- 1Department of Applied Physiology & Kinesiology, University of Florida, Gainesville, FL, United States
- 2KBR, Inc., Houston, TX, United States
- 3Department of Psychology, University of Michigan, Ann Arbor, MI, United States
- 4NASA Johnson Space Center, Houston, TX, United States
- 5Norman Fixel Institute for Neurological Diseases, University of Florida, Gainesville, FL, United States
Astronauts returning from spaceflight typically show transient declines in mobility and balance. Other sensorimotor behaviors and cognitive function have not been investigated as much. Here, we tested whether spaceflight affects performance on various sensorimotor and cognitive tasks during and after missions to the International Space Station (ISS). We obtained mobility (Functional Mobility Test), balance (Sensory Organization Test-5), bimanual coordination (bimanual Purdue Pegboard), cognitive-motor dual-tasking and various other cognitive measures (Digit Symbol Substitution Test, Cube Rotation, Card Rotation, Rod and Frame Test) before, during and after 15 astronauts completed 6 month missions aboard the ISS. We used linear mixed effect models to analyze performance changes due to entering the microgravity environment, behavioral adaptations aboard the ISS and subsequent recovery from microgravity. We observed declines in mobility and balance from pre- to post-flight, suggesting disruption and/or down weighting of vestibular inputs; these behaviors recovered to baseline levels within 30 days post-flight. We also identified bimanual coordination declines from pre- to post-flight and recovery to baseline levels within 30 days post-flight. There were no changes in dual-task performance during or following spaceflight. Cube rotation response time significantly improved from pre- to post-flight, suggestive of practice effects. There was also a trend for better in-flight cube rotation performance on the ISS when crewmembers had their feet in foot loops on the “floor” throughout the task. This suggests that tactile inputs to the foot sole aided orientation. Overall, these results suggest that sensory reweighting due to the microgravity environment of spaceflight affected sensorimotor performance, while cognitive performance was maintained. A shift from exocentric (gravity) spatial references on Earth toward an egocentric spatial reference may also occur aboard the ISS. Upon return to Earth, microgravity adaptions become maladaptive for certain postural tasks, resulting in transient sensorimotor performance declines that recover within 30 days.
Introduction
There are well-documented changes in human sensorimotor performance following spaceflight, including post-flight declines in locomotion, balance, and fine motor control (Thornton and Rummel, 1977; Paloski et al., 1992, 1994; Reschke et al., 1994a,b, 1998; Black et al., 1995; McDonald et al., 1996; Bloomberg et al., 1997; Layne et al., 1997, 1998; Newman et al., 1997; Bock et al., 2003; Campbell et al., 2005; Rafiq et al., 2006). However, the effects of spaceflight on human cognition and other motor behaviors have not been as thoroughly investigated (Strangman et al., 2014; Garrett-Bakelman et al., 2019). Performance of whole-body postural control typically returns to pre-flight levels within approximately 2 weeks of return to Earth (Wood et al., 2015; Ozdemir et al., 2018), however, it is not clear whether the same is true for other sensorimotor or cognitive behaviors.
Vestibular inputs are altered during spaceflight; in particular, otolith (small structures within the inner ear that senses linear accelerations and tilt) signaling of head tilt, which rely upon gravity, is absent and is likely down-weighted (Paloski et al., 1992, 1994; Reschke et al., 1994a,b, 1998; Black et al., 1995, 1999; Clément et al., 2020). The central nervous system adapts to altered vestibular inputs in-flight due to microgravity with as little as 2 weeks spent in spaceflight (Layne et al., 1998). Upon return to Earth, however, these adaptive changes may become maladaptive, resulting in difficulties with whole-body motor control. Post-flight impairments have been reported during locomotion (McDonald et al., 1996; Bloomberg et al., 1997; Layne et al., 1998; Miller et al., 2018; Mulavara et al., 2018), balance (Paloski et al., 1992, 1994; Reschke et al., 1994a,b, 1998; Black et al., 1995, 1999), jumping (Newman et al., 1997), obstacle navigation (Mulavara et al., 2010; Bloomberg et al., 2015), and eye-head coordination (Reschke et al., 2017). Sensorimotor re-adaptation to the Earth’s gravity occurs in the weeks following return, with performance returning to pre-flight levels with about 6 days on a variety of functional tasks (Miller et al., 2018) to 15 days for the functional mobility test (FMT; Mulavara et al., 2010).
In-flight changes in performance of fine motor tasks have also been identified. For instance, astronauts maintained their manual dexterity while performing survival surgery on rats during a Neurolab shuttle mission. However, there was a significant increase in operative time, in some cases taking 1.5–2 times longer than on Earth (Campbell et al., 2005), which may be indicative of a speed-accuracy trade-off or slowing down to avoid compromising in accuracy. Indices of movement variability, reaction time, and movement duration also increased on a hand pointing task executed without visual feedback during Neurolab shuttle missions (Bock et al., 2003), in addition to a significant increase in movement amplitude shortly following landing. During Skylab missions, impairments in reaching and grasping were also documented (Thornton and Rummel, 1977). Additionally, decreases in both force regulation and performance quality while tying surgical knots were identified in the low gravity phase of parabolic flight (Rafiq et al., 2006). Recently, it has been shown that long duration spaceflight results in decreases in fine motor control, as seen by an increase in completion time on a grooved pegboard test (Mulavara et al., 2018). Here we evaluate bimanual motor coordination pre- and post-flight using the bimanual Purdue Pegboard Test, in which astronauts were asked to place small metal pegs into fitted holes as quickly as possible using both hands simultaneously (Tiffin and Asher, 1948).
Several spaceflight stressors have the potential to impact cognition in-flight, including the effects sleep loss, motion sickness, and social isolation. Astronauts anecdotally report so-called “space fog,” which includes attention lapses, short term memory problems, confusion, and psychomotor problems (Clément et al., 2020). To date, empirical evidence for cognitive effects of spaceflight have been equivocal (c.f. Strangman et al., 2014). One study showed crewmembers were better able to mentally rotate the visual image of their environment as their exposure to microgravity increased, yet also a decreased ability in spatial orientation of written letters during the first 5 days in-flight (Clement et al., 1987). The authors posited that the absence of a gravitational reference field (e.g., the ground) may affect the central representation of movements.
Manzey et al. (1995) and Manzey and Lorenz (1998) have also reported declines in crewmembers ability to perform simultaneous cognitive and motor dual-tasking in-flight. The authors suggested that an increased demand for cognitive control of movement in microgravity may interfere with simultaneous cognitive task performance. Deficits in dual-tasking was further supported by Bock et al. (2010), who found higher tracking error inflight in both the single and dual-task conditions as well as higher dual-task cost in a rhythm production reaction-time task compared to a visuospatial reaction-time task and a choice reaction-time task. The authors suggested that there may a scarcity of neural resources required for complex motor programming due to sensorimotor adaptation to microgravity. Dual-tasking deficits in astronauts post-flight were also identified when astronauts performed a tracking task whilst responding and entering numerical codes with their non-dominant hand (Moore et al., 2019). In addition, NASA’s “Twins Study” also showed increased risk-taking on a cognitive task throughout spaceflight, as well as decreased accuracy in a visual object learning task, decreased abstract shape matching, and decreased cognitive speed for all measures on a subset of tasks from the Penn Computer Neurocognitive Battery, except for the digit symbol substitution task post-flight (Garrett-Bakelman et al., 2019). However, the Twins Study only tested one astronaut in-flight and compared performance to that of their Earth-bound twin, and other previous investigations similarly were case studies (Manzey et al., 1995; Manzey and Lorenz, 1998) or had small sample sizes (n = 3; Bock et al., 2010). Thus it remains unclear whether or how cognitive function is impacted by spaceflight. Spaceflight analog environments, such as extended isolation (Stahn et al., 2019) have been shown to reduce spatial cognition. Moreover, head-down tilt bed rest (HDBR) analogs has been shown to result in an overall cognitive slowing (Basner et al., 2021). Moreover, spatial orientation and distance estimation are impaired during both hypergravity and microgravity phases of parabolic flight (Clément et al., 2016). Here we also evaluated performance on a range of cognitive assessments pre- and post-flight.
As NASA’s goals shift from the International Space Station (ISS) to the Moon and Mars, mission duration will increase. It is imperative that we understand how other factors may interact with microgravity to affect sensorimotor and cognitive function, particularly flight duration, age and sex. Exploration missions to Mars’ surface are estimated to take around 30 months in total (Clément et al., 2020), making it important to understand how mission duration interacts with changes in sensorimotor and cognitive function with spaceflight. Associations between mission duration and the magnitude of brain structural changes, free water shifts, and ventricular enlargement have been previously reported (Roberts et al., 2015; Alperin et al., 2017; Hupfeld et al., 2020a). There is also evidence that longer flight duration results in prolonged brain and behavior recovery profiles (Bryanov et al., 1976; Hupfeld et al., 2020a). Flight duration may also be correlated with the magnitude of sensorimotor and cognitive changes that occur with spaceflight, or that effects of flight duration may be due to an interaction of microgravity with isolation and confinement hazards.
As age increases, sensorimotor adaptability declines (Seidler et al., 2010; Anguera et al., 2011). Astronaut training requires years to complete, and the average age for an astronaut at the onset of their first mission is 39.8 (±5.28) years (Smith et al., 2020). It is important to consider the impact of age on behavioral changes with spaceflight; thus we include age as a covariate in all statistical models for exploratory purposes. Sex differences in the effects of microgravity have rarely been considered [as the Astronaut Corps has been historically male (Reschke et al., 2014)], but with the future Artemis program having equal representation of the sexes, it is important to identify any sex related differences. While our sample size of 15 astronauts is not large enough for a well-powered investigation of sex effects, we include sex as a model covariate for exploratory purposes.
Here we aimed to investigate how spaceflight impacts sensorimotor and cognitive performance. We included several assessments of whole-body sensorimotor behaviors including the Functional Mobility Test (FMT; Mulavara et al., 2010) in which astronauts completed a short obstacle course and the Sensory Organization Test-5 (SOT-5; Reschke et al., 2009; Wood et al., 2012), which was implemented using computerized dynamic posturography and required astronauts to maintain upright posture. We also assessed fine motor control using the bimanual Purdue Pegboard Test (Tiffin and Asher, 1948). Finally, we assayed multiple aspects of cognitive function including processing speed, mental rotation, spatial working memory and cognitive-motor dual-tasking. Most tests were administered pre- and post-flight, with a subset of the test battery performed on three occasions on the ISS. Follow-up performance measurements were obtained over 6 months post-flight to characterize the trajectory of re-adaptation following return to Earth.
Based on prior investigations of behavioral changes with spaceflight (Mulavara et al., 2010; Wood et al., 2015), we hypothesized that performance on all sensorimotor tasks would decline from pre- to post-flight, and then recover to pre-flight levels within 1 month following return to Earth. We further hypothesized that performance on cognitive tasks would decrease from pre- to post-flight, with a similar recovery profile as the sensorimotor tasks. Finally, we hypothesized that astronauts’ sensorimotor and cognitive (i.e., dual-tasking and spatial working memory) performance would be disrupted following their arrival to the ISS, and would then resolve throughout the flight as they adapted to the microgravity environment.
Materials and Methods
Participants
Fifteen astronauts participated in this study (Table 1). One withdrew from the study prior to their last post-flight testing session. The mean age at launch in this study was 47.7 years (±6.3 SD). 27.6% of the participants were female. Mission duration to the ISS lasted an average of 188 days (±57 SD). 40% of astronauts had previous flight experience, having spent an average of 75 days (±131 SD) in space across an average of 0.8 (±1.15 SD) previous missions. An average of 5.8 years (±1.6 SD) had elapsed since the end of their previous mission. The University of Michigan, University of Florida, and NASA Institutional Review Boards approved all study procedures. All participants provided their written informed consent. This study was implemented as part of a larger NASA-funded project (NASA #NNX11AR02G) aiming to investigate the extent, longevity, and neural bases of long-duration spaceflight-induced changes in sensorimotor and cognitive performance (Koppelmans et al., 2013).
Behavioral Assessments
Sensorimotor Measures
Whole-body postural and locomotor control
To assess performance changes in relation to spaceflight for whole-body postural control, we administered several balance and locomotion tests. We used the Functional Mobility Test (FMT; Mulavara et al., 2010) to assess ambulatory mobility. This test was designed to assess movements similar to those required during spacecraft egress, which are measured by total completion time. The FMT is a 6 m × 4 m obstacle course that requires participants to step over, under and around foam obstacles and change heading direction. Participants start from an upright seated position, buckled into a 5-point harness. After releasing their harness and standing up, they walked on a firm surface for the first half of the test and on a medium density foam for the second half. This compliant foam makes surface support and proprioceptive inputs unreliable (Mulavara et al., 2010). Astronauts performed the FMT 10 times as quickly as possible. For analysis purposes we only analyzed completion time on the first trial to minimize the effects of task learning.
Dynamic postural control was assessed using Computerized Dynamic Posturography (Equitest, NeuroCom International, Clackamas, OR, United States; Reschke et al., 2009). Specifically, astronauts completed the Sensory Organization Test-5 (SOT-5 and SOT-5M). We administered SOT-5, in which the eyes are closed and the platform is sway-referenced, requiring greater reliance on vestibular inputs. We also administered SOT-5M, in which participants make ±20° head pitch movements at 0.33 Hz paced by an auditory tone (Wood et al., 2012). At each pre- and post-flight time point, we administered three trials each of the SOT-5 and SOT-5M. Equilibrium Quotient scores were derived from peak-to-peak excursion of the center of mass (estimated at 55% of total height) over a 20-s trial (Nashner, 1972; Paloski et al., 1992). As in our previous work, we used the median Equilibrium Quotient score from each time point in all statistical analyses (Lee et al., 2019).
Fine motor control
To assess bimanual coordination, we used the bimanual Purdue Pegboard Test (Tiffin and Asher, 1948). The bimanual Purdue Pegboard Test is a well-validated measure of bimanual manual dexterity. Participants were instructed to place 15 small metal pegs into fitted holes. We used their completion time to place all the pegs with both hands for statistical analysis.
Cognitive Measures
Cognitive-motor dual-tasking
We assessed dual-tasking using a motor and a cognitive task, each performed separately and simultaneously. The motor task required the participant to perform a two-choice button press in response to an “X” displayed in one of two boxes positioned on either side of the computer screen, cueing the participants to press the button on the corresponding side. The cognitive task required participants to monitor a separate box that rapidly changed colors and to count the number of times that the box turned blue (this occurred infrequently relative to other colors, making this similar to an oddball detection task). Each task was performed alone in a single task (ST) conditions as well as together in a dual-task (DT) condition. Performance declines between single to dual-task conditions are frequently referred to as dual-task cost (DTC). DTC has been shown to be a marker of resource limitation for task performance (Tombu and Jolicoeur, 2003) and served as our performance metric. DTC was calculated as the change in performance accuracy of dual-tasking relative to single tasking [(DT−ST)/ST∗100]. Higher DTC during spaceflight would suggest more interference and higher processing loads. We have previously used this task to analyze dual-tasking changes in HDBR analog environments (Yuan et al., 2016; Mahadevan et al., 2021).
Spatial working memory
We used three tasks to assess spatial working memory; (1) a spatial working memory task (SWM; Anguera et al., 2010), (2) Thurstone’s 2D card rotation test (Ekstrom and Harman, 1976) and (3) three-dimensional cube figure mental rotation task (Shepard and Metzler, 1988). During the SWM task, participants were instructed to mentally connect three dots that formed the points of a triangle. Then, after a 3,000 ms retention phase three new dots appeared on the screen and the participant had to decide if those dots formed the same triangle but rotated, or a different triangle. Participants also performed a control task in which they were shown three dots forming a triangle and then, following a 500 ms retention phase, one dot appeared and they were asked to identify if that dot was one of the original three (Anguera et al., 2010; Salazar et al., 2020). We collected 30 trials of each task. For both tests, we used the response time and number of correct responses as our outcome measures. During the 2D card rotation task, participants first were presented with a 2D drawing of an abstract shape. Then they were presented with another drawing and were instructed to identify if it was the same shape rotated or a different shape (the original shape mirrored or altogether different) (Ekstrom and Harman, 1976; Salazar et al., 2020). The completion time, trials completed and accuracy were recorded and utilized for subsequent statistical analyses. Finally, the cube rotation task required participants to observe a 3D cube assembly for 3 s. Following a 2 s retention phase, two new cube assemblies appeared on the screen and the participant was instructed to identify which of the two matched the initial target image (Shepard and Metzler, 1988; Salazar et al., 2020). Reaction time and accuracy were analyzed for this task. The 3D cube rotation task was administered twice per session while in spaceflight; it was first performed while participants free floating in microgravity (referred to as Cube 1, tethered to a workstation), then with the crewmember in a posture that mimics a seated position with the feet on the “floor” in foot loops (referred to as Cube 2).
Rod and frame test
Visual field dependence was assessed with the Rod and Frame Test (RFT); in which the participant looks into a “tunnel” (to remove peripheral visual cues) and attempts to align a rod to a vertical position parallel to Earth’s gravitational vector, despite a frame around the rod which may be tilted. This test has been shown to identify visual reliance (Witkin and Asch, 1948). Outcome measures for the RFT were frame effect, measured as the angular deviation between the participants perceived vertical and true vertical, and the response consistency (sometimes referred to as response “variability,” although in the present work we will refer to this metric as response consistency).
Digit symbol substitution task
We utilized the digit symbol substitution test (DSST) to analyze cognitive processing speed. During this task, participants were presented with a sheet of paper that required them to match numbers with symbols according to a key that is provided at the top of the page (Weschler, 1986). We used completion time and the number correct as outcome measures for our subsequent statistical analyses.
Testing Timeline
As shown in Figure 1, astronauts performed all behavioral tasks prior to launch (180 and 60 days pre-flight), and four times following their return to Earth (approximately 4, 30, 90, and 180 days post-flight). The initial testing point of 180 days before launch (L-180) was used as a familiarization session and was not included in the analyses here. Sensorimotor (FMT, SOT-5, SOT-5M, and Bimanual Pegboard) and cognitive (DSST, Card Rotation, RFT, SWM, and dual-tasking) tasks were all measured 60 days before launch (L-60) and then within a few days of returning to Earth to elucidate the effects of long-term microgravity exposure. SOT-5 and SOT-5M data had an additional data collection time point approximately 1 day following post-flight. These same measures were all recorded over the following 6 months post-flight, which allowed us to investigate recovery from any performance changes that occurred due to spaceflight and the microgravity environment. The first post-flight testing session occurred between 1 and 7 days after landing; to account for inter-subject differences in this timing, the number of day’s difference between landing and the first post-flight time point was used as a model covariate in our statistical analyses. In addition, a subset of tasks (cube rotation and dual-tasking) were collected three times during spaceflight [FD (Flight Day) 30, FD90 and FD150]; this allowed us to determine the direct effects of microgravity on performance of these tasks.
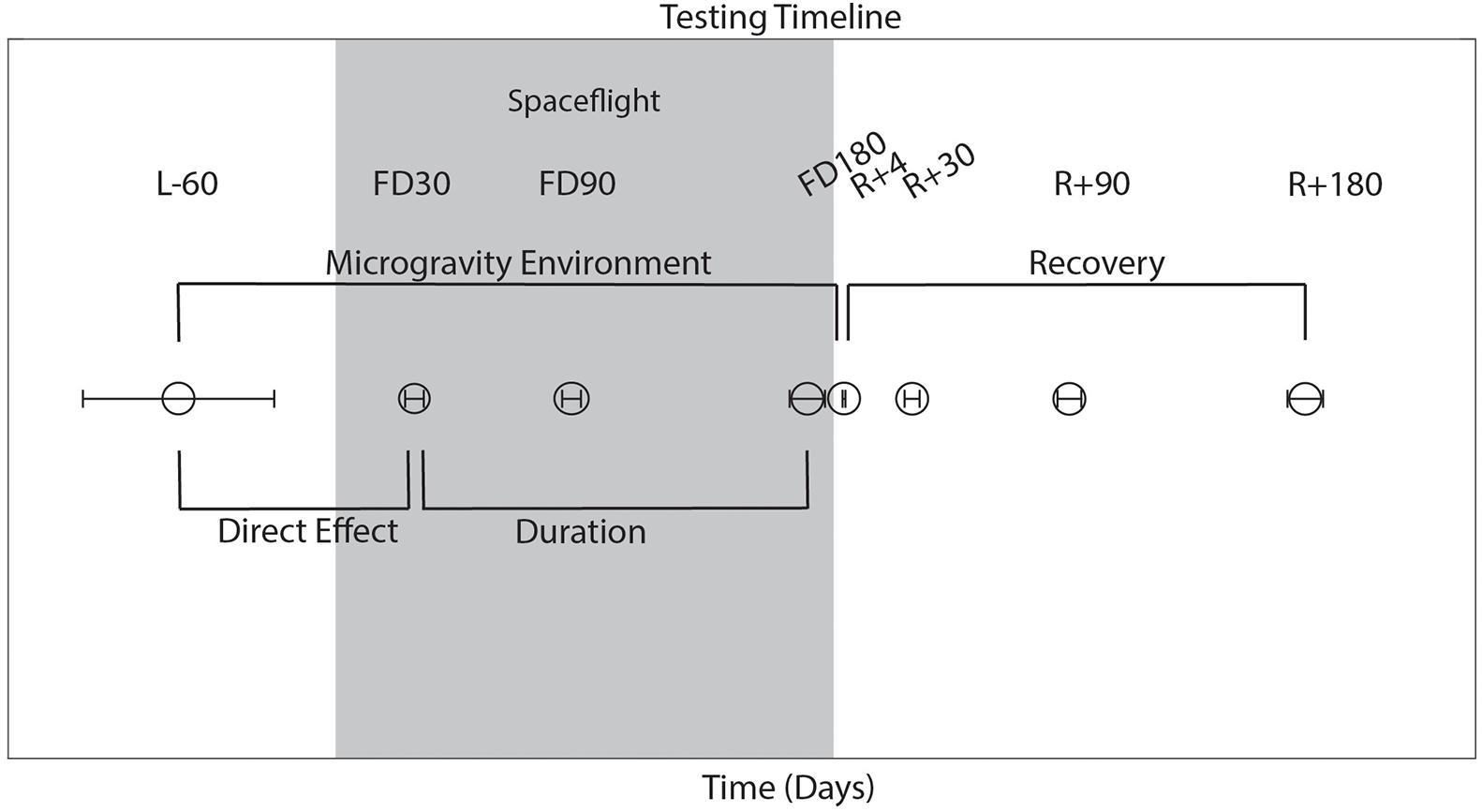
Figure 1. Testing timeline. L, launch; R, return; FD, flight day, time spent during spaceflight. Launch occurred on day 0. The average day of data collection is plotted relative to launch, with error bars indicating standard deviation.
Statistical Analyses
We used the nlme package (Pinheiro et al., 2021) in R 3.6.1 (R Core Team, 2019) to fit linear mixed effects models with restricted maximum likelihood (REML) estimation for performance changes over time. In each model subject we entered subject as a random intercept to allow for different starting points for each person (as in our previous work Koppelmans et al., 2017). Specifically, our first model evaluated the effect of the microgravity environment, testing for pre-flight (L-60) to post-flight (R + 1/R + 4) changes. Our second model evaluated the recovery from the microgravity environment, testing for changes across post-flight time points (R + 1/R + 4, R + 30, R + 90, and R + 180) in measures that showed significant change pre- to post-flight. Our third model evaluated the direct effects of microgravity, testing for performance changes from pre-flight (L-60) to the first in-flight test day (FD30). Our final model evaluated the effects of duration aboard the ISS, testing for changes in performance across the three in-flight test sessions (FD30, FD90, and FD150) on select measures. For 3 of the 17 measures analyzed (Card rotation completed, Tap DTC, and Count DTC), the residuals were not normally distributed. We addressed this by log transforming the data prior to statistical analyses (Ives, 2015), however, for these three measures transformation did not normally distribute the residuals. Given this, the results of the Card rotation number completed, Tap DTC, and Count DTC models should be interpreted with caution. To account for multiple comparisons, we corrected p-values within each of the models using the Benjamini–Hochberg false discovery rate (FDR) correction (Benjamini and Hochberg, 1995); we present the FDR-corrected p-values in Tables 2–5.
Model (1) the effect of the microgravity environment
In this model, we considered time as a (fixed effect) categorical variable (pre-flight versus post-flight). We were primarily interested in the statistical significance of this categorical variable (i.e., whether any pre-flight to post-flight changes in performance occurred). We adjusted for the timing variability of the first post-flight session day (R + 1 or R + 4) by including the (mean-centered) time between landing and the first post-flight session as a covariate, as re-adaptation likely begins as soon as astronauts return to Earth. Mean centered age at launch, sex, and total flight duration were also entered into the model as covariates.
Model (2) recovery from the microgravity environment
This model was only applied for measures where we observed significant changes from pre- to post-flight in model 1, in order to assess post-flight re-adaptation to Earth’s gravitational environment. Here, the fixed effect of time was considered as a continuous variable; we were primarily interested in whether there was a significant effect of time across these post-flight session, to assess the post-flight recovery profile. As in model 1, mean centered age at launch, sex, and total flight duration were included as covariates.
Model (3) direct effects of microgravity
This model only measured in-flight performance. We utilized time as a continuous variable to evaluate performance changes from pre-flight (L-60) to the first in-flight time point (FD30). Only the in-flight metrics (cube rotation, dual-tasking) were included in this analysis. Mean centered age and sex were included as covariates.
Model (4) effects of duration aboard the ISS
This model only measured in-flight performance for the duration of the mission. We utilized time as a continuous variable to evaluate changes in performance across the three testing periods during spaceflight (FD30, FD90, and FD150). Mean centered age and sex were included as covariates. Since conditions for Cube 2 could only be replicated in spaceflight, we tested for a main effect (cube 1 vs. cube 2) for this task.
Results
Tables 3–6 present all results from the statistical models. Bolded and underlined results remained significant at FDR < 0.05. Italicized and underlined results were significant before FDR correction, but did not remain significant following FDR correction.
The Effect of the Microgravity Environment
We identified significant pre-flight to post-flight performance declines in all sensorimotor tasks (Table 3). FMT completion time increased from pre- to post-flight (p = 0.001, Figure 2) as astronauts were slower post-flight. We also identified significant pre-flight to post-flight balance declines, reflected as decreases in Equilibrium Quotient scores on both the SOT-5 (p = 0.010, Figure 3), and SOT-5M (p = 0.001, Figure 4). Astronauts also showed a significant increase in completion time on the bimanual Purdue Pegboard Test; that is, they were slower to complete the task post-flight (p = 0.008, Figure 5).
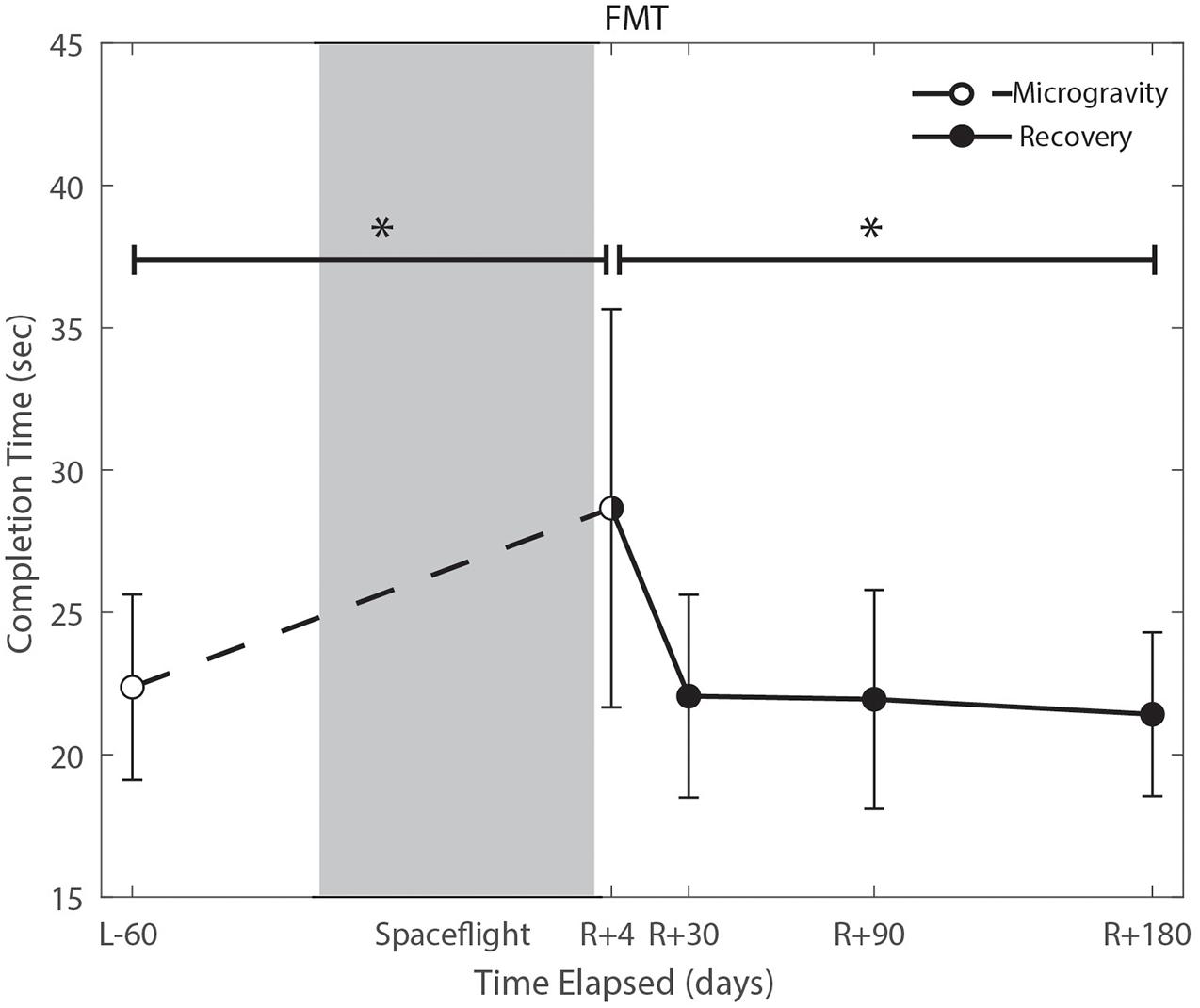
Figure 2. Functional mobility test (FMT) performance changes from pre- to post-flight and post-flight recovery. Spaceflight resulted in a significant decrease in completion time (p = 0.001). Completion time recovered to baseline levels by approximately 30 days post-flight (p = 0.0001). *Indicates statistical results that are p < 0.05.
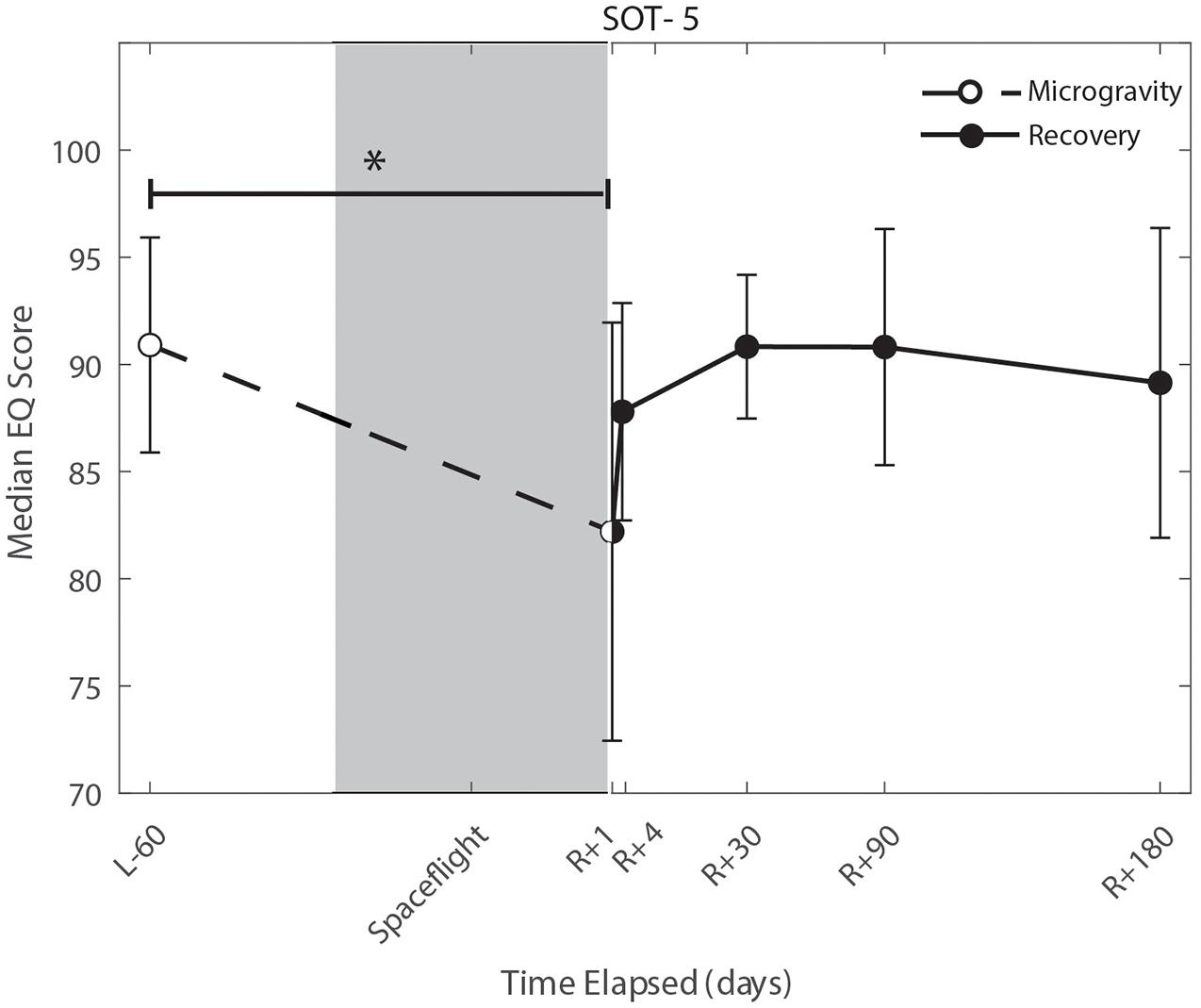
Figure 3. Balance (SOT-5) changes from pre- to post-flight and post-flight recovery. The Sensory Organization Task 5 (SOT-5) performance changes indicate that the microgravity environment resulted in a significant decrease in Equilibrium Score (p = 0.01), that did not show statistically significant recovery. *Indicates statistical results that are p < 0.05.
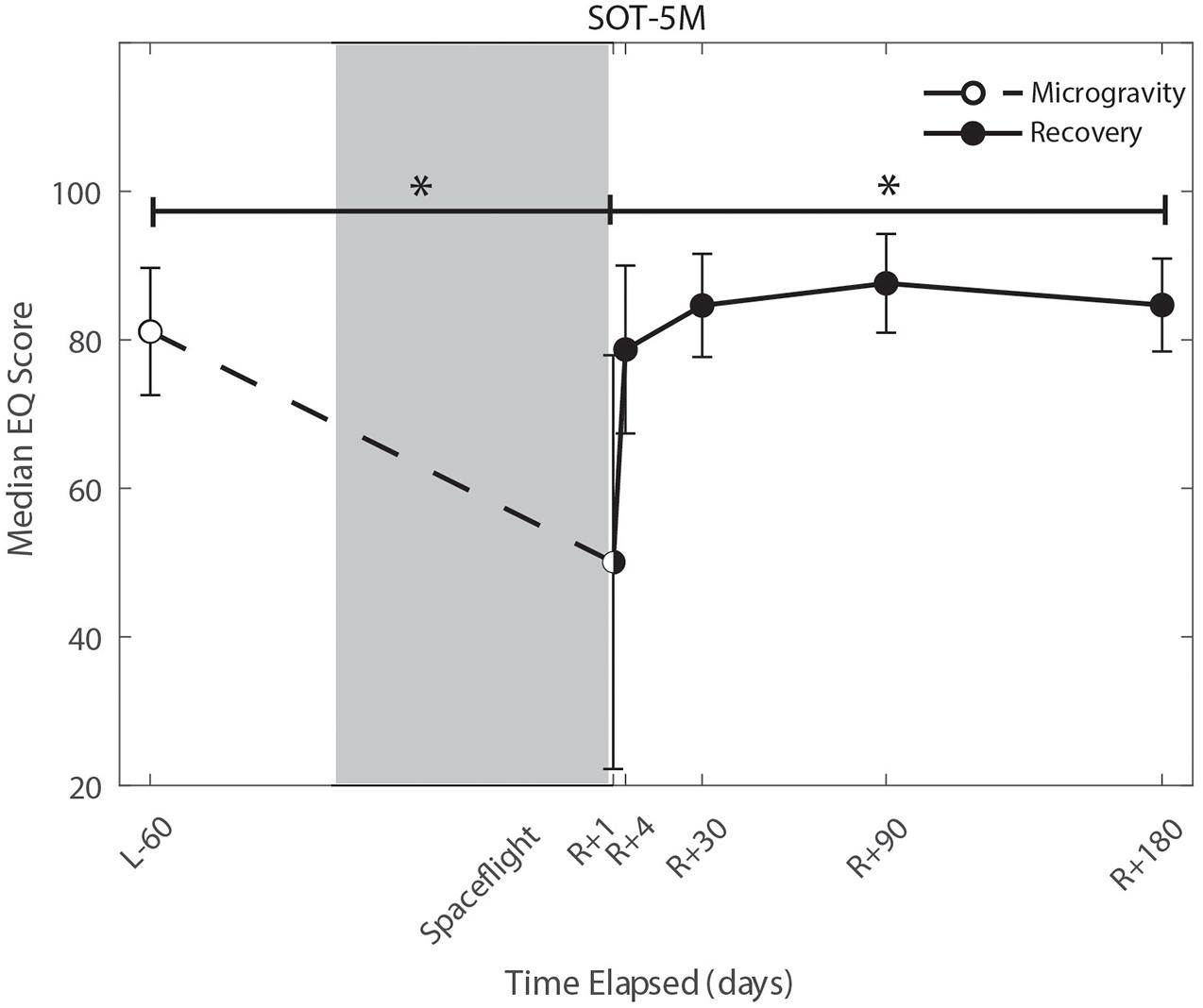
Figure 4. Balance (SOT-5M) changes from pre- to post-flight and post-flight recovery. Sensory Organization Task 5 with head movements (SOT-5M) performance changes indicate that the microgravity environment resulted in a significant decrease in Equilibrium Score (p = 0.001). There was a significant recovery of performance following spaceflight (p = 0.005). *Indicates statistical results that are p < 0.05.
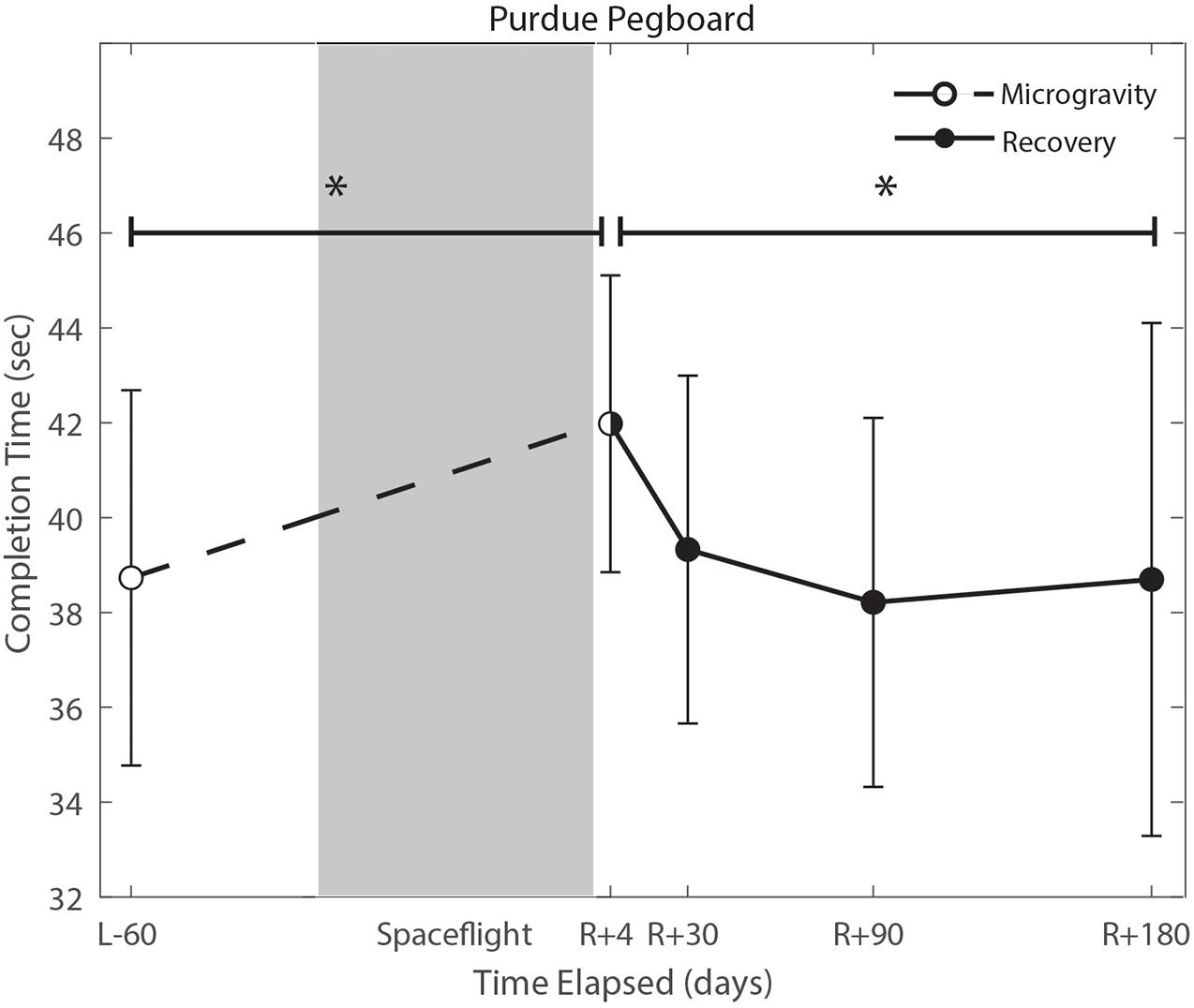
Figure 5. Bimanual purdue pegboard completion time changes from pre- to post-flight and post-flight recovery. There was a significant increase in completion time (p = 0.008) pre- to post-flight. There was a significant change in recovery (p = 0.016). *Indicates statistical results that are p < 0.05.
With the exception of cube rotation, no cognitive assessments showed pre-flight to post-flight changes. Cube rotation response time decreased significantly post-flight (p = 0.004; Figure 6); as astronauts showed faster cube rotation completion time post-flight. We also identified a significant effect of days since landing on the SWM control task (p = 0.049), such that a longer time delay between landing and the first post-flight session was associated with better SWM control task performance.
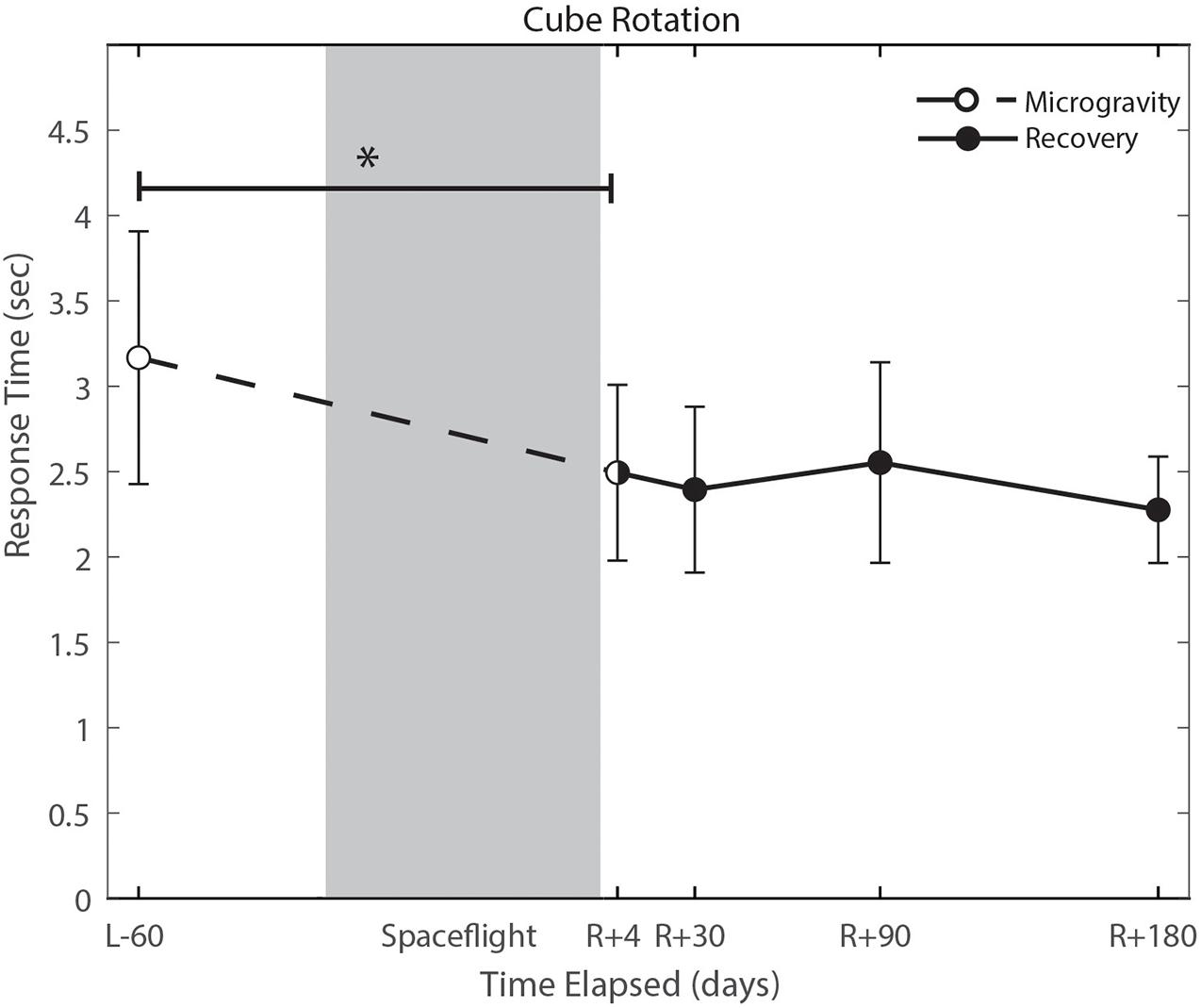
Figure 6. Cube rotation performance changes from pre- to post-flight and post-flight recovery. Subject’s response time decreased significantly (p = 0.004). *Indicates statistical results that are p < 0.05.
Recovery From the Microgravity Environment
Of the measures that changed significantly from pre-flight to post-flight, we observed significant post-flight recovery (Table 4) on the bimanual Purdue Pegboard Test (p = 0.016; Figure 5), FMT (p = 0.0001; Figure 2) and the SOT-5M (p = 0.005; Figure 4). Astronauts’ performance on the bimanual Purdue Pegboard Test returned to near baseline levels by 30 days post-flight and continued to improve by 90 days post-flight (Figure 5). FMT performance showed similar trends, with a return to pre-flight performance levels by R + 30 (Figure 2). SOT-5M scores showed substantial improvements in performance from R + 1 to R + 4 that continued to improve at R + 30 and R + 90 before plateauing (Figure 4).
Direct Effects of Microgravity
Astronauts performed two cognitive tasks (cube rotation and dual-tasking) aboard the ISS, first approximately 30 days after their arrival. There were no significant pre- to in-flight performance changes on these tasks (Table 5).
Effects of Duration on the International Space Station
There were no significant changes in performance of the cube rotation or dual-tasking assessments across the three in-flight time points (Table 6).
Discussion
The current study was designed to investigate sensorimotor and cognitive performance changes associated with long-duration spaceflight and their subsequent recovery post-flight. Consistent with previous results (Reschke et al., 1994a,b, 1998; McDonald et al., 1996; Bloomberg et al., 1997; Layne et al., 1998; Mulavara et al., 2010; Miller et al., 2018; Mulavara et al., 2018), we found pre- to post-flight declines in balance and mobility. There were also declines in bimanual coordination from pre- to post-flight, as indicated by poorer performance on the bimanual Purdue Pegboard Test. All of these measures were shown to recover by 30 days after return to Earth. There were no significant effects of spaceflight on the cognitive measures collected here, including pre- to post-flight and pre- to in-flight performance comparisons.
Whole-Body Postural and Locomotor Control
Sensorimotor deficits due to spaceflight have been previously reported following both short (weeks) and long (months) duration spaceflight (Reschke et al., 1994a,b, 1998; McDonald et al., 1996; Bloomberg et al., 1997; Layne et al., 1998; Mulavara et al., 2010; Miller et al., 2018; Mulavara et al., 2018); here, we find similar declines and subsequent recovery profiles in locomotion and balance. These balance and gait findings support the argument that adaptive sensory reweighting occurs during spaceflight. While in the microgravity environment of space, the otoliths cannot signal head position relative to gravity but rather just linear accelerations. Thus, head movements do not result in the same sensory feedback as on Earth; the central nervous system adapts to this by upweighting other sensory inputs (e.g., visual and proprioceptive inputs) to maintain the body’s ability to orient (Clarke et al., 2000; Hupfeld et al., 2021). Upon return to Earth, vestibular afferent inputs are first overly sensitive to linear accelerations (Boyle et al., 2001) and otolith mediated reflexes sensitive to head tilt are reduced (Kornilova et al., 2012; Hallgren et al., 2016). This suggests that in-flight sensory reweighting is likely maladaptive upon return to Earth, requires re-adaptation. In Figures 3, 4, SOT-5 and SOT-5M performance show significant deficits at R + 1; however, by R + 4 postural control has returned to near baseline levels. There appear to be some slow, persisting effects out to R + 30, suggesting both rapid and slower re-adaptation processes. Adaptation of reaching movements to visuomotor conflict (e.g., visuomotor rotation where visual feedback is offset as a perturbation) on Earth has been well-studied. This literature suggests that early adaptive changes are more cognitive and strategic in nature whereas slower changes reflect more implicit, procedural processes (Anguera et al., 2010; Taylor and Ivry, 2013; McDougle et al., 2015; Christou et al., 2016). It is unclear whether similar processes are at work when adapting to sensory conflict on Earth and adapting to the sensory conflict created by microgravity, but the initial fast recovery followed by a slower timeline to reach pre-flight levels suggests the possibility of similar processes.
Fine Motor Control
Novel findings here include a significant increase in bimanual Purdue Pegboard Test completion time. We fit a linear regression model between age and bimanual Purdue Pegboard completion time in a control sample of 24 subjects (mean age 33.3 years, 8 female), and found that completion time increased by 0.13 s per year of age. The reported increase of 3.25 s exhibited by crewmembers is approximately equivalent to a 25 years age difference. However, it should be noted that the controls were, on average, 14 years younger than astronaut crewmembers, which may result in overestimation of years decline pre to postflight. Previous reports of fine motor control declines following spaceflight include impairments in force modulation (Rafiq et al., 2006), surgical operating completion time (Campbell et al., 2005), keyed pegboard completion time (Mulavara et al., 2018), decreased unimanual Purdue Pegboard performance (Moore et al., 2019), and prolonged reaction time, movement duration, and response amplitude (Bock et al., 2003). These findings have raised concerns that astronauts will face increased risk of operational task failure (Paloski and Oman, 2008). The results from the current study further support previous findings that there is a marked impairment in fine motor control due to spaceflight, including bimanual coordination. Moreover, these changes are evident up until 30 days post-flight. While the specific mechanisms underlying these manual motor control declines are unclear, previous work has reported an increase in skin sensitivity for fast skin receptors, and decreased sensitivity for slow receptors following spaceflight (Lowrey et al., 2014). This upweighting of tactile inputs may be adaptive inflight when the body is unloaded, but could potentially be maladaptive upon return to Earth, resulting in these transient manual motor performance declines.
Cognitive Measures
Cognitive declines with spaceflight have not conclusively been observed. Changes that have been reported include an increased ability to mentally rotate stimuli, and decreased ability to spatially orient letters in a word during early short duration spaceflight (Clement et al., 1987), reduced cognitive-motor dual-tasking ability (Manzey et al., 1995; Manzey and Lorenz, 1998; Bock et al., 2010), increases in risky behavior in a single subject case study (Garrett-Bakelman et al., 2019), and anecdotal reports of “space fog” (Clément et al., 2020). In the present study, we investigated a range of cognitive domains both from pre- to post-flight and while astronauts were aboard the ISS. The only significant change from pre- to post-flight that survived FDR correction was in the cube rotation response time, which showed a decrease in response time that is likely attributable to a practice effect. Astronauts performed the cube rotation task twice per test session aboard the ISS, once while free floating yet tethered to the laptop console and again while tethered with feet in loops on the “floor.” These two setups allowed us to identify whether somatosensory feedback associated with having the feet on the “floor” and performing the task in a “seated” posture provides spatial orientation cues to aid in mental rotation performance. There were no statistically significant differences between cube 1 (feet unattached) and cube 2 (feet attached), however, there were trend level effects of a faster response time on cube 2 (p = 0.093; Supplementary Figure 1). These results may be limited by our small sample size, but could potentially have operational relevance. This trend-level effect could reflect practice.
Mission Duration
A current focus in spaceflight research is understanding the effects of flight duration on the human brain and behavior. NASA is planning to return to the Moon with the Artemis program and Mars by the 2030’s. A round trip to Mars is estimated to be around 30 months, which is longer than any current astronaut has spent in space on any given mission. This makes it imperative to understand whether there is a “dose-dependent” effect of spaceflight stressors/hazards on human performance. In the current study, most astronauts had mission durations of approximately 6 months, but there was a range with some crewmembers spending nearly 12 months (ranging from 4 to 11 months in space). We included mission duration in our statistical models to investigate its effect, finding only an uncorrected decline in bimanual Purdue Pegboard Test completion time with longer flight duration. The lack of spaceflight duration effects on our results may suggest that there are little functional changes associated with mission duration; however, it has been shown recently that the magnitude of spaceflight-associated structural brain changes is directly related to mission duration. Hupfeld et al. (2020a) recently reported that astronauts who spent 1 year in space exhibited larger magnitude brain fluid shifts, greater right precentral gyrus gray matter volume and cortical thickness changes, greater supplementary motor area gray matter volume changes, and greater free water volume changes within the frontal pole. Six-month missions were shown to result in greater increases in cerebellar volume as compared to 12-month missions. Brain changes exhibited only partial recovery at 6 months post-flight (Hupfeld et al., 2020a). Work by our group and others have also reported persisting ventricular volume changes evident at 6 months and 1 year post-flight (Van Ombergen et al., 2019; Hupfeld et al., 2020a; Jillings et al., 2020; Kramer et al., 2020), and functional vestibular brain changes that required 3 months post-flight to recover (Hupfeld et al., 2021). It is important to consider these brain changes; it is possible that behavior has returned to pre-flight levels by 1 month post-flight without a concomitant return to pre-flight neural control patterns. That is, there may be a substitution of brain networks or compensation that is still taking place post-flight even when behavior has recovered (Rothi and Horner, 1983; Hupfeld et al., 2020b).
This study is one of the few to have collected longitudinal data from astronauts on the ISS, allowing us to directly examine the effects of initial and longer term microgravity exposure. One of the tasks measured during spaceflight required single and dual-tasking. Dual- tasking has been evaluated previously during spaceflight; results showed impairments in both cognitive and motor behaviors in long duration spaceflight missions (Manzey et al., 1995; Manzey and Lorenz, 1998), with dual-task costs greater in space than on Earth. Additionally, these impairments were greatest during early flight and stabilized after approximately 9 months in space. However, these two reports were single subject case studies. Bock et al. (2010) further investigated dual-tasking in microgravity with a larger cohort of 3 astronauts performing a tracking task while also performing one of four reaction time tasks. They found an overall increase in tracking error and reaction time under dual-task conditions. Here, we found no differences in dual-task costs upon arrival to the ISS (performance measured at approximately 30 days into the flight and compared to pre-flight), nor as flight duration increased (performance measured at approximately 90 and 180 days into the flight). This may be due to a difference in complexity of the cognitive and motor tasks, a difference in the underlying task mechanisms, or due to the larger sample evaluated here.
There are few countermeasures that have proved effective for mitigating spaceflight- associated performance declines. Astronauts perform about 2.5 h of exercise daily a mix of aerobic and anaerobic, in order to maintain muscle mass and bone density (English et al., 2019, 2020). This has been found to partially counteract some spaceflight-associated sensorimotor declines, yet exercise alone is not sufficient (Wood et al., 2011; English et al., 2020). HDBR studies have examined the effects of artificial gravity applied via centrifugation as a potential countermeasure for spaceflight-associated brain and behavioral changes (Frett et al., 2020). Recent data shows that artificial gravity does not prevent aerobic capacity declines during bed rest, although it does mitigate some muscular function decay (Kramer et al., 2021) and partially counteract upright balance (De Martino et al., 2021).
Limitations
One of the primary limitations of this study is the small number of female astronauts; of the fifteen participants, only four were female. This does not provide us with sufficient power to evaluate sex differences. Another limitation in this study is the time delay between landing and the initial post-flight data collection, as astronauts re-adapt to Earth’s gravity relatively quickly. We found that postural control returned to baseline levels within roughly 4 days post-flight. It is possible that some of our other measures respond in a similar manner; this would mean that, by post-flight day 4, we may have missed many spaceflight-related performance changes. Moreover, we did not have test sessions between post-flight days 4 and 30, limiting our ability to delineate post-flight rapid recovery curves.
Conclusion and Future Directions
In this study, we evaluated the effects of the microgravity environment on astronauts’ sensorimotor and cognitive performance with a range of behavioral measures collected before, during, and following missions to the ISS. We found marked decreases in balance, mobility and bimanual coordination following exposure to the microgravity environment. These declines are transient and return to baseline levels within roughly 30 days. Additionally, we identified a trend for increased cognitive performance on some measures when astronauts had their feet on the “floor” of the ISS, suggesting that additional orientation cues may increase spatial working memory ability in microgravity. In the same sample, we also collected functional MRI data during task performance before and following spaceflight as well as measures of brain structure (structural MRI and diffusion weighted MRI). In future analyses, we will examine brain changes and their relation to behavioral performance. It may be that, in cases where we do not see behavioral changes, the underlying networks engaged for task performance will have changed in a compensatory fashion due to spaceflight. Further analyses of our neuroimaging data in conjunction with these performance measures will give us insight into the adaptive or maladaptive effects of spaceflight.
Data Availability Statement
The raw data supporting the conclusions of this article will be made available by the authors, without undue reservation.
Ethics Statement
The studies involving human participants were reviewed and approved by NASA. The patients/participants provided their written informed consent to participate in this study.
Author Contributions
GT analyzed the behavioral data, created the figures and tables, and wrote the first draft of the manuscript. KH, HM, and AS assisted with processing of the data and preparation of the initial manuscript. YD and NB collected and analyzed the initial data. IK participated in project design and software development. SW, JB, AM, PR-L, and RS designed the project, secured funding and led the interpretation, and discussion of the results. All authors participated in revision of the manuscript.
Funding
This work was supported by grants from the National Aeronautics and Space Administration (NASA NNX11AR02G to RS, AM, SW, PR-L, and JB). During the completion of this work GT was supported by the University of Florida’s (UF) Graduate Student Funding Award. KH was supported by a National Science Foundation Graduate Research Fellowship under Grant Nos. DGE-1315138 and DGE-1842473, National Institute of Neurological Disorders and Stroke training grant T32-NS082128, and National Institute on Aging fellowship 1F99AG068440. HM was supported by a Natural Sciences and Engineering Research Council of Canada (NSERC) Postdoctoral Fellowship and a NASA Human Research Program Augmentation Grant.
Conflict of Interest
YD, NB, IK, and AM were employed by the company KBR, Inc.
The remaining authors declare that the research was conducted in the absence of any commercial or financial relationships that could be construed as a potential conflict of interest.
Publisher’s Note
All claims expressed in this article are solely those of the authors and do not necessarily represent those of their affiliated organizations, or those of the publisher, the editors and the reviewers. Any product that may be evaluated in this article, or claim that may be made by its manufacturer, is not guaranteed or endorsed by the publisher.
Acknowledgments
The authors would like to thank all of the astronauts who volunteered their time; without them this project would not have been possible.
Supplementary Material
The Supplementary Material for this article can be found online at: https://www.frontiersin.org/articles/10.3389/fncir.2021.723504/full#supplementary-material
References
Alperin, N., Bagci, A. M., and Lee, S. H. (2017). Spaceflight-induced changes in white matter hyperintensity burden in astronauts. Neurology 89, 2187–2191. doi: 10.1212/wnl.0000000000004475
Anguera, J. A., Reuter-Lorenz, P. A., Willingham, D. T., and Seidler, R. D. (2010). Contributions of spatial working memory to visuomotor learning. J. Cogn. Neurosci. 22, 1917–1930. doi: 10.1162/jocn.2009.21351
Anguera, J. A., Reuter-Lorenz, P. A., Willingham, D. T., and Seidler, R. D. (2011). Failure to engage spatial working memory contributes to age-related declines in visuomotor learning. J. Cogn. Neurosci. 23, 11–25. doi: 10.1162/jocn.2010.21451
Basner, M., Stahn, A. C., Nasrini, J., Dinges, D. F., Moore, T. M., Gur, R. C., et al. (2021). Effects of head-down tilt bed rest plus elevated CO2 on cognitive performance. J. Appl. Physiol. 130, 1235–1246. doi: 10.1152/japplphysiol.00865.2020
Benjamini, Y., and Hochberg, Y. (1995). Controlling the false discovery rate: a practical and powerful approach to multiple testing. J. R. Stat. Soc. Ser. B Stat. Methodol. 57, 289–300. doi: 10.1111/j.2517-6161.1995.tb02031.x
Black, F. O., Paloski, W. H., Doxey-Gasway, D. D., and Reschke, M. F. (1995). Vestibular plasticity following orbital spaceflight: recovery from postflight postural instability. Acta Oto Laryngol. Suppl. 520 Pt 2, 450–454. doi: 10.3109/00016489509125296
Black, F. O., Paloski, W. H., Reschke, M. F., Igarashi, M., Guedry, F., and Anderson, D. J. (1999). Disruption of postural readaptation by inertial stimuli following space flight. J. Vestib. Res. 9, 369–378. doi: 10.3233/ves-1999-9506
Bloomberg, J. J., Peters, B. T., Cohen, H. S., and Mulavara, A. P. (2015). Enhancing astronaut performance using sensorimotor adaptability training. Front. Syst. Neurosci. 9:129. doi: 10.3389/fnsys.2015.00129
Bloomberg, J. J., Peters, B. T., Smith, S. L., Huebner, W. P., and Reschke, M. F. (1997). Locomotor head-trunk coordination strategies following space flight. J. Vestib. Res. 7, 161–177. doi: 10.3233/ves-1997-72-307
Bock, O., Abeele, S., and Eversheim, U. (2003). Sensorimotor performance and computational demand during short-term exposure to microgravity. Aviat. Space Environ. Med. 74, 1256–1262.
Bock, O., Weigelt, C., and Bloomberg, J. J. (2010). Cognitive demand of human sensorimotor performance during an extended space mission: a dual-task study. Aviat. Space Environ. Med. 81, 819–824. doi: 10.3357/asem.2608.2010
Boyle, R., Mensinger, A. F., Yoshida, K., Usui, S., Intravaia, A., Tricas, T., et al. (2001). Neural readaptation to Earth’s gravity following return from space. J. Neurophysiol. 86, 2118–2122. doi: 10.1152/jn.2001.86.4.2118
Bryanov, I. I., Yemel’yanov, M. D., Matveyev, A. D., Mantsev, E. I., Tarasov, I. K., Yakovleva, I. K., et al. (1976). Space Flights In The Soyuz Spacecraft: Biomedical Research. Redwood City, CA: Kanner Associates.
Campbell, M. R., Williams, D. R., Buckey, J. C. Jr., and Kirkpatrick, A. W. (2005). Animal surgery during spaceflight on the Neurolab Shuttle mission. Aviat. Space Environ. Med. 76, 589–593.
Christou, A. I., Miall, R. C., McNab, F., and Galea, J. M. (2016). Individual differences in explicit and implicit visuomotor learning and working memory capacity. Sci. Rep. 6:36633.
Clarke, A. H., Grigull, J., Mueller, R., and Scherer, H. (2000). The three-dimensional vestibulo-ocular reflex during prolonged microgravity. Exp. Brain Res. 134, 322–334. doi: 10.1007/s002210000476
Clément, G. R., Boyle, R. D., George, K. A., Nelson, G. A., Reschke, M. F., Williams, T. J., et al. (2020). Challenges to the central nervous system during human spaceflight missions to Mars. J. Neurophysiol. 123, 2037–2063. doi: 10.1152/jn.00476.2019
Clement, G., Berthoz, A., and Lestienne, F. (1987). Adaptive changes in perception of body orientation and mental image rotation in microgravity. Aviat. Space Environ. Med. 58(9 Pt 2), A159–A163.
Clément, G., Loureiro, N., Sousa, D., and Zandvliet, A. (2016). Perception of egocentric distance during gravitational changes in parabolic flight. PLoS One 11:e0159422. doi: 10.1371/journal.pone.0159422
De Martino, E., Salomoni, S. E., Hodges, P. W., Hides, J., Lindsay, K., Debuse, D., et al. (2021). Intermittent short-arm centrifugation is a partially effective countermeasure against upright balance deteroiation following 60-day head-down tilt bed rest. J. Appl. Phys. 131, 689–701. doi: 10.1152/japplphysiol.00180.2021
Ekstrom, R. B., and Harman, H. H. (1976). Manual For Kit Of Factor-Referenced Cognitive Tests, 1976. Princeton, NJ: Educational testing service.
English, K. L., Bloomberg, J. J., Mulavara, A. P., and Ploutz-Snyder, L. L. (2019). Exercise countermeasures to neuromuscular deconditioning in spaceflight. Compr. Physiol. 10, 171–196. doi: 10.1002/cphy.c190005
English, K. L., Downs, M., Goetchius, E., Buxton, R. E., Ryder, J. W., Ploutz-Snyder, R. J., et al. (2020). High intensity training during spaceflight: results from the NASA Sprint Study. NPJ Microgravity 6:21.
Frett, T., Green, D. A., Mulder, E., Noppe, A., Arz, M., Pustowalow, W., et al. (2020). Tolerability of daily intermittent or continuous short-arm centrifugation during 60-day 6o head down bed rest (AGBRESA study). PLoS One 15:e0239228. doi: 10.1371/journal.pone.0239228
Garrett-Bakelman, F. E., Darshi, M., Green, S. J., Gur, R. C., Lin, L., Macias, B. R., et al. (2019). The NASA twins study: a multidimensional analysis of a year-long human spaceflight. Science 364:eaau8650.
Hallgren, E., Kornilova, L., Fransen, E., Glukhikh, D., Moore, S. T., Clément, G., et al. (2016). Decreased otolith-mediated vestibular response in 25 astronauts induced by long-duration spaceflight. J. Neurophysiol. 115, 3045–3051. doi: 10.1152/jn.00065.2016
Hupfeld, K. E., McGregor, H. R., Koppelmans, V., Beltran, N. E., Kofman, I. S., De Dios, Y. E., et al. (2021). Brain and behavioral evidence for reweighting of vestibular inputs with long-duration spaceflight. Cereb. Cortex bhab239. doi: 10.1093/cercor/bhab239 [Epub ahead of print].
Hupfeld, K. E., McGregor, H. R., Lee, J. K., Beltran, N. E., Kofman, I. S., De Dios, Y. E., et al. (2020a). The impact of 6 and 12 months in space on human brain structure and intracranial fluid shifts. Cereb. Cortex Commun. 1:tgaa023.
Hupfeld, K. E., McGregor, H. R., Reuter-Lorenz, P. A., and Seidler, R. D. (2020b). Microgravity effects on the human brain and behavior: dysfunction and adaptive plasticity. Neurosci. Biobehav. Rev. 122, 176–189. doi: 10.1016/j.neubiorev.2020.11.017
Ives, A. R. (2015). For testing the significance of regression coefficients, go ahead and log-transform count data. Methods Ecol. Evol. 6, 828–835. doi: 10.1111/2041-210X.12386
Jillings, S., Van Ombergen, A., Tomilovskaya, E., Rumshiskaya, A., Litvinova, L., Nosikova, I., et al. (2020). Macro- and microstructural changes in cosmonauts’ brains after long-duration spaceflight. Sci. Adv. 6:eaaz9488. doi: 10.1126/sciadv.aaz9488
Koppelmans, V., Bloomberg, J. J., De Dios, Y. E., Wood, S. J., Reuter-Lorenz, P. A., Kofman, I. S., et al. (2017). Brain plasticity and sensorimotor deterioration as a function of 70 days head down tilt bed rest. PLoS One 12:e0182236. doi: 10.1371/journal.pone.0182236
Koppelmans, V., Erdeniz, B., De Dios, Y. E., Wood, S. J., Reuter-Lorenz, P. A., Kofman, I., et al. (2013). Study protocol to examine the effects of spaceflight and a spaceflight analog on neurocognitive performance: extent, longevity, and neural bases. BMC Neurol. 13:205. doi: 10.1186/1471-2377-13-205
Kornilova, L. N., Naumov, I. A., Azarov, K. A., and Sagalovitch, V. N. (2012). Gaze control and vestibular-cervical-ocular responses after prolonged exposure to microgravity. Aviat. Space Environ. Med. 83, 1123–1134. doi: 10.3357/asem.3106.2012
Kramer, A., Venegas-Carro, M., Mulder, E., Lee, J. K., Moreno-Villanueva, M., Bürkle, A., et al. (2020). Cardiorespiratory and neuromuscular demand of daily centrifugation: results from the 60-day AGBRESA bed rest study. Front. Physiol. 11:562377. doi: 10.3389/fphys.2020.562377
Kramer, A., Venegas-Carro, M., Zange, J., Sies, W., Maffiuletti, N. A., Gruber, M., et al. (2021) Daily 30-min exposure to artificial gravity during 60 days of bed rest does not maintain aerobic exercise capacity but mitigates some deteriorations of muscle function: results from the AGBRESA RCT. Eur. J. Appl. Physiol. 121, 2015–2026. doi: 10.1007/s00421-021-04673-w
Layne, C. S., Lange, G. W., Pruett, C. J., McDonald, P. V., Merkle, L. A., Mulavara, A. P., et al. (1998). Adaptation of neuromuscular activation patterns during treadmill walking after long-duration space flight. Acta Astronaut. 43, 107–119. doi: 10.1016/s0094-5765(98)00148-9
Layne, C. S., McDonald, P. V., and Bloomberg, J. J. (1997). Neuromuscular activation patterns during treadmill walking after space flight. Exp. Cereb. 113, 104–116. doi: 10.1007/bf02454146
Lee, J. K., De Dios, Y., Kofman, I., Mulavara, A. P., Bloomberg, J. J., and Seidler, R. D. (2019). Head down tilt bed rest plus elevated CO2 as a spaceflight analog: effects on cognitive and sensorimotor performance. Front. Hum. Neurosci. 13:355. doi: 10.3389/fnhum.2019.00355
Lowrey, C. R., Perry, S. D., Strzalkowski, N. D. J., Williams, D. R., Wood, S. J., and Bent, L. R. (2014). Selective skin sensitivity changes and sensory reweighting following short-duration space flight. J. Appl. Physiol. 116, 683–692. doi: 10.1152/japplphysiol.01200.2013
Mahadevan, A. D., Hupfeld, K. E., Lee, J. K., De Dios, Y. E., Kofman, I. S., Beltran, N. E., et al. (2021). Head-down-tilt bed rest with elevated CO2-effects of a pilot spaceflight analogue on neural function and performance during a cognitive-motor dual task. Front. Physiol. 12:1200. doi: 10.3389/fphys.2021.654906
Manzey, D., and Lorenz, B. (1998). Mental performance during short-term and long-term spaceflight. Brain Res. Brain Res. Rev. 28, 215–221. doi: 10.1016/s0165-0173(98)00041-1
Manzey, D., Lorenz, B., Schiewe, A., Finell, G., and Thiele, G. (1995). Dual-task performance in space: results from a single-case study during a short-term space mission. Hum. Factors 37, 667–681. doi: 10.1518/001872095778995599
McDonald, P. V., Basdogan, C., Bloomberg, J. J., and Layne, C. S. (1996). Lower limb kinematics during treadmill walking after space flight: implications for gaze stabilization. Experimental brain research. Exp. Hirnforschung Exp. Cereb. 112, 325–334.
McDougle, S. D., Bond, K. M., and Taylor, J. A. (2015). Explicit and implicit processes constitute the fast and slow processes of sensorimotor learning. J. Neurosci. 35, 9568–9579. doi: 10.1523/jneurosci.5061-14.2015
Miller, C. A., Kofman, I. S., Brady, R. R., May-Phillips, T. R., Batson, C. D., Lawrence, E. L., et al. (2018). Functional task and balance performance in bed rest subjects and astronauts. Aerosp. Med. Hum. Perform. 89, 805–815. doi: 10.3357/amhp.5039.2018
Moore, S. T., Dilda, V., Morris, T. R., Yungher, D. A., MacDougall, H. G., and Wood, S. J. (2019). Long-duration spaceflight adversely affects post-landing operator proficiency. Sci. Rep. 9, 1–14. doi: 10.1038/s41598-019-39058-9
Mulavara, A. P., Feiveson, A. H., Fiedler, J., Cohen, H., Peters, B. T., Miller, C., et al. (2010). Locomotor function after long-duration space flight: effects and motor learning during recovery. Exp. Brain Res. Exp. Hirnforschung Exp. Cereb. 202, 649–659. doi: 10.1007/s00221-010-2171-0
Mulavara, A. P., Peters, B. T., Miller, C. A., Kofman, I. S., Reschke, M. F., Taylor, L. C., et al. (2018). Physiological and functional alterations after spaceflight and bed rest. Med. Sci. Sports Exerc. 50, 1961–1980. doi: 10.1249/mss.0000000000001615
Nashner, L. M. (1972). Vestibular postural control model. Kybernetik 10, 106–110. doi: 10.1007/bf00292236
Newman, D. J., Jackson, D. K., and Bloomberg, J. J. (1997). Altered astronaut lower limb and mass center kinematics in downward jumping following space flight. Exp. Brain Res. Exp. Hirnforschung Exp. Cereb. 117, 30–42. doi: 10.1007/pl00005788
Ozdemir, R. A., Goel, R., Reschke, M. F., Wood, S. J., and Paloski, W. H. (2018). Critical role of somatosensation in postural control following spaceflight: vestibularly deficient astronauts are not able to maintain upright stance during compromised somatosensation. Front. Physiol. 9:1680. doi: 10.3389/fphys.2018.01680
Paloski, W. H., and Oman, C. M. (2008). Risk of sensory-motor performance failures affecting vehicle control during space missions: a review of the evidence. J. Grav. Physiol. 15, 1–29.
Paloski, W. H., Bloomberg, J. J., Reschke, M. F., and Harm, D. L. (1994). Spaceflight-induced changes in posture and locomotion. J. Biomech. 27:812. doi: 10.1016/0021-9290(94)91366-8
Paloski, W. H., Reschke, M. F., Black, F. O., Doxey, D. D., and Harm, D. L. (1992). Recovery of postural equilibrium control following spaceflight. Ann. N. Y. Acad. Sci. 656, 747–754. doi: 10.1111/j.1749-6632.1992.tb25253.x
Pinheiro, J., Bates, D., DebRoy, S., Sarkar, D., and R Core Team (2021). nlme: Linear and Nonlinear Mixed Effects Models. R Package Version 3.1-153. Available online at: https://CRAN.R-project.org/package=nlme
R Core Team (2019). R: A Language And Environment For Statistical Computing. Vienna: R Foundation for Statistical Computing.
Rafiq, A., Hummel, R., Lavrentyev, V., Derry, W., Williams, D., and Merrell, R. C. (2006). Microgravity effects on fine motor skills: tying surgical knots during parabolic flight. Aviat. Space Environ. Med. 77, 852–856.
Reschke, M. F., Bloomberg, J. J., Harm, D. L., and Paloski, W. H. (1994a). Space flight and neurovestibular adaptation. J. Clin. Pharmacol. 34, 609–617. doi: 10.1002/j.1552-4604.1994.tb02014.x
Reschke, M. F., Bloomberg, J. J., Harm, D. L., Paloski, W. H., Layne, C., and McDonald, V. (1998). Posture, locomotion, spatial orientation, and motion sickness as a function of space flight. Brain Res. Brain Res. Rev. 28, 102–117. doi: 10.1016/s0165-0173(98)00031-9
Reschke, M. F., Bloomberg, J. J., Paloski, W. H., Harm, D. L., and Parker, D. E. (1994b). “Neurophysiologic aspects: sensory and sensorimotor function,” in Space Physiology And Medicine, eds A. E. Nicogossian, C. L. Huntoon, and S. L. Pool (Philadelphia, PA: Lea & Febiger).
Reschke, M. F., Bloomberg, J. J., Paloski, W. H., Mulavara, A. P., Feiveson, A. H., and Harm, D. L. (2009). Postural reflexes, balance control, and functional mobility with long-duration head-down bed rest. Aviat. Space Environ. Med. 80(5 Suppl), A45–A54.
Reschke, M. F., Cohen, H. S., Cerisano, J. M., Clayton, J. A., Cromwell, R., Danielson, R. W., et al. (2014). Effects of sex and gender on adaptation to space: neurosensory systems. J. Women Health 23, 959–962. doi: 10.1089/jwh.2014.4908
Reschke, M. F., Kolev, O. I., and Clément, G. (2017). Eye-head coordination in 31 space shuttle astronauts during visual target acquisition. Sci. Rep. 7:14283. doi: 10.1038/s41598-017-14752-8
Roberts, D. R., Zhu, X., Tabesh, A., Duffy, E. W., Ramsey, D. A., and Brown, T. R. (2015). Structural brain changes following long-term 6 head-down tilt bed rest as an analog for spaceflight. AJNR Am. J. Neuroradiol. 36, 2048–2054. doi: 10.3174/ajnr.a4406
Rothi, L. J., and Horner, J. (1983). Restitution and substitution: two theories of recovery with application to neurobehavioral treatment. J. Clin. Neuropsychol. 5, 73–81. doi: 10.1080/01688638308401152
Salazar, A. P., Hupfeld, K. E., Lee, J. K., Beltran, N. E., Kofman, I. S., De Dios, Y. E., et al. (2020). Neural working memory changes during a spaceflight analog with elevated carbon dioxide: a pilot study. Front. Syst. Neurosci. 14:48. doi: 10.3389/fnsys.2020.00048
Seidler, R. D., Bernard, J. A., Burutolu, T. B., Fling, B. W., Gordon, M. T., Gwin, J. T., et al. (2010). Motor control and aging: links to age-related brain structural, functional, and biochemical effects. Neurosci. Biobehav. Rev. 34, 721–733. doi: 10.1016/j.neubiorev.2009.10.005
Shepard, S., and Metzler, D. (1988). Mental rotation: effects of dimensionality of objects and type of task. J. Exp. Psychol. Hum. Percept. Perform. 14, 3–11. doi: 10.1037/0096-1523.14.1.3
Smith, M. G., Kelley, M., and Basner, M. (2020). A brief history of spaceflight from 1961 to 2020: an analysis of missions and astronaut demographics. Acta Astronaut. 175, 290–299. doi: 10.1016/j.actaastro.2020.06.004
Stahn, A. C., Gunga, H.-C., Kohlberg, E., Gallinat, J., Dinges, D. F., and Kühn, S. (2019). Brain changes in response to long antarctic expeditions. N. Engl. J. Med. 381, 2273–2275.
Strangman, G. E., Sipes, W., and Beven, G. (2014). Human cognitive performance in spaceflight and analogue environments. Aviat. Space Environ. Med. 85, 1033–1048.
Taylor, J. A., and Ivry, R. B. (2013). “Implicit and explicit processes in motor learning,” in Action Science, eds W. Prinz, M. Beisert, and A. Herwug (Cambridge, MA: MIT Press), 63–87.
Thornton, W. E., and Rummel, J. A. (1977). NASA Technical Reports Server (NTRS). NTRS.NASA.GOV. Available online at: https://ntrs.nasa.gov/search.jsp?R=19770026857 (accessed April 4, 2021).
Tiffin, J., and Asher, E. J. (1948). The Purdue pegboard; norms and studies of reliability and validity. The Journal of Appl. Psychol. 32, 234–247.
Tombu, M., and Jolicoeur, P. (2003). A central capacity sharing model of dual-task performance. J. Exp. Psychol. Hum. Percept. Perform. 29, 3–18.
Van Ombergen, A., Jillings, S., Jeurissen, B., Tomilovskaya, E., Rumshiskaya, A., Litvinova, L., et al. (2019). Brain ventricular volume changes induced by long-duration spaceflight. Proc. Natl. Acad. Sci. U.S.A. 116, 10531–10536.
Weschler, D. (1986). Wechsler Adult Intelligence Scale–Revised UK Edition. San Antonio, TX: The Psychological Corporation.
Witkin, H. A., and Asch, S. E. (1948). Studies in space orientation; perception of the upright in the absence of a visual field. J. Exp. Psychol. 38, 603–614. doi: 10.1037/h0055372
Wood, S. J., Loehr, J. A., and Guilliams, M. E. (2011). Sensorimotor reconditioning during and after spaceflight. NeuroRehabilitation 29, 185–195. doi: 10.3233/NRE-2011-0694
Wood, S. J., Paloski, W. H., and Clark, J. B. (2015). Assessing sensorimotor function following iss with computerized dynamic posturography. Aerosp. Med. Hum. Perform. 86(12 Suppl), A45–A53. doi: 10.3357/AMHP.EC07.2015
Wood, S. J., Reschke, M. F., and Owen Black, F. (2012). Continuous equilibrium scores: factoring in the time before a fall. Gait Posture 36, 487–489. doi: 10.1016/j.gaitpost.2012.04.014
Keywords: spaceflight, balance, mobility, cognition, sensorimotor, microgravity
Citation: Tays GD, Hupfeld KE, McGregor HR, Salazar AP, De Dios YE, Beltran NE, Reuter-Lorenz PA, Kofman IS, Wood SJ, Bloomberg JJ, Mulavara AP and Seidler RD (2021) The Effects of Long Duration Spaceflight on Sensorimotor Control and Cognition. Front. Neural Circuits 15:723504. doi: 10.3389/fncir.2021.723504
Received: 10 June 2021; Accepted: 22 September 2021;
Published: 26 October 2021.
Edited by:
Raffaella Ricci, University of Turin, ItalyReviewed by:
Rodrigo Suárez, The University of Queensland, AustraliaAnna Maria Berti, University of Turin, Italy
Copyright © 2021 Tays, Hupfeld, McGregor, Salazar, De Dios, Beltran, Reuter-Lorenz, Kofman, Wood, Bloomberg, Mulavara and Seidler. This is an open-access article distributed under the terms of the Creative Commons Attribution License (CC BY). The use, distribution or reproduction in other forums is permitted, provided the original author(s) and the copyright owner(s) are credited and that the original publication in this journal is cited, in accordance with accepted academic practice. No use, distribution or reproduction is permitted which does not comply with these terms.
*Correspondence: Rachael D. Seidler, cmFjaGFlbHNlaWRsZXJAdWZsLmVkdQ==