- 1Doctoral Research Program on Climate Change and Climate Systems, West African Science Service Center on Climate Change and Adapted Land Use (WASCAL), Department of Meteorology and Climate Science, Federal University of Technology, Akure, Nigeria
- 2Laboratoire de Physique de l’Atmosphere et de l’Ocean Simeon Fongang (LPAO-SF), Ecole Supérieure Polytechnique, Universite Cheikh Anta Diop, Dakar, Senegal
- 3Departamento de Física de la Tierra, Astronomía y Astrofísica I (Geofísica y Meteorología), Universidad Complutense de Madrid, Madrid, Spain
- 4Instituto de Geociencias (IGE), Madrid, Spain
- 5Faculté des Sciences et Techngiques (FST), Université de Labé, Labé, Guinea
- 6Institut Pierre Simon Laplace (IPSL), École Polytechnique, Sorbonne Université, Palaiseau, France
Introduction: Bacterial meningitis outbreak's, associated with high mortality, remain a significant public health challenge in West Africa. The environmental factors and mechanisms that trigger these outbreaks are not yet fully understood.
Methods: This study investigates the seasonal and inter-annual variability of meningitis incidence and its association with environmental variables. The analysis considers two distinct periods, 2006–2009 and 2010–2020, based on the MenAfriVac introduction's in 2010, across two climatic zones: the Sahelian (SAH) and Gulf of Guinea (GG) countries.
Results: The results reveal that changes in the timing and magnitude of meningitis outbreaks between 2006 and 2009 and 2010–2020 are linked to variations in dust distribution, temperature trends, and their impact on relative humidity (RH). High concentrations of particulate matter (PM10) and increased aerosol optical depth (AOD) in January were identified as key precursors of meningitis outbreaks in both regions. In the SAH, meningitis outbreaks and their severity are strongly associated with increased dust levels and temperatures combined with RH below 20%. In contrast, in the GG, outbreaks are driven by high PM10 levels, temperature anomalies, and RH below 45%.
Discussion: The study shows that rising RH and shifts in wind patterns signal the end of the meningitis season. Additionally, vaccination programs slow bacterial interactions with antibodies, delaying disease transmission and prolonging the outbreak period. Despite the availability of vaccines, climate factors remain critical drivers of meningitis outbreaks. Given the ongoing challenges posed by climate change, enhanced surveillance systems and strategic public health interventions are essential for mitigating the impact of future outbreaks.
1 Introduction
Meningitis epidemics occur annually in West Africa during the dry season from January to early May, causing thousands of deaths among children under five and young adults (1–3). Additionally, 50% of survivors suffer from severe long-term neurological sequelae, including cognitive impairment and hearing loss (4–7).
As the most populous region in Africa, West African faces significant challenges in achieving adequate health care (8, 43). At the same time, the emergence and re-emergence of infectious diseases continue to rise globally (9). Bacterial meningitis remains one of the leading causes of mortality and morbidity, disproportionately affecting children under 5 years of age, who bear the greatest burden of disease (2, 10).
The African meningitis belt, which extends from Senegal to Ethiopia (11), is predominantly affected by Streptococcus pneumoniae and Neisseria meningitidis (Nm), which are the primary causes of bacterial meningitis in adults and children older than one year. Pneumococcal meningitis outbreaks occur sporadically, while Nm remains the major pathogen responsible for epidemic of meningitis in this region (2).
Since 2010, 2011 and 2015, several vaccines such have been introduced across the meningitis belt, including MenAfriVac produced by the Serum Institute of India Ltd, which targets Nm serogroup A (NmA) and the pneumococcal conjugate vaccines (PCV-10 and PCV-13), which protect against S.pneumoniae infections (2). Despite the success of these vaccines in eliminating NmA outbreaks and reducing overall meningitis epidemics, new epidemics caused by serogroups C, W135, X and Y continue to occur across sub-Saharan Africa (10).
During the epidemic in 2016–2017, 72% of meningitis cases were attributed to NmC, followed by NmW and NmX, with outbreaks recorded in 11 countries, particularly in Togo, Ghana and Burkina (12). In 2019, a total of 1,171,321 meningitis cases, including 139,590 deaths, were reported across Africa (13). In Burkina, 301 cases were recorded, 103 were due to NmC and 13 to NmX (14). Similarly, in Niger, 559 cases, including 18 deaths, were attributed to NmC between November 2022 and January (15).
Several multidisciplinary studies have suggested climatic conditions as key factors in meningitis outbreaks, seasonality, and hyperendemicity (3, 10, 12, 16–21). These environmental factors influencing meningitis epidemics in the African meningitis belt include surface winds, air temperature variations, relative humidity levels, and surface dust load (3, 22, 23). Agier et al. (20) found that dust concentrations lead in 10-days meningitis incidence in Niger, highlighting dust as a key driver of meningitis seasonality. Similarly, Martiny & Chiapello (19), reported meningitis peaks February and March in Niger and Mali coinciding with cumulative dust exposure. Additional studies in Nigeria and Senegal have also linked poor air quality and intense dust storms to increased meningitis cases (18, 24). Meanwhile, global warming has been shown to significantly heighten the risk of infectious disease outbreaks (25). The increased frequency of dust events facilitates the spread of airborne microorganisms, including bacteria, viruses and parasites, via the trade winds (26). As a result, meningitis epidemics may become more widespread, more intense, and the geographic location of the disease may expand beyond the traditional African meningitis belt.
Although these studies provide strong evidence of environmental influences on meningitis, the mechanisms underlying these relationship remain largely hypothetical. Previous research has also been limited in scope, focusing primarily on national or district-level studies, which fail to capture broader climatic variations across the West African Meningitis Belt (WAMB). Moreover, most studies have concentrated on the most affected regions, without considering potential differences between diverse climatic zones.
The WAMB is defined as a high-incidence and epidemic-prone region, characterized by mean annual rainfall of 300–1,100 mm (27). It is bordered by the Saharan Desert and Bodélé Depression to the north (28) and the Gulf of Guinea savanna in the south. Climate variability plays a crucial role in shaping meningitis patterns in this region. The intertropical convergence zone (ITCZ) and the Intertropical Front (ITF) significantly influence atmospheric circulation affecting surface wind patterns, precipitation levels (29), and dust distribution and concentration (28). As the ITF shifts northward during the dry season, it enhances Harmattan winds, creating the Saharan air layer and triggering high altitude dust events (21). These meteorological conditions align closely with meningitis outbreaks.
Given the availability of meningitis cases data from 2006 to 2020 spanning both pre-and post vaccination, this study seeks to evaluate the impact of climate variability on meningitis patterns across different climatic zones, specifically the SAH and the GG regions. Furthermore, we also explore the robustness of climate-health relationships by analysing separately two different periods according to vaccines introduction.
This study aims to investigate the evolution of meningitis incidence across West African Meningitis Belt (WAMB), focusing on the SAH and GG climatic zones. It examines seasonal and inter-annual climate variability to determine its influence on disease dynamics and compares meningitis patterns before and after vaccines introduction to identify potential shift. By integrating climate variability and vaccines effects, this study provides new insights into the interaction between climate, vaccination and meningitis outbreaks.
The following sections detail our methodology in Section 2, present the results from various statistical analyses in Section 3, and discuss key findings and future research direction in Section 4.
2 Materials and methods
2.1 Materials
2.1.1 Meningitis dataset
Weekly meningitis case data are collected at the country level and shared with the World Health Organization (WHO), which makes this information publicly available through the following link: https://www.menafrinet.org/. For this study, we downloaded data covering the period from January to June (26 weeks per year) from 2006 to 2020, except for Senegal and Guinea, where data were only available from 2010 onward. The selection of these months was based the seasonality of meningitis in most countries within the African Meningitis Belt (AMB) (3, 19, 21).
2.1.2 Environmental dataset
2.1.2.1 Dust dataset
In this study, we utilized two dust aerosol indices: the Aerosol Optical Depth (AOD) and the Particulate Matter index (PM10). AOD data were obtained from the Modern-Era Retrospective Analysis for Research and Applications (30), which provides information on the vertical distribution of atmospheric aerosols. The AOD-550 nm dataset is accessible via at this link: (https://goldsmr4.gesdisc.eosdis.nasa.gov/data/MERRA2/M2I3NXGAS.5.12.4/). The 550 nm wavelength was chosen due to its proximity to visible radiation, which significantly influences visibility during dust events.
PM10 data were obtained from (31) https://ads.atmosphere.copernicus.eu/cdsapp#/), which measures air pollution by quantifying the concentration of particulate matter with a diameter of less than 10 micrometers. A strong correlation exists between PM10 and AOD at 550 nm during boreal winter and spring, with peak values observed in March and April.
To better explore the relationship between dust and meningitis, we combined both parameters. PM10 and AOD, have a temporal resolution of 3 h, with respective horizontal resolutions of 0.75° × 0.75° and 0.5° × 0.625°, respectively.
2.1.2.2 Meteorological datasets
The meteorological variables used in this study include air temperature, zonal (u) and meridional (v) surface wind components, as well as relative humidity (RH). These data were obtained from the (32) hourly data on pressure levels, which provides climate and weather data from 1940 to present. For this study, we selected the 1,000 hPa pressure level, which is closest to the Earth's surface and most relevant for near-surface atmospheric conditions. The datasets are accessible via the following link: https://cds.climate.copernicus.eu/cdsapp#!/dataset/reanalysis-era5-pressure-levels?tab=overview.
3 Methods
In this study, we employed various statistical methods to better understand how environmental conditions influence the temporal and spatial distribution of meningitis over WAMB. The analysis was divided into two main periods based on the introduction of MenAfriVac: the pre-vaccination period (2006–2009) and the post-vaccination period (2010–2020). In addition, the study area was divided into two distinct regions, the SAH and the GG, in which climatic factors and meningitis cases are spatially averaged for the associated countries (Figures 2a–d).
To explore the seasonal cycle, we use weekly values ranging from week 1 to week 26 (January–June) for both environmental variables and meningitis incidence (Figure 3).
To investigate the intra-seasonal variability of environmental conditions (Figures 1b–e), we focused on:
• boreal winter (December, January, February: DJF): during this period, the Sahel is dry and affected by the Harmattan trade winds, while the Gulf of Guinea experiences dry but humid conditions with dust events originating from Bodélé Depression (33)
• boreal spring (March, April, May: MAM): is characterized by extreme heat with increasing humidity in the SAH, whereas the GG enters in the rainy season.
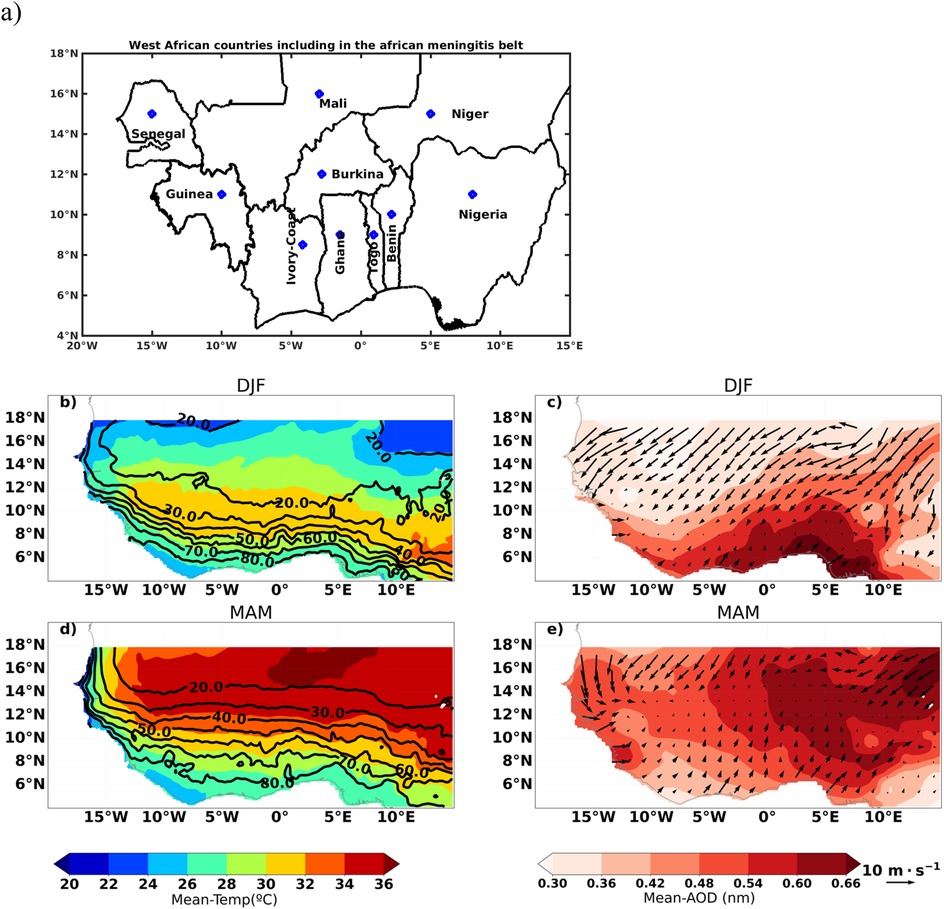
Figure 1. Meningitis belt and climatological environmental conditions. Climatology of intra-seasonal variability of environmental variables over the West African Meningitis Belt (WAMB) averaged for 2006–2020: (a) West African countries included in the African Meningitis Belt, (b) and (d) temperature (shaded) with relative humidity (black contours), and (c) and (e) aerosol optical depth (AOD, shaded) with wind vectors (arrows). Panels (b) and (c) represent the December–January–February (DJF) season, while panels (d) and (e) correspond to March–April–May (MAM).
For the inter-annual variability analysis (Figures 4, 5), we applied the Empirical Orthogonal Functions (EOF) analysis, also known as Principal Component Analysis (PCA) (3, 34). Given an environmental variable Y with (space, time), dimensions, the PCA methodology involves the following step:
1. Calculating the weekly standardized anomalies of Y by subtracting for each grid point, the mean of each week over all years from the respective weekly values.
2. Calculating the covariance matrix of Y as C = Y*Y' (Y' being the transpose of Y and Y dimensioned in [space (rows), time (columns)].
3. Diagonalizing the matrix C computing the the eigenvalues, eigenvectors (EOFs), and principal components (PCs) of Y, considering the SAH and GG regions separately over the entire study period.
4. Selecting the leading modes of variability of Y and investigating their impact on meningitis, relating the PCs with the standardized weekly anomalies of cases.
To quantify the relationship between environmental variability and meningitis incidence, we performed a Spearman correlation analysis between the first PCs of each environmental variable and the weekly standardized anomalies of meningitis cases (w1–w26).
The results are presented in Tables 1, 2.
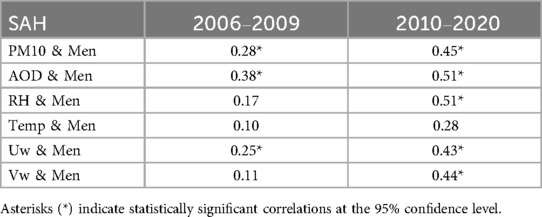
Table 1. Spearman correlation between the leading principal component (PC1) of each environmental variable and the normalized anomalous meningitis cases over the sahel (SAH) for the periods 2006–2009 (pre-vaccine) and 2010–2020 (post-vaccine).
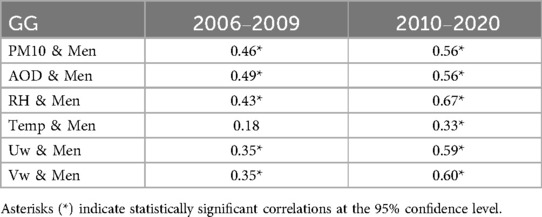
Table 2. Spearman correlation between the leading principal component (PC1) of each environmental variable and the normalized anomalous meningitis cases over the Gulf of Guinea (GG) for the periods 2006–2009 (pre-vaccine) and 2010–2020 (post-vaccine).
To explore the precursor role of environmental variables on the disease incidence, a led-lag correlation analysis was conduced.
• For the SAH region, the anomalous meningitis cases index was fixed in MAMJ (week 9 to week 24) while the lags were calculated over a period of 16 weeks, moving backward one week at a time. For instance, lag −1 is corresponds to weeks 8–23, lag −2 to weeks 7–22, and so on.
• For the GG region, the anomalous cases index was fixed in FMAMJ (week 5 to week 24) with lags calculated over 20 weeks, shifting one week backward. Thus, lag −1 corresponds to weeks 5–23, lag −2 to weeks 4–22 and so on.
Finally, a Student's t-test was applied to assess the statistical significance of the correlations calculated at a 90% confidence level.
4 Results
4.1 Meningitis belt and climatological environmental conditions
The spatial extension of the meningitis belt in West Africa, along with the climatological values of key environmental variables (temperature, RH, surface winds) in boreal winter and spring is depicted in Figure 1. Countries within the “meningitis belt” extending from Senegal in the west to Ethiopia are also shown. This region includes countries such as Mali, Niger, Nigeria, Burkina Faso, Ivory Coast, Ghana, Togo, and Benin (Figure 1a).
During boreal winter (DJF), warm temperatures ranging from 28°C to 32°C are observed in the northern and central parts of the GG. These conditions are associated with relative humidity levels between 30% and 80% and high dust concentrations originating from the Bodélé Depression, which increase from the northern GG region towards the south (Figures 1b,c).
During boreal spring (MAM), temperatures rise progressively from the GG region to the SAH, where they exceed 37°C. Conversely, relative humidity increases from 20% to 40% in the SAH, while it remains between 50% and 80% in the GG zone. However, Aerosol Optical Depth (AOD) increases significantly in the SAH, indicating higher dust concentrations (Figures 1d,e).
The position of the Intertropical Front (ITF) located at 8°N during DJF, and shifts northward to approximately 13°N in MAM (Figures 1c–e).
In conclusion, dust particles observed at the surface during DJF, are lifted into higher atmospheric layers by the end of May, significantly influencing meteorological conditions during the dry season.
4.2 Seasonal cycle of meningitis in WAMB before and after the vaccination
Weekly meningitis cases reported from 2006 to 2009, prior to the introduction of MenAfriVac, reveal that the SAH countries (Figure 2a) experienced the highest burden of the disease, with a weekly average exceeding 2,000 cases, whereas the GG countries (Figure 2c) recorded a significantly lower incidence, averaging fewer than 100 cases per week. The epidemic peak in the SAH region occurred between the 4th week of March and the 2nd week of April, in contrast to the GG region, where peaks were observed earlier, between the 3rd and 4th weeks of February.
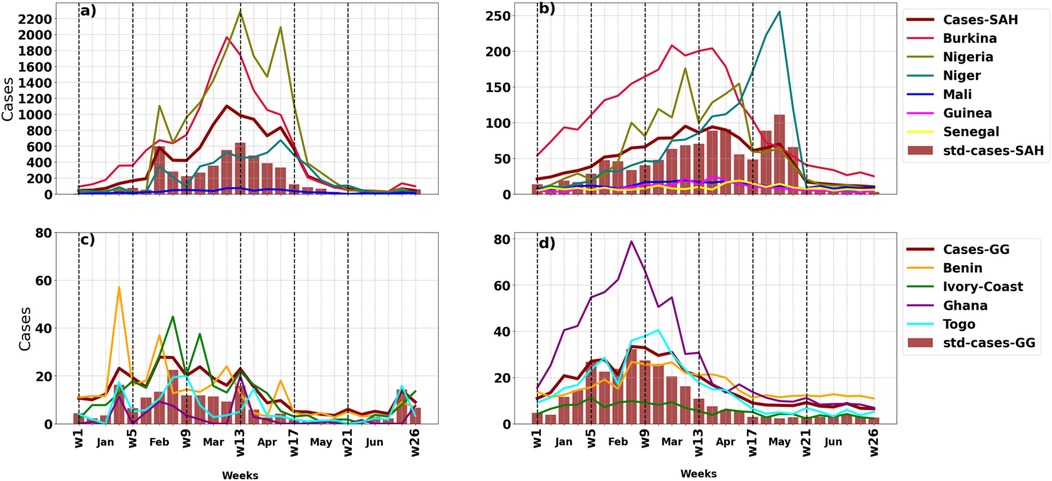
Figure 2. Seasonal cycle of meningitis in WAMB before and after the vaccination. Seasonal cycle of meningitis prevalence from January to June (weeks 1 to 26) between 2006 and 2020 in the two West African climate zones, (a) and (b) for Sahel (SAH) and (c) and (d) for Gulf of Guinea (GG), during the pre- and post-MenAfriVac periods: 2006–2009 (a,c) and 2010–2020 (b,d). The upper panels represent the Sahelian countries, while the lower panels show the Gulf of Guinea countries. The solid brown lines indicate the average prevalence for each region, with error bars representing the standard deviation. Each country is represented by a distinct color.
From 2010 onward, a shift in the disease pattern is evident, with a significant reduction in the number of recorded meningitis cases in the SAH (Figure 2b), due to the introduction of the vaccination.
Conversely, the GG experienced an increase and extension in the duration of the epidemic, with a consistent average number of cases between 2006 and 2009 and 2010–2020, except in Ivory Coast where the trend differed (Figure 2b). The maximum number of cases were recorded from the 2nd week of February to the 4th week of May in the SAH and from the week 4 of January to week 4 of April (corresponding to W13) in the GG region.
In summary, the periods 2006–2009 and 2010–2020 show notable changes in meningitis patterns, both in terms of the weekly number of cases and the overall magnitude of the disease throughout the season.
4.3 Seasonal cycle of environmental parameters and mean cases in WAMB before and after the vaccination
Figure 3 presents a cross-analysis of environmental variables and meningitis cases in the Sahel (SAH, left panel) and Gulf of Guinea (GG, right panel) regions. The results indicate that the onset of the meningitis season is preceded by high dust concentration events in January in both regions (Figures 3e,f), corresponding to an increase in temperature and a decrease in relative humidity (RH) (Figures 3a,b). Remarkably, the increase in surface dust concentration until boreal spring seems to influence in the duration and intensity of the meningitis season (Figures 3e,f). Peak PM10 concentrations are observed in March, while Aerosol Optical Depth (AOD) peaks in April. In the SAH, meningitis cases peak at week 12, typically preceded by a peak in PM10 at week 11 and coinciding with the AOD peak for the period 2006–2009, when RH begins to exceed 38%. In contrast, meningitis peaks occur earlier, around week 8, in the GG region and are associated with RH levels above 45%.
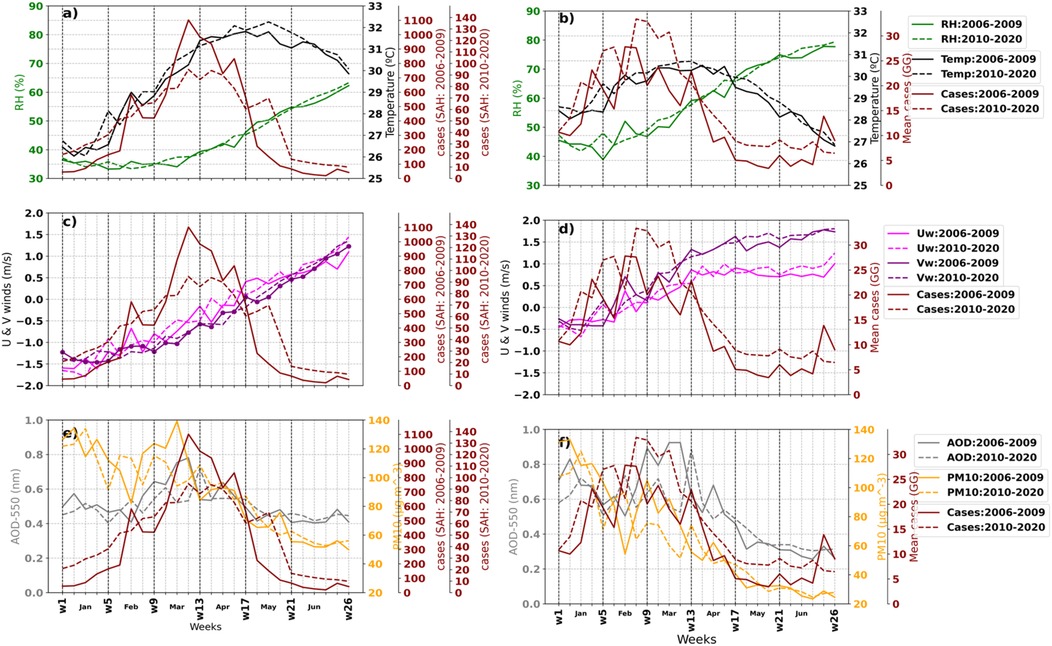
Figure 3. Seasonal cycle of environmental parameters in WAMB before and after the vaccination. Seasonal cycles of environmental variables (weeks 1 to 26) and mean meningitis cases (brown line) over the Sahel (SAH) for and Gulf of Guinea (GG: b–d,f) zones from January to June, before and after the introduction of MenAfriVac. The pre-vaccination period (2006–2009) is represented by solid lines, while the post-vaccination period (2010–2020) is shown with dashed lines. The left panel (a,c,e) illustrates environmental variables for the SAH, whereas the right panel (b,d,f) presents data for the GG. Variables include temperature (black), relative humidity (green), meridional wind (purple), zonal wind (magenta), and dust concentrations, represented by aerosol optical depth (AOD, gray) and PM10 (orange).
A reversal in the surface wind direction (Figures 4c,d) together with increased RH (Figures 4a,b) mark the end of the meningitis season. In the GG region, the meningitis season concludes earlier, around the 4th week of April, when PM10 concentrations have already decreased significantly.
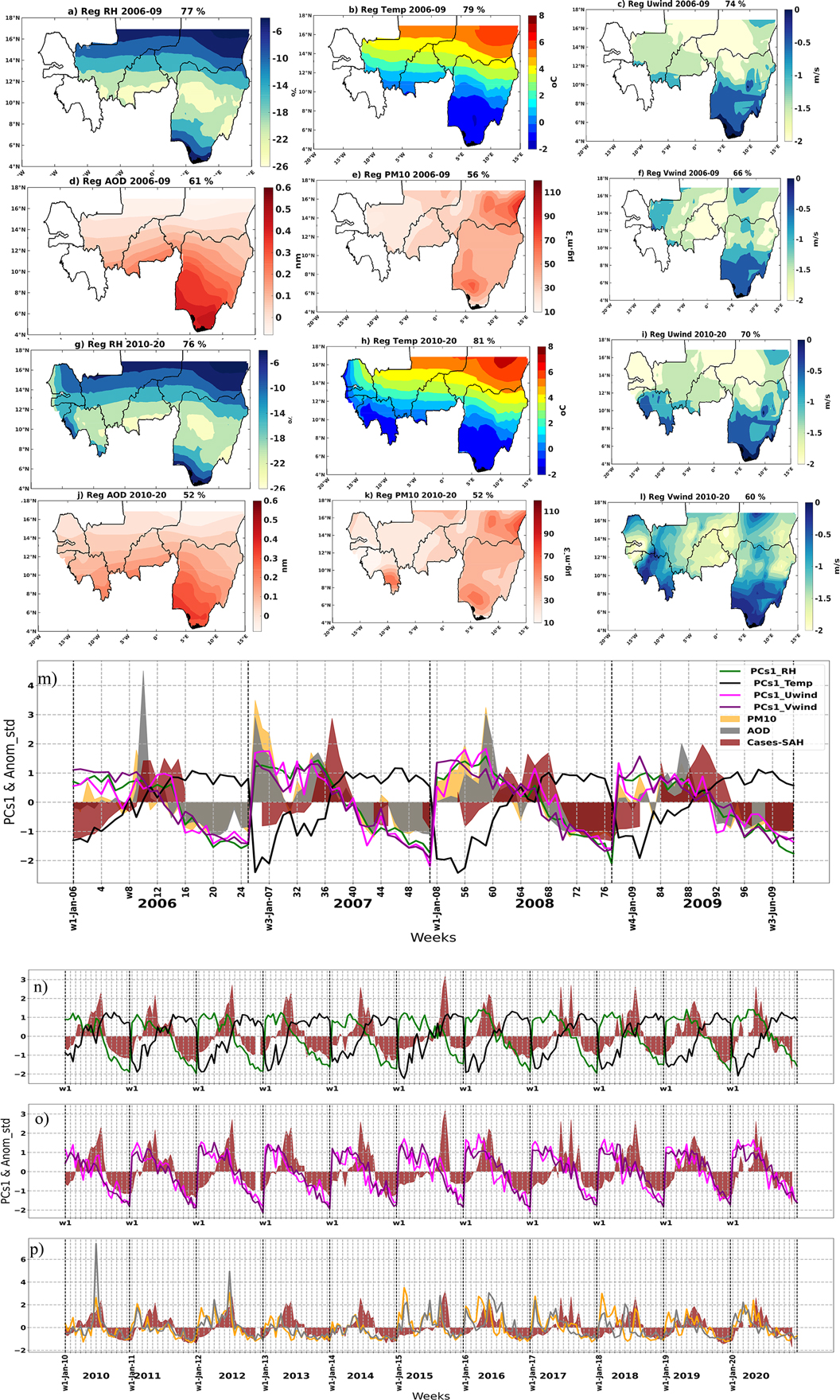
Figure 4. Inter-annual variability modes for environmental parameters in SAH before and after vaccination. Regression between anomalous environmental variables and the leading mode (PC1) from the principal component analysis (PCA) of the corresponding variables from January to June for both study periods: 2006–2009 (a–f,m) and 2010–2020 (g–l,n–p) over the Sahel (SAH) at an inter-annual scale. The PC1 of temperature (black solid line), relative humidity (green), meridional wind (purple), zonal wind (magenta), and dust concentrations, represented by aerosol optical depth (AOD, gray) and PM10 (orange), are plotted alongside normalized anomalous meningitis cases (brown shading). The regression results are displayed on the maps. Due to missing meningitis data, Senegal and Guinea are excluded from the 2006–2009 analysis, and consequently, environmental variables for these countries were not considered in that period.
Notice that from 2010, the number of cases substantially decrease in SAH region because of vaccination (dashed brown line, Figure 3a). Nevertheless, there is still a seasonal cycle of the cases in which dry, and dusty environmental conditions enhance the meningitis along both study periods and wet, and reduced dust conditions reduce the disease.
4.4 Inter-annual environmental variability in SAH and GG before and after vaccination
In this section, we explore the climatic patterns driving the emergence of meningitis outbreaks over the SAH and GG regions. To this end, the leading modes of inter-annual variability of environmental factors were computed before and after the introduction of vaccines (Figure 4).
During 2006–2009, the dominant environmental mode, which accounts for 56%–79% of the total variability, is characterized by anomalous northeasterly winds (Figures 4c,f), lower relative humidity (RH) (Figure 4a), higher concentrations of PM10 and AOD (Figures 4d,e), and elevated air temperatures (Figure 4b). Notably, air temperature and dust patterns exhibit a meridional gradient, with maximum positive values in the northeastern SAH region (Figures 4b,d,e). This spatial configuration aligns with Saharan dust transport by Harmattan winds, inducing local warm and dry conditions.
Interestingly, a similar mode of environmental variability persists after vaccination (Figures 4g–l), although with a reduction in meridional wind intensity (Figure 4l), leading to less southward transport of Saharan dust between 2010 and 2020 (Figures 4J,k).
Regarding temporal evolution, these climate variability modes appear to precede meningitis outbreaks in both study periods in the SAH (Figures 4m–p). Before vaccination programmes, only dust concentrations and zonal wind exhibited significant correlations with meningitis cases, while the association with air temperature was negligible (Table 1). However, during 2010–2020, all environmental factors showed stronger and more significant correlations with meningitis cases (Table 1) emphasizing the precursor role of climatic factors in the emergence of the disease.
Notably, the timing and intensity of meningitis outbreaks display considerable variability, with early outbreaks in 2011 and 2016 contrasting with delayed ones in 2015 and 2017 (Figure 4n). Interestingly, the most intense meningitis outbreaks coincide with persistent northeasterly winds and prolonged dusty conditions, as observed in 2012, 2015, and 2017, among other years (Figures 4o,p).
In summary, our findings demonstrate a strong and persistent influence of environmental factors on the timing and magnitude of meningitis outbreaks in the SAH region, even after the introduction of vaccines.
In the GG region, the leading mode of variability, which accounts for 60%–88% of the total variance, is characterized by northeasterly winds weakening toward the equator. These winds tend to converge over the 6°N–8°N latitudinal band, where maximum dust concentrations are observed (Figures 5c–f,i–l). The GG region experiences low relative humidity and warm conditions, with maximum values recorded in the northernmost area (Figures 5a,b,g,h).
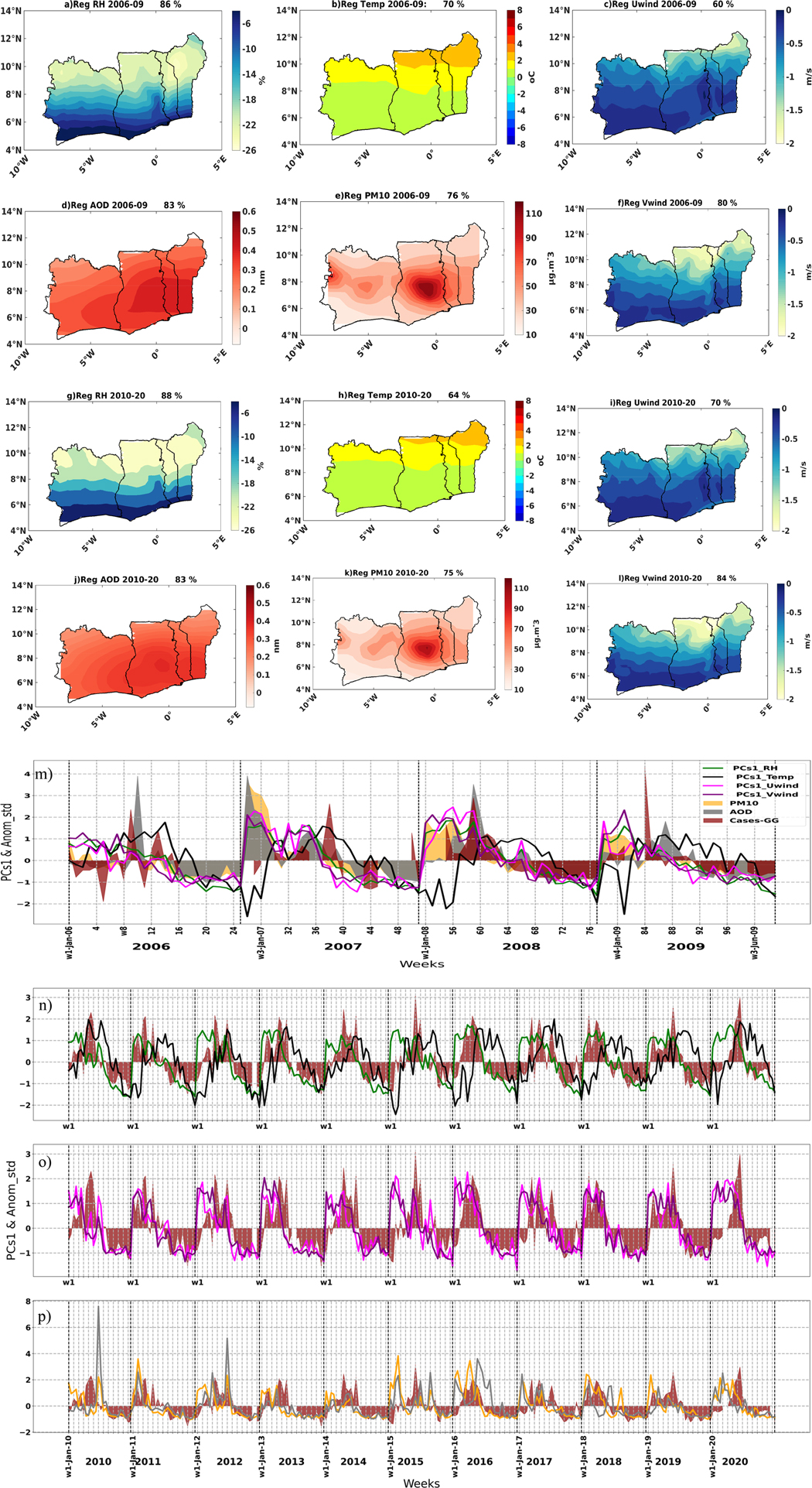
Figure 5. Inter-annual variability modes for environmental parameters in GG before and after vaccination. Regression maps showing the relationship between anomalous environmental variables and the leading principal components (PC1) of the corresponding inter-annual variability mode from January to June over the Gulf of Guinea (GG) for the periods 2006–2009 (a–f,m) and 2010–2020 (g–l,n–p). The PC1s of temperature (black solid lines), relative humidity (green), meridional wind (purple), zonal wind (magenta), and dust concentrations, represented by aerosol optical depth (AOD, gray) and PM10 (orange), are plotted alongside normalized anomalous meningitis cases (brown shading). The regression results are visualized on the maps.
This spatial configuration highlights the transport of substantial amounts of dust from the desert to the GG region, contributing to warm and dry local conditions. Despite these similarities, after vaccination, dust concentration anomalies were weaker and shifted northward (Figures 5j,k), accompanied by an intensification of drier conditions (Figure 5g).
It is worth noting that these favorable climatic factors not only precede but also persist during disease outbreaks (Figures 5m–p). In fact, environmental interannual variability exhibits a pronounced and persistent impact on meningitis in the GG region, as reflected in the high and significant correlation scores observed both before and after vaccination (Table 2). Notably, the influence of air temperature on meningitis became significant only in the post-vaccination period (2010–2020).
Similar to the SAH region, GG countries exhibit substantial variability in meningitis timing, with certain outbreaks occurring early in the dry season (e.g., 2011 and 2018), shorter episodes in late spring (e.g., 2015 and 2020), and prolonged meningitis outbreaks extending throughout the entire dry season (e.g., 2012, 2017, and 2019) (Figure 5n).
Our results demonstrate the key role of climatic variables in meningitis incidence across the whole West African Meningitis Belt, independent of the vaccination campaign. These findings open new opportunities for anticipating disease outbreaks. In this sense, the relative contribution of each environmental factor and the associated lead time are explored in the following section.
4.5 Environmental precursors of meningitis in SAH and GG
In the previous section, we provided evidence about the precursor role of environmental in meningitis outbreaks in both the SAH and GG regions. However, it is necessary to determine the lead times for each climatic predictor more precisely, as this information is crucial for integration into early warning systems. To this end, anomalies in meningitis cases were fixed in FMAMJ for GG and MAMJ for SAH, based on the disease peak identified in Figure 2. The environmental PC1s were then lagged from 0 to week 4 (GG) and from 0 to week 8 (SAH). These represent negative lags, as they evaluate environmental conditions preceding meningitis incidence.
In SAH, during 2006–2009, significant positive correlations were found between surface winds, dust (AOD and PM10), and relative humidity (RH) from lag −8 to lag −2, and from lag −8 to lag 0, respectively (solid lines, Figure 6a). Notably, maximum correlation scores occur at lag −6, except for PM10, which peaks at lag −3, suggesting that climatic information up to six months in advance could help anticipate disease emergence. Notably, air temperature exhibits significant positive scores only at lag 0 (solid dark line), indicating its limited predictive role.
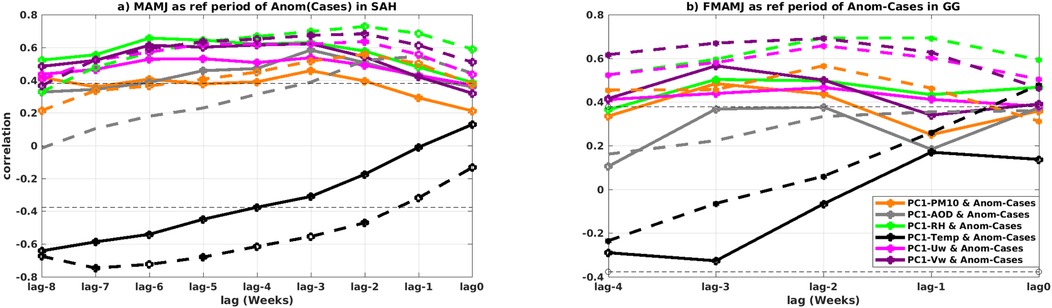
Figure 6. Environmental precursors of meningitis in SAH and GG. Lagged correlations between the leading principal component (PC1) of each environmental variable and the normalized anomalous meningitis cases, with cases fixed at MAMJ and FMAMJ for the Sahel (SAH, a) and Gulf of Guinea (GG, b), respectively. The analysis covers the pre-vaccine (2006–2009, solid lines) and post-vaccine (2010–2020, dashed lines) MenAfriVac periods. Negative lags represent the environmental PC shifted from 1 to 4 weeks (GG) or 1 to 8 weeks (SAH) before the peak of meningitis, which is set as lag 0 (MAMJ for SAH and FMAMJ for GG). Dashed thin horizontal lines indicate the 95% confidence level for statistical significance based on a t-test.
During 2010–2020, zonal and meridional winds, along with RH, continue to show significant positive correlations with meningitis cases from lag −8 to lag 0 (dashed pink, purple, and green lines, Figure 6a). However, dust concentrations display shorter lead times, correlating with meningitis cases from lag −4 to lag 0 (dashed gray and orange lines, Figure 6a). Consequently, after vaccination, the strongest correlations (ranging from 0.5 to 0.8) for environmental precursors occur approximately two months before the meningitis peak (Figure 6). No significant correlation is observed between air temperature and meningitis cases after vaccination (dashed dark line, Figure 6a).
In GG, before vaccination, significant correlations were observed for surface winds (lag −4 to lag −2), PM10 (lag −3 to lag −2), and RH (lag −4 to lag 0) (solid lines, Figure 6b). However, AOD and air temperature do not exhibit significant correlations with meningitis cases (gray line). The optimal lead time for early warning systems appears to be lag −3, based on the highest correlation scores.
During the 2010–2020 period, similar to SAH, positive correlations between zonal wind, meridional wind, PM10, and RH persist from lag −1 to lag −4 (dashed pink, purple, and green lines). Maximum correlation scores occur at lag −2 (ranging from 0.5 to 0.7), suggesting that meningitis outbreaks could be predicted approximately two weeks in advance based on environmental information (Figure 6b). Regarding air temperature, significant correlations emerge at lag −1, suggesting its contribution to a favorable environment for meningitis outbreaks after vaccination (dashed dark line, Figure 6b).
In summary, our results highlight climatic factors as fundamental drivers of meningitis outbreaks in SAH and GG. Moreover, environmental information from 2 to 6 weeks in advance could aid in anticipating meningitis outbreaks in these vulnerable regions.
5 Discussion
The present study examines the incidence of meningitis and the influence of environmental factors across countries in the West African Meningitis Belt (WAMB) from 2006 to 2020. This region encompasses diverse climatic zones and has undergone several vaccination campaigns since 2010. Accordingly, the study period was divided into two segments: before and after the introduction of bacterial meningitis vaccines. This fact let us test if the environmental conditions play a role in the variability of the disease. Results show that, regardless of the vaccination, environmental factors affect meningitis outbreaks.
The study area was subdivided into two climatic zones: the Sahel (SAH), a vast and highly vulnerable semi-arid region, and the Gulf of Guinea (GG), a humid tropical savanna zone.
During the dry season, meningitis epidemics are predominantly observed in regions near desert dust sources, within the dust belt (44, 45), particularly affecting the northern parts of GG countries. The 2006–2009 and 2010–2020 periods show notable changes in meningitis patterns, both in terms of weekly case numbers and the overall magnitude of the disease from onset to conclusion.
Dust episodes in January are identified as triggers for the meningitis season, a finding consistent with Martiny & Chiapello (19) and Sultan et al. (3), who demonstrated that dust plays a key role in the onset of meningitis. High temperatures and low relative humidity, along with peak dust levels in March, are closely associated with epidemic progression. During the post-vaccination period, meningitis peaks occur in March and April, aligning with Deroubaix et al. (21).
The inter-annual variability of environmental variables reveals a dominant mode characterized by northeasterly winds transporting high dust concentrations to the SAH and GG regions, where dry and warm conditions prevail. This mode persists before and after the vaccination period, suggesting a strong link between climatic conditions and meningitis outbreaks.
Additionally, high temperatures and excessive humidity favor the survival, reproduction, and growth of microorganisms such as bacteria, viruses, and parasites (35). Conversely, low humidity dries out respiratory mucous membranes and affects immune function, increasing vulnerability to inflammatory infectious diseases (36). Moreover, high temperatures and dust concentrations encourage indoor gatherings, facilitating meningitis transmission. These environmental conditions impair immune defenses and damage respiratory mucous membranes, heightening susceptibility to infection.
Our findings align with previous studies highlighting the role of environmental conditions in infectious respiratory diseases (3, 10, 19, 22, 35, 36). However, our study provides additional insights:
A comprehensive climate-meningitis analysis across the entire WAMB region.
Changes in the seasonal cycle and variability of environmental factors before and after vaccination.
The robustness of the climate-meningitis relationship despite the presence of multiple vaccines against bacterial meningitis, predominantly caused by Neisseria meningitidis (Nm) and Streptococcus pneumoniae in the African meningitis belt.
More importantly, surface winds, dust concentrations, and relative humidity serve as key precursors of meningitis outbreaks. These climatic variables enable early anticipation of meningitis cases—2–3 weeks in advance for dust concentrations and 4–6 weeks in advance for winds and relative humidity (Figure 6).
Unlike previous studies, our results suggest a weak relationship between air temperature and meningitis emergence in SAH and GG (37, 38). Changes in surface winds and dust transport over WAMB may be linked to large-scale atmospheric patterns, influenced by local and remote climatic forces such as the El Niño-Southern Oscillation (ENSO) (42), the Intertropical Convergence Zone (ITCZ), and the North Atlantic Oscillation (NAO) (39–41). These relations should be checked in future studies.
The findings of this study underscore the critical role of environmental factors in the epidemiology of meningitis in West African countries. However, in the context of ongoing climate change, there is an urgent need to assess its potential impact on meningitis outbreaks. Rising temperatures, shifting precipitation patterns, and increased variability in atmospheric circulation are expected to intensify environmental conditions that favor meningitis transmission. As temperatures rise and humidity decreases, conditions conducive to bacterial survival and transmission could expand beyond the traditional African meningitis belt, potentially increasing the geographic reach of the disease. Moreover, changes in wind patterns may alter the transport and deposition of dust, a key environmental driver of meningitis outbreaks. Future research should explore how projected climate scenarios may influence meningitis seasonality, intensity, and distribution, allowing for the development of adaptive public health strategies and early warning systems.
It is also important to mention that regarding the meningitis patterns in the Sahel (SAH) as well as the Gulf od Guinea (GG) countries for the year 2020, we observe a delay in the onset of disease compared with previous years and the meningitis season was also significantly shorter. As result, that could be related to the beginning and spread of COVID-19 pandemic in Africa. Moreover, several implementation of containment measures in West African countries including a reduction of human mobility, school closures and restrictions on large gatherings, could have influenced reduce the transmission by reducing the spread of meningitis. Therefore, it will be interesting to investigate the effects of SARS-CoV-2 on other pathogen particularly on others infectious diseases.
This study presents several key strengths that contribute to the field of climate-health research. First, the analysis integrates a robust statistical framework, including principal component analysis (PCA) and lead-lag correlation methods, to assess the relationship between environmental variables and meningitis outbreaks. Second, the study spans an extensive period (2006–2020), allowing for a comparative analysis of meningitis patterns before and after vaccine introduction. Third, the inclusion of two distinct climatic zones, the Sahel (SAH) and Gulf of Guinea (GG), provides a broader understanding of how environmental variability influences disease dynamics across different ecological contexts.
Despite these strengths, the study has certain limitations that should be acknowledged. The availability and quality of health data pose a significant challenge, as gaps in meningitis case records, particularly before 2010, may introduce bias in trend analysis. Additionally, while satellite-derived dust (AOD, PM10) and reanalysis meteorological data offer valuable insights, they may not fully capture localized environmental conditions that influence meningitis outbreaks. Furthermore, the study focuses primarily on climatic drivers, without incorporating socio-economic, demographic, and healthcare access factors, which also play a crucial role in disease transmission and mitigation. Future studies could benefit from integrating climate-health models with socio-environmental determinants to provide a more comprehensive approach to meningitis risk assessment.
6 Conclusion
This study provides critical insights into meningitis dynamics in West Africa, emphasizing the significant impact of vaccination on extending disease duration and delaying transmission. Additionally, it identifies dust events in January as key triggers for meningitis outbreaks in both the SAH and GG regions.
However, in the SAH region, the persistence of high temperatures, low relative humidity (<20%), and intensified surface dust concentrations prolongs disease prevalence. Meanwhile, in the GG region, PM10, moderate relative humidity (<45%), and maximum temperatures—though generally lower than in SAH—are critical factors shaping the meningitis season.
At the inter-annual scale, dominant climatic modes are linked to northeasterly winds transporting high dust concentrations over WAMB, creating a dry and warm environment that favors meningitis outbreaks. Notably, anomalous PM10, AOD, RH, and surface winds emerge as key drivers of meningitis incidence, with predictive lead times of 3–6 weeks.
As human health remains a top priority under the WHO's “Defeating Meningitis by 2030” global roadmap, this study contributes to a better understanding of how meteorological conditions influence meningitis outbreaks. The findings suggest that combining climate information with vaccine impact assessments is essential for designing more effective strategies to prepare for, prevent, and mitigate the effects of the disease, ultimately protecting vulnerable population.
Data availability statement
Publicly available datasets were analyzed in this study. This data can be found at the following links: WHO meningitis data: https://www.menafrinet.org/who-meningitis-bulletins MERRA2: https://disc.gsfc.nasa.gov/datasets?keywords=Aerosols&page=1 PM10: https://ads.atmosphere.copernicus.eu/datasets/cams-global-reanalysis-eac4?tab=download ERA5: https://cds.climate.copernicus.eu/datasets/reanalysis-era5-single-levels?tab=download.
Ethics statement
Ethical approval was not required for the study involving humans in accordance with the local legislation and institutional requirements. Written informed consent to participate in this study was not required from the participants or the participants' legal guardians/next of kin in accordance with the national legislation and the institutional requirements.
Author contributions
DD: Conceptualization, Data curation, Formal analysis, Investigation, Methodology, Project administration, Visualization, Writing – original draft, Writing – review & editing. MM-R: Conceptualization, Data curation, Formal analysis, Investigation, Methodology, Project administration, Writing – original draft, Writing – review & editing. BR-F: Conceptualization, Data curation, Formal analysis, Investigation, Methodology, Project administration, Resources, Supervision, Validation, Writing – original draft, Writing – review & editing. ID: Conceptualization, Formal analysis, Investigation, Methodology, Project administration, Writing – review & editing. CD: Conceptualization, Data curation, Formal analysis, Investigation, Methodology, Writing – original draft, Writing – review & editing. AG: Conceptualization, Formal analysis, Investigation, Project administration, Resources, Supervision, Writing – review & editing. AA: Conceptualization, Investigation, Resources, Supervision, Validation, Writing – review & editing.
Funding
The author(s) declare that financial support was received for the research and/or publication of this article. This study was done under the Doctoral Research Program in the West African Climate System (DRP-WACS) of the West African Science Service Centre on Climate Change and Adapted Land Use (WASCAL) sponsored by the German Federal Ministry of Education and Research. M.M.R. has been supported by Ramón y Cajal (RYC2022-038454-I, funded by MCIN/AEI/10.13039/501100011033 and co-funded by the FSE+, European Union) and the Spanish project DISTROPIA (PID2021-125806NB-I00).
Acknowledgments
The authors express their gratitude to WASCAL whose scholarship awarded enabled the student to produce this paper. The authors also extend their thanks to the Department of Meteorology and Geophysics, Faculty of Physics of the Universidad Complutence de Madrid (UCM) for their support and hospitality toward the student during the research process, as well as the Laboratory of Atmospheric Physics and Oceanography–Simeon Fongang (LPAO-SF) for their invaluable collaboration and provision of data resources. This research has been also developed during Diarra Diouf scientific stays at Universidad Complutense de Madrid, funded by Erasmus + program “Higher Education Learning Agreement for Studies” and the next cooperational projects: COOPB24107: “Collaboration with Dakar Universities in climate change variability and social impacts” funded by the CSIC; COOPB20632: “Study of Climate Variability in West Africa for a Society for Climate Variability in West Africa”. “UCadMet: Proyecto de colaboración educativa, científica e innovación tecnológica sobre la meteorología y el clima del Sahel. UCAD (Dakar) y UCM (Madrid)”. XIX Convocatoria UCM de Proyectos de Cooperación al Desarrollo and “UCadMet: Plataforma de colaboración científica, educativa y de innovación tecnológica entre UCAD (Dakar) y UCM (Madrid) sobre meteorología y clima (XX Convocatoria UCM de Proyectos de Cooperación al Desarrollo 2024).
Conflict of interest
The authors declare that the research was conducted in the absence of any commercial or financial relationships that could be construed as a potential conflict of interest.
Generative AI statement
The author(s) declare that no Generative AI was used in the creation of this manuscript.
Publisher's note
All claims expressed in this article are solely those of the authors and do not necessarily represent those of their affiliated organizations, or those of the publisher, the editors and the reviewers. Any product that may be evaluated in this article, or claim that may be made by its manufacturer, is not guaranteed or endorsed by the publisher.
References
1. WHO. Meningitis: overview (2023). Available at: https://www.menafrinet.org/ (Accessed August 10, 2024).
2. Barichello T, Rocha Catalão CH, Rohlwink UK, Kuip MVD, Zaharie D, Solomons RS, et al. Bacterial meningitis in Africa. Front Neurol. (2023) 14:822575. doi: 10.3389/fneur.2023.822575
3. Sultan B, Labadi K, Guégan JF, Janicot S. Climate drives the meningitis epidemics onset in West Africa. PLoS Med. (2005) 2(1):e6. doi: 10.1371/journal.pmed.0020006
4. Gabriel-Job N, Wobo NK. Profile of paediatric neurologic emergencies at the children emergency ward in a tertiary hospital in Port Harcourt, Nigeria. Int J Contemp Pediatr. (2023) 10:1176–80. doi: 10.18203/2349-3291.ijcp20232232
5. Farmen K, Tofiño-Vian M, Iovino F. Neuronal damage and neuroinflammation, a bridge between bacterial meningitis and neurodegenerative diseases. Front Cell Neurosci. (2021) 15:680858. doi: 10.3389/fncel.2021.680858
6. Svendsen MB, Ring Kofoed I, Nielsen H, Schønheyder HC, Bodilsen J. Neurological sequelae remain frequent after bacterial meningitis in children. Acta Paediatr. (2020) 109(2):361–7. doi: 10.1111/apa.14942
7. Lucas MJ, Brouwer MC, van de Beek D. Neurological sequelae of bacterial meningitis. J Infect. (2016) 73(1):18–27. doi: 10.1016/j.jinf.2016.04.009
8. Adebisi YA, Alaran A, Badmos A, Bamisaiye AO, Emmanuella N, Etukakpan AU, et al. How West African countries prioritize health. Trop Med Health. (2021) 49:1–7. doi: 10.1186/s41182-021-00380-6
9. Zumla A, Hui DS. Emerging and reemerging infectious diseases: global overview. Infect Dis Clin North Am. (2019) 33(4):xiii–xix. doi: 10.1016/j.idc.2019.09.001
10. Dione C, Talib J, Bwaka AM, Kamga AF, Bita Fouda AA, Hirons L, et al. Improved sub-seasonal forecasts to support preparedness action for meningitis outbreak in Africa. Clim Serv. (2022) 28:100326. doi: 10.1016/j.cliser.2022.100326
11. WHO. Control of Epidemic Meningococcal Disease. WHO Practical Guidelines. 2nd ed. Geneva: World Health Organization (1998).
12. Broutin H, Thiongane O, Agier L, Van-Engelgem I, Ghomsi JPJ, Ouattara A, et al. Fighting meningitis in Africa: a call for a multi sectorial action. J Trop Med Health. (2018) 2018(03). doi: 10.29011/JTMH-129.000029
13. Kwambana-Adams BA, Liu J, Okoi C, Mwenda JM, Mohammed NI, Tsolenyanu E, et al. Etiology of pediatric meningitis in West Africa using molecular methods in the era of conjugate vaccines against pneumococcus, meningococcus, and Haemophilus influenzae Type b. Am J Trop Med Hyg. (2020) 103(2):696. doi: 10.4269/ajtmh.19-0566
14. Kekeisen-Chen JF, Tarbangdo FT, Sharma S, Marasini D, Marjuki H, Kibler JL, et al. Expansion of Neisseria meningitidis serogroup C clonal complex 10217 during meningitis outbreak, Burkina Faso, 2019. Emerg Infect Dis. (2024) 30(3):460–8. doi: 10.3201/eid3003.221760
15. World Health Organization. Disease outbreak news; Meningitis - Niger (2023). Available at: https://www.who.int/emergencies/disease-outbreak-news/item/2023-DON439 (Accessed August 10, 2024).
16. Woringer M, Martiny N, Porgho S, Bicaba BW, Bar-Hen A, Mueller JE. Atmospheric dust, early cases, and localized meningitis epidemics in the African meningitis belt: an analysis using high spatial resolution data. Environ Health Perspect. (2018) 126(9):097002. doi: 10.1289/EHP2752
17. Agier L, Martiny N, Thiongane O, Mueller JE, Paireau J, Watkins ER, et al. Towards understanding the epidemiology of Neisseria meningitidis in the African meningitis belt: a multi-disciplinary overview. Int J Infect Dis. (2017) 54:103–12. doi: 10.1016/j.ijid.2016.10.032
18. Diokhane AM, Jenkins GS, Manga N, Drame MS, Mbodji B. Linkages between observed, modeled saharan dust loading and meningitis in Senegal during 2012 and 2013. Int J Biometeorol. (2016) 60:557–75. doi: 10.1007/s00484-015-1051-5
19. Martiny N, Chiapello I. Assessments for the impact of mineral dust on the meningitis incidence in West Africa. Atmos Environ. (2013) 70:245–53. doi: 10.1016/j.atmosenv.2013.01.01
20. Agier L, Deroubaix A, Martiny N, Yaka P, Djibo A, Broutin H. Seasonality of meningitis in Africa and climate forcing: aerosols stand out. J R Soc Interface. (2013) 10(79):20120814. doi: 10.1098/rsif.2012.0814
21. Deroubaix A, Martiny N, Chiapello I, Marticorena B. Suitability of OMI aerosol index to reflect mineral dust surface conditions: preliminary application for studying the link with meningitis epidemics in the Sahel. Remote Sens Environ. (2013) 133:116–27. doi: 10.1016/j.rse.2013.02.009
22. Yaka P, Sultan B, Broutin H, Janicot S, Philippon S, Fourquet N. Relationships between climate and year-to-year variability in meningitis outbreaks: a case study in Burkina Faso and Niger. Int J Health Geogr. (2008) 7:1–13. doi: 10.1186/1476-072X-7-34
23. Thomson MC, Molesworth AM, Djingarey MH, Yameogo KR, Belanger F, Cuevas LE. Potential of environmental models to predict meningitis epidemics in Africa. Trop Med Int Health. (2006) 11(6):781–8. doi: 10.1111/j.1365-3156.2006.01630.x
24. Abdussalam AF, Monaghan AJ, Dukić VM, Hayden MH, Hopson TM, Leckebusch GC, et al. Climate influences on meningitis incidence in northwest Nigeria. Weather Clim Soc. (2014) 6(1):62–76. doi: 10.1175/WCAS-D-13-00004.1
25. Cristea D, Ciobanu E, Croitoru C. The use of the specialized questionnaire for assessing the risks of global warming and the development of infectious diseases in the Republic of Moldova. Bull Științific Supl Cadet Inova. (2024) 9:289–98.
26. Aghababaeian H, Ostadtaghizadeh A, Ardalan A, Asgary A, Akbary M, Yekaninejad MS, et al. Global health impacts of dust storms: a systematic review. Environ Health Insights. (2021) 15:11786302211018390. doi: 10.1177/11786302211018390
27. Lapeyssonnie L. La méningite cérébro-spinale en Afrique [Cerebrospinal meningitis in Africa]. Bull World Health Organ. (1963) 28 Suppl(Suppl):1–114.14259333
28. Engelstaedter S, Washington R. Atmospheric controls on the annual cycle of North African dust. J Geophys Res. (2007) 112(D3). doi: 10.1029/2006JD007195
29. Rodríguez-Fonseca B, Suárez-Moreno R, Ayarzagüena B, López-Parages J, Gómara I, Villamayor J, et al. A review of ENSO influence on the North Atlantic. A non-stationary signal. Atmosphere (Basel). (2016) 7(7):87. doi: 10.3390/atmos7070087
30. Gelaro R, McCarty W, Suárez MJ, Todling R, Molod A, Takacs L, et al. The modern-era retrospective analysis for research and applications, version 2 (MERRA-2). J Climate. (2017) 30(14):5419–54. doi: 10.1175/JCLI-D-16-0758.1
31. Inness A, Ades M, Agustí-Panareda A, Barré J, Benedictow A, Blechschmidt A-M, et al. The CAMS reanalysis of atmospheric composition. Atmos Chem Phys. (2019) 19:3515–56. doi: 10.5194/acp-19-3515-2019
32. Hersbach H, Bell B, Berrisford P, Biavati G, Horányi A, Muñoz Sabater J, et al. ERA5 hourly data on single levels from 1940 to present. Copernicus Climate Change Service (C3S) Climate Data Store (CDS) (2023). doi: 10.24381/cds.adbb2d47
33. Diop AB, Wade M, Sy A, Mbodji AK, Farota AK, Deme EH, et al. Atmospheric conditions for uplift and dust transport in the latitudinal 10° north–20° North band in Africa. Atmosphere. (2022) 13(7):1083. doi: 10.3390/atmos13071083
34. Von Storch H, Frankignoul C. Empirical modal decomposition in coastal oceanography. In: Brink KH, Robinson AR, editors. The Sea: Vol. 10, The Global Coastal Ocean. Processes and Methods. New York, NY: John Wiley & Sons Inc (1998). p. 419–55. doi: 10.13140/2.1.1760.0805
35. Deng L, Xu H, Liu P, Wu S, Shi Y, Lv Y, et al. Prolonged exposure to high humidity and high temperature environment can aggravate influenza virus infection through intestinal flora and Nod/RIP2/NF-κB signaling pathway. Vet Microbiol. (2020) 251:108896. doi: 10.1016/j.vetmic.2020.108896
36. Guarnieri G, Olivieri B, Senna G, Vianello A. Relative humidity and its impact on the immune system and infections. Int J Mol Sci. (2023) 24(11):9456. doi: 10.3390/ijms24119456
37. Yarber AY, Jenkins GS, Singh A, Diokhane A. Temporal relationships between Saharan dust proxies, climate, and meningitis in Senegal. GeoHealth. (2023) 7(2):e2021GH000574. doi: 10.1029/2021GH000574
38. Ayanlade A, Nwayor IJ, Sergi C, Ayanlade OS, Di Carlo P, Jeje OD, et al. Early warning climate indices for malaria and meningitis in tropical ecological zones. Sci Rep. (2020) 10(1):14303. doi: 10.1038/s41598-020-71094-8
39. Le T, Bae DH. Causal influences of El Niño–Southern oscillation on global dust activities. Atmos Chem Phys. (2022) 22(8):5253–63. doi: 10.5194/acp-22-5253-2022
40. Sabatier P, Nicolle M, Piot C, Colin C, Debret M, Swingedouw D, et al. Past African dust inputs in the western Mediterranean area are controlled by the complex interaction between the intertropical convergence Zone, the North Atlantic oscillation, and total solar irradiance. Clim Past. (2020) 16(1):283–98. doi: 10.5194/cp-16-283-2020
41. Ridley DA, Heald CL, Prospero JM. What controls the recent changes in African mineral dust aerosol across the Atlantic? Atmos Chem Phys. (2014) 14(11):5735–47. doi: 10.5194/acp-14-5735-2014
42. Li J, Garshick E, Huang S, Koutrakis P. Impacts of El Niño-Southern oscillation on surface dust levels across the world during 1982–2019. Sci Total Environ. (2021) 769:144566. doi: 10.1016/j.scitotenv.2020.144566
43. Jegede A, Conteh A, Sow K, Boyon M, Grant C, Schmidt-Sane M, et al. SSHAP West Africa Hub: Health Emergency Cycles and Social Context in West Africa. Brighton: Institute of Development Studies (2024). doi: 10.19088/SSHAP.2024.023
44. De Longueville F, Ozer P, Doumbia S, Henry S. Desert dust impacts on human health: an alarming worldwide reality and a need for studies in West Africa. Int J Biometeorol. (2013) 57:1–19. doi: 10.1007/s00484-012-0541-y
Keywords: meningitis, West Africa, vaccination, dust, temperature, humidity, intra-seasonal and inter-annual variability
Citation: Diouf D, Martín-Rey M, Rodríguez-Fonseca B, Diouf I, Dione C, Akinbobola A and Gaye AT (2025) Influence of environmental variability on meningitis in West African countries: pre- and post-vaccination. Front. Environ. Health 4:1531076. doi: 10.3389/fenvh.2025.1531076
Received: 19 November 2024; Accepted: 10 April 2025;
Published: 9 May 2025.
Edited by:
Pam Factor-Litvak, Columbia University, United StatesReviewed by:
Stavros Panagiotou, The University of Manchester, United KingdomAbigail Whitehouse, Queen Mary University of London, United Kingdom
Alvaro Soto, University of La Frontera, Chile
Copyright: © 2025 Diouf, Martín-Rey, Rodríguez-Fonseca, Diouf, Dione, Akinbobola and Gaye. This is an open-access article distributed under the terms of the Creative Commons Attribution License (CC BY). The use, distribution or reproduction in other forums is permitted, provided the original author(s) and the copyright owner(s) are credited and that the original publication in this journal is cited, in accordance with accepted academic practice. No use, distribution or reproduction is permitted which does not comply with these terms.
*Correspondence: Diarra Diouf, ZGlvdWYuZEBlZHUud2FzY2FsLm9yZw==