just as, or even more, important. Firstly, FSIs can change their pattern of firing even without marked shifts in rate. For example, the cell shown in Figure 1 (recorded during the operant left/right task described above) shows erratic bursting during the early part of each trial. Once the instruction tone is played, however, firing becomes far more regular, and this regularity persists throughout the delay period until the rat executes his chosen movement, at which point the erratic bursting resumes. In a quantitative analysis (Lau et al., 2010) we found that the instruction tone leads to broad regularization of FSI firing, that was similar to that observed in artificial networks of coupled FSIs following a shift from uniformly random to heterogeneously patterned inputs. In the simulations we found that the gap-junction coupling between FSIs was essential for this phenomenon, which we suggested reflects the transition from a “searching” to a “selected” network state. More direct manipulations of gap-junction connectivity will certainly be needed to test this idea.
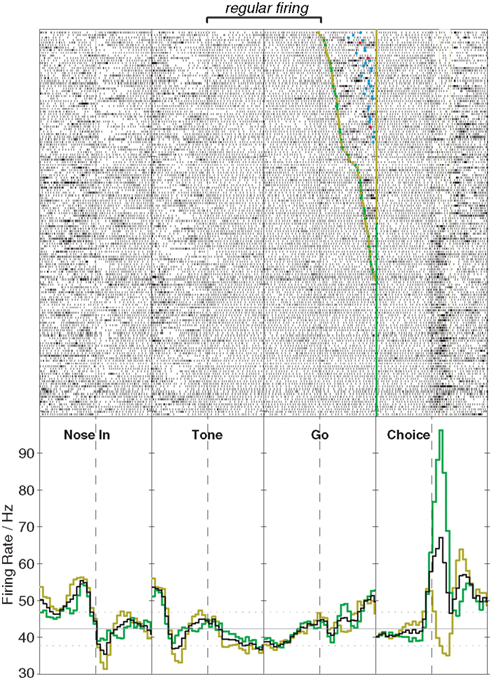
Figure 1. Stabilization of FSI firing pattern with action programming. This example shows activity of a single striatal FSI during performance of an instructed left/right choice task (Gage et al., 2010). Upper panels show raster plots (151 trials total), lower panels show peri-event histograms of firing rate (time range: –0.5 to 0.5s in each case). The rat begins the trial by inserting his nose into an illuminated port (Nose In) and waits for an instruction cue (Tone) that indicates whether a subsequent left or right movement will be rewarded, provided the rat waits for a second cue (Go) before initiating his movement (Choice). For the raster aligned on the Go cue, trials are ordered by reaction time. The FSI shows a sharp increase in firing rate beginning shortly before movement onset, that in this case is stronger for ipsilateral (green) than contralateral movements (gold). The onset of the tone is not associated with a substantial firing rate change, but causes the cell to spike far more regularly until the action is executed (for quantitative analysis, see Lau et al., 2010).
Secondly, FSIs participate in multiple forms of local field potential oscillation in the awake striatum (Berke et al., 2004; Berke, 2005), and can modulate their entrainment even without changes in firing rate (Berke, 2009a). Although the contributions of specific frequencies to striatal information processing remain unclear, LFPs (especially in ventral striatum) show frequent spontaneous transitions between one state involving beta (∼20 Hz) and low-gamma (∼50 Hz), and another state involving theta (∼8 Hz) and high-gamma (∼80 Hz). Dopaminergic drugs such as amphetamine or apomorphine push the network into the theta/high-gamma state, and the same transition is seen around reward receipt (Berke, 2009a) or cues predictive of rewards (unpublished observations). FSIs are much more likely to show clear locking to gamma oscillations than MSNs, and it appears that distinct subpopulations of FSI participate in theta/high-gamma and beta/low-gamma respectively (Berke, 2009a). Other groups investigating striatal LFPs have also reported similar gamma state transitions and FSI involvement (van der Meer et al., 2010). Such phenomena need to be interpreted with caution, given uncertainties about how LFP oscillations are generated in non-laminar structures such as striatum (Berke, 2005). Nonetheless, oscillations are an important potential mechanism by which FSIs might contribute to the dynamic gating of distinct afferent input streams (Akam and Kullmann, 2010) and the formation of projection cell ensembles (Harris et al., 2003), and need to be investigated further in striatum.
Conclusion
What Have We Learned About FSIs, and Next Steps
The advent of striatal FSI monitoring in behaving animals offers an opportunity to move beyond examining “coding” by single neurons to explore how cellular interactions within local microcircuits help achieve behavioral functions. Perhaps the most striking finding so far is how unexpectedly FSIs seem to behave in the context of awake circuit dynamics, compared to brain slices, anesthesia, or sleep. Whereas FSIs in slices are quiescent but strongly inhibit MSNs when artificially stimulated, awake FSIs usually emit a near-continuous stream of single spikes whose influence on nearby MSNs is not readily detectable. FSIs are coupled together by functional gap junctions, yet we have so far seen no clear evidence for precise spike synchronization. Although broad feed-forward inhibition of cortical signals is thought to be an important aspect of FSI function, we have instead found evidence for their transient coordination by a pallidostriatal “feedback” pathway. Putting together many results, our working hypothesis is that three distinct types of inputs to FSIs control three distinct aspects of FSI firing: (1) heterogeneous cortical inputs produce idiosyncratic patterns of behavior-linked FSI firing, that influence cell assembly formation within local striatal modules; (2) gap junctions between FSIs help stabilize their activity as actions are firmly selected; and (3) the pallidostriatal pathway helps coordinate FSI firing rates across wide areas of striatum, perhaps helping to provide timed suppression of unwanted, incompatible actions.
Exactly how FSIs sculpt MSN activity in behaving animals remains mysterious. Perhaps the most likely possibility is that an inhibitory response of MSNs to FSI spikes is highly contingent upon additional factors that change at specific moments during behavioral tasks, such as short-term synaptic depression, simultaneous spiking of multiple FSIs, or the status of cholinergic modulation. In part such hypotheses can be tested using standard methods, but two further types of approach are highly promising for exploring the behavioral functions of FSIs. The first is based on novel tools for manipulating select cell types in awake animals. For example, although it is well established that local interference with striatal GABA transmission can produce dyskinesias including dystonia, the specific contributions of distinct GABAergic elements in striatum has been unclear. Using drugs that interfere with glutamatergic input to FSIs but not MSNs, we have recently found that a selective reduction in FSI activity within sensorimotor striatum is sufficient to produce dystonic symptoms (Gittis et al., submitted), without substantial changes to mean firing rates of nearby MSNs. In addition to confirming the importance of FSIs for avoiding aberrant action selection, this observation supports our previous suggestion (Wiltschko et al., 2010) that FSI suppression is a promising candidate mechanism by which antipsychotics often result in acute dystonic side effects (Kamin et al., 2000). To test ideas about FSI function during normal behaviors it will be useful to perform more precisely timed selective suppression of FSIs (e.g., using optogenetic methods), and assess – as one example – if and when this interferes with the normal behavioral inhibition of unwanted choices during action selection.
A complementary second promising approach is simultaneous monitoring of large (30–100+) numbers of cells within small striatal subregions, using either electrodes with large numbers of nearby contacts or optical recording methods. The spontaneous formation and switching between assemblies of synchronous striatal neurons has been observed both in vitro and in large-scale simulations (Ponzi and Wickens, 2010). FSIs seem to have a vital role controlling assembly formation (Humphries et al., 2009; Humphries, 2011), via processes that may not be easily captured when considering pairwise interactions alone. Yet there has been little network-level examination of striatum in behaving animals, and in combination with selective manipulations this is an exciting frontier for future investigation of FSIs, neuromodulation, and other aspects of striatal function.
Conflict of Interest Statement
The author declares that the research was conducted in the absence of any commercial or financial relationships that could be construed as a potential conflict of interest.
Acknowledgments
For the experiments described here that were performed in my laboratory, primary funding came from the Tourette Syndrome Foundation, the Whitehall Foundation, the National Institute on Drug Abuse, and the National Institute of Mental Health, together with the University of Michigan.
References
Akam, T., and Kullmann, D. M. (2010). Oscillations and filtering networks support flexible routing of information. Neuron 67, 308–320.
Bennett, B. D., and Bolam, J. P. (1994). Synaptic input and output of parvalbumin-immunoreactive neurons in the neostriatum of the rat. Neuroscience 62, 707–719.
Bennett, B. D., and Wilson, C. J. (2003). “TANs, PANs and STANs,” in The Basal Ganglia VI, eds A. M. Graybiel, M. R. DeLong, and S. T. Kitai (New York: Plenum Press), 225–236.
Berke, J. D. (2005). “Participation of striatal neurons in large-scale oscillatory networks,” in The Basal Ganglia VIII, eds J. P. Bolam, C. A. Ingham, and P. J. Magill (New York: Springer), 25–35.
Berke, J. D. (2008). Uncoordinated firing rate changes of striatal fast-spiking interneurons during behavioral task performance. J. Neurosci. 28, 10075–10080.
Berke, J. D. (2009a). Fast oscillations in cortical-striatal networks switch frequency following rewarding events and stimulant drugs. Eur. J. Neurosci. 30, 848–859.
Berke, J. D. (2009b). “Procedural learning: striatum,” in Encyclopedia of Neuroscience, eds L. R. Squire, T. Albright, F. E. Bloom, F. H. Gage, and N. Spitzer (Oxford: Elsevier).
Berke, J. D., Okatan, M., Skurski, J., and Eichenbaum, H. B. (2004). Oscillatory entrainment of striatal neurons in freely moving rats. Neuron 43, 883–896.
Bevan, M. D., Booth, P. A., Eaton, S. A., and Bolam, J. P. (1998). Selective innervation of neostriatal interneurons by a subclass of neuron in the globus pallidus of the rat. J. Neurosci. 18, 9438–9452.
Billings, L. M., and Marshall, J. F. (2003). D2 antagonist-induced c-fos in an identified subpopulation of globus pallidus neurons by a direct intrapallidal action. Brain Res. 964, 237–243.
Blomeley, C. P., and Bracci, E. (2009). Serotonin excites fast-spiking interneurons in the striatum. Eur. J. Neurosci. 29, 1604–1614.
Bracci, E., Centonze, D., Bernardi, G., and Calabresi, P. (2003). Voltage-dependent membrane potential oscillations of rat striatal fast-spiking interneurons. J. Physiol. (Lond.) 549, 121–130.
Bracci, E., and Panzeri, S. (2006). Excitatory GABAergic effects in striatal projection neurons. J. Neurophysiol. 95, 1285–1290.
Carrillo-Reid, L., Tecuapetla, F., Tapia, D., Hernandez-Cruz, A., Galarraga, E., Drucker-Colin, R., and Bargas, J. (2008). Encoding network states by striatal cell assemblies. J. Neurophysiol. 99, 1435–1450.
Centonze, D., Grande, C., Usiello, A., Gubellini, P., Erbs, E., Martin, A. B., Pisani, A., Tognazzi, N., Bernardi, G., Moratalla, R., Borrelli, E., and Calabresi, P. (2003). Receptor subtypes involved in the presynaptic and postsynaptic actions of dopamine on striatal interneurons. J. Neurosci. 23, 6245–6254.
Chuhma, N., Tanaka, K. F., Hen, R., and Rayport, S. (2011). Functional connectome of the striatal medium spiny neuron. J. Neurosci. 31, 1183–1192.
Deng, J. V., Rodriguiz, R. M., Hutchinson, A. N., Kim, I. H., Wetsel, W. C., and West, A. E. (2010). MeCP2 in the nucleus accumbens contributes to neural and behavioral responses to psychostimulants. Nat. Neurosci. 13, 1128–1136.
Fino, E., Deniau, J. M., and Venance, L. (2008). Cell-specific spike-timing-dependent plasticity in GABAergic and cholinergic interneurons in corticostriatal rat brain slices. J. Physiol. (Lond.) 586, 265–282.
Fino, E., Glowinski, J., and Venance, L. (2005). Bidirectional activity-dependent plasticity at corticostriatal synapses. J. Neurosci. 25, 11279–11287.
Fujisawa, S., Amarasingham, A., Harrison, M. T., and Buzsaki, G. (2008). Behavior-dependent short-term assembly dynamics in the medial prefrontal cortex. Nat. Neurosci. 11, 823–833.
Fukuda, T. (2009). Network architecture of gap junction-coupled neuronal linkage in the striatum. J. Neurosci. 29, 1235–1243.
Gage, G. J., Stoetzner, C. R., Wiltschko, A. B., and Berke, J. D. (2010). Selective activation of striatal fast-spiking interneurons during choice execution. Neuron 67, 466–479.
Gerfen, C. R., Baimbridge, K. G., and Miller, J. J. (1985). The neostriatal mosaic: compartmental distribution of calcium-binding protein and parvalbumin in the basal ganglia of the rat and monkey. Proc. Natl. Acad. Sci. U.S.A. 82, 8780–8784.
Gittis, A. H., Leventhal, D. K., Fensterheim, B. A., Pettibone, J. R., Berke, J. D., and Kreitzer, A. C. (submitted). Selective inhibition of striatal fast-spiking interneurons elicits dystonia.
Gittis, A. H., Nelson, A. B., Thwin, M. T., Palop, J. J., and Kreitzer, A. C. (2010). Distinct roles of GABAergic interneurons in the regulation of striatal output pathways. J. Neurosci. 30, 2223–2234.
Golomb, D., Donner, K., Shacham, L., Shlosberg, D., Amitai, Y., and Hansel, D. (2007). Mechanisms of firing patterns in fast-spiking cortical interneurons. PLoS Comput. Biol. 3, e156. doi: 10.1371/journal.pcbi.0030156
Gustafson, N., Gireesh-Dharmaraj, E., Czubayko, U., Blackwell, K. T., and Plenz, D. (2006). A comparative voltage and current-clamp analysis of feedback and feedforward synaptic transmission in the striatal microcircuit in vitro. J. Neurophysiol. 95, 737–752.
Harris, K. D., Csicsvari, J., Hirase, H., Dragoi, G., and Buzsaki, G. (2003). Organization of cell assemblies in the hippocampus. Nature 424, 552–556.
Hjorth, J., Blackwell, K. T., and Hellgren Kotaleski, J. (2009). Gap junctions between striatal fast-spiking interneurons regulate spiking activity and synchronization as a function of cortical activity. J. Neurosci. 29, 5276–5286.
Humphries, M. D. (2011). Spike-train communities: finding groups of similar spike trains. J. Neurosci. 31, 2321–2336.
Humphries, M. D., Wood, R., and Gurney, K. (2009). Dopamine-modulated dynamic cell assemblies generated by the GABAergic striatal microcircuit. Neural. Netw. 22, 1174–1188.
Kalanithi, P. S., Zheng, W., Kataoka, Y., DiFiglia, M., Grantz, H., Saper, C. B., Schwartz, M. L., Leckman, J. F., and Vaccarino, F. M. (2005). Altered parvalbumin-positive neuron distribution in basal ganglia of individuals with Tourette syndrome. Proc. Natl. Acad. Sci. U.S.A. 102, 13307–13312.
Kamin, J., Manwani, S., and Hughes, D. (2000). Emergency psychiatry: extrapyramidal side effects in the psychiatric emergency service. Psychiatr. Serv. 51, 287–289.
Kataoka, Y., Kalanithi, P. S., Grantz, H., Schwartz, M. L., Saper, C., Leckman, J. F., and Vaccarino, F. M. (2010). Decreased number of parvalbumin and cholinergic interneurons in the striatum of individuals with Tourette syndrome. J. Comp. Neurol. 518, 277–291.
Kawaguchi, Y. (1993). Physiological, morphological, and histochemical characterization of three classes of interneurons in rat neostriatum. J. Neurosci. 13, 4908–4923.
Kincaid, A. E., Zheng, T., and Wilson, C. J. (1998). Connectivity and convergence of single corticostriatal axons. J. Neurosci. 18, 4722–4731.
Kita, H., Kosaka, T., and Heizmann, C. W. (1990). Parvalbumin-immunoreactive neurons in the rat neostriatum: a light and electron microscopic study. Brain Res. 536, 1–15.
Klaus, A., Planert, H., Hjorth, J., Berke, J. D., Silberberg, G., and Kotaleski, J. H. (2011). Striatal fast-spiking interneurons: from firing patterns to postsynaptic impact. Front. Syst. Neurosci. 5:57. doi: 10.3389/fnsys.2011.00057
Koos, T., and Tepper, J. M. (1999). Inhibitory control of neostriatal projection neurons by GABAergic interneurons. Nat. Neurosci. 2, 467–472.
Koos, T., and Tepper, J. M. (2002). Dual cholinergic control of fast-spiking interneurons in the neostriatum. J. Neurosci. 22, 529–535.
Koos, T., Tepper, J. M., and Wilson, C. J. (2004). Comparison of IPSCs evoked by spiny and fast-spiking neurons in the neostriatum. J. Neurosci. 24, 7916–7922.
Lansink, C. S., Goltstein, P. M., Lankelma, J. V., and Pennartz, C. M. (2010). Fast-spiking interneurons of the rat ventral striatum: temporal coordination of activity with principal cells and responsiveness to reward. Eur. J. Neurosci. 32, 494–508.
Lau, T., Gage, G. J., Berke, J. D., and Zochowski, M. (2010). Local dynamics of gap-junction-coupled interneuron networks. Phys. Biol. 7, 16015.
Luk, K. C., and Sadikot, A. F. (2001). GABA promotes survival but not proliferation of parvalbumin-immunoreactive interneurons in rodent neostriatum: an in vivo study with stereology. Neuroscience 104, 93–103.
Mahon, S., Vautrelle, N., Pezard, L., Slaght, S. J., Deniau, J. M., Chouvet, G., and Charpier, S. (2006). Distinct patterns of striatal medium spiny neuron activity during the natural sleep-wake cycle. J. Neurosci. 26, 12587–12595.
Mallet, N., Ballion, B., Le Moine, C., and Gonon, F. (2006). Cortical inputs and GABA interneurons imbalance projection neurons in the striatum of parkinsonian rats. J. Neurosci. 26, 3875–3884.
Mallet, N., Le Moine, C., Charpier, S., and Gonon, F. (2005). Feedforward inhibition of projection neurons by fast-spiking GABA interneurons in the rat striatum in vivo. J. Neurosci. 25, 3857–3869.
Morra, J. T., Glick, S. D., and Cheer, J. F. (2010). Neural encoding of psychomotor activation in the nucleus accumbens core, but not the shell, requires cannabinoid receptor signaling. J. Neurosci. 30, 5102–5107.
Parthasarathy, H. B., and Graybiel, A. M. (1997). Cortically driven immediate-early gene expression reflects modular influence of sensorimotor cortex on identified striatal neurons in the squirrel monkey. J. Neurosci. 17, 2477–2491.
Planert, H., Szydlowski, S. N., Hjorth, J. J., Grillner, S., and Silberberg, G. (2010). Dynamics of synaptic transmission between fast-spiking interneurons and striatal projection neurons of the direct and indirect pathways. J. Neurosci. 30, 3499–3507.
Plenz, D. (2003). When inhibition goes incognito: feedback interaction between spiny projection neurons in striatal function. Trends Neurosci. 26, 436–443.
Plenz, D., and Kitai, S. T. (1998). Up and down states in striatal medium spiny neurons simultaneously recorded with spontaneous activity in fast-spiking interneurons studied in cortex-striatum-substantia nigra organotypic cultures. J. Neurosci. 18, 266–283.
Ponzi, A., and Wickens, J. (2010). Sequentially switching cell assemblies in random inhibitory networks of spiking neurons in the striatum. J. Neurosci. 30, 5894–5911.
Rajakumar, N., Elisevich, K., and Flumerfelt, B. A. (1994). The pallidostriatal projection in the rat: a recurrent inhibitory loop? Brain Res. 651, 332–336.
Ramanathan, S., Hanley, J. J., Deniau, J. M., and Bolam, J. P. (2002). Synaptic convergence of motor and somatosensory cortical afferents onto GABAergic interneurons in the rat striatum. J. Neurosci. 22, 8158–8169.
Rebec, G. V. (2006). Behavioral electrophysiology of psychostimulants. Neuropsychopharmacology 31, 2341–2348.
Schmitzer-Torbert, N. C., and Redish, A. D. (2008). Task-dependent encoding of space and events by striatal neurons is dependent on neural subtype. Neuroscience 153, 349–360.
Shen, W., Flajolet, M., Greengard, P., and Surmeier, D. J. (2008). Dichotomous dopaminergic control of striatal synaptic plasticity. Science 321, 848–851.
Spooren, W. P., Lynd-Balta, E., Mitchell, S., and Haber, S. N. (1996). Ventral pallidostriatal pathway in the monkey: evidence for modulation of basal ganglia circuits. J. Comp. Neurol. 370, 295–312.
Taverna, S., Canciani, B., and Pennartz, C. M. (2007). Membrane properties and synaptic connectivity of fast-spiking interneurons in rat ventral striatum. Brain Res. 1152, 49–56.
Turner, R. S., and Desmurget, M. (2010). Basal ganglia contributions to motor control: a vigorous tutor. Curr. Opin. Neurobiol. 20, 704–716.
van der Meer, M. A., Kalenscher, T., Lansink, C. S., Pennartz, C. M., Berke, J. D., and Redish, A. D. (2010). Integrating early results on ventral striatal gamma oscillations in the rat. Front. Neurosci. 4:300. doi: 10.3389/fnins.2010.00300
Wilson, C. (2009). “What controls the timing of striatal spiny cell action potentials?” in The Basal Ganglia IX, eds H. J. Groenewegen, P. Voorn, H. W. Berendse, A. B. Mulder, and A. R. Cools (Heidelberg: Springer Drodrecht), 49–61.
Wilson, C. J., Chang, H. T., and Kitai, S. T. (1990). Firing patterns and synaptic potentials of identified giant aspiny interneurons in the rat neostriatum. J. Neurosci. 10, 508–519.
Wiltschko, A. B., Pettibone, J. R., and Berke, J. D. (2010). Opposite effects of stimulant and antipsychotic drugs on striatal fast-spiking interneurons. Neuropsychopharmacology 35, 1261–1270.
Wu, Y., and Parent, A. (2000). Striatal interneurons expressing calretinin, parvalbumin or NADPH-diaphorase: a comparative study in the rat, monkey and human. Brain Res. 863, 182–191.
Keywords: striatum, interneuron, GABA, basal ganglia, gamma oscillations, dopamine, amphetamine, antipsychotic
Citation: Berke JD (2011) Functional properties of striatal fast-spiking interneurons. Front. Syst. Neurosci. 5:45. doi: 10.3389/fnsys.2011.00045
Received: 12 April 2011;
Paper pending published: 10 May 2011;
Accepted: 03 June 2011;
Published online: 20 June 2011.
Edited by:
Charles J. Wilson, University of Texas at San Antonio, USAReviewed by:
Enrico Bracci, University of Manchester, UKGiuseppe Sciamanna, Santa Lucia Foundation, Italy
Copyright: © 2011 Berke. This is an open-access article subject to a non-exclusive license between the authors and Frontiers Media SA, which permits use, distribution and reproduction in other forums, provided the original authors and source are credited and other Frontiers conditions are complied with.
*Correspondence: Joshua D. Berke, Department of Psychology, University of Michigan, 530 Church Street, Ann Arbor, MI 48109, USA. e-mail:amRiZXJrZUB1bWljaC5lZHU=