- 1Graham Centre for Agricultural Innovation, New South Wales Department of Primary Industries and Charles Sturt University, Wagga Wagga Agricultural Institute, Wagga Wagga, NSW, Australia
- 2New South Wales Department of Primary Industries, Weed Research Unit, Wagga Wagga, NSW, Australia
- 3State Key Laboratory of Cotton Biology, Institute of Cotton Research, Chinese Academy of Agricultural Sciences, Anyang, China
Witchgrass (Panicum capillare L.) is a summer growing grass weed species and is increasing its prevalence in southern Australia. A better understanding of the seed biology is needed to effectively manage this weed. A series of field and laboratory studies were conducted to determine seed germination factors, field emergence patterns, and soil seedbank longevity. Witchgrass germination was stimulated by light and it germinated better at temperature over 20°C, with 93–100% germination at the two constant temperatures of 20 and 30°C, and the two alternating day/night temperatures of 30/25 and 35/25°C. It is highly tolerant to moisture stress at germination, with 2–7% germination even at −0.48 Mpa. Witchgrass seed lost 47–68% viability after 12 months of burial in the soil, however the seed persisted for more than 4 years if buried at 10 cm in the soil. Witchgrass emergence in southern New South Wales (NSW) commenced in mid spring (early October), with peak emergence of 63–83% in November and then significantly reduced to 16–37% emergence in December. Little emergence (<1%) occurred in the summer months from January to February. These results provide useful information for designing effective management strategies and the optimum timing of control. Climate change could favor the phenological development and the further spread of this weed, which present new challenges for its effective management. Further study is needed to investigate the impact of climate change on the biology, spread, and management of witchgrass.
Introduction
The genus Panicum is one of the largest genera in the Poaceae, with more than 500 species worldwide, mostly occurring in the tropics (Crins, 1991; Aliscioni et al., 2003; Byng, 2014). Panicum species are also widespread in Australia, with 24 indigenous and nine introduced species (CHAH (Council of Heads of Australasian Herbaria), 2021). These Panicum species are among the key summer weeds in Australia (Llewellyn et al., 2016). Due to the taxonomic confusions in the genus Panicum (Darbyshire and Cayouette, 1995; Dirkse and Holverda, 2016), DNA barcoding techniques have been employed to assist the species identification and the subsequent control (Chen et al., 2018).
Witchgrass (Panicum capillare L.) is an annual grass belongs to the genus Panicum and it is indigenous to North America (Baskin and Baskin, 1986). It is a summer active growing C4 grass weed species and has invaded many non-native ranges throughout the world, from subtropical to temperate areas. These include Asia, South America (Argentina and Chile), New Zealand, Australia, Morocco, Russia, and many European countries (Clements et al., 2004). In Australia, the earliest herbarium record of witchgrass was collected in South Australia in 1911, followed by the second earliest report in Sydney in 1927 (AVH, 2021). Witchgrass has increased in abundance in southern Australia in recent years. Currently, it has widely distributed in the south western states of Australia. A recent summer weed survey from Western Plains through the Riverina districts of New South Wales (NSW) showed that witchgrass was the second most prevalent annual weed in the region (Weston et al., 2016). The weed is commonly found in the cropping fields soon after harvest, in fallows and pastures, waste land, along fence lines and on roadsides. In Australia, it can be easily confused with a native perennial grass, Hairy Panic (Panicum effusum R.Br.) (Phillips, 2010).
Witchgrass is more problematic in no-till farming systems than conventional tillage systems (Frick et al., 1990). It can cause yield loss in crops, toxicity in animals and is a host to several pests and diseases. It often infests summer crops in the northern hemisphere such as corn, soybeans, sorghum, and in winter wheat (Clements et al., 2004). If left uncontrolled, it can cause 4–5% yield loss in corn and soybean at a weed density of 5 plants/m2 (Ontario Weed Committee, 2021). In southern NSW, witchgrass thrives in bare areas of winter crops and grows rapidly to a thick mat soon after crop harvest due to the removal of crop competition. It is also a competitive weed in degraded pastures under drought conditions (Phillips, 2010). The vigorous growth in the summer consumes valuable stored soil moisture and nutrients which could otherwise be used by crops sown in autumn.
Witchgrass can pose significant animal health issues. It has been found to accumulate nitrate which could be toxic to livestock under certain conditions (Kingsbury, 1964). Additionally, hepatogenous photosensitization was reported in Merino sheep grazing on witchgrass in Australia (Quinn et al., 2014). Furthermore, witchgrass is an alternative host to a range of pests and diseases, including cereal aphids such as Rhopalosiphum padi L. and R. maidis Fiotch (Kieckhefer and Lunden, 1983), western corn rootworm (Coleoptera: Chrysomelidae) (Chege et al., 2005), and wheat streak mosaic virus (Christian and Willis, 1993; Coutts et al., 2008).
The prevalence of witchgrass is associated with its high seed production, unique spreading mechanism, and persistent seedbank (Clements et al., 2004). Witchgrass is a prolific seed producer, producing upto 56,400 seeds per plant in the absence of competition (Stevens, 1932). It has an unusual tumbleweed-like seed dispersal mechanism. The mature spherical inflorescence is brittle, easily breaking off and is spread long distances by wind. Large piles of grass inflorescence often engulf country roads and streets, fenceline, yards, garages, sheds, and houses in wet summers in southern Australia.
The seed of witchgrass has a hard seed coat and possesses strong innate dormancy. Brecke (1974) found that witchgrass seeds collected in autumn in Central New York State had broken all dormancy after dry storage for 5 months in the dark at room temperature. In the field, dormancy releases during late autumn and winter and the seeds subsequently germinate in spring and summer, while hot summer tends also to induce secondary dormancy (Baskin and Baskin, 1986). The seed germinates better under light and alternating temperatures (Cross, 1931; Brecke, 1974; Baskin and Baskin, 1986). Witchgrass germination is low at temperatures below 20°C and is greatest above 25°C (Rivera and Peters, 1971; Vengris and Damon, 1976; Baskin and Baskin, 1986).
Witchgrass is a poor competitor and does not grow well in shaded environments (Vengris and Damon, 1976; Clements et al., 2004). Shading delayed both the vegetative and reproductive growth of witchgrass and reduced the number of tillers and panicles (Vengris and Damon, 1976). Brecke (1974) found that witchgrass mostly emerged from near or on the soil surface (0–2.5 cm), with limited emergence occurring below 5 cm of burial.
Despite the increasing prevalence of witchgrass in pastures and arable land, little is known of the seed germination biology, persistence and emergence of this weed in Australia. Improved understanding of the biology and ecology of witchgrass has become increasingly important, particularly in relation to the changing climate. The altered temperature and rainfall patterns associated with global climate change will have direct effects on seed dormancy, germination, emergence, persistence, and reproduction (Walck et al., 2011). These characteristics will subsequently affect the distribution, species shift, population dynamics, life cycle, phenology, competitive potential, and herbicide control efficacy (Peters et al., 2014; Varanasi et al., 2016; Ramesh et al., 2017). Therefore, this study was conducted to determine: (1) the factors that affect the seed germination of witchgrass; (2) the emergence patterns and the longevity of the seed in the soil seedbank. This information will help in determining the population dynamics of this problematic species and in identifying the suitable timing for its early control.
Materials and Methods
Seed Collection and Preparation
Mature seeds of witchgrass were collected from a summer fallow paddock (147.337207E, 35.029273S) in February 2007 (Source A) and in March 2009 (Source B) in Wagga Wagga, New South Wales (NSW), Australia. Both seed sources were highly dormant at the time of collection. The freshly collected seed was stored at room temperature for 1 year to break the dormancy. The germination was 95 and 60% for the seed Sources A and B prior to the following experiments, respectively. The seed Source A was used in all the experiments except the light and moisture stress experiments when the seed Source B was used.
Temperature on Germination
One hundred seeds of witchgrass (seed Source A) were placed on a piece of filter paper (Whatman No.2) moistened with 5 ml of deionised water in a 9 cm petri dish. Petri dishes were sealed with parafilm and incubated at three constant temperatures of 10, 20, and 30°C, and four fluctuating nigh day/night temperatures of 20/15, 25/15, 30/25, 35/25°C with a 12-h photoperiod. These fluctuating temperature regimes were a good representation of the wide emergence window of this weed from spring to summer in southern NSW. The germinated seeds were recorded after 7 d of incubation based on our preliminary studies.
Light on Germination
The influence of light on the germination of witchgrass was tested at four sets of fluctuating temperatures (20/15, 25/15, 30/25, and 35/25°C) in April 2010 by using the seed Source B as the viability of seed Source A was low 3 years after collection. Two layers of aluminum foil were used to wrap petri dishes to achieve complete darkness during incubation. Germination recorded at 7 days after incubation in a 12-h photoperiod at the respective temperature regimes was compared.
Moisture Stress on Germination
The effect of drought stress was studied using the seed Source B at three fluctuating day/night temperatures of 25/15, 30/25, and 35/25°C with a 12-h photoperiod. Solutions with osmotic pressures of 0, −0.03, −0.06, −0.12, −0.24, −0.48, and −0.96 MPa were prepared by dissolving polyethylene glycol (PEG) 8000 in 80 ml of distilled water using appropriate quantities for each temperature as determined from Michel (1983). The PEG 8000 quantities were determined from the average for the two fluctuating temperatures.
Seed Persistence in the Field
Seed burial experiments were commenced on 15 April 2008 on the Research Farm (147.334958E, 35.029780S) of Wagga Wagga Agricultural Institute, NSW. One hundred witchgrass seeds (seed Source A) were placed in a 4 × 6 cm mesh packet together with a small quantity of sieved soil to improve soil and seed contact. The packets were then placed at three soil depths (0, 5, and 10 cm) in a minimum-tilled field free of vegetation under natural conditions. The soil type was a duplex Red Kandosol. The packets were recovered at six intervals [3, 6, 12, 24, 36, and 48 months (mo)] in a randomized complete block design with three replicates.
Recovered seeds were counted and placed on moistened Whatman No.2 filter paper in a 9-cm petri dish, which was then sealed with parafilm and incubated for 7 d under the fluctuating temperatures of 30/25°C with a 12-h photoperiod. The number of germinated seeds was recorded and viability of ungerminated seeds determined by tetrazolium staining. Briefly, seeds were cut in half and incubated in darkness at 30°C for 5 h in a 0.5% 2,3,5-triphenyltetrazolium chloride solution. Seeds were deemed to be viable but dormant if the radical had stained red. Total viability of the exhumed seeds included the germinated seeds and the viable but dormant seeds.
Seed Emergence in the Field
Three trials were conducted to determine the emergence of witchgrass under field conditions in the summer of 2008/2009 and 2012/2013 in southern NSW. In the 1st trial (Site A), four permanent quadrats (1 × 1 m2) were randomly placed in a cropping paddock (147.353080E, 35.049480S) on 17 April 2008. The site was heavily infested with witchgrass in the summer of 2007. Two hundred additional seeds (seed Source A) were evenly spread onto the surface in each quadrat and lightly harrowed to increase the seedbank size for subsequent emergence monitoring. No further tillage or harrowing was imposed.
Two additional trials (Sites B and C) were conducted in the summer of 2012/2013. Four permanent quadrats (1 × 1 m2) were randomly placed in August in two fields (147.360067E, 35.032868S, Site B and 147.353080E, 35.049480S, Site C) with a known history of witchgrass. No additional seeds were required due to the heavy infestation in the previous season.
The trials were maintained under natural conditions without additional irrigation. Emerged seedlings in each quadrat in the three trials were assessed from September to February until no further emergence. Seedlings were counted and removed by spraying with glyphosate on fortnightly or monthly intervals, dependent upon rainfall events.
Statistical Analysis
A randomized complete block design with three replications was used in all experiments. The experiments of temperature and light conditions on germination were repeated twice. Data from repeated experiments were combined as there were no significant differences over time. Homogeneity of variance was not improved by transformation, therefore analysis was performed on raw percentage germination. Data variance was visually inspected by plotting residuals to confirm homogeneity of variance before statistical analysis. Data were analyzed using analysis of variance and post-hoc Fishers tests used to determine statistically different means.
Results
Temperature
Temperatures significantly affected the germination of witchgrass (Figure 1). It germinated poorly at the lower temperature treatments, with no germination at 10°C and only 10–20% germination at the fluctuating temperatures of 20/15 and 25/15°C. Germination did not significantly differ between constant temperatures of 20 and 30°C, and the alternating temperatures of 30/25 and 35/25°C. The highest germination was achieved at temperatures over 20°C, with 93–100% germination at the two constant temperatures of 20 and 30°C, and the two alternating temperatures of 30/25 and 35/25°C in a 12-h light/dark cycle.
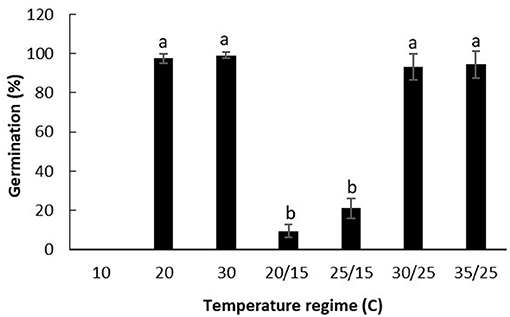
Figure 1. Impact of constant and alternating temperatures on the germination of P. capillare. Vertical bars represent the standard error of the mean.
Light
The germination of witchgrass was significantly encouraged by light (Figure 2). The germination was 56% under the photoperiod of 12 h at 30/25 and 35/25°C, while it was only 1–8% in complete darkness under the same temperature conditions. Light stimulation of the germination was also evidenced at the lower day/night temperature regime 25/15°C.
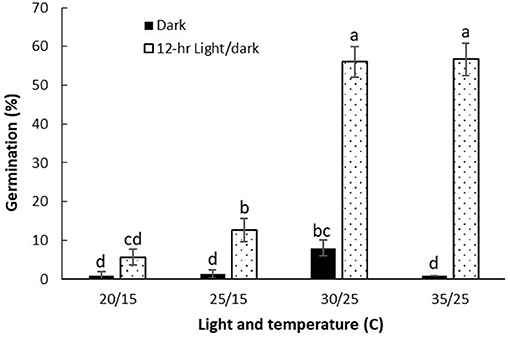
Figure 2. Impact of light on the germination of P. capillare. Vertical bars represent the standard error of the mean.
Moisture Stress
Osmotic potential significantly influenced the germination of witchgrass (Figure 3). Without the osmotic stress, the germination of witchgrass was 12.7, 48.0, and 56.7% at the temperatures of 25/15, 30/25, and 35/25°C, respectively. Increasing osmotic stress levels reduced germination under all temperature regimes. At the fluctuating temperatures of 35/25°C, seed germination decreased from 56.7 to 2% as osmotic potentials decreased from 0 to −0.48 MPa, and no germination was observed at −0.96 MPa. Similarly, the germination at −0.48 MPa was very low (4–7%) at 25/15and 30/25°C. Even at −0.96 MPa, limited germination (0.7%) occurred at the temperatures of 30/25°C.
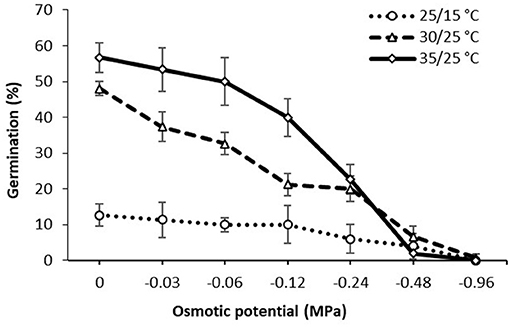
Figure 3. Effect of osmotic potential on germination of P. capillare seeds incubated at alternating temperature regimes with a 12-h photoperiod. Vertical bars represent the standard error of the mean.
Seed Persistence
Both duration and depth of burial significantly reduced (P < 0.01) germination and viability of witchgrass seed (Figure 4). There was no interaction between duration and depth of burial. The initial germination at the time of burial (0-mo) was 95% and declined slightly after 3 months of burial at 0- and 5-cm depths, which was then followed by a sharp decrease after 6 months of burial at both depths (Figure 4A). However, at the burial depth of 10 cm, the germination at 6-mo remained high at 88%, then decreased rapidly to 53% at 12-mo. After 12 months of burial, the germination at the three burial depths followed similar declining trend, with 29–46, 0–10, and 0–5% germination after 2, 3, and 4 years of burial, respectively.
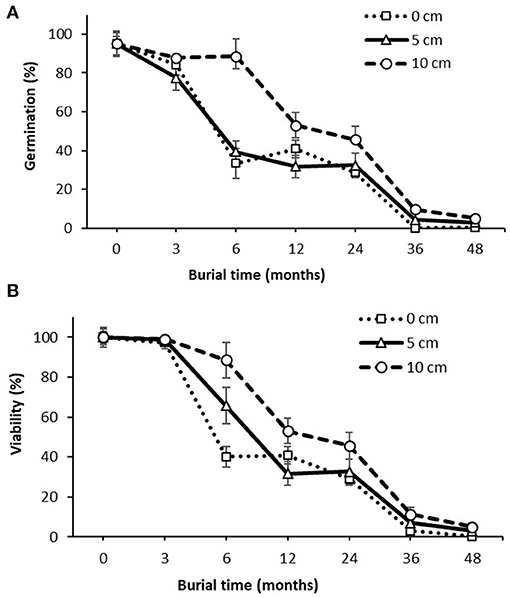
Figure 4. Effect of burial duration and depth on P. capillare seed (A) germination (total germinated seeds from the recovered seeds divided by the buried seeds, %) and (B) viability (total viable seeds from the recovered seeds divided by the buried seeds, %). Vertical bars represent the standard error of the mean.
The viability of buried seeds remained little changed during the first 3 months of burial, which was then followed by a steady decline, irrespective to the burial depth (Figure 4B). Most of the viability loss occurred within the first year after burial. The viability was reduced from the initial 100% before burial (0-mo) to 32–53% after burial for 12 months. The viability continued to decline to 3–11 and 0–5% after 3 and 4 years of burial, respectively, with seeds exhumed from deeper in the soil profile having higher viability than the shallower depths. No viable seed remained on the soil surface (0 cm) after 4 year, compared to 3–5% at the 5- and 10-cm burial depth during the same period.
Emergence in the Field
The average rainfall was 413.5, 389.2, 560.8, and 390.8 mm for the respective year 2008, 2009, 2012, and 2013, compared to the long-term average of 528.7 mm (115 years) (Figure 5). The rainfall received during the active weed growth period from September to February was 187.7 mm in 2008/2009 and 176.9 mm in 2012/2013, which was only about 68–73% of the long-term average. Spring rainfall is critical to trigger the emergence events as temperature is not a limiting factor during this period. However, the total rainfall in September and October in both 2008 and 2012 was only 24–45% of the long-term average, which could restrict the emergence of witchgrass.
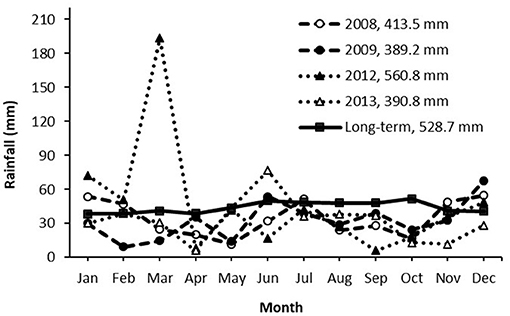
Figure 5. Annual rainfall in years 2008, 2009, 2003, and 2013 as compared with the long-term average (115 years).
Despite of the significantly lower than long-term average rainfall in spring, witchgrass still managed to emerge, with total emerged seedlings of 428 plants/m2 at Site A in the summer of 2008/2009, and 203 and 1,557 plants/m2 at Sites B and C in 2012/2013, respectively (Figure 6). The emergence patterns followed similar trends between the sites and years. Witchgrass emergence initiated in late September, with 0–18% seedlings counted in early October, followed by a major emergence of 63–83% in November and 16–37% emergence in December. Little emergence (<1%) was recorded from January to February across the three sites.
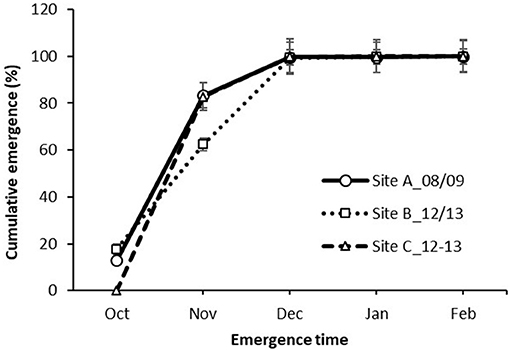
Figure 6. Emergence of P. capillare over time in the field. Emerged seedlings were counted and removed by spraying glyphosate. Vertical bars represent the standard error of the mean. Cumulative emergence (%) was calculated from the cumulative number of emerged seedlings at the respective month divided by the total number of emerged seedlings during the entire experimental period.
Discussion
Witchgrass is a warm season C4 grass and prefers higher temperatures (over 20°C) to improve germination, which is consistent with previous studies (Cross, 1931; Brecke, 1974; Baskin and Baskin, 1986). Higher constant temperatures of 20 and 30°C achieved a high level of germination (97–100%), which is not different to 93–94% germination achieved under the alternating temperatures of 30/25 and 35/25°C. However, better germination under alternating temperatures have been reported previously in witchgrass (Cross, 1931; Brecke, 1974).
The high sensitivity of witchgrass germination to light reported in this study agrees with Baskin and Baskin (1986). They found that high germination of witchgrass (76–100%) was achieved in light at a wide range of alternating temperatures, while the germination was only 1–24% under complete darkness. These results indicate that inversion tillage and stubble cover could be effective control options in suppressing the germination and emergence in the field. However, shallow soil disturbance could encourage the emergence as it will bring buried seed to the surface where light becomes unlimiting (Baskin and Baskin, 1986). We propose that response to light might be an adaptation tactic to ensure the germination of this small-seeded species, particularly when they are near or at the soil surface.
Studies related to water stress tolerance in witchgrass are scant. This study found that decreases in osmotic potential resulted in reduced germination in witchgrass. Limited germination can even occur at the water potential of −0.96 MPa under optimum alternating temperatures of 30/25°C, indicating that witchgrass is drought tolerant at germination. It is incongruent with many other annual grasses that require adequate soil moisture for germination, with complete germination suppression at osmotic potentials between −0.6 and −1.3 MPa (Masin et al., 2005; Seepaul et al., 2012; Fernando et al., 2016). Seepaul et al. (2012) reported that germination of switchgrass (Panicum virgatum L.) was significantly suppressed at −0.8 MPa, with no germination at the osmotic potential between −1.2 MPa. Wild proso millet (Panicum miliaceum L.) also had low germination (2%) at −1.0 and −1.4 MPa (Stump, 1984). These results indicate that witchgrass germination is tolerant to moisture stress, which is supported by the high seedling numbers up to 1,560 plants/m2 emerged in the field during late spring and early summer of 2008/2009 and 2012/2013 even when the spring rainfall between September and October (a critical period for emergence) was only 24–45% of the long-term average. The success of witchgrass under drought conditions could also be due to its unique seedling root structure. Gross et al. (1992) reported that the root growth of witchgrass exhibited a herringbone topology (i.e., magnitude = altitude), which favors plants growing in water-limited habitats.
Viability of seeds in the soil decreases with the duration of burial and increases with the depth of burial. The seed of witchgrass persists well in the soil, possibly due to the presence of a hard seed coat. Although majority of seed viability (47–68%) was lost after 12 months of burial, seed can survive after 4 years of burial at the deeper burial depths of 5 and 10 cm. The rapid loss of viability within 12 months of burial and the long persistence after several years of burial have also been documented in other Panicum species (Alex, 1980; Burnside et al., 1981; Stump, 1984). After 21 months of burial, the seed viability of P. miliaceum was only 23% at the burial depth of 5 cm, but it was 77 and 93% at 10 and 30 cm, respectively. Alex (1980) reported that loss of viability of Panicum dichotomiflorum was influenced by soil type, depth and duration of burial. Seeds in gravelly loam lost viability faster than those in silt loam soil, and seeds at 1 and 2 cm lost viability faster than those at deeper depths. After 54 mo of burial, seeds buried at depths between 5 and 20 cm in silt loam remained 5–14% viability. These results indicate that witchgrass seed could persist well in the field and deeper burial would result in longer persistence with a low but tenacious tail of more than 4 years. Failure to stop seedset would mean the on-going battle of this weed. Consistent control efforts over several years are required to overcome the persistent nature of the seed and to effectively manage this weed.
Better understanding the emergence patterns is important to the effective management as better control timing can be tailored to individual circumstances. Witchgrass has a staggered emergence pattern in southern NSW. It started to emerge in mid spring, with major emergences in late spring and early summer. This emergence pattern is similar to a study in Tennessee (Baskin and Baskin, 1986) who reported that witchgrass emerges over an extended period during spring and summer. Earlier emergence cohorts have taller plant height, tillers, more panicles per plant as compared to later emergence cohorts (Vengris and Damon, 1976). Therefore, management efforts should be directed to control the early and major emergence cohorts in late spring and early summer before setting seeds. Controlling early cohorts can stop seedset and reduce further infestation.
Witchgrass is predominantly a surface germinator. Most emergence is likely to come from the seeds on or near the soil surface due to the light requirements for germination. Seeds buried in the soil are unlikely to emerge in summer (Baskin and Baskin, 1986). Brecke (1974) reported 52–95% emergence occurred at depths between 0 and 2.5 cm and no emergence at depths over 5 cm. Also, optimum emergence at shallow burial depths was documented in P. dichotomiflorum (Fausey and Renner, 1997). However, another Panicum species, P. miliaceum, was able to emerge from deeper depths, with a maximum depth of emergence at 14 cm (Stump, 1984). Strategic tillage could be an effective option to bury witchgrass seed into deeper soil layers to suppress the emergence. However, prolonged persistence of witchgrass seeds in the deeper soil means that care should be taken not to re-introduce the buried but viable seeds back to the soil surface through tillage operations.
Successful seed emergence is the most important phase of plant establishment for weed to survive, thrive and proliferate, which is strongly influenced by temperature and moisture. However, these two important environmental clues are being influenced by climate change.
Rising CO2, temperature and altered precipitation patterns are three key indicators of climate change. Under the current rates of CO2 emissions, the concentration of CO2 is predicted to reach ~1000 ppm by the end of the twenty-first century, resulting in an increase of 2–4°C in the Earth's annual surface temperature (IPCC, 2007). However, the predictions for long-term rainfall patterns are far less certain (Giannini et al., 2008; Varanasi et al., 2016). In Australia, it is generally agreed that summer rainfall had increased in eastern Australia over the past century, while winter rainfall had decreased in the southwest, with large decadal-scale variations and distinct changes in different regions (Liu et al., 2020). They further reported an increasing trend in long-term rainfall in Northern and Central Australia, and a decreasing trend across Southern Australia.
The anticipated changes in temperature and moisture projected under changing climates will directly affect seed longevity, dormancy release, germination, and emergence (Walck et al., 2011; Ooi et al., 2014; Jaganathan and Liu, 2015). Indirectly, climate change will also affect seed predators and soil microbial activities, which in turn could affect the fate of seeds in the soil seedbank (Wu, 2015). The seed aging process is accelerated under high temperature and moisture conditions (Walck et al., 2011; Fenollosa et al., 2020). However, little research is available in this emerging field in regard to climate change.
Temperature is the key driver for the onset of emergence, flowering and other phenological traits (Menzel et al., 2006a,b; Otto et al., 2007). The increasing temperature would accelerate the accumulation of growing degree days required for the growth and development of plants, which would result in the changes in dormancy release, emergence timing and phenological development. Therefore, weeds are likely to grow and develop quicker, with earlier emergence and flowering and maturity (Lee, 2011; Singh et al., 2011; Pagare et al., 2017). Vengris and Damon (1976) claimed that witchgrass normally completes its life cycle between 80 and 105 days. However, later emerged plants only require 35 days from seeding to maturity under warm conditions.
Witchgrass exhibits substantial phenotypic variations and it is well-suited to a broad range of soil types and climatic conditions (Darbyshire and Cayouette, 1995), enabling witchgrass to adapt well in the changing climate. Witchgrass is a C4 grass (Hattersley, 1984). The lower water requirement of plants with the C4 photosynthetic pathway, along with higher optimal temperatures makes it more adaptable to changing climate as compared to C3 plants (Tubiello et al., 2007; Pagare et al., 2017; Ramesh et al., 2017). Increasing numbers of fall panicum (P. dichotomiflorum) have been observed in more northern areas of Europe (Peters et al., 2014). Shahidul et al. (2014) also reported that some tropical and subtropical C4 species could shift northwards to temperate zones. The impact of climate change on the distribution range of witchgrass deserves further investigation.
Understanding weed biology and the timing of phenological development is critical for determining the most effective control timing. Future research on the impact of climate change on weed phenology, morphology, physiology, and reproduction through altered temperature and rainfall patterns will assist in monitoring and adaptive management of the weeds.
Data Availability Statement
The raw data supporting the conclusions of this article will be made available by the authors, without undue reservation.
Author Contributions
HW planned and designed the research. AS and MA performed the experiments and conducted fieldwork. MA and XM analyzed the data. HW and XM wrote the manuscript. All authors contributed critically to the drafts and gave final approval for publication.
Funding
This research has been funded by Grains Research and Development Corporation Australia and NSW Department of Primary Industries.
Conflict of Interest
The authors declare that the research was conducted in the absence of any commercial or financial relationships that could be construed as a potential conflict of interest.
Acknowledgments
Bernie Dominiak provided useful comments on an earlier version of the manuscript.
References
Alex, J. F. (1980). Emergence from buried seed and germination of exhumed seed of Fall panicum. Can. J. Plant Sci. 60, 635–642. doi: 10.4141/cjps80-090
Aliscioni, S. S., Giussani, L. M., Zuloaga, F. O., and Kellogg, E. A. (2003). A molecular phylogeny of Panicum (Poaceae: Paniceae): tests of monophyly and phylogenetic placement within the Panicoideae. Am. J. Bot. 90, 796–821. doi: 10.3732/ajb.90.5.796
AVH (Australia's Virtual Herbarium) (2021). Available online at: https://avh.ala.org.au/occurrences/search?taxa=Panicum+capillare#tab_mapView (accessed January 10, 2021).
Baskin, J. M., and Baskin, C. C. (1986). Seasonal changes in the germination responses of buried witchgrass (Panicum capillare) seeds. Weed Sci. 34, 22–24. doi: 10.1017/S0043174500026370
Brecke, B. J. (1974). Life cycle studies of Panicum dichotomiflorum Michx. and Panicum capillare L. (MS thesis). Cornell University, Ithaca, NY, United States.
Burnside, O. C., Fenster, C. R., Evetts, L. L., and Mumm, R. F. (1981). Germination of exhumed weed seed in Nebraska. Weed Sci. 29, 577–586. doi: 10.1017/S0043174500063761
Byng, J. W. (2014). The Flowering Plants Handbook: A Practical Guide to Families and Genera of the World. Hertford: Plant Gateway.
CHAH (Council of Heads of Australasian Herbaria) (2021). Australian Plant Census. Available online at: https://biodiversity.org.au/nsl/services/APC (accessed January 10, 2021).
Chege, P. G., Clark, T. L., and Hibbard, B. E. (2005). Alternate host phenology affects survivorship, growth, and development of western corn rootworm (Coleoptera: Chrysomelidae) larvae. Environ. Entomol. 34, 1441–1447. doi: 10.1603/0046-225X-34.6.1441
Chen, Y. C., Zhu, X. C., Albrecht, D. E., Loukopoulos, P., Quinn, J. C., and Weston, L. A. (2018). “Identification of eight Panicum species in Riverina region of NSW using DNA sequence analysis,” in Proceedings of the 21st Australasian Weeds Conference (Sydney, NSW),312–217.
Christian, M. L., and Willis, W. G. (1993). Survival of wheat streak mosaic virus in grass hosts in Kansas from wheat harvest to fall wheat emergence. Plant Dis. 77, 239–242. doi: 10.1094/PD-77-0239
Clements, D. R., DiTommaso, A., Darbyshire, S. J., Cavers, P. B., and Sartonov, A. D. (2004). The biology of Canadian weeds. 127. Panicum capillare L. Can. J. Plant Sci. 84, 327–341. doi: 10.4141/P02-147
Coutts, B. A., Strickland, G. R., Kehoe, M. A., Severtson, D. L., and Jones, R. A. C. (2008). The epidemiology of Wheat streak mosaic virus in Australia: case histories, gradients, mite vectors, and alternative hosts. Aust. J. Agric. Res. 59, 844–853. doi: 10.1071/AR07475
Crins, W. J. (1991). The genera of Paniceae (Gramineae: Panicoideae) in the southeastern United States. J. Arnold Arbor. 1, 171–312.
Darbyshire, S. J., and Cayouette, J. (1995). Identification of the species in the Panicum capillare complex (Poaceae) from eastern Canada and adjacent New York State. Can. J. Bot. 73, 333–348. doi: 10.1139/b95-035
Dirkse, G., and Holverda, W. (2016). Panicum capillare L. (Draadgierst) en Panicum barbipulvinatum Nash in Nederland: eerherstel voor een miskende sort. Gorteria 38, 34–42.
Fausey, J. C., and Renner, K. A. (1997). Germination, emergence, and growth of giant foxtail (Setaria faberi) and fall panicum (Panicum dichotomiflorum). Weed Sci. 45, 423–425. doi: 10.1017/S0043174500093097
Fenollosa, E., Jené, L., and Munné-Bosch, S. A. (2020). Rapid and sensitive method to assess seed longevity through accelerated aging in an invasive plant species. Plant Methods 16:64. doi: 10.1186/s13007-020-00607-3
Fernando, N., Humphries, T., Florentine, S. K., and Chauhan, B. S. (2016). Factors affecting seed germination of feather fingergrass (Chloris virgata). Weed Sci. 64, 605–612. doi: 10.1614/WS-D-15-00212.1
Frick, B., Thomas, A., and Wise, R. F. (1990). Weeds of Corn, Soybean, and Winter Wheat Fields Under Conventional, Conservation and No-till Management Systems in Southwestern Ontario 1988 and 1989. Regina, SK: Weed Survey Series, Publication 90-1, Agriculture Canada.
Giannini, A., Biasutti, M., Held, I. M., and Sobel, A. H. (2008). A global perspective on African climate. Clim. Change 90, 359–383. doi: 10.1007/s10584-008-9396-y
Gross, K. L., Maruca, D., and Pregitzer, K. S. (1992). Seedling growth and root morphology of plants with different life-histories. New Phytol. 120, 535–542. doi: 10.1111/j.1469-8137.1992.tb01803.x
Hattersley, P. W. (1984). Characterization of C4 type leaf anatomy in grasses (Poaceae). Mesophyll: bundle sheath area ratios. Ann. Bot. 53, 163–179. doi: 10.1093/oxfordjournals.aob.a086678
IPCC (2007). “Summary for policymakers,” in Climate Change 2007: The Physical Science Basis. Contribution of Working Group I to the Fourth Assessment Report of the Intergovernmental Panel on Climate Change, eds S. Solomon, D. Qin, M. Manning, Z. Chen, M. Marquis, K. B. Averyt, M. Tignor, and H. L. Miller (Cambridge: Cambridge University Press). Available online at: https://www.ipcc.ch/site/assets/uploads/2018/02/ar4-wg1-spm-1.pdf
Jaganathan, G. K., and Liu, B. (2015). Role of seed sowing time and microclimate on germination and seedling establishment of Dodonaea viscosa (Sapindaceae) in a seasonal dry tropical environment—an insight into restoration efforts. Botany 93, 23–29. doi: 10.1139/cjb-2014-0159
Kieckhefer, R. W., and Lunden, A. O. (1983). Host preferences and reproduction of 4 cereal aphids (Hemiptera: Homoptera: Aphididae) on some common weed grasses of the Northern Plains (USA). Environ. Entomol. 12, 986–989. doi: 10.1093/ee/12.3.986
Kingsbury, J. M. (1964). Poisonous Plants of the United States and Canada. Englewood Clifs, NJ: Prentice Hall.
Lee, J. (2011). Combined effect of elevated CO2 and temperature on the growth and phenology of two annual C3 and C4 weedy species. Agric. Ecosys. Environ. 140, 484–491. doi: 10.1016/j.agee.2011.01.013
Liu, D. L., Teng, J., Ji, F., Anwar, M. R., Feng, P. Y., Wang, B., et al. (2020). Characterizing spatiotemporal rainfall changes in 1960–2019 for continental Australia. Int. J. Climatol. 41, E2420–E2444. doi: 10.1002/joc.6855
Llewellyn, R. S., Ronning, D., Ouzman, J., Walker, S., Mayfield, A., and Clarke, M. (2016). Impact of Weeds on Australian Grain Production: The Cost of Weeds to Australian Grain Growers and the Adoption of Weed Management and Tillage Practices Report for GRDC. CSIRO.
Masin, R., Zuin, M. C., Archer, D. W., Forcella, F., and Zanin, G. (2005). WeedTurf: a predictive model to aid control of annual summer weeds in turf. Weed Sci. 53, 193–201. doi: 10.1614/WS-04-066R1
Menzel, A., Sparks, T. H., Estrella, N., et al. (2006a). European phenological response to climate change matches the warming pattern. Glob. Change Biol. 12, 1969–1976. doi: 10.1111/j.1365-2486.2006.01193.x
Menzel, A., Von Vopelius, J., Estrella, N., Schleip, C., and Dose, V. (2006b). Farmers' annual activities are not tracking the speed of climate change. Clim. Res. 32, 201–207. Available online at: https://www.jstor.org/stable/24869308
Michel, B. E. (1983). Evaluation of the water potentials of solutions of polyethylene glycol 8000 both in the absence and presence of other solutes. Plant Physiol. 72, 66–70. doi: 10.1104/pp.72.1.66
Ontario Weed Committee (2021). Witch Grass, Panicum capillare. Available online at: http://www.weedinfo.ca/en/weed-index/view/id/PANCA (accessed April 11, 2021).
Ooi, M. K. J., Denham, A. J., Santana, V. M., and Auld, T. D. (2014). Temperature thresholds of physically dormant seeds and plant functional response to fire: variation among species and relative impact of climate change. Ecol. Evol. 4, 656–671. doi: 10.1002/ece3.973
Otto, S., Masin, R., Chistè, G., and Zanin, G. (2007). Modelling the correlation between plant phenology and weed emergence for improving weed control. Weed Res. 47, 488–498. doi: 10.1111/j.1365-3180.2007.00594.x
Pagare, S., Bhatia, M., Tripathi, N., Pagare, S., and Kumar, B. (2017). Weeds: an introduction and their responses to climate change. Trends Biosci. 10, 2123–2129.
Peters, K., Breitsameter, L., and Gerowitt, B. (2014). Impact of climate change on weeds in agriculture: a review. Agron. Sustain. Dev. 34, 707–721. doi: 10.1007/s13593-014-0245-2
Quinn, J. C., Kessell, A. E., and Weston, L. A. (2014). Secondary plant products causing photo-sensitization in grazing herbivores: their structure, activity, and regulation. Int. J. Mol. Sci. 15, 1441–1465. doi: 10.3390/ijms15011441
Ramesh, K., Matloob, A., Aslam, F., Florentine, S. K., and Chauhan, B. S. (2017). Weeds in a changing climate: vulnerabilities, consequences, and implications for future weed management. Front. Plant Sci. 8:95. doi: 10.3389/fpls.2017.00095
Rivera, C. M., and Peters, R. A. (1971). A preliminary report on formation of seed and germination of fall panicum (Panicum dichotomiflorum Michx.) and witchgrass (P. capillare L.). Proc. NEWCC 25:30.
Seepaul, R., Macoon, B., and Reddy, K. R. (2012). Ecotypic differences in switchgrass seed germination responses to in vitro osmotic stress. Seed Technol. 34, 173–182. Available online at: https://www.jstor.org/stable/23433396
Shahidul, M. H., Eom, M. Y., Uddin, M. R., Park, T. S., Kang, H. W., Kim, D. S., et al. (2014). Weed population dynamics under climatic change. Asian J. Turf Grass Sci. 3, 174–182. doi: 10.5660/WTS.2014.3.3.174
Singh, R. P., Singh, R. K., and Singh, M. K. (2011). Impact of climate and carbon dioxide change on weeds and their management—a review. Indian J. Weed Sci. 43, 1–11.
Stevens, O. A. (1932). The number and weight of seeds produced by weeds. Am. J. Bot. 19, 784–794. doi: 10.1002/j.1537-2197.1932.tb08859.x
Stump, W. L. (1984). Germination, emergence, and seed persistence of Panicum miliaceum L. (Master thesis), Colorado State University, Fort Collins, CO, United States.
Tubiello, F. N., Soussana, J. F., and Howden, S. M. (2007). Crop and pasture response to climate change. Proc. Natl. Acad. Sci. U.S.A. 104, 19686–19690. doi: 10.1073/pnas.0701728104
Varanasi, A., Prasad, P. V. V., and Jugulam, M. (2016). Impact of climate change factors on weeds and herbicide efficacy. Adv. Agron. 135, 107–146. doi: 10.1016/bs.agron.2015.09.002
Vengris, J., and Damon, R. A. Jr. (1976). Field growth of fall panicum and witchgrass. Weed Sci. 24, 205–208. doi: 10.1017/S0043174500065760
Walck, J. L., Hidayati, S. N., Dixon, K. W., Thompson, K., and Poschlod, P. (2011). Climate change and plant regeneration from seed. Glob. Change Biol. 17, 2145–2161. doi: 10.1111/j.1365-2486.2010.02368.x
Weston, L. A., Brown, W., Haque, S., and Broster, H. (2016). Status of Key Summer Fallow Weeds in the Riverina an Update. Available online at: https://grdc.com.au/Research-and-Development/GRDC-Update-Papers/2016/02/Status-of-key-summer-fallow-weeds-in-the-Riverina-an-update (accessed April 11, 2021).
Keywords: germination biology, seed viability, temperature, seedbank, seed burial, field emergence, persistence
Citation: Wu H, Asaduzzaman M, Shephard A and Ma X (2021) Seed Biology of Witchgrass (Panicum capillare L.) Ensures Its Success Under Different Environmental Conditions. Front. Agron. 3:657785. doi: 10.3389/fagro.2021.657785
Received: 24 January 2021; Accepted: 29 March 2021;
Published: 22 April 2021.
Edited by:
Maor Matzrafi, Agricultural Research Organization, Volcani Center, IsraelReviewed by:
Simerjeet Kaur, Punjab Agricultural University, IndiaRoberta Masin, University of Padua, Italy
Copyright © 2021 Wu, Asaduzzaman, Shephard and Ma. This is an open-access article distributed under the terms of the Creative Commons Attribution License (CC BY). The use, distribution or reproduction in other forums is permitted, provided the original author(s) and the copyright owner(s) are credited and that the original publication in this journal is cited, in accordance with accepted academic practice. No use, distribution or reproduction is permitted which does not comply with these terms.
*Correspondence: Hanwen Wu, aGFud2VuLnd1QGRwaS5uc3cuZ292LmF1