- 1Department of Physiology, Wakayama Medical University, Wakayama, Japan
- 2Lab of Histology and Cytology, Graduate School of Medicine, Hokkaido University, Sapporo, Japan
- 3Department of Genetic and Behavioral Neuroscience, Gunma University Graduate School of Medicine, Maebashi, Japan
Classically, the cerebellum has been thought to play a significant role in motor coordination. However, a growing body of evidence for novel neural connections between the cerebellum and various brain regions indicates that the cerebellum also contributes to other brain functions implicated in reward, language, and social behavior. Cerebellar Purkinje cells (PCs) make inhibitory GABAergic synapses with their target neurons: other PCs and Lugaro/globular cells via PC axon collaterals, and neurons in the deep cerebellar nuclei (DCN) via PC primary axons. PC-Lugaro/globular cell connections form a cerebellar cortical microcircuit, which is driven by serotonin and noradrenaline. PCs’ primary outputs control not only firing but also synaptic plasticity of DCN neurons following the integration of excitatory and inhibitory inputs in the cerebellar cortex. Thus, strong PC-mediated inhibition is involved in cerebellar functions as a key regulator of cerebellar neural networks. In this review, we focus on physiological characteristics of GABAergic transmission from PCs. First, we introduce monoaminergic modulation of GABAergic transmission at synapses of PC-Lugaro/globular cell as well as PC-large glutamatergic DCN neuron, and a Lugaro/globular cell-incorporated microcircuit. Second, we review the physiological roles of perineuronal nets (PNNs), which are organized components of the extracellular matrix and enwrap the cell bodies and proximal processes, in GABA release from PCs to large glutamatergic DCN neurons and in cerebellar motor learning. Recent evidence suggests that alterations in PNN density in the DCN can regulate cerebellar functions.
Introduction
Although the cerebellum is well known to play a crucial role in fine movement and motor activity (Ito, 1984), recent evidence suggest that the cerebellum is increasingly implicated in a variety of brain functions, including reward prediction (Wagner et al., 2017; Heffley et al., 2018; Carta et al., 2019; Kostadinov et al., 2019), motor planning (Gao et al., 2018; Chabrol et al., 2019; Wagner et al., 2019), and higher cognitive functions such as language and social behavior (Fiez and Petersen, 1998; Van Overwall et al., 2014; Schmahmann et al., 2019). Although the novel neural connections between the cerebellum and other brain areas and individual cellular elements are being unveiled rapidly (Judd et al., 2020; Kebschull et al., 2020; Kozareva et al., 2020), to achieve a comprehensive understanding of cerebellar functions, characterization of synaptic transmission and modulatory actions on neuronal and synaptic factors in the cerebellum is required. Inhibitory GABAergic transmission from Purkinje cells (PCs), the sole output neuron of the cerebellar cortex, has not been fully understood. This is because in vitro studies using cerebellar brain slices are mostly accompanied by PCs with damaged or cut axons, and it is difficult in identifying their target neurons among the numerous heterogeneous interneurons in the cerebellar cortex (Lainé and Axelrad, 2002; Crook et al., 2007; Simat et al., 2007; Schilling et al., 2008; Hirono, 2016; Prestori et al., 2019). In the historical view of the canonical neuronal network of the cerebellum, PCs project their primary axons to neurons in the deep cerebellar nuclei (DCN; Figure 1A): the fastigial (medial), interpositus, and dentate (lateral) (Ito et al., 1964; Obata et al., 1967; Chan-Palay, 1977; De Zeeuw and Berrebi, 1996; Mouginot and Gähwiler, 1995; Teune et al., 1998; Gauck and Jaeger, 2000; Najac and Raman, 2015). PC-mediated GABAergic inhibition regulates the rate and timing of DCN excitatory neuron output to cortical targets (Shin and De Schutter, 2006; Shin et al., 2007). DCN neurons produce the final output of the cerebellum by integrating the inhibitory inputs with excitatory inputs from mossy and climbing axon collaterals (Gauck and Jaeger, 2000; Rowland and Jaeger, 2005; Bengtsson et al., 2011; Steuber and Jaeger, 2013; Bengtsson and Jörntell, 2014). In the cerebellar cortex, the neuronal types targeted by PC axon collaterals have been controversial. PC axon collaterals have been suggested to form functional synaptic contacts with other PCs during early mouse development (Orduz and Llano, 2007; Watt et al., 2009). Recent electrophysiological studies demonstrated that even in adult mice, PC axon collaterals form synapses onto all PCs, Lugaro cells, globular cells (a subgroup of Lugaro cells) (Figures 1A,B), and one third of molecular layer interneurons (Hirono et al., 2012; Witter et al., 2016). Additionally, PCs form synaptic contacts with granule cells in a lobule-dependent manner (Guo C. et al., 2016). Therefore, it is important to characterize GABAergic transmission from PCs and their neuronal modulation.
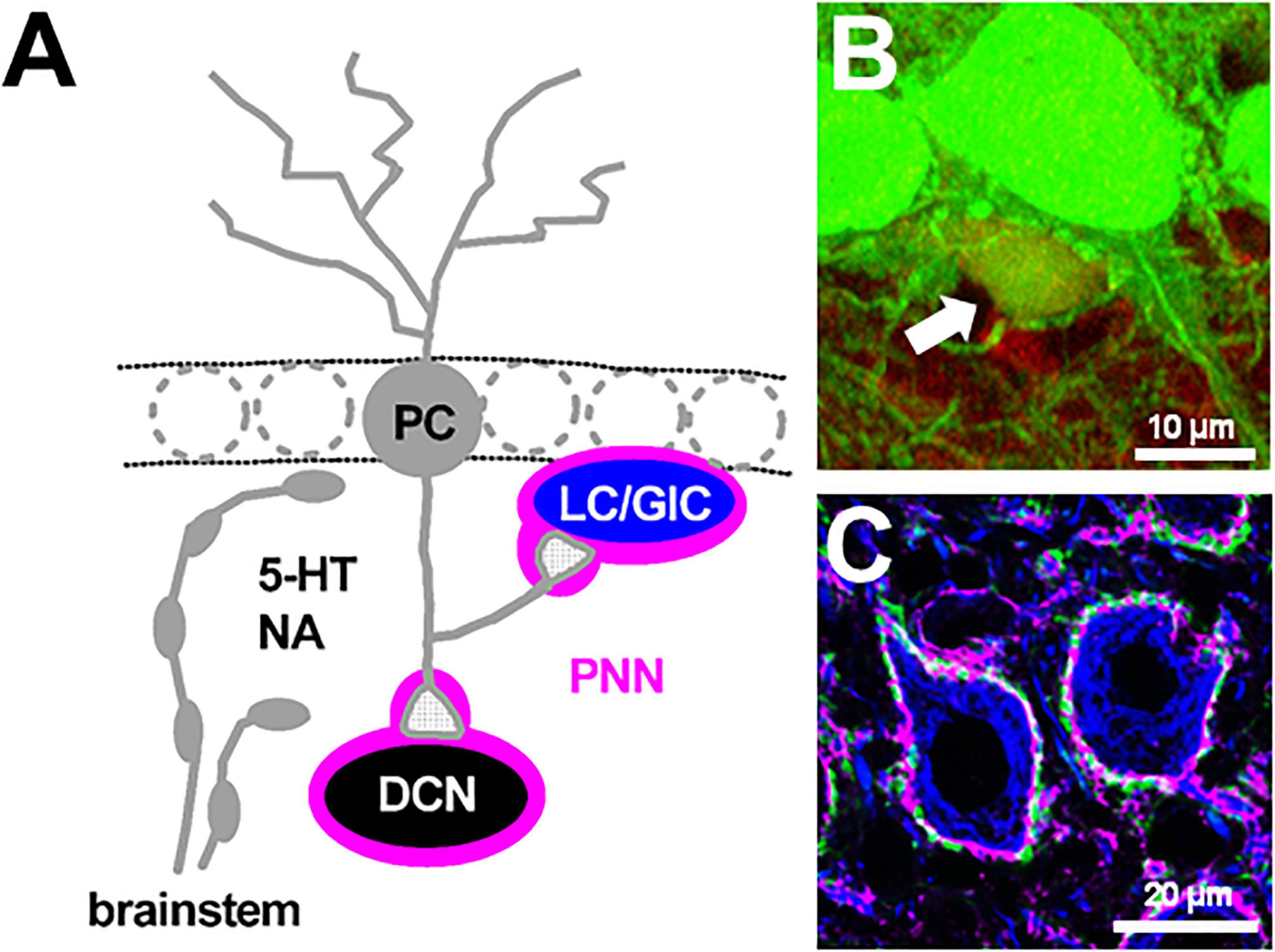
Figure 1. Perineuronal net-expressing cerebellar neurons targeted by Purkinje cells. (A) A Purkinje cell (PC) axon collateral makes GABAergic synapses with a Lugaro/globular cell (LC/GlC). A PC primary axon innervates a large glutamatergic neuron in the deep cerebellar nuclei (DCN). These PC target neurons are enwrapped by perineuronal nets (PNNs). 5-HT and NA are released from their beaded afferent fibers originated from the brainstem. (B) A GlC in the GAD67+/GFP mouse cerebellum shows immunoreactivity for anti-calretinin antibody (red), thus its cell body is show in yellow (indicated by an arrow). (C) Cell bodies of large glutamatergic DCN neurons are immunolabeled by SMI32 antibodies (blue) and surrounded by PNNs as shown by Wisteria floribunda agglutinin-staining (magenta) and PC axon terminals, which show immunoreactivity against calbindin (green). (B) is from Hirono et al. (2012). (C) is our unpublished data.
The cerebellum receives beaded fibers, including various amines and peptides, such as serotonin (5-HT) and noradrenaline (NA) (Ito, 2009; Figure 1A). Serotonergic beaded fibers, the third largest population of afferent fibers into the cerebellum only to mossy and climbing fibers, arise from the raphe nuclei and the gigantocellular reticular formation adjacent to the raphe nuclei, forming dense plexuses in the granule cell layer and the PC layer (Bishop and Ho, 1985; Kerr and Bishop, 1991; Longley et al., 2020). A dense plexus of serotonergic fibers extending from various brainstem nuclei is also present in the DCN, however, they are not collaterals of the fibers innervating into the cortex (Kitzman and Bishop, 1994), suggesting that the cerebellar cortex and DCN could be controlled independently by serotonergic signals. By contrast, noradrenergic neurons in the brainstem nucleus, locus coeruleus, project their beaded afferent fibers not only to the cerebellar cortex, which are densely expressed in the granule cell layer especially under the PC layer (Nelson et al., 1997), but also to the DCN (Hökfelt and Fuxe, 1969; Olson and Fuxe, 1971; Siggins et al., 1971; Nedelescu et al., 2017). Although cerebellar motor learning is affected by 5-HT (Miyashita and Watanabe, 1984; Trouillas et al., 1995; Oades et al., 2008; Frings et al., 2010) and NA (Bickford et al., 1992; Paredes et al., 2009; Wakita et al., 2017), there has been few evidence for their modulatory action on GABAergic transmission from PCs and intrinsic neuronal excitability of Lugaro/globular cells and large glutamatergic DCN neurons. Therefore, first, we introduce the characteristics of GABAergic transmission at synapses between PC axon collaterals and Lugaro/globular cells, and PC primary axons and large glutamatergic neurons in the DCN, focusing on 5-HT- and NA-mediated modulatory effects on GABAergic transmission.
In the mature central nervous system (CNS), perineuronal nets (PNNs), which are extracellular matrices enwrapping the cell bodies and proximal processes of neurons, form ladder-like structures and restrict the structural neuronal plasticity (Fawcett et al., 2019; Reichelt et al., 2019). It has been reported that PNNs not only control excitability of neurons and synaptic activity as a neuromodulator (Dityatev and Rusakov, 2011; Balmer, 2016), but also regulate action of other neuromodulators by changing their diffusion in the extracellular space (Sylantyev et al., 2008; Gundelfinger et al., 2010). Interestingly, PC target neurons, Lugaro/globular cells in the cerebellar cortex and large DCN neurons, express the formation of PNNs around their soma and proximal processes, where PC terminals innervated predominantly (Figure 1; De Zeeuw and Berrebi, 1996; Chan-Palay, 1997; Uusissari and Knöpfel, 2008). Thus, second, we especially focus on the physiological significance of PNNs, which are expressed in the DCN and enwrap synapses between PCs and the large DCN neurons, in dynamic regulation of GABAergic transmission as well as in cerebellar motor learning.
Lugaro/Globular Cells in the Cerebellar Cortex
Morphological Characteristics and Incorporated Microcircuit of Lugaro/Globular Cells
Inhibitory interneurons in the cerebellar cortex are more heterogeneous than knowledge in traditional categorization (Lainé and Axelrad, 2002; Crook et al., 2006; Simat et al., 2007; Schilling et al., 2008; Hirono, 2016; Prestori et al., 2019; Kozareva et al., 2020). Lugaro cells, which were reported for the first time in the cat cerebellum, have unique morphological characteristics and are located just below PCs in the granular cell layer, or within the PC layer (Lugaro, 1894). Their typical cell bodies are spindle-shaped and dendrites project on both sides of the cell bodies oriented in the parasagittal plane (Lugaro, 1894; Fox, 1959). Their axons project into the molecular layer and then travel long in the mediolateral axis alongside parallel fibers (Palay and Chan-Palay, 1974; Lainé and Axelrad, 1996). Lugaro cells are glycinergic/GABAergic interneurons and their number is very low, i.e., it is one fifteenth of that of PCs in the rat cerebellar cortex (Sahin and Hockfield, 1990; Dieudonné and Dumoulin, 2000). Lugaro cells are mainly divided into two subgroups on the basis of their morphology: fusiform Lugaro cells within the granule cell layer (Lugaro, 1894; Geurts et al., 2001; Crook et al., 2006) and globular cells, whose cell body is small and globular-shaped and located under the PC layer (Lainé and Axelrad, 2002; Simat et al., 2007; Schilling et al., 2008; Hirono et al., 2012). Immunohistochemical staining with antibodies against Kv4.3, mGluR1α, Rat303, SMI311, and chondroitin sulfate proteoglycans (CSPGs) has been performed for detailed anatomical studies of Lugaro/globular cells (Sahin and Hockfield, 1990; Geurts et al., 2001; Hsu et al., 2003; Víg et al., 2003; Crook et al., 2007). A novel reporter mouse line, which expresses Yellow Cameleon preferentially in Lugaro/globular cells, demonstrated their dendritic and axonal arborizations in relation to cerebellar compartments (Miyazaki et al., 2020). Furthermore, a recent transcriptomic study suggested cell type markers for putative Lugaro cells (Htr2a or Edil3) and for putative globular cells (Aldh1a3 or Slc6a5) (Kozareva et al., 2020).
In the parasagittal plane, axons of Lugaro/globular cells make synapses with basket/stellate cells (Lainé and Axelrad, 1998), and the transverse axons of Lugaro/globular cells innervate Golgi cells (Dieudonné and Dumoulin, 2000; Dumoulin et al., 2001; Hirono et al., 2012). Inhibitory synaptic signals from approximately 10 Lugaro/globular cells converge on one Golgi cell. One Lugaro/globular cell presumably forms divergent contacts with approximately 150 Golgi cells (Dieudonné and Dumoulin, 2000; Dieudonné, 2001; Dumoulin et al., 2001), suggesting that Lugaro/globular cells can synchronize activity among Golgi cells.
Lugaro/globular cells make a transverse microcircuit within the cerebellar cortex that contains not only PC-Lugaro/globular cell synapses but also other synapses of Lugaro/globular cells to basket/stellate cells, which in turn inhibit PCs, and to Golgi cell-granule cell contacts (Dieudonné, 2001; Simat et al., 2007; Hirono et al., 2012; Miyazaki et al., 2020; Figure 2A). The basal firing levels of PCs regulates the membrane potential of Lugaro/globular cells via a PC-Lugaro/globular cell feedback loop. The firing of Lugaro/globular cells, evoked by glutamatergic or monoaminergic synaptic inputs, thus, causes rectifying the PC firing (Strahlendorf et al., 1984; Hirono et al., 2012; Prestori et al., 2019). A recent morphological study also proposed that Lugaro cells can disinhibit cerebellar cortical activities in a compartment-dependent manner (Miyazaki et al., 2020). Therefore, the Lugaro/globular cell-incorporated microcircuit can synchronize the firing of PCs among different microzones and presumably play a significant role in cerebellar motor control leading to the optimization of multiple muscle activity in motor tasks.
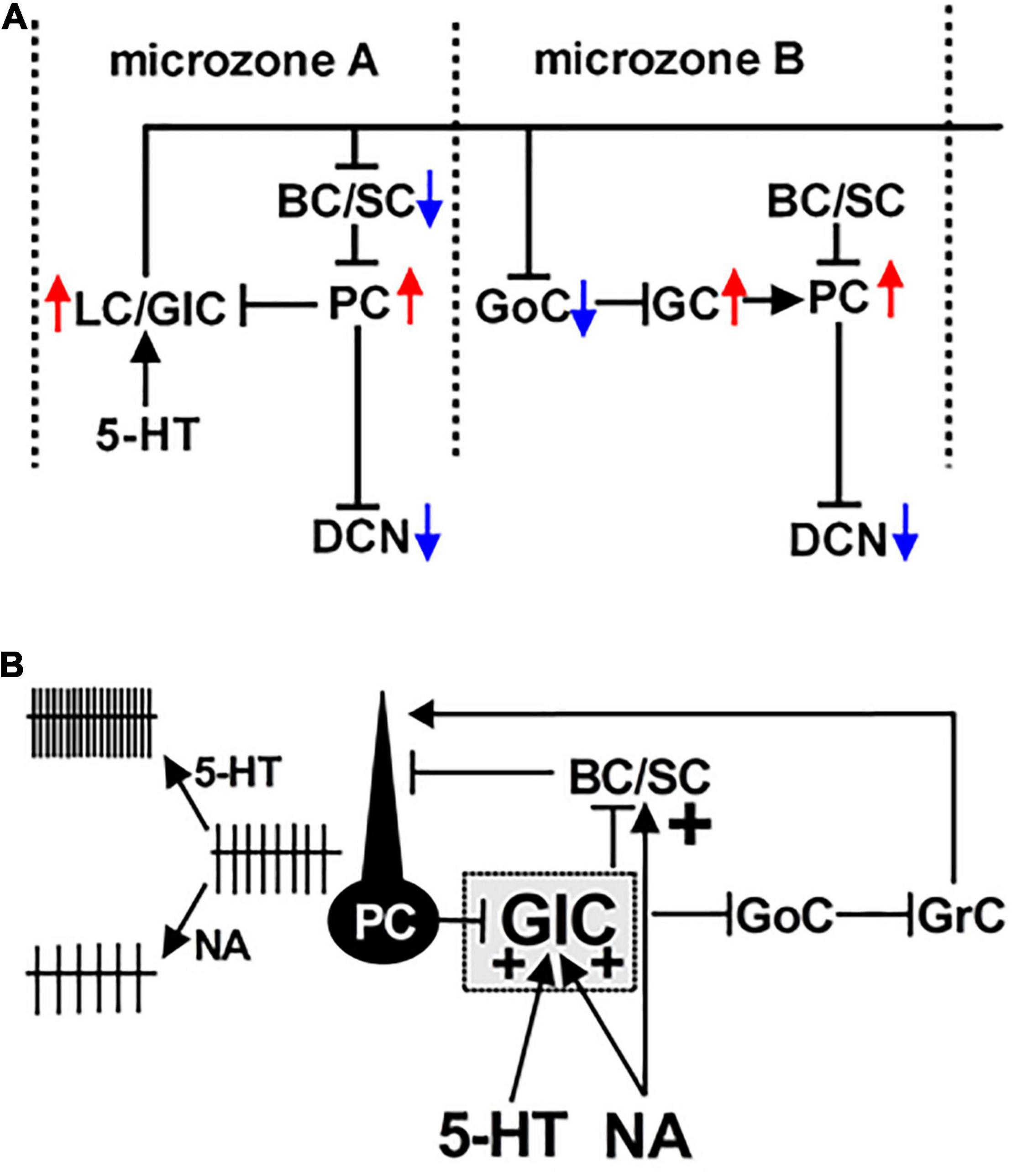
Figure 2. Schematic representation of a Lugaro/globular cell-incorporated microcircuit. (A) The functional connection between a Purkinje cell (PC) and a Lugaro/globular cell (LC/GlC) is represented in microzone A. The LC/GlC forms synaptic connections with a basket/stellate cell (BC/SC) in the sagittal plane and project its long and transversal axons, which intersect several microzones. Its axon collaterals form synaptic contacts with Golgi cells (GoCs) in microzone B. Thus, firing of the LC/GlC likely synchronize firing of PC groups between different microzones. Red and blue arrows denote excitation and hyperpolarization of neurons, respectively. (B) A microcircuit equipped with PC-GlC synapses suggesting serotonergic excitation of the GlC enhancing GABA/glycine release, which in turn facilitates the PC firing resulting from disinhibition at BC/SC-PC and GoC-granule cell (GrC) synapses. NA can excite the GlC, and enhance directly GABA release from a BC/SC to a PC, resulting in entirely the reduction of PC firing rate. (A) is modified from Dieudonné (2001); Simat et al. (2007) and Hirono et al. (2012). (B) is from Hirono et al. (2017).
Electrophysiological Characteristics of Lugaro/Globular Cells
Identification of Lugaro/globular cells under Nomarski optical microscopy is difficult because of their low number and the size of their cell body being similar to that of Golgi cells. Whole-cell patch-clamp recordings, however, were applied to them in acute slices of the rodent cerebellum. These electrophysiological studies revealed that Lugaro/globular cells are normally quiescent but get excited and exhibit robust firing following administration of 5-HT (Dieudonné and Dumoulin, 2000; Dumoulin et al., 2001; Dean et al., 2003; Hirono et al., 2012). This evidence suggests that these cells are the main targets of 5-HT released from serotonergic beaded afferent fibers in the cerebellar cortex. Lugaro/globular cells exhibit inhibitory synaptic currents at higher frequencies compared to Golgi cells (Dieudonné, 2001; Hirono et al., 2012, 2017), since their somata and proximal dendrites are enwrapped by calbindin-positive boutons, meaning they are synaptic inputs from PCs (Palay and Chan-Palay, 1974; Lainé and Axelrad, 2002; Crook et al., 2007; Simat et al., 2007). Electrophysiological and optogenetic approaches have demonstrated that PCs form direct and GABAergic monosynapses onto Lugaro/globular cells, meaning that outputs from several PCs inhibit them (Hirono et al., 2012; Witter et al., 2016). By contrast, fast glutamatergic excitatory synaptic inputs onto Lugaro/globular cells show depression by paired-pulse stimulation only at short interpulse intervals (< 100 ms), meaning that they receive mossy fiber inputs (Dieudonné, 2001; Hirono et al., 2012). Other synaptic contacts to Lugaro/globular cells, such as climbing fibers, granule cell fibers, Golgi cell axons, and Lugaro cell axons have been demonstrated morphologically (Miyazaki et al., 2020) but not yet physiologically.
Neuromodulation of GABAergic Transmission Between PCs and Lugaro/Globular Cells
5-HT released from serotonergic dense plexuses in the granule cell layer and the PC layer could control the excitability of Lugaro/globular cells by acting on their pre- and post-synaptic sites. The 5-HT-mediated membrane depolarization of Lugaro/globular cells is likely attributed to the activation of 5-HT2 or 5-HT6 receptors postsynaptically (Dieudonné, 2001). Our recent evidence indicates that globular cells are depolarized through the activation of 5-HT2A receptors, which facilitates phosphatidylinositol turnover, but not 5-HT6 receptors (Hirono et al., 2017). On the other hand, 5-HT and agonists for 5-HT1B receptors exert a reduction in GABA release from PCs to globular cells, indicating that 5-HT1B receptors are expressed on presynaptic terminals of PC axon collaterals (Hirono et al., 2017; Table 1). Because 5-HT1B receptors are normally coupled to the G protein (Gi/o), which negatively regulates adenylyl cyclase, the 5-HT-mediated inhibitory effects could be attributed to a decrease in phosphorylation levels of proteins associated with synaptic transmitter release. This indicates that 5-HT regulates the membrane excitability of PCs and Lugaro/globular cells via the differential modulatory effects on both GABAergic synaptic circuits and the membrane potential.
Noradrenergic beaded fibers are abundant just below the PC layer (Nelson et al., 1997). Thus, NA is likely released abundantly to cell bodies of Lugaro/globular cells to be more effective. Interestingly, globular, but not Lugaro cells, are excited to elicit firing during administration of NA (Hirono et al., 2012), suggesting that globular cells could express α1- and/or β2-adrenoceptors similar to molecular layer interneurons (Saitow et al., 2000; Hirono and Obata, 2006). NA also inhibits synaptic release of GABA from the PC axon collateral terminals onto globular cells via α2-adrenoceptor activation (Hirono et al., 2017; Table 1). Because the mouse cerebellar cortex expresses α2A and α2B, but not α2C-adrenoceptors (Talley et al., 1996; Hirono et al., 2008), α2A- and/or α2B-adrenoceptors located on presynaptic terminals of the PC axon collateral could mediate the noradrenergic attenuation of GABAergic transmission. Previous papers have reported that NA enhances GABAergic inhibition at basket/stellate cell-PC synapses through the activation of presynaptic α1- and β-adrenoceptors (Llano and Gerschenfeld, 1993; Cheun and Yeh, 1996; Kondo and Marty, 1998; Mitoma and Konishi, 1999; Saitow et al., 2000; Hirono and Obata, 2006). Thus, soon after PC firing facilitation mediated by the NA-evoked firing of globular cells, PC firing is thought to be reduced by NA-induced enhancement of GABAergic inhibition onto PCs (Figure 2B). Furthermore, in contrast to serotonergic afferent fibers in the cerebellum, which run along the mediolateral direction and influence many microzones (Hökfelt and Fuxe, 1969; Bishop and Ho, 1985; Longley et al., 2020), noradrenergic afferent fibers travel mainly along the rostrocaudal axis in a few microzones (Longley et al., 2020), suggesting that NA-induced firing of globular cells could synchronize PC firing in a few microzones transiently just after NA-evoked globular cell firing. In addition to the control of PC firing mediated by the NA-driven globular cell-incorporated microcircuit, NA modulates excitatory inputs onto PCs as follows: NA enhances parallel fibers and increases spontaneous spike firing of PCs under in vivo conditions (Guo A. et al., 2016). The activation of β2-adrenoceptors potentiates parallel fiber-PC synaptic transmission, while the activation of α1- and α2-adrenoceptors depresses the transmission (Lippiello et al., 2015). NA also reduces the glutamate release at climbing fiber-PC synapses via presynaptic α2-adrenoceptor activation (Carey and Regehr, 2009). Therefore, NA exerts more complicated effects in the cerebellar cortex through activating various types of adrenoceptors with the affinity for NA: α2- (high affinity), α1- (intermediate affinity) and β-adrenoceptors (low affinity) (Atzori et al., 2016). As the locus coeruleus project their afferent fibers to the cerebellar cortex (Nelson et al., 1997), firing modes of the locus coeruleus neurons can change the concentration of NA in the cerebellar cortex. In response to the different behavioral states such as sleep, quiet wake, active wake, and fight-or-flight, projection neurons in the locus coeruleus fire in distinct modes (Aston-Jones and Cohen, 2005; Atzori et al., 2016). Thus, at each behavioral state, the locus coeruleus-NA system play a role in fine tuning of PC firing to contribute to adequate cerebellar functions.
Large Glutamatergic Neurons in the DCN
GABAergic Transmission Between PCs and Large Glutamatergic DCN Neurons
The DCN, lateral, interpositus and medial, consist of six types of neurons: glutamatergic, GABAergic and two glycinergic projection neurons, and GABAergic or GABAergic/glycinergic and glutamatergic local interneurons (Chan-Palay, 1977; Uusisaari et al., 2007; Uusisaari and Knöpfel, 2011; Ankri et al., 2015). Large glutamatergic DCN neurons provide the sole output of the cerebellum to various brain areas, including the brainstem and thalamus (Kelly and Strick, 2003; Proville et al., 2014). Recent studies have demonstrated that large glutamatergic DCN neurons make monosynaptic transmission to the ventral tegmental area (VTA) (Carta et al., 2019), and that climbing fibers contribute to reward prediction but not error prediction for cerebellar motor learning (Holloway and Lerner, 2019). The VTA is known to project its dopaminergic axons to the frontal cortex which controls reward, motivation, and cognition, suggesting that neuronal activity of the DCN neurons can play a crucial role in higher cognitive functions, as well as in motor learning.
Direct GABAergic inhibition from PCs generates post-hyperpolarization rebound firing of large DCN neurons (Llinás and Mühlethaler, 1988; Aizenman and Linden, 1999; McKay et al., 2005; Baumel et al., 2009; Hoebeek et al., 2010; Bengtsson et al., 2011). Based on the reports that the firing rates of large DCN neurons are regulated in a linear manner by the inhibitory input rate (Gauck and Jaeger, 2000; Shin and De Schutter, 2006; Shin et al., 2007), the synchronicity and the extent of inhibitory PC input from the cerebellar cortex can play a role not only in the rate but also in the temporal precision patterns of firing of DCN neurons. Rebound firing responses depend on the duration and strength of membrane hyperpolarization and are significant for creating cerebellar timing signals (Aizenman and Linden, 1999; Koekkoek et al., 2003). Furthermore, it is involved in the formation of excitatory synaptic long-term potentiation (LTP) of transmission between mossy fibers onto large DCN neurons (Racine et al., 1986; Pugh and Raman, 2006, 2008; Person and Raman, 2010). The LTP has been thought to contribute to facilitating firing of large neurons in the DCN (Wu and Raman, 2017; Yarden-Rabinowitz and Yarom, 2017), and to be one of critical synaptic mechanisms underlying cerebellar motor learning, including ocular reflex adaptation (Miles and Lisberger, 1981; Kassardjian et al., 2005; Shutoh et al., 2006; Okamoto et al., 2011) and delay eyeblink conditioning (Krupa et al., 1993; Medina and Mauk, 1999; Attwell et al., 2002; Christian and Thompson, 2003; Kistler and De Zeeuw, 2003; Wetmore et al., 2008).
Monoaminergic Modulation of GABAergic Transmission Between PCs and Large Glutamatergic DCN Neurons
Compared to the large number of reports that neural activity in the cerebellar cortex is modulated by 5-HT, there are fewer reports studying serotonergic modulation of neuronal activity of the DCN (Gardette et al., 1987; Kitzman and Bishop, 1997; Di Mauro et al., 2003; Saitow et al., 2009; Murano et al., 2011). Exogenous administration of 5-HT facilitated spontaneous firing in most neurons in the DCN. A study on the interpositus nuclei demonstrated that 5-HT elicits a slow depolarization in their neurons (Saitow et al., 2009), whose underlying mechanism is that the activation of 5-HT5 receptors, which are normally coupled to the Gs proteins, increases intracellular cAMP levels and augments HCN channel activation, leading to the induction of inward currents in large DCN neurons. In the cerebellar fastigial nucleus of adult rats, 5-HT2A receptors are expressed and their activation elicits excitatory effect on neurons (Zhang et al., 2014; Table 1). These discrepancies are likely caused by differences in experimental aspects such as the contribution of synaptic transmission regulating neuronal excitability, the experimental sample (brain slices vs. in vivo), and the animal age (juvenile vs. adults). By contrast, 5-HT applied microiontophoretically suppressed firing of neurons of the medial nucleus and caused complicated effects (inhibitory, excitatory, and biphasic) on neurons not only of the interpositus but also of lateral nuclei (Di Mauro et al., 2003). As described below, 5-HT evokes slow excitatory inward currents postsynaptically, which facilitate discharge of DCN neurons, and subsequently attenuate the impact of rebound firing after repetitive inhibition by suppressing the GABA-mediated hyperpolarization. The impact of rebound depolarization is interrupted by 5-HT at relatively high concentrations because the 5-HT-mediated depolarization facilitates significantly the background spikes of DCN neurons. Thus, 5-HT may prevent the induction of LTP at synapses between mossy fibers and large DCN neurons. By contrast, 5-HT also modulates GABA release from presynaptic terminals on large DCN neurons (Saitow et al., 2009). GABAergic transmission is inhibited by the activation of presynaptic 5-HT1B receptors (Table 1).
It is possible that NA released from noradrenergic beaded fibers modulates activity of large DCN neurons. The β-adrenergic agonist isoproterenol can enhance the responses of DCN neurons to GABA applied microiontophoretically (Gould et al., 1997), meaning that β-adrenoceptors are postsynaptically expressed on large DCN neurons and modulate GABA inhibition. Di Mauro et al. (2013) tested the NA-mediated effects on inhibitory GABA responses in neurons of the various DCN (medial, posterior and anterior interpositus, and lateral), and showed that NA levels alter the responsiveness of DCN neurons to GABA. They indicated that NA modifies GABA responses through the activation of likely postsynaptic α2- and/or β-adrenoceptors (Table 1). In the inferior vestibular nucleus, NA directly regulates the excitability of neurons by the activation of α1-, α2- and β2-adrenoceptors (Peng et al., 2016). In any case, NA-mediated alteration of neuronal activity in each of these nuclei would have functional significances. Changes in NA concentration, as commonly observed in behavioral state, stress or aging, could influence neural activity of DCN neurons. Whereas spontaneous firing of PCs in each behavioral state is regulated by NA-mediated complex mechanisms as described previously, the NA-mediated effect on GABA release from presynaptic terminals of PC primary axons in the DCN has yet to be tested.
Physiological Roles of Perineuronal Nets in the DCN
General Information on Perineuronal Nets
A PNN is the fourth most important element for the tetrapartite synapse in addition to presynapses, postsynapses, and glial cells, and serves as a regulator of synaptic functions and plasticity (Dityatev and Rusakov, 2011; Chelini et al., 2018). The major components of PNNs are CSPGs, which have a core protein with long chondroitin sulfate chains, tenascin-R, link proteins, and hyaluronic acid, and are synthesized by both neurons and glial cells (Oohashi et al., 2015). To date, it has been reported that during brain development, PNNs contribute to the normal maturation of neuronal circuits, including fast-spiking parvalbumin-positive neurons (Cabungcal et al., 2013; Reichelt et al., 2019). PNNs morphologically restrict the production of new synapses and the pruning of old synapses, and contribute to the regulation of neuronal plasticity in various brain areas, such as the visual cortex and the amygdala (Pizzorusso et al., 2002; Gogolla et al., 2009; Carulli et al., 2010; Shen, 2018). To remove PNNs, enzymatic or genetic techniques have been widely adopted, and studies using these methods have demonstrated that PNN deletion enhances the formation of memories by facilitating plasticity and encoding new information that occurs by attenuating forgetting or learning information easily (Sorg et al., 2016; Fawcett et al., 2019; Reichelt et al., 2019). Moreover, PNNs have been focused on as the cause of the pathophysiology of brain disorders. Aberrant PNNs have been reported to be associated with neurodegenerative and neuropsychiatric disorders through abnormal neuroplasticity (Sorg et al., 2016; Suttkus et al., 2016; Wen et al., 2018; Fawcett et al., 2019; Reichelt et al., 2019). By contrast, it has not been elucidated whether PNNs can regulate cerebellar neuronal circuits dynamically and functionally, nor how PNNs contribute to the regulation of motor learning.
Expression of Perineuronal Nets in the Cerebellum
Perineuronal nets are detected in the brain using labeled Wisteria floribunda agglutinin (WFA), which is a lectin that recognizes the N-acetylgalactosamine segments of sugar chains in PNNs. In many brain regions, PNNs preferentially enwrap inhibitory parvalbumin-positive neurons, which are highly active and involved in critical periods of brain development, and play a crucial role in the regulation of neuronal functions and synaptic plasticity (Cabungcal et al., 2013; Fawcett et al., 2019; Reichelt et al., 2019). In the cerebellar cortex, Lugaro/globular cells, which receive strong GABAergic inhibition from PCs via their axon collaterals, are recognized by a monoclonal antibody against aggrecan, Cat-301 (Sahin and Hockfield, 1990; Zaremba et al., 1990; Crook et al., 2007), which means that they are surrounded by PNNs. On the other hand, both the cell bodies and the proximal dendrites of large and excitatory DCN neurons, which are primarily glutamatergic (Telgkamp and Raman, 2002; Uusisaari et al., 2007), are surrounded by PNNs (Figures 1C, 3A) in which aggrecan is the primary CSPG (Zaremba et al., 1990; Carulli et al., 2004, 2006, 2007; Zimmermann and Dours-Zimmermann, 2008; Foscarin et al., 2011; Bekku et al., 2012). Later, we will focus on the physiologically significant roles of PNNs surrounding large glutamatergic DCN neurons in dynamic regulation of GABAergic transmission as well as in cerebellar motor learning.
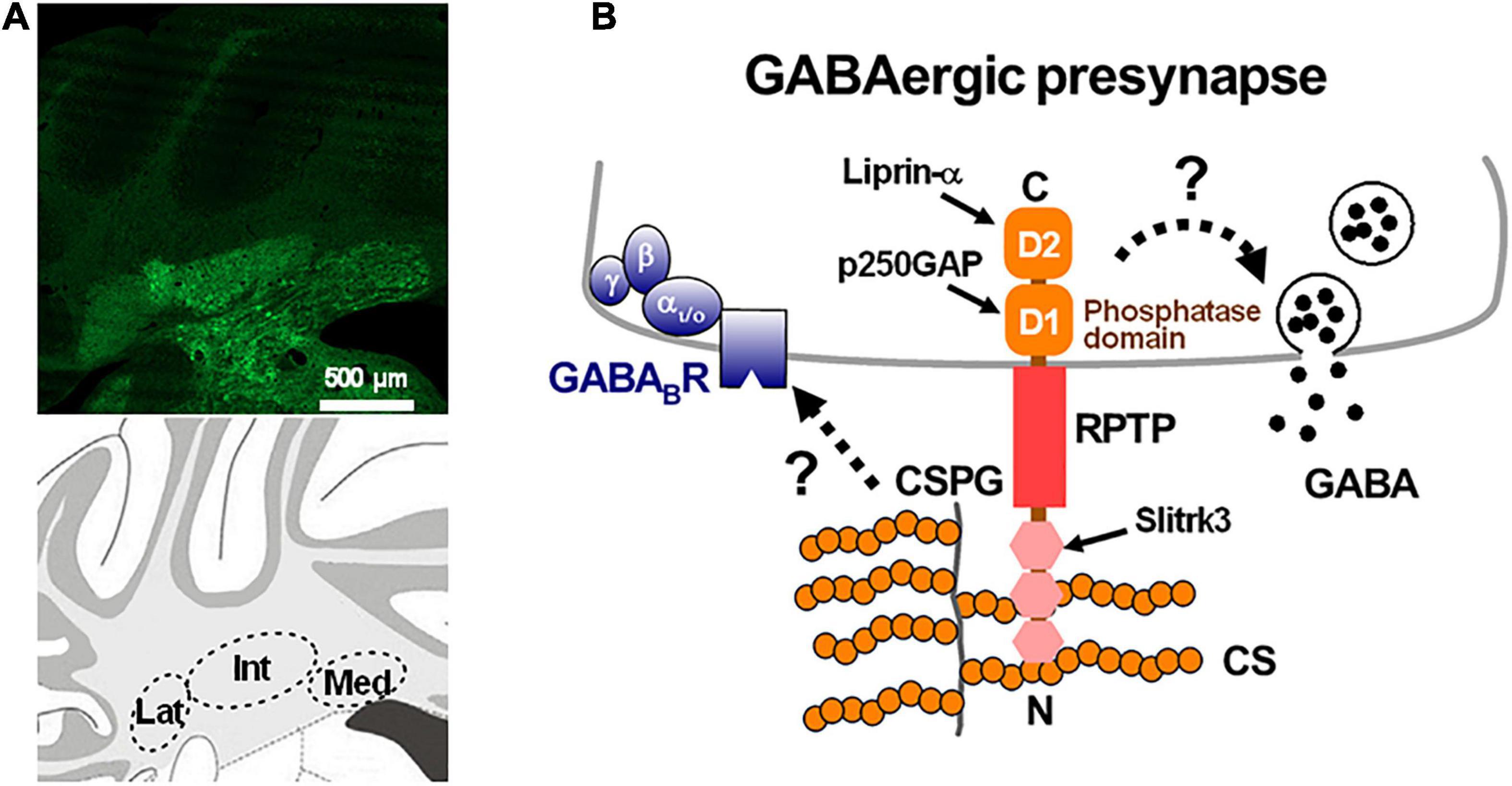
Figure 3. Perineuronal net-expressing in the deep cerebellar nuclei and schematic diagram of a GABAergic presynaptic terminal. (A) Perineuronal nets, which are labeled by Wisteria floribunda agglutinin (green), are expressed in the deep cerebellar nuclei: interpositus (Int), lateral (Lat) and medial (Med) nucleus of a mouse coronal section (upper panel). Schematic view of the three nuclei in the mouse cerebellar coronal section (lower panel). (B) A chondroitin sulfate proteoglycan (CSPG) binds to receptor-type protein tyrosine phosphatase (RPTP) via the chondroitin sulfate binding N-terminal of the RPTP. The intracellular domains of the RPTP may alter the release of GABA by directly controlling the release machinery or the intracellular Ca2+ concentration through changes of the phosphatase activity, or by interacting with Slitrk3, p250GAP, and liprin-α (Südhof, 2012). The CSPG may also regulate the expression of presynaptic GABAB receptors (Dzyubenko et al., 2020). (A) is our unpublished data.
Functional Roles of PNNs in GABAergic Transmission Between PC and Large Glutamatergic DCN Neuron
To examine the role of PNNs, techniques for the pharmacological or genetic removal of PNNs have been used. Chondroitinase ABC (ChABC) is a commonly used enzyme that degrades chondroitin sulfate glycosaminoglycans (GAGs). The DCN acutely treated with ChABC indicated a significant reduction in the intensity of WFA labeling by 30% around large DCN neurons and showed potentiates presynaptic release of GABA (Hirono et al., 2018; Table 2). Under the lower density of PNNs, a decrease in the amplitude of inhibitory postsynaptic currents (IPSCs) evoked by a high frequent repetitive stimulation is steeper, which is similar to that observed in the DCN of juvenile mice (Turecek et al., 2016; Saitow et al., 2018), reflecting a high tendency for neuronal plasticity and cerebellar motor learning compared to adults. On the other hand, stable overexpression of ChABC for a long time in the DCN through a lentiviral vector (LV) removed 90% PNNs from large DCN neurons (Carulli et al., 2020). The LV-ChABC mice showed that chronic ChABC treatment evoked the configuration of new GABAergic terminals and a decrease in expression of vesicular glutamate transporter (VGLUT) 1 in the DCN, leading to the attenuation of the spontaneous firing of large DCN neurons (Table 2).
Manipulation of specific genes related to PNNs can resolve PNN components. In mice lack of each PNN component such as HAPLN1, HAPLN4, and tenascin-C, the number of PC terminals on large neurons in the DCN was reported to be affected (Bekku et al., 2003, 2012; Foscarin et al., 2011; Stamenkovic et al., 2017; Edamatsu et al., 2018). By contrast, mouse cerebellar slices acutely treated with ChABC did not show anatomical changes of synapses on large DCN neurons; thus, acute enzymatic removal of PNNs did not change the size or number of PC axon terminals on large DCN neurons (Hirono et al., 2018). Thus, ChABC-mediated acute removal of PNNs by enhances the release probability of GABA rather than the new formation of presynaptic terminals of PC axons. Similarly, in the hippocampus, after treatment with ChABC for 24 h, CA1 pyramidal cells also showed an increase in the frequency of sIPSCs without changing the amplitude, and a theta burst stimulation-induced long-term plasticity in GABAergic transmission in the opposite way (Shi et al., 2019). Thus, it is concluded that PNNs can control presynaptic functions of PC axons in the DCN, and modulate synaptic plasticity and membrane excitation of large neurons in the DCN.
Potential Mechanisms Underlying PNN Regulation of Presynaptic GABA Release
There are likely four potential mechanisms underlying the augmentation of GABA release from terminals of PC axons with acute ChABC-mediated digestion of PNNs: first, releasing the limitation of extracellular Ca2+ availability, second, altering the conductance of presynaptic voltage-dependent Ca2+ channels, third, facilitating the release machinery of GABA, and fourth, augmenting the presynaptic basal intracellular Ca2+ concentrations in PC axon terminals. The most possible mechanism could be that negatively charged CSPGs buffer Ca2+ through interacting electrically (Crank, 1975) and confine the efficiency of extracellular Ca2+ around PC axon terminals (Hrabětová et al., 2009; Nicholson and Hrabětová, 2017). The extracellular matrix slows down Ca2+ diffusion around synapses, with this effect being attenuated by ChABC-mediated PNN deletion. However, the ChABC-mediated GABA release facilitation was not affected either by alterations in extracellular Ca2+ concentrations or by inhibition of voltage-dependent Ca2+ channels (Hirono et al., 2018). Thus, the latter two explanations described above could be plausible. PNN degradation could facilitate the release machinery by enhancement of its Ca2+ sensitivity and/or the intrinsic activity, or actuation of intracellular Ca2+ release in terminals of PC axons. CSPG removal could change the activity of type IIa receptor-type protein tyrosine phosphatases (RPTPs), leukocyte common antigen-related (LAR) proteins, PTPσ, and PTPδ, because they bind to CSPG-chondroitin sulfates (Shen et al., 2009; Fisher et al., 2011). CSPGs are known to control PTPσ and LAR receptors since ablation of the carbohydrate chains of CSPGs by ChABC causes regeneration of axons by the inactivation of PTPσ through its clustering (Coles et al., 2015; Lang et al., 2015). Cerebellar PCs are reported to express PTPσ and NgR3, which bind to CSPG-chondroitin sulfates (Funahashi et al., 2008; Brown et al., 2020). Therefore, ChABC-mediated chondroitin sulfate digestion, which can inactivate these receptors, likely change the balance between tyrosine phosphorylation and dephosphorylation, resulting in facilitating the release machinery for GABA release (Figure 3B). Recently, an alternative mechanism has been proposed that PNN depletion by ChABC administration reduces presynaptic GABAB receptors (Dzyubenko et al., 2020). Further investigations are needed to identify the exact molecular mechanism of the PNN-mediated modulation of presynaptic GABA release.
Large DCN neurons display post-inhibitory rebound firing after the relief of hyperpolarization induced by GABAergic transmission from PCs. Acute in vitro PNN depletion by ChABC treatment remarkably enhanced inhibitory GABAergic transmission between PCs and large DCN neurons, which induced an augmentation of hyperpolarization, thus, facilitating the rebound firing in the neurons, meaning improvements in cerebellar motor learning, as described in the next section (Hirono et al., 2018). In vivo electrophysiological recordings from neurons in the interpositus nuclei of LV-ChABC mice indicated that chronic PNN deletion markedly suppressed the baseline activity of the neurons (Carulli et al., 2020). They suggested that this firing reduction of DCN neurons could reduce the level of rebound firing in DCN neurons at the time of expression of conditioned responses (CRs) seen during delay eyeblink conditioning (ten Brinke et al., 2017). On the other hand, the LV-ChABC mice also demonstrated an increase in the density of GABAergic terminals around DCN neurons. Thus, the enhanced inhibition could facilitate the rebound firing as observed in the cerebellar slices acutely treated with ChABC.
PNNs in the Interpositus Nuclei Control Motor Learning of Delay Eyeblink Conditioning
Chondroitinase ABC was directly injected into various brain areas, and in vivo experiments with PNN deletion demonstrated the significance of PNNs in brain functions such as learning and memory (Pizzorusso et al., 2002; Corvetti and Rossi, 2005; Gogolla et al., 2009; de Vivo et al., 2013; Romberg et al., 2013; Banerjee et al., 2017). Inactivation of the DCN via lesions or pharmacological treatments has been reported to restrict the increases in CRs of trained mice (Yeo et al., 1985; Attwell et al., 2002; Ohyama et al., 2006; Boele et al., 2010; Sakamoto and Endo, 2010). Additionally, the activation of the interpositus nuclei is required for obtaining CRs during delay eyeblink conditioning (Heiney et al., 2014; ten Brinke et al., 2017). Therefore, the roles of PNNs expressed in the interpositus nuclei in delay eyeblink conditioning were tested pharmacologically and genetically by PNN digestion. Both the ChABC-injected and LV-ChABC mice exhibited significant enhancements in their CR rates, suggesting that PNN depletion in the interpositus nuclei facilitates CR acquisition (Hirono et al., 2018; Carulli et al., 2020). This facilitation of motor learning is similar to observations in other brain regions, where PNN loss leads to synaptic reorganization structurally and facilitates memory formation in adulthood (Corvetti and Rossi, 2005; Gogolla et al., 2009; Romberg et al., 2013; de Vivo et al., 2013), however, a novel role of PNNs in controlling GABA release from presynaptic terminals in the DCN is proposed, and a novel mechanistic insight for the functional synaptic plasticity regulated by PNNs has been demonstrated to influence behavioral flexibility in adulthood (Hirono et al., 2018). The developmental increase in PNN expression suppresses GABA release from PC axons to large DCN neurons and disturbs new formation of memories. This developmental change may also conserve cerebellar memories encoded before adulthood. Intriguingly, a reduction of PNNs in the DCN is observed during the acquisition and consolidation of eyeblink conditioning of adult mice (Carulli et al., 2020). These findings demonstrate that modulation of PNNs is significant for the dynamic regulation of GABAergic transmission in the DCN and for fine control of cerebellar motor learning.
Regulation of PNN Density by Voluntary Exercise
As described above, degradation of PNNs in the DCN enhances cerebellar motor learning. Additionally, a reduction in PNNs surrounding large DCN neurons occurs during the acquisition and consolidation of eyeblink conditioning (Carulli et al., 2020). These findings lead us to suggest that environmental stimuli could be useful in facilitating cerebellar functions including motor learning and cerebellum-mediated cognitive functions, because it was proposed that animals exposed to an enriched environment (EE) show decrease in PNNs in the CNS (Sale et al., 2007; Madinier et al., 2014), leading to facilitate neural plasticity. The EE has been suggested to enhance learning, facilitate recovery from brain lesions and brain disease, and postpone age-dependent cognitive decline (Nithianantharajah and Hannan, 2006; Pang and Hannan, 2013; Hirase and Shinohara, 2014). Indeed, mice exposed to an EE have also demonstrated a reduction in PNNs in the DCN (Foscarin et al., 2011; Stamenkovic et al., 2017). Foscarin et al. (2011) reported that the EE decreased the expression of mRNAs coding for key PNN molecules, and enhanced the activity of matrix degrading enzymes matrix metalloproteinases 2 (MMP-2) and MMP-9, via a dynamic interaction between PC axons and DCN neurons. It is possible that these mice could exhibit the CR at a significantly higher rate in delay eyeblink conditioning, suggesting an improvement in their motor performance (Figure 4). This expectation could be supported by the evidence that acquisition and expression of learning of delay eyeblink conditioning in mice are enhanced in a locomotor activity-dependent manner on a running wheel (Albergaria et al., 2018). They suggest that the enhanced eyeblink conditioning could be attributed to facilitation of the mossy fiber-granule cell connection by locomotor activity, however, there is another likely reason that the locomotor activity could reduce PNN expression in the DCN and improve cerebellar motor learning (Figure 4).
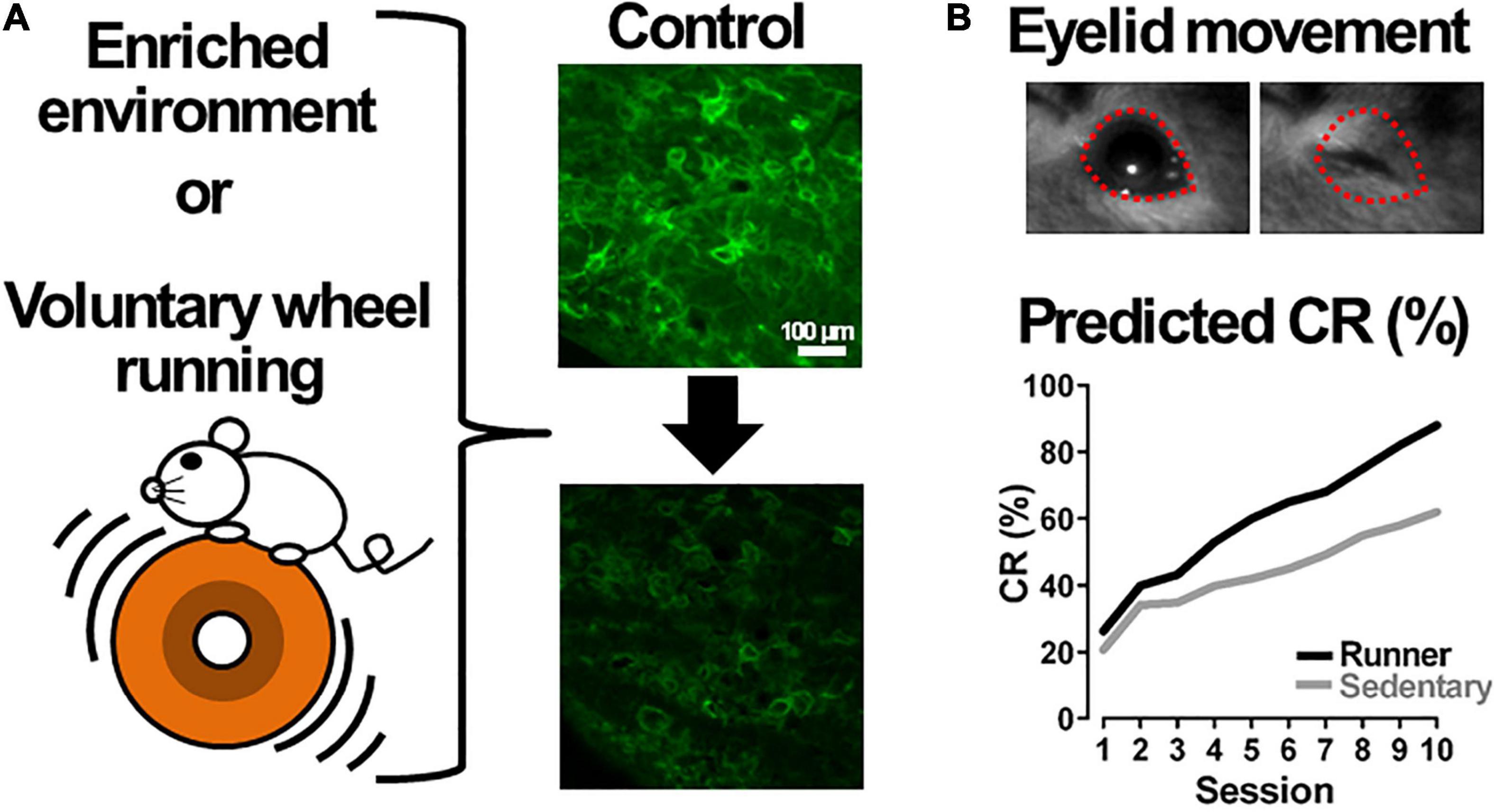
Figure 4. Voluntary exercise in an enriched environment or on a running wheel could facilitate cerebellar motor learning. (A) A mouse exercises in an enriched environment (EE) or on a running wheel. EE or voluntary wheel running likely reduces the density of perineuronal nets (right panels): the upper image shows large neurons surrounded by perineuronal nets labeled by Wisteria floribunda agglutinin (green) in the mouse deep cerebellar nucleus, and the lower shows a predicted image from a mouse kept in an EE or on a running wheel. The upper image is our unpublished data. (B) Voluntary exercise could improve conditioned responses (CRs) of cerebellar motor learning in delay eyeblink conditioning. The upper image is an example of eyelid movement during mouse delay eyeblink conditioning (Hirono et al., 2018). The lower graph shows predicted learning curves obtained from a mouse kept with (Runner) or without (Sedentary) a running wheel, respectively.
Increased motor activity such as voluntary wheel running can reduce the PNN expression. In rat experiments, free access to a running wheel caused PNN deletion in the hippocampus, but not in the DCN (Smith et al., 2015). Thus, additional studies in which mice are exposed to a running wheel are required, and a correlation between the running distances and the PNN expression levels in the DCN should be demonstrated. Considering that the voluntary wheel running evokes structural plasticity of the NA system: shortened inter-varicosity intervals in the cerebellum (Nedelescu et al., 2017), it is still possible that voluntary exercises in an EE and on a running wheel can improve not only cerebellar motor learning but also cognitive functions through several neuronal mechanisms, presumably including alternations of PNN density in the DCN.
Conclusion and Future Directions
A variety of neuronal modulators are involved in controlling cerebellar neural circuits. The characterization of the modulation of synaptic transmission and intrinsic neuronal excitation is significant for a precise comprehension of cerebellar motor learning and cognitive processing depending on the cerebellum. PCs project not only their primary axons to the DCN but also collaterals within the cerebellar cortex, especially around the PC layer to form negative feedback connections. Both the GABAergic transmission from PCs is important because the primary axons transmit signals, which are formed by PCs after integrating excitatory and inhibitory input signals, and the axon collaterals form Lugaro/globular cell-incorporated microcircuits. Thus, we focused on the characteristics of PC-mediated GABAergic transmission and the modulatory effects of monoamines and PNNs on synaptic transmission.
5-HT excites Lugaro/globular cells and large DCN neurons through pre- and post-synaptic mechanisms. The activation of presynaptic 5-HT1B suppresses the release of GABA from PC axon terminals, and the activation of 5-HT2A receptors (and 5-HT5 receptors for large DCN neurons) depolarizes the membrane potential of postsynaptic neurons. Thus, 5-HT released in the cerebellar cortex activates the Lugaro/globular cell-incorporated microcircuit, facilitating and synchronizing the activity of PC clusters, followed by a return to the basal activity of Lugaro/globular cells. 5-HT-evoked excitatory effects on large glutamatergic DCN neurons could be independent of the 5-HT-mediated activation of the microcircuit in the cerebellar cortex, because serotonergic fibers from various brainstem nuclei innervate separately into the cerebellar cortex and DCN (Kitzman and Bishop, 1994). In the cerebellar cortex, NA postsynaptically excites globular cells but not Lugaro cells presumably via the activation of α1- and/or β2-adrenoceptors. This postsynaptic excitation could be reinforced by a NA-mediated presynaptic effect, which is a NA-mediated inhibition of GABA release from PC axon collaterals through α2A and/or α2B–adrenoceptor activation. Thus, NA-mediated activation of a globular cell-incorporated microcircuit could also synchronize PC firing, followed by inhibition caused by NA-evoked excitation of basket/stellate cells. However, little is known regarding the direct effects of NA on the membrane potential of the large DCN neurons and GABA release from the terminals of PC primary axons. It has been reported that postsynaptic activation of α2- and β-adrenoceptors enhanced and decreased GABA responses, respectively. Further studies will be needed to determine NA-mediated direct effects on pre- and post-synaptic sites between PC-large DCN neuron synapses. Awake animals show sustained discharge of noradrenergic neurons in the locus coeruleus and of serotonergic neurons in the raphe nuclei. Animals in more active conditions such as in an aspect of active waking, alertness, or stress exhibit facilitation of firing of monoaminergic neurons. Previous studies demonstrated several relationships between the firing of these monoaminergic neurons and motor activity (Jacobs et al., 1991; Veasey et al., 1995, 1997; Mendlin et al., 1996). It will be of importance to demonstrate that in active animals, the excitatory effects of 5-HT and NA on Lugaro/globular cells can contribute to motor coordination of the cerebellum additively or synergistically.
In the CNS, PNNs, which predominantly wrap inhibitory parvalbumin-positive neurons, regulate synaptic plasticity and neuronal functions and are involved in controlling various brain functions such as learning and memory. PNN digestion facilitates GABAergic transmission at PC-large DCN neuron synapses. Acute pharmacological PNN removal functionally facilitates GABA release from presynaptic PC terminals, whereas chronic PNN removal increases the number of PC terminals on large DCN neurons. Mice that received ChABC pharmacologically or genetically in the interpositus nuclei showed CRs in delay eyeblink conditioning at a higher rate than that of control mice. This evidence suggests that PNN alteration makes memories flexible or consolidated, thereby affecting the flexibility of functions of the mature cerebellum. Whereas Lugaro/globular cells are known to be parvalbumin-negative, large glutamatergic DCN neurons surrounded by PNNs may be parvalbumin-positive neurons because it has been reported that parvalbumin-positive large bipolar neurons are present in the DCN (Bastianelli, 2003) and their synaptic dysfunction generates an action tremor (Zhou et al., 2020). As PNNs regulate spontaneous firing of large glutamatergic DCN neurons, which project their axons to various brain areas such as the brainstem, thalamus, and VTA, alternations in PNN density in the DCN can regulate not only cerebellar motor learning but also cognitive functions that depend on the cerebellum. Thus, future studies on mechanisms underlying the manipulation of specific components in the PNNs formed in the DCN will be important to unravel the physiology of cerebellar motor learning and cognitive processing.
Whereas PNNs in the mature CNS are known to restrict neuronal plasticity, a reduction in PNNs has been observed in multiple brain disorders, including cognitive dysfunction (Paylor et al., 2018), depression (Yu et al., 2020), Alzheimer’s (Bruckner et al., 1999; Baig et al., 2005; Morawski et al., 2010; Crapser et al., 2020), epilepsy (McRae and Porter, 2012; Pollock et al., 2014; Tewari et al., 2018; Chelyshev et al., 2020), schizophrenia (Pantazopoulos et al., 2010; Mauney et al., 2013; Enwright et al., 2016; Steullet et al., 2017), and bipolar disorders (Testa et al., 2019). Thus, to elucidate mechanisms underlying the regulation of PNN density related to neuronal plasticity in the CNS (Zaki and Cai, 2020) could be useful for finding out effective treatments for neurological disorders and psychiatric illnesses.
Author Contributions
MH, FK, and YY designed the project of this review, carried out the literature search and analysis, and wrote and edited the manuscript. All authors contributed to the article and approved the submitted version.
Funding
This work was supported by JSPS KAKENHI Grant Number JP19K06890 (MH).
Conflict of Interest
The authors declare that the research was conducted in the absence of any commercial or financial relationships that could be construed as a potential conflict of interest.
References
Aizenman, C. D., and Linden, D. J. (1999). Regulation of the rebound depolarization and spontaneous firing patterns of deep nuclear neurons in slices of rat cerebellum. J. Neurophysiol. 82, 1697–1709. doi: 10.1152/jn.1999.82.4.1697
Albergaria, C., Silva, N. T., Pritchett, D. L., and Carey, M. R. (2018). Locomotor activity modulates associative learning in mouse cerebellum. Nat. Neurosci. 21, 725–735. doi: 10.1038/s41593-018-0129-x
Ankri, L., Husson, Z., Pietrajtis, K., Proville, R., Léna, C., Yarom, Y., et al. (2015). A novel inhibitory nucleo-cortical circuit controls cerebellar Golgi cell activity. ELife 4:e06262.
Aston-Jones, G., and Cohen, J. D. (2005). An integrative theory of locus coeruleus-norepinephrine function: adaptive gain and optimal performance. Annu. Rev. Neurosci. 28, 403–450. doi: 10.1146/annurev.neuro.28.061604.135709
Attwell, P. J., Ivarsson, M., Millar, L., and Yeo, C. H. (2002). Cerebellar mechanisms in eyeblink conditioning. Ann. N.Y. Acad. Sci. 978, 79–92. doi: 10.1111/j.1749-6632.2002.tb07557.x
Atzori, M., Cuevas-Olguin, R., Esquivel-Rendon, E., Garcia-Oscos, F., Salgado-Delgado, R. C., Saderi, N., et al. (2016). Locus ceruleus norepinephrine release: a central regulator of CNS spatio-temporal activation? Front. Synaptic Neurosci. 8:25. doi: 10.3389/fnsyn.2016.00025
Baig, S., Wilcock, G. K., and Love, S. (2005). Loss of perineuronal net N-acetylgalactosamine in Alzheimer’s disease. Acta Neuropathol. 110, 393–401. doi: 10.1007/s00401-005-1060-2
Balmer, T. S. (2016). Perineuronal nets enhance the excitability of fast-spiking neurons. eNeuro 3, e112–e116.
Banerjee, S. B., Gutzeit, V. A., Baman, J., Aoued, H. S., Doshi, N. K., Liu, R., et al. (2017). Perineuronal nets in the adult sensory cortex are necessary for fear learning. Neuron 95, 169–179. doi: 10.1016/j.neuron.2017.06.007
Bastianelli, E. (2003). Distribution of calcium-binding proteins in the cerebellum. Cerebellum 2, 242–262. doi: 10.1080/14734220310022289
Baumel, Y., Jacobson, G. A., and Cohen, D. (2009). Implications of functional anatomy on information processing in the deep cerebella nuclei. Front. Cell. Neurosci. 3:14. doi: 10.3389/neuro.03.014.2009
Bekku, Y., Saito, M., Moser, M., Fuchigami, M., Maehara, A., Nakayama, M., et al. (2012). Bral2 is indispensable for the proper localization of brevican and the structural integrity of the perineuronal net in the brainstem and cerebellum. J. Comp. Neurol. 520, 1721–1736. doi: 10.1002/cne.23009
Bekku, Y., Su, W. D., Hirakawa, S., Fässler, R., Ohtsuka, A., Kang, J. S., et al. (2003). Molecular cloning of Bral2, a novel brain-specific link protein, and immunohistochemical colocalization with brevican in perineuronal nets. Mol. Cell. Neurosci. 24, 148–159. doi: 10.1016/s1044-7431(03)00133-7
Bengtsson, F., and Jörntell, H. (2014). Specific relationship between excitatory inputs and climbing fiber receptive fields in deep cerebellar nuclear neurons. PLoS One 9:e84616. doi: 10.1371/journal.pone.0084616
Bengtsson, F., Ekerot, C. F., and Jörntell, H. (2011). In vivo analysis of inhibitory synaptic inputs and rebounds in deep cerebellar nuclear neurons. PLoS One 6:e18822. doi: 10.1371/journal.pone.0018822
Bickford, P., Heron, C., Young, D. A., Gerhardt, G. A., and De La Garza, R. (1992). Impaired acquisition of novel locomotor tasks in aged and norepinephrine-depleted F344 rats. Neurobiol. Aging 13, 475–481. doi: 10.1016/0197-4580(92)90075-9
Bishop, G. A., and Ho, R. H. (1985). The distribution and origin of serotonin immunoreactivity in the rat cerebellum. Brain Res. 331, 195–207. doi: 10.1016/0006-8993(85)91545-8
Boele, H. J., Koekkoek, S. K. E., and De Zeeuw, C. J. (2010). Cerebellar and extracerebellar involvement in mouse eyeblink conditioning: the ACDC model. Front. Cell. Neurosci. 3:19. doi: 10.3389/neuro.03.019.2009
Brown, A. S., Meera, P., Quinones, G., Magri, J., Otis, T. S., Pulst, S. M., et al. (2020). Receptor protein tyrosine phosphatases control Purkinje neuron firing. Cell Cycle 19, 153–159. doi: 10.1080/15384101.2019.1695995
Bruckner, G., Hausen, D., Hartig, W., Drlicek, M., Arendt, T., and Brauer, K. (1999). Cortical areas abundant in extracellular matrix chondroitin sulphate proteoglycans are less affected by cytoskeletal changes in Alzheimer’s disease. Neuroscience 92, 791–805. doi: 10.1016/s0306-4522(99)00071-8
Cabungcal, J. H., Steullet, P., Morishita, H., Kraftsik, R., Cuenod, M., Hensch, T. K., et al. (2013). Perineuronal nets protect fast-spiking interneurons against oxidative stress. Proc. Natl. Acad. Sci. U.S.A. 110, 9130–9135. doi: 10.1073/pnas.1300454110
Carey, M. R., and Regehr, W. G. (2009). Noradrenergic control of associative synaptic plasticity by selective modulation of instructive signals. Neuron 62, 112–122. doi: 10.1016/j.neuron.2009.02.022
Carta, I., Chen, C. H., Schott, A. L., Dorizan, S., and Khodakhah, K. (2019). Cerebellar modulation of the reward circuitry and social behavior. Science 363:eaav0581. doi: 10.1126/science.aav0581
Carulli, D., Broersen, R., de Winter, F., Muir, E. M., Meškovic, M., de Waal, M., et al. (2020). Cerebellar plasticity and associative memories are controlled by perineuronal nets. Proc. Natl. Acad. Sci. U.S.A. 117, 6855–6865. doi: 10.1073/pnas.1916163117
Carulli, D., Buffo, A., and Strata, P. (2004). Reparative mechanisms in the cerebellar cortex. Prog. Neurobiol. 72, 373–398. doi: 10.1016/j.pneurobio.2004.03.007
Carulli, D., Pizzorusso, T., Kwok, J. C., Putignano, E., Poli, A., Forostyak, S., et al. (2010). Animals lacking link protein have attenuated perineuronal nets and persistent plasticity. Brain 133, 2331–2347. doi: 10.1093/brain/awq145
Carulli, D., Rhodes, K. E., and Fawcett, J. W. (2007). Upregulation of aggrecan, link protein 1, and hyaluronan synthases during formation of perineuronal nets in the rat cerebellum. J. Comp. Neurol. 501, 83–94. doi: 10.1002/cne.21231
Carulli, D., Rhodes, K. E., Brown, D. J., Bonnert, T. P., Pllack, S. J., Oliver, K., et al. (2006). Composition of perineuronal nets in the adult rat cerebellar and the cerebellar origin of their components. J. Comp. Neurol. 494, 559–577. doi: 10.1002/cne.20822
Chabrol, F. P., Blot, A., and Mrsic-Flogel, T. D. (2019). Cerebellar contribution to preparatory activity in motor neocortex. Neuron 103, 506–519.e504.
Chan-Palay, V. (1977). Cerebellar Dentate Nucleus. Organization, Cytology, and Transmitters. New York, NY: Springer.
Chelini, G., Pantazopoulos, H., Durning, P., and Berretta, S. (2018). The tetrapartite synapse: a key concept in the pathophysiology of schizophrenia. Eur. Psychiatry 50, 60–69. doi: 10.1016/j.eurpsy.2018.02.003
Chelyshev, Y. A., Kabdesh, I. M., and Mukhamedshina, Y. O. (2020). Extracellular matrix in neural plasticity and regeneration. Cell. Mol. Neurobiol. doi: 10.1007/s10571-020-00986-0
Cheun, J. E., and Yeh, H. H. (1996). Noradrenergic potentiation of cerebellar Purkinje cell responses to GABA: cyclic AMP as intracellular intermediary. Neuroscience 74, 835–844. doi: 10.1016/0306-4522(96)00130-3
Christian, K. M., and Thompson, R. F. (2003). Neural substrates of eyeblink conditioning: acquisition and retention. Learn. Mem. 10, 427–455. doi: 10.1101/lm.59603
Coles, C. H., Jones, E. Y., and Aricescu, A. R. (2015). Extracellular regulation of type Iia receptor protein tyrosine phosphatases: mechanistic insights from structural analysis. Semin. Cell Dev. Biol. 37, 98–107. doi: 10.1016/j.semcdb.2014.09.007
Corvetti, L., and Rossi, F. (2005). Degradation of chondroitin sulfate proteoglycans induces sprouting of intact Purkinje axons in the cerebellar of the adult rat. J. Neurosci. 25, 7150–7158. doi: 10.1523/jneurosci.0683-05.2005
Crapser, J. D., Spangenberg, E. E., Barahona, R. A., Arreola, M. A., Hohsfield, L. A., and Green, K. N. (2020). Microglia facilitate loss of perineuronal nets in the Alzheimer’s disease brain. EBioMedicine 58:102919. doi: 10.1016/j.ebiom.2020.102919
Crook, J. D., Hendrickson, A., Erickson, A., Possin, D., and Robinson, F. R. (2007). Purkinje cell axon collaterals terminate on Cat-301+ neurons in Macaca monkey cerebellum. Neuroscience 149, 834–844. doi: 10.1016/j.neuroscience.2007.08.030
Crook, J., Hendrickson, A., and Robinson, F. R. (2006). Co-localization of glycine and gaba immunoreactivity in interneurons in Macaca monkey cerebellar cortex. Neuroscience 141, 1951–1959. doi: 10.1016/j.neuroscience.2006.05.012
de Vivo, L., Landi, S., Panniello, M., Baroncelli, L., Chiezi, S., Mariotti, L., et al. (2013). Extracellular matrix inhibits structural and functional plasticity of dendritic spines in the adult visual cortex. Nat. Commun. 4:1484.
De Zeeuw, C. I., and Berrebi, A. S. (1996). Individual Purkinje cell axons terminate on both inhibitory and excitatory neurons in the cerebellar and vestibular nuclei. Ann. N.Y. Acad. Sci. 781, 607–610. doi: 10.1111/j.1749-6632.1996.tb15736.x
Dean, I., Robertson, S. J., and Edwards, F. A. (2003). Serotonin drives a novel GABAergic synaptic current recorded in rat cerebellar Purkinje cells: a Lugaro cell to Purkinje cell synapse. J. Neurosci. 23, 4457–4469. doi: 10.1523/jneurosci.23-11-04457.2003
Di Mauro, M., Fretto, G., Caldera, M., Li Volsi, G., Licata, F., Ciranna, L., et al. (2003). Noradrenaline and 5-hydroxytryptamine in cerebellar nuclei of the rat: functional effects on neuronal firing. Neurosci. Lett. 347, 101–105. doi: 10.1016/s0304-3940(03)00509-3
Di Mauro, M., Li Volsi, G., and Licata, F. (2013). Noradrenergic control of neuronal firing in cerebellar nuclei: modulation of GABA responses. Cerebellum 12, 350–361. doi: 10.1007/s12311-012-0422-2
Dieudonné, S. (2001). Serotonergic neuromodulation in the cerebellar cortex: cellular, synaptic, and molecular basis. Neuroscientist 7, 207–219. doi: 10.1177/107385840100700306
Dieudonné, S., and Dumoulin, A. (2000). Serotonin-driven long-range inhibitory connections in the cerebellar cortex. J. Neurosci. 20, 1837–1848. doi: 10.1523/jneurosci.20-05-01837.2000
Dityatev, A., and Rusakov, D. A. (2011). Molecular signals of plasticity at the tetrapartite synapse. Curr. Opin. Neurobiol. 21, 353–359. doi: 10.1016/j.conb.2010.12.006
Dumoulin, A., Triller, A., and Dieudonné, S. (2001). IPSC kinetics at identified GABAergic and mixed GABAergic and glycinergic synapses onto cerebellar Golgi cells. J. Neurosci. 21, 6045–6057. doi: 10.1523/jneurosci.21-16-06045.2001
Dzyubenko, E., Fleischer, M., Manrique-Castano, D., Borbor, M., Kleinschnitz, C., Faissner, A., et al. (2020). Extracellular matrix supports excitation-inhibition balance in neuronal networks by stabilizing inhibitory synapses. bioRxiv [preprint] doi: 10.1101/2020.07.13.200113
Edamatsu, M., Miyano, R., Fujikawa, A., Fujii, F., Hori, T., Sakaba, T., et al. (2018). Hapln4/Bral2 is a selective regulator for formation and transmission of GABAergic synapses between Purkinje and deep cerebellar nuclei neurons. J. Neurochem. 147, 748–763. doi: 10.1111/jnc.14571
Enwright, J. F., Sanapala, S., Foglio, A., Berry, R., Fish, K. N., and Lewis, D. A. (2016). Reduced labeling of parvalbumin neurons and perineuronal nets in the dorsolateral prefrontal cortex of subjects with schizophrenia. Neuropsychopharmacology 41, 2206–2214. doi: 10.1038/npp.2016.24
Fawcett, J. W., Oohashi, T., and Pizzorusso, T. (2019). The roles of perineuronal nets and the perinodal extracellular matrix in neuronal function. Nat. Rev. Neurosci. 20, 451–465. doi: 10.1038/s41583-019-0196-3
Fiez, J. A., and Petersen, S. E. (1998). Neuroimagin studies of word reading. Proc. Natl. Acad. Sci. U.S.A. 95, 914–921.
Fisher, D., Xing, B., Dill, J., Li, H., Hoang, H. H., Zhao, Z., et al. (2011). Leukocyte common antigen-related phosphatase is a functional receptor for chondroitin sulfate proteoglycan axon growth inhibitors. J. Neurosci. 31, 14051–14066. doi: 10.1523/jneurosci.1737-11.2011
Foscarin, S., Ponchione, D., Pajaj, E., Leto, K., Gawlak, M., Wilczynski, G. M., et al. (2011). Experience-dependen plasticity and modulation of growth regulatory molecules at central synapses. PLoS One 6:e1666. doi: 10.1371/journal.pone.0016666
Fox, C. A. (1959). The intermediate cells of Lugaro in the cerebellar cortex of the monkey. J. Comp. Neurol. 112, 39–53. doi: 10.1002/cne.901120108
Frings, M., Gaertner, K., Buderath, P., Gerwig, M., Christiansen, H., Schoch, B., et al. (2010). Timing of conditioned eyeblink responses is impaired in children with attention-deficit/hyperactivity disorder. Exp. Brain Res. 201, 167–176. doi: 10.1007/s00221-009-2020-1
Funahashi, S., Hasegawa, T., Nagano, A., and Sato, K. (2008). Differential expression patterns of messenger RNAs encoding nogo receptors and their ligands in the rat central nervous system. J. Comp. Neurol. 506, 141–160. doi: 10.1002/cne.21541
Gao, Z., Davis, C., Thomas, A. M., Economo, N. M., Abrego, A. M., Svoboda, K., et al. (2018). A cortico-cerebellar loop for motor planning. Nature 563, 113–116. doi: 10.1038/s41586-018-0633-x
Gardette, R. G., Krupa, F., and Crepel, K. F. (1987). Differential effects of serotonin on the spontaneous discharge and on the excitatory amino acid-induced responses of deep cerebellar nuclei neurons in rat cerebellar slices. Neuroscience 23, 491–500. doi: 10.1016/0306-4522(87)90072-8
Gauck, V., and Jaeger, D. (2000). The control of rate and timing of spikes in the deep cerebellar nuclei by inhibition. J. Neurosci. 20, 3006–3016. doi: 10.1523/jneurosci.20-08-03006.2000
Geurts, F. J., Timmermans, J. P., Shigemoto, R., and De Schutter, E. (2001). Morphological and neurochemical differentiation of large granular layer interneurons in the adult rat cerebellum. Neuroscience 104, 499–512. doi: 10.1016/s0306-4522(01)00058-6
Gogolla, N., Caroni, P., Lüthi, A., and Herry, C. (2009). Perineuronal nets protect fear memories from erasure. Science 325, 1258–1261. doi: 10.1126/science.1174146
Gould, T. J., Adams, C. E., and Bickford, P. C. (1997). β-adrenergic modulation of GABAergic inhibition in the deep cerebellar nuclei of F344 rats. Neuropharmacology 36, 75–81. doi: 10.1016/s0028-3908(96)00148-7
Gundelfinger, E. D., Frischknecht, R., Choquet, D., and Heine, M. (2010). Converting juvenile into adult plasticity: a role for the brain’s extracellular matrix. Eur. J. Neurosci. 31, 2156–2165. doi: 10.1111/j.1460-9568.2010.07253.x
Guo, A., Feng, J. Y., Li, J., Ding, N., Li, Y. J., Qiu, D. L., et al. (2016). Effects of norepinephrine on spontaneous firing activity of cerebellar Purkinje cells in vivo in mice. Neurosci. Lett. 629, 262–266. doi: 10.1016/j.neulet.2016.06.058
Guo, C., Witter, L., Rudolph, S., Elliott, H. L., Ennis, K. A., and Regehr, W. G. (2016). Purkinje cells directly inhibit granule cells in specialized regions of the cerebellar cortex. Neuron 91, 1330–1341. doi: 10.1016/j.neuron.2016.08.011
Heffley, W., Song, E. Y., Xu, Z., Taylor, B. N., Hughes, M. A., McKinney, A., et al. (2018). Coordinated cerebellar climbing fiber activity signals learned sensorimotor predictions. Nat. Neurosci. 21, 1431–1441. doi: 10.1038/s41593-018-0228-8
Heiney, S. A., Wohl, M. P., Chettih, S. N., Ruffolo, L. I., and Medina, J. F. (2014). Cerebellar-dependent expression of motor leaning during eyeblink conditioning in head-fixed mice. J. Neurosci. 34, 14845–14853. doi: 10.1523/jneurosci.2820-14.2014
Hirase, H., and Shinohara, Y. (2014). Transformation of cortical and hippoxampal neural circuit by environment enrichment. Neuroscience 280, 282–298. doi: 10.1016/j.neuroscience.2014.09.031
Hirono, M. (2016). “Lugaro cells,” in Essentials of Cerebellum and Cerebellar Disorders, eds D. Gruol, N. Koibuchi, M. Manto, M. Molinari, J. D. Schmahmann, and Y. Shen (Cham: Springer), 207–211. doi: 10.1007/978-3-319-24551-5_25
Hirono, M., and Obata, K. (2006). α-Adrenoceptive dual modulation of inhibitory GABAergic inputs to Purkinje cells in the mouse cerebellum. J. Neurophysiol. 95, 700–708. doi: 10.1152/jn.00711.2005
Hirono, M., Matsunaga, W., Chimura, T., and Obata, K. (2008). Developmental enhancement of α2-adrenoceptor-mediated suppression of inhibitory synaptic transmission onto mouse cerebellar Purkinje cells. Neuroscience 156, 143–154. doi: 10.1016/j.neuroscience.2008.07.018
Hirono, M., Nagao, S., Yanagawa, Y., and Konishi, S. (2017). Monoaminergic modulation of GABAergic transmission onto cerebellar globular cells. Neuropharmacology 118, 79–89. doi: 10.1016/j.neuropharm.2017.03.011
Hirono, M., Saitow, F., Kudo, M., Suzuki, H., Yanagawa, Y., Yamada, M., et al. (2012). Cerebellar globular cells receive monoaminergic excitation and monosynaptic inhibition from Purkinje cells. PLoS One 7:e29663. doi: 10.1371/journal.pone.0029663
Hirono, M., Watanabe, S., Karube, F., Fujiyama, F., Kawahara, S., Nagao, S., et al. (2018). Perineuronal nets in the deep cerebellar nuclei regulate GABAergic transmission and delay eyeblink conditioning. J Neurosci. 38, 6130–6144. doi: 10.1523/jneurosci.3238-17.2018
Hoebeek, F. E., Witter, L., Ruigrok, T. J. H., and De Zeeuw, C. I. (2010). Differential olovo-cerebellar cortical control of rebound activity in the cerebellar nuclei. Proc. Natl. Acad. Sci. U.S.A. 107, 8410–8415. doi: 10.1073/pnas.0907118107
Hökfelt, T., and Fuxe, K. (1969). Cerebellar monoamine nerve terminals, a new type of afferent fibers to the cortex cerebelli. Exp. Brain Res. 9, 63–72.
Hrabětová, S., Masri, D., Tao, L., Xiao, F., and Nicholson, C. (2009). Calcium diffusion enhanced after cleavage of negatively charged components of brain extracellular matrix by chondroitinase ABC. J. Physiol. 587, 4029–4049. doi: 10.1113/jphysiol.2009.170092
Hsu, Y. H., Huang, H. Y., and Tsaur, M. L. (2003). Contrasting expression of Kv4.3 an A-type K+ channel, in migrating Purkinje cells and other post-migratory cerebellar neurons. Eur. J. Neurosci. 18, 601–612. doi: 10.1046/j.1460-9568.2003.02786.x
Ito, M. (2009). Functional roles of neuropeptides in cerebellar circuits. Neuroscience 162, 666–672. doi: 10.1016/j.neuroscience.2009.01.019
Ito, M., Yoshida, M., and Obata, K. (1964). Monosynaptic inhibition of the intracerebellar nuclei induced from the cerebellar cortex. Experientia 20, 575–576. doi: 10.1007/bf02150304
Jacobs, B. L., Abercrombie, E. D., Fornal, C. A., Levine, E. S., Morilak, D. A., and Stafford, I. L. (1991). Single-unit and physiological analyses of brain norepinephrine function in behaving animals. Prog. Brain Res. 88, 159–165. doi: 10.1016/s0079-6123(08)63805-4
Judd, E. N., Lewis, S. M., Heck, D. G., and Person, A. L. (2020). Widespread inhibitory projections from the interposed cerebellar nucleus. BioRxiv [preprint] doi: 10.1101/2020.12.31.425011
Kassardjian, C. D., Tan, Y. F., Chung, J.-Y., Heskin, R., Peterson, M. J., and Broussard, D. M. (2005). The site of a memory shifts with consolidation. J. Neurosci. 25, 7979–7985. doi: 10.1523/jneurosci.2215-05.2005
Kebschull, J. M., Richman, E. B., Ringach, N., Friedmann, D., Albarran, E., Kolluru, S. S., et al. (2020). Cerebellar nuclei evolved by repeatedly duplicating a conserved cell-type set. Science 370:eabd5059.
Kelly, R. M., and Strick, P. L. (2003). Cerebellar loops with motor cortex and prefrontal cortex of a nonhuman primate. J. Neurosci. 23, 8432–8444. doi: 10.1523/jneurosci.23-23-08432.2003
Kerr, C. W., and Bishop, G. A. (1991). Topographical organization in the origin of serotoninergic projections to different regions of the cat cerebellar cortex. J. Comp. Neurol. 304, 502–515. doi: 10.1002/cne.903040313
Kistler, W. M., and De Zeeuw, C. I. (2003). Time windows and reverberating loops: a reverse-engineering approach to cerebellar function. Cerebellum 2, 44–54. doi: 10.1080/14734220309426
Kitzman, P. H., and Bishop, G. A. (1994). The origin of serotoninergic afferents to the cat’s cerebellar nuclei. J. Comp. Neurol. 340, 541–550. doi: 10.1002/cne.903400407
Kitzman, P. H., and Bishop, G. A. (1997). The physiological effects of serotonin on spontaneous and amino acid-induced activation of cerebellar nuclear cells: an in vivo study in the cat. Prog. Brain Res. 114, 209–223. doi: 10.1016/s0079-6123(08)63366-x
Koekkoek, S. K. E., Hulscher, H. C., Dortland, B. R., Hensbroek, R. A., Elgersma, Y., Ruigrok, T. J. H., et al. (2003). Cerebellar LTD and learning-dependent timing of conditioned eyelid responses. Science 301, 1736–1739. doi: 10.1126/science.1088383
Kondo, S., and Marty, A. (1998). Differential effects of noradrenaline on evoked, spontaneous and miniature IPSCs in rat cerebellar stellate cells. J. Physiol. 509, 233–243. doi: 10.1111/j.1469-7793.1998.233bo.x
Kostadinov, D., Beau, M., Pozo, M. B., and Hausser, M. (2019). Predictive and reactive reward signals conveyed by climbing fiber inputs to cerebellar Purkinje cells. Nat. Neurosci. 22, 950–962. doi: 10.1038/s41593-019-0381-8
Kozareva, V., Martin, C., Osorno, T., Rudolph, S., Guo, C., Vanderburg, C., et al. (2020). A transcriptomic atlas of the mouse cerebellum reveals regional specializations and novel cell types. bioRxiv [preprint] doi: 10.1101/2020.03.04.976407
Krupa, D. J., Thompson, J. K., and Thompson, R. F. (1993). Localization of a memory trace in the mammalian brain. Science 260, 989–991. doi: 10.1126/science.8493536
Lainé, J., and Axelrad, H. (1996). Morphology of the Golgi-impregnated lugaro cell in the rat cerebellar cortex: a reappraisal with a description of its axon. J. Comp. Neurol. 375, 618–640. doi: 10.1002/(sici)1096-9861(19961125)375:4<618::aid-cne5>3.0.co;2-4
Lainé, J., and Axelrad, H. (1998). Lugaro cells target basket and stellate cells in the cerebellar cortex. Neuroreport 9, 2399–2403. doi: 10.1097/00001756-199807130-00045
Lainé, J., and Axelrad, H. (2002). Extending the cerebellar Lugaro cell class. Neuroscience 115, 363–374. doi: 10.1016/s0306-4522(02)00421-9
Lang, B. T., Cregg, J. M., DePaul, M. A., Tran, A. P., Xu, K., Dyck, S. M., et al. (2015). Modulation of the proteoglycan receptor PTP promotes recovery after spinal cord injury. Nature 518, 404–408. doi: 10.1038/nature13974
Lippiello, P., Hoxha, E., Volpicelli, F., Duca, G. L., Tempia, F., and Miniaci, M. C. (2015). Noradrenergic modulation of the parallel fiber-Purkinje cell synapse in mouse cerebellum. Neuropharmacology 89, 33–42. doi: 10.1016/j.neuropharm.2014.08.016
Llano, I., and Gerschenfeld, H. M. (1993). β-Adrenergic enhancement of inhibitory synaptic activity in rat cerebellar stellate and Purkinje cells. J. Physiol. 468, 201–224. doi: 10.1113/jphysiol.1993.sp019767
Llinás, R., and Mühlethaler, M. (1988). Electrophysiology of guinea-pig cerebellar nuclear cells in the in vivo brain stem-cerebellar preparation. J. Physiol. 404, 241–258. doi: 10.1113/jphysiol.1988.sp017288
Longley, M., Ballard, J., Andres-Alonso, M., Varatharajah, R. C., Cuthbert, H., and Yeo, C. H. (2020). A patterned architecture of monoaminergic afferents in the cerebellar cortex: noradrenergic and serotonergic fibre distributions within lobules and parasagittal zones. Neuroscience 462, 106–121. doi: 10.1016/j.neuroscience.2020.09.001
Lugaro, E. (1894). Sulle connessioni tra gli elemente nervosa della corteccia cerebellare con considerazioni generali sulsignificato fisiologico dei rapporti tra gli elementi nervosi. Riv. Sper. Freniatr. 20, 297–331.
Madinier, A., Quattromani, M. J., Sjolund, C., Ruscher, K., and Wieloch, T. (2014). Enriched housing enhances recovery of limb placement ability and reduces aggrecan-containing perineuronal nets in the rat somatosensory cortex after experimental stroke. PLoS One 9:e93121. doi: 10.1371/journal.pone.0093121
Mauney, S. A., Athanas, K. M., Pantazopoulos, H., Shaskan, N., Passeri, E., Berretta, S., et al. (2013). Developmental pattern of perineuronal nets in the human prefrontal cortex and their deficit in schizophrenia. Biol. Psychiatry 74, 427–435. doi: 10.1016/j.biopsych.2013.05.007
McKay, B. E., Molineux, M. L., Mehaffey, W. H., and Tuner, R. W. (2005). KV1 K+ channels control Purkinje cell output to facilitate postsynaptic rebound discharge in deep cerebellar neurons. J. Neurosci. 25, 1481–1492. doi: 10.1523/jneurosci.3523-04.2005
McRae, P. A., and Porter, B. E. (2012). The perineuronal net component of the extracellular matrix in plasticity ans epilepsy. Neurochem. Intern. 61, 963–972. doi: 10.1016/j.neuint.2012.08.007
Medina, J. F., and Mauk, M. D. (1999). Stimulations of cerebellar motor learning: computational analysis of plasticity at the mossy fiber to deep nucleus synapse. J. Neurosci. 19, 7140–7151. doi: 10.1523/jneurosci.19-16-07140.1999
Mendlin, A., Martin, F. J., Rueter, L. E., and Jacobs, B. L. (1996). Neuronal release of serotonin in the cerebellum of behaving rats: an in vivo microdialysis study. J. Neurochem. 67, 617–622. doi: 10.1046/j.1471-4159.1996.67020617.x
Miles, F. A., and Lisberger, S. G. (1981). Plasticity in the vestibule-ocular reflex: a new hypothesis. Annu. Rev. Neurosci. 4, 273–299. doi: 10.1146/annurev.ne.04.030181.001421
Mitoma, M., and Konishi, S. (1999). Monoaminergic long-term facilitation of GABA-mediated inhibitory transmission at cerebellar synapses. Neuroscience 88, 871–883. doi: 10.1016/s0306-4522(98)00260-7
Miyashita, Y., and Watanabe, E. (1984). Loss of vision-guided adaptation of the vestibule-ocular reflex after depletion of brain serotonin in the rabbit. Neurosci. Lett. 51, 177–182. doi: 10.1016/0304-3940(84)90547-0
Miyazaki, T., Yamasaki, M., Tanaka, K. F., and Watanabe, M. (2020). Compartmentalized input-output organization of Lugaro cells in the cerebellar cortex. Neuroscience 462, 89–105. doi: 10.1016/j.neuroscience.2020.05.026
Morawski, M., Brückner, G., Jäger, C., Seeger, G., and Arendt, T. (2010). Neurons associated with aggrecan-based perineuronal nets are protected against tau pathology in subcortical regions in Alzheimer’s disease. Neuroscience 169, 1347–1363. doi: 10.1016/j.neuroscience.2010.05.022
Mouginot, D., and Gähwiler, B. H. (1995). Characterization of synaptic connections between cortex and depp nuclei of the rat cerebellum in vitro. Neuroscience 64, 699–712. doi: 10.1016/0306-4522(94)00456-f
Murano, M., Saitow, F., and Suzuki, H. (2011). Modulatory effects of serotonin on glutamatergic synaptic transmission and lon-term depression in the deep cerebellar nuclei. Neuroscience 172, 118–128. doi: 10.1016/j.neuroscience.2010.10.037
Najac, M., and Raman, I. (2015). Integration of Purkinje cell inhibition by cerebellar nucleo-olivary neurons. J. Neurosci. 35, 544–549. doi: 10.1523/jneurosci.3583-14.2015
Nedelescu, H., Chowdhury, T. G., Wable, G. S., Arbuthnott, G., and Aoki, C. (2017). Cerebellar sub-divisions differ in exercise-induced plasticity of noradrenergicaxons and in their association with resilience to activity-based anorexia. Brain Struct. Funct. 222, 317–339. doi: 10.1007/s00429-016-1220-2
Nelson, T. E., King, J. S., and Bishop, G. A. (1997). Distribution of tyrosine hydroxylase-immunoreactive afferents the the cerebellum differs between species. J. Comp. Neurol. 379, 443–454. doi: 10.1002/(sici)1096-9861(19970317)379:3<443::aid-cne9>3.0.co;2-3
Nicholson, C., and Hrabětová, S. (2017). Brain extracellular space: the final frontier of neuroscience. Biophys. J. 113, 2133–2142. doi: 10.1016/j.bpj.2017.06.052
Nithianantharajah, J., and Hannan, A. J. (2006). Enriched environments, experience-dependet plasticity and disorders of the nervous system. Nat. Rev. Neurosci. 7, 697–709. doi: 10.1038/nrn1970
Oades, R. D., Lasky-Su, J., Christiansen, H., Faraone, S. V., Sonuga-Barke, E. J., Banaschewski, T., et al. (2008). The influence of serotonin- and other genes on impulsive behavioral aggression and cognitive impulsivity in children with attention-deficit/hyperactivity disorder (ADHD): Findings from a family-based association test (FBAT) analysis. Behav. Brain Funct. 4:48. doi: 10.1186/1744-9081-4-48
Obata, K., Ito, M., Ochi, R., and Sato, N. (1967). Pharmacological properties of the postsynaptic inhibition by Purkinje cell axons and the action of γ–aminobutyric acid on Deiters neurons. Exp. Brain Res. 4, 43–57.
Ohyama, T., Nores, W. L., Medina, J. F., Riusech, F. A., and Mauk, M. D. (2006). Learning-induced plasticity in deep cerebellar nucleus. J. Neurosci. 26, 12656–12663. doi: 10.1523/jneurosci.4023-06.2006
Okamoto, T., Endo, S., Shirao, T., and Nagao, S. (2011). Role of cerebellar cortical protein synthesis in transfer of memory trace of cerebellum-dependent motor learning. J. Neurosci. 31, 8958–8966. doi: 10.1523/jneurosci.1151-11.2011
Olson, L., and Fuxe, K. (1971). On the projections from the locus coeruleus noradrenaline neurons: the cerebellar innervation. Brain Res. 28, 165–171. doi: 10.1016/0006-8993(71)90533-6
Oohashi, T., Edamatsu, M., and Carulli, D. (2015). The hyaluronan and proteoglycan link proteins: organizers of the brain extracellular matrix and key molecules for neuronal function and plasticity. Exp. Neurol. 274, 134–144. doi: 10.1016/j.expneurol.2015.09.010
Orduz, D., and Llano, I. (2007). Recurrent axon collaterals underlie facilitating synapses between cerebellar Purkinje cells. Proc. Natl. Acad. Sci. U.S.A. 104, 17831–17836. doi: 10.1073/pnas.0707489104
Palay, S. L., and Chan-Palay, V. (1974). Cerebellar Cortex: Cytology and Organization. Berlin: Springer.
Pang, T. Y. C., and Hannan, A. J. (2013). Enhancement of cognitive function in models of brain disease through environmental enrichment and physical activity. Neuropharmacology 64, 515–528. doi: 10.1016/j.neuropharm.2012.06.029
Pantazopoulos, H., Tsung-Ung, W., Maribel, P., Lange, N., and Berretta, S. (2010). Extracellular matrix-glial abnormalities in the amygdala and entorhinal cortex of subjects diagnosed with schizophrenia. Arch. Gen. Psychiatry 67, 155–166. doi: 10.1001/archgenpsychiatry.2009.196
Paredes, D. A., Cartford, M. C., Catlow, B. J., Samec, A., Avilas, M., George, A., et al. (2009). Neurotransmitter release during delay eyeblink classical conditioning: role of norepinephrine in consolidation and effect of age. Neurobiol. Learn. Mem. 92, 267–282. doi: 10.1016/j.nlm.2008.08.008
Paylor, J. W., Wendlandt, E., Freeman, T. S., Greba, Q., Matks, W. N., Howland, J. G., et al. (2018). Impaired cognitive function after perineuronal net degradation in the medial prefrontal cortex. eNeuro 5:e0253.
Peng, S. Y., Zhuang, Q. X., Zhang, Y. X., Zhang, X. Y., Wang, J. J., and Zhu, J. N. (2016). Excitatory effect of norepinephrine on neurons in the inferior vestibular nucleus and the Underlying receptor mechanism. J. Neurosci. Res. 94, 736–748. doi: 10.1002/jnr.23745
Person, A. L., and Raman, I. M. (2010). Deactivation of L-type Ca current by inhibition controls LTP at excitatory synapses in the cerebellar nuclei. Neuron 66, 550–559. doi: 10.1016/j.neuron.2010.04.024
Pizzorusso, T., Medini, P., Berardi, N., Chierzi, S., Fawcett, J. W., and Maffei, L. (2002). Reactivation of ocular dominance plasticity in the adult visual cortex. Science 298, 1248–1251. doi: 10.1126/science.1072699
Pollock, E., Everest, M., Brown, A., and Poulter, M. O. (2014). Metalloproteinase inhibition prevents inhibitory synapse reorganization and seizure genesis. Neurobiol. Dis. 70, 21–31. doi: 10.1016/j.nbd.2014.06.003
Prestori, F., Mapelli, L., and D’Angelo, E. (2019). Diverse neuron properties and complex network dynamics in the cerebellar cortical inhibitory circuit. Front. Mol. Neurosci. 12:267. doi: 10.3389/fnmol.2019.00267
Proville, R. D., Spolidoro, M., Guyon, N., Dugué, G. P., Selimi, F., Isope, P., et al. (2014). Cerebellum involvement in cortical sensorimotor circuits for the control of voluntary movements. Nat. Neurosci. 17, 1233–1239. doi: 10.1038/nn.3773
Pugh, J. R., and Raman, I. M. (2006). Potentiation of mossy fiber EPSCs in the cerebellar nuclei by NMDA receptor activation followed by postinhibitory rebound current. Neuron 51, 113–123. doi: 10.1016/j.neuron.2006.05.021
Pugh, J. R., and Raman, I. M. (2008). Mechanisms of potentiation of mossy fiber EPSCs in the cerebellar nuclei by coincident synaptic excitation and inhibition. J. Neurosci. 28, 10549–10560. doi: 10.1523/jneurosci.2061-08.2008
Racine, R. J., Wilson, D. A., Gingell, R., and Sunderland, D. (1986). Long-term potentiation in the interpositus and vestibular nuclei in the rat. Exp. Brain Res. 63, 158–162.
Reichelt, A. C., Hare, D. J., Bussey, T. J., and Saksida, L. M. (2019). Perineuronal nets: plasticity, protection and therapeutic potential. Trends Neurosci. 42, 458–470. doi: 10.1016/j.tins.2019.04.003
Romberg, C., Yang, S., Melani, R., Andrews, M. R., Horner, A. E., Spillantini, M. G., et al. (2013). Depletion of perineuronal nets enhances recognition memory and long-term depression in the perirhinal cortex. J. Neurosci. 33, 7057–7065. doi: 10.1523/jneurosci.6267-11.2013
Rowland, N. C., and Jaeger, D. (2005). Coding of tactile response properties in the rat deep cerebellar nuclei. J. Neurophysiol. 94, 1236–1251. doi: 10.1152/jn.00285.2005
Sahin, M., and Hockfield, S. (1990). Molecular identification of the Lugaro cell in the cat cerebellar cortex. J. Comp. Neurol. 301, 575–584. doi: 10.1002/cne.903010407
Saitow, F., Murano, M., and Suzuki, H. (2009). Modulatory effects of serotonin on GABAergic synaptic transmission and membrane properties in the deep cerebellar nuclei. J. Neurophysiol. 101, 1361–1374. doi: 10.1152/jn.90750.2008
Saitow, F., Nagano, M., and Suzuki, H. (2018). Developmental changes in serotonergic modulation of GABAergic synaptic transmission and postsynaptic GABAA receptor composition in the cerebellar nuclei. Cerebellum 17, 346–358. doi: 10.1007/s12311-018-0922-9
Saitow, F., Satake, S., Yamada, J., and Konishi, S. (2000). β-Adrenergic receptor-mediated presynaptic facilitation of inhibitory GABAergic transmission at cerebellar interneuron-Purkinje cell synapses. J. Neurophysiol. 84, 2016–2025. doi: 10.1152/jn.2000.84.4.2016
Sakamoto, T., and Endo, S. (2010). Amygdala, deep cerebellar nuclei and red nucleus contribute to delay eyeblink conditioning in C57BL/6 mice. Eur. J. Neurosci. 32, 1537–1551. doi: 10.1111/j.1460-9568.2010.07406.x
Sale, A., Vetencourt, J. F. M., Medini, P., Cenni, M. C., Baroncelli, L., De Pasquale, R., et al. (2007). Environmental enrichment in adulthood promotes amblyopia recovery through a reduction of intracortical inhibition. Nat. Neurosci. 10, 679–681. doi: 10.1038/nn1899
Schilling, K., Oberdick, J., Rossi, F., and Baader, S. L. (2008). Besides Purkinje cells and granule neurons: an appraisal of the cell biology of the interneurons of the cerebellar cortex. Histochem. Cell Biol. 130, 601–615. doi: 10.1007/s00418-008-0483-y
Schmahmann, J. D., Guell, X., Stoodley, C. J., and Halko, M. A. (2019). The theory and neuroscience of cerebellar cognition. Annu. Rev. Neurosci. 42, 337–364. doi: 10.1146/annurev-neuro-070918-050258
Shen, H. H. (2018). Perineuronal nets gain prominence for their role in learning, memory, and plasticity. Proc. Natl. Acad. Sci. U.S.A. 115, 9813–9815. doi: 10.1073/pnas.1815273115
Shen, Y., Tenney, A. P., Busch, S. A., Horn, K. P., Cuascut, F. X., Liu, K., et al. (2009). PTPsigma is a receptor for chondroitin sulfate proteoglycan, an inhibitor of neural regeneration. Science 326, 592–596. doi: 10.1126/science.1178310
Shi, W., Wei, X., Wang, X., Du, S., Liu, W., Song, J., et al. (2019). Perineuronal nets protect;ong-term memory by limiting activity-dependent inhibition from parvalbumin interneurons. Proc. Natl. Acad. Sci. U.S.A. 116, 27063–27073.
Shin, S. L., and De Schutter, E. (2006). Dynamic synchronization of Purkinje cell simple spikes. J. Neurophysiol. 96, 3485–3491. doi: 10.1152/jn.00570.2006
Shin, S. L., Hoebeek, F. E., Schonewille, M., De Zeeuw, C. I., Aertsen, A., and De Schutter, E. (2007). Regular patterns in cerebellar Purkinje cell simple spike trains. PLoS One 5:e485. doi: 10.1371/journal.pone.0000485
Shutoh, F., Ohki, M., Kitazawa, H., Itohara, S., and Nagao, S. (2006). Memory trace of motor learning shifts transsynaptically from cerebellar cortex to nuclei for consolidation. Neuroscience 139, 767–777. doi: 10.1016/j.neuroscience.2005.12.035
Siggins, G. R., Hoffer, B. J., Oliver, A. P., and Bloom, F. E. (1971). Activation of a central noradrenergic projection to cerebellum. Nature 233, 481–483. doi: 10.1038/233481a0
Simat, M., Parpan, F., and Fritschy, J. M. (2007). Heterogeneity of glycinergic and GABAergic interneurons in the granule cell layer of mouse cerebellum. J. Comp. Neurol. 500, 71–83. doi: 10.1002/cne.21142
Smith, C. C., Mauricio, R., Nobre, L., Marsh, B., Wüst, R. C. I., Rossiter, H. B., et al. (2015). Differential regulation of perineuronal nets in the brain and spinal cord with exercise training. Brain Res. Bull. 111, 20–26. doi: 10.1016/j.brainresbull.2014.12.005
Sorg, B. A., Berretta, S., Blacktop, J. M., Fawcett, J. W., Kitagawa, H., Kwok, J. C. F., et al. (2016). Casting a wide net: role of perineuronal nets in neuronal plasticity. J. Neurosci. 36, 11459–11468. doi: 10.1523/jneurosci.2351-16.2016
Stamenkovic, V., Stamenkovic, S., Jaworski, T., Gawlak, M., Jovanovic, M., Jakovcevski, I., et al. (2017). The extracellular matrix glycoprotein tenascin-C and matrix metalloproteinases modify cerebellar structural plasticity by exposure to an enriched environment. Brain Struct. Funct. 222, 393–415. doi: 10.1007/s00429-016-1224-y
Steuber, V., and Jaeger, D. (2013). Modeling the generation of output by the cerebellar nuclei. Neural Netw. 47, 112–119. doi: 10.1016/j.neunet.2012.11.006
Steullet, P., Cabungcal, J. H., Coyle, J., Didriksen, M., Gill, K., Grace, A. A., et al. (2017). Oxidative streee-driven parvalbumin interneuron impairment as a common mechanism in models of schizophrenia. Mol. Psychiatry 22, 936–943. doi: 10.1038/mp.2017.47
Strahlendorf, J. C., Lee, M., and Strahlendorf, H. K. (1984). Effects of serotonin on cerebellar Purkinje cells are dependent on the baseline firing rate. Exp. Brain Res. 56, 50–58.
Südhof, T. C. (2012). The presynaptic active zone. Neuron 75, 11–25. doi: 10.1016/j.neuron.2012.06.012
Suttkus, A., Morawski, M., and Arendt, T. (2016). Protective properties of neural extracellular matrix. Mol. Neurobiol. 53, 73–82. doi: 10.1007/s12035-014-8990-4
Sylantyev, S., Savtchennko, L. P., Niu, Y. P., Ivanov, A. I., Jensen, T. P., Kullmann, D. M., et al. (2008). Electric fields due to synaptic currents sharpen excitatory transmission. Science 319, 1845–1849. doi: 10.1126/science.1154330
Talley, E. M., Rosin, D. L., Lee, A., Guyenet, P. G., and Lynch, K. R. (1996). Distribution of α2A–adrenergic receptor-like immunoreactivity in the rat central nervous system. J. Comp. Neurol. 372, 111–134. doi: 10.1002/(sici)1096-9861(19960812)372:1<111::aid-cne8>3.0.co;2-6
Telgkamp, P., and Raman, I. M. (2002). Depression of inhibitory synaptic transmission between Purkinje cells and neurons of the cerebellar nuclei. J. Neurosci. 22, 8447–8457. doi: 10.1523/jneurosci.22-19-08447.2002
ten Brinke, M. M., Heiney, S. A., Wang, X., Proietti-Onori, M., Boele, H. J., Bakermans, J., et al. (2017). Dynamic modulation of activity in cerebellar nuclei neurons during pavlovian eyeblink conditioning in mice. ELife 6:e28132.
Testa, D., Prochiantz, A., and Di Nardo, A. A. (2019). Perineuronal nets in brain physiology and disease. Semin. Cell Dev. Biol. 89, 125–135. doi: 10.1016/j.semcdb.2018.09.011
Teune, T. M., van der Burg, J., De Zeeuw, C. I., Voogd, J., and Ruigrok, T. J. H. (1998). Single Purkinje cell can innervate multiple classes of projection neurons in the cerebellar nuclei of the rat: a light microscopic and ultrastructural triple-tracer study in the rat. J. Comp. Neurol. 392, 164–178. doi: 10.1002/(sici)1096-9861(19980309)392:2<164::aid-cne2>3.0.co;2-0
Tewari, B. P., Chaunsali, L., Campbell, S. I., Patel, D. C., Goode, A. E., and Sontheimer, H. (2018). Perineuronal nets decrease membrane capacitance of peritumoral fast spiking interneurons in a model of epilepsy. Nat. Commun. 9:4724.
Trouillas, P., Serratrice, G., Laplane, D., Rascol, A., Augustin, P., Barroche, G., et al. (1995). Levorotatory form of 5-hydroxytryptophan in Friedreich’s ataxia. Results of a double-blind drug-placebo cooperative study. Arch. Neurol. 52, 456–460. doi: 10.1001/archneur.1995.00540290042016
Turecek, J., Jackman, S. L., and Regehr, W. G. (2016). Synaptic specializations support frequency-independent Purkinje cell output from the cerebellar cortex. Cell Rep. 17, 3256–3268. doi: 10.1016/j.celrep.2016.11.081
Uusisaari, M., and Knöpfel, T. (2011). Functional classification of neurons in the mouse lateral cerebellar nuclei. Cerebellum 10, 637–646. doi: 10.1007/s12311-010-0240-3
Uusisaari, M., Obata, K., and Knöpfel, T. (2007). Morphological and electrophysiological properties of GABAergic and non-GABAergic cells in the deep cerebellar nuclei. J. Neurophysiol. 97, 901–911. doi: 10.1152/jn.00974.2006
Uusissari, M., and Knöpfel, T. (2008). GABAergic synaptic communication in the GABAergic and non-GABAergic cells in the deep cerebellar nuclei. Neuroscience 156, 537–549. doi: 10.1016/j.neuroscience.2008.07.060
Van Overwall, F., Baetens, K., Martien, P., and Vandekerckhove, M. (2014). Social cognition and the cerebellum: a meta-analysis of over 350 fMRI studies. Neuroimage 86, 554–572. doi: 10.1016/j.neuroimage.2013.09.033
Veasey, S. C., Fornal, C. A., Metzler, C. W., and Jacobs, B. L. (1995). Response of serotonergic caudal raphe neurons in relation to specific motor activities in freely moving cats. J. Neurosci. 15, 5346–5359. doi: 10.1523/jneurosci.15-07-05346.1995
Veasey, S. C., Fornal, C. A., Metzler, C. W., and Jacobs, B. L. (1997). Single-unit responses of serotonergic dorsal raphe neurons to specific motor challenges in freely moving cats. Neuroscience 79, 161–169. doi: 10.1016/s0306-4522(96)00673-2
Víg, J., Takacs, J., Vastagh, C., Baldauf, Z., Veisenberger, E., and Hamori, J. (2003). Distribution of mGluR1alpha and SMI311 immunoreactive Lugaro cells in the kitten cerebellum. J. Neurocytol. 31, 217–227. doi: 10.1023/b:neur.0000010081.54613.94
Wagner, M. J., Kim, T. H., Kadmon, J., Nguyen, N. D., Ganguli, S., Schnitzer, M. J., et al. (2019). Shared cortex-cerebellum dynamics in the execution and learning of a motor task. Cell 177, 669–682.e624.
Wagner, M. J., Kim, T. H., Savall, J., Schnitzer, M. J., and Luo, L. (2017). Cerebellar granule cells encode the expectation of reward. Nature 544, 96–100. doi: 10.1038/nature21726
Wakita, R., Tanabe, S., Tabei, K., Funaki, A., Inoshita, T., and Hirano, T. (2017). Differential regulations of vestibule-ocular reflex and optokinetic response by β- and α2-adrenergic receptors in the cerebellar flocculus. Sci. Rep. 7:3944.
Watt, A. J., Cuntz, H., Mori, M., Nusser, Z., Sjöström, P. J., and Häusser, M. (2009). Traveling waves in developing cerebellar cortex mediated by asymmetrical Purkinje cell connectivity. Nat. Neurosci. 12, 463–473. doi: 10.1038/nn.2285
Wen, T. H., Binder, D. K., Ethell, I. M., and Razak, K. A. (2018). The perineuronal ‘safety’ net? Perineuronal net abnormalities in neurological disorders. Front. Mol. Neurosci. 11:270. doi: 10.3389/fnmol.2018.00270
Wetmore, D. Z., Mukamel, E. A., and Schnizer, M. S. (2008). Lock-and-key mechanisms of cerebellar memory recall based on rebound currents. J. Neurophysiol. 100, 2328–2347. doi: 10.1152/jn.00344.2007
Witter, L., Rudolph, S., Pressler, R. T., Lahlaf, S. I., and Regehr, W. G. (2016). Purkinje cell collaterals enable output signals from the cerebellar cortex to feed back to Purkinje cells and interneurons. Neuron 91, 312–319. doi: 10.1016/j.neuron.2016.05.037
Wu, Y., and Raman, I. M. (2017). Facilitation of mossy fibre-driven spiking in the cerebellar nuclei by the synchrony of inhibition. J. Physiol. 595, 5245–5264. doi: 10.1113/jp274321
Yarden-Rabinowitz, Y., and Yarom, Y. (2017). In vivo analysis of synaptic activity in cerebellar nuclei neurons unravels the efficacy of excitatory inputs. J. Physiol. 595, 5945–5963. doi: 10.1113/jp274115
Yeo, C. H., Hardiman, M. J., and Glickstein, M. (1985). Classical conditioning of the nictitating membrane response of the rabbit. Exp. Brain Res. 60, 114–126. doi: 10.1007/bf00427434
Yu, Z., Chen, N., Hu, D., Chen, W., Yuan, Y., Meng, S., et al. (2020). Decreased density of perineuronal net in prelimbic cortex is linked to depressive-link behavior in young-age rats. Front. Mol. Neurosci. 13:4. doi: 10.3389/fnmol.2020.00004
Zaki, Y., and Cai, D. J. (2020). Creating space for synaptic formation-a new role for microglia in synaptic plasticity. Cell 182, 265–267. doi: 10.1016/j.cell.2020.06.042
Zaremba, S., Naegele, J. R., Barnstable, C. J., and Hockfield, S. (1990). Neuronal subsets express multiple high-molecular-weight cell-surface glycoconjugates defined by monoclonal antibodies Cat-301 and VC1.1. J. Neurosci. 10, 2985–2995. doi: 10.1523/jneurosci.10-09-02985.1990
Zhang, C. Z., Zhuang, Q. X., He, Y. C., Li, G. Y., Zhu, J. N., and Wang, J. J. (2014). 5-HT2A receptor-mediated excitation on cerebellar fastigial nucleus neurons and promotion of motor behaviors in rats. Pflugers Arch. 466, 1259–1271. doi: 10.1007/s00424-013-1378-x
Zhou, M., Melin, M. D., Xu, W., and Sudhof, T. C. (2020). Dysfunction of parvalbumin neurons in the cerebellar nuclei produces an action tremor. J. Clin. Invest. 130, 5142–5156. doi: 10.1172/jci135802
Keywords: Deep cerebellar nuclei, serotoinin, eyeblink conditioning, axon collateral, noradrenaline, Lugaro cell, globular cell, chondroitinase ABC
Citation: Hirono M, Karube F and Yanagawa Y (2021) Modulatory Effects of Monoamines and Perineuronal Nets on Output of Cerebellar Purkinje Cells. Front. Neural Circuits 15:661899. doi: 10.3389/fncir.2021.661899
Received: 31 January 2021; Accepted: 12 May 2021;
Published: 14 June 2021.
Edited by:
Edouard Pearlstein, Independent researcher, Marseille, FranceReviewed by:
Naofumi Uesaka, The University of Tokyo, JapanTianyi Mao, Oregon Health & Science University, United States
Copyright © 2021 Hirono, Karube and Yanagawa. This is an open-access article distributed under the terms of the Creative Commons Attribution License (CC BY). The use, distribution or reproduction in other forums is permitted, provided the original author(s) and the copyright owner(s) are credited and that the original publication in this journal is cited, in accordance with accepted academic practice. No use, distribution or reproduction is permitted which does not comply with these terms.
*Correspondence: Moritoshi Hirono, bWhpcm9ub0B3YWtheWFtYS1tZWQuYWMuanA=