- 1Department of Organisms and Ecosystems Research, National Institute of Biology, Ljubljana, Slovenia
- 2Jovan Hadži Institute of Biology, Research Centre of the Slovenian Academy of Sciences and Arts, Ljubljana, Slovenia
- 3University of Ljubljana, Ljubljana, Slovenia
- 4Department of Entomology, National Museum of Natural History, Smithsonian Institution, Washington, DC, United States
- 5Centre for Behavioural Ecology and Evolution, College of Life Sciences, Hubei University, Wuhan, Hubei, China
This perspective identifies the grand challenges in arachnid science: 1. Grasp the arachnid species diversity. There is a need to accelerate taxonomic research to obtain a sense of arachnid species diversity, however, at the same time, taxonomy needs to increase its quality, rigor, and repeatability. 2. Standardize arachnid systematics research. A solid phylogenetic definition and morphological diagnosis of Arachnida and its composing subgroups, usually treated at the rank of order, are needed. Studies should aim to stabilize and standardize phylogenetic efforts at all levels of hierarchy, and systematists should adopt criteria for higher level ranks in arachnid classification. 3. Interpret arachnid trait evolution through omics approaches. Among the field’s grand challenges is to define the genetic diversity encoding for the diverse arachnid traits, including developmental, morphological and ecological characteristics, biomaterials such as silks, venoms, digestive fluids, or allergens and bioproducts that cause diseases. Comparative genomics, transcriptomics, and proteomics will provide the empirical basis for biotechnology to modify arachnid genomes to fit numerous applications. 4. Facilitate biotechnological applications of arachnid molecules and biomaterials. Among the grand field challenges is to define potential applications of arachnid bioproducts from therapeutics to industry. New natural and biodegradable products, e.g. from spider silks, should ease our burden on ecosystems. 5. Utilize arachnids as models in ecological and biogeographic research. Biodiversity inventory sampling and analytical techniques should be extended from spiders to other arachnid groups. Spiders and their webs could be used as environmental DNA samplers, measuring or monitoring ecosystems’ overall biodiversity. Arachnids are excellent models to address biogeographical questions at the global to local scales. 6. Disentangle evolutionary drivers of arachnid diversity. Among the field grand challenges is a more precise evaluation to what extent the emergence of arachnid phenotypes is shaped by classical selection processes, and under what conditions, if any, sexual conflict needs to be invoked. 7. Define effective conservation measures for arachnids in the light of global changes. Effective conservation measures in arachnology should integrate the data from phylogenetic diversity, physiology, ecology, biogeography, and global change biology.
Introduction
Arachnids are an incredibly diverse, ancient lineage of chelicerate arthropods. The phylogenetic limits of Arachnida have yet to be precisely established (Packard, 1882; Harvey, 2002; Coddington et al., 2004; Sharma et al., 2021), but Arachnida certainly contains groups of terrestrial and aquatic chelicerates including spiders (Araneae), harvestmen (Opiliones), scorpions (Scorpiones), mites (Acariformes), ticks (Parasitiformes), pseudoscorpions (Pseudoscorpiones), camel spiders (Solifugae), whip spiders (Amblypygi), vinegaroons (Thelyphonida, also Uropygi), shorttailed whipscorpions (Schizomida), microwhip scorpions (Palpigradi), and hooded tick-spiders (Ricinulei). Arachnids also include several extinct lineages of varying ranks (Wang et al., 2018), some of them marine, and may also include the marine horseshoe crabs (Xiphosura) (Ban et al., 2022). Together, arachnid lineages comprise at least hundreds of thousands, perhaps even millions of species, of which most remain undiscovered.
Arachnids contain representatives that are terrestrial and aquatic, carnivorous and herbivorous, specialized and generalist, tiny-bodied and large, sexually monomorphic and dimorphic. A selected few arachnid species are medically important, examples being venomous scorpions and spiders (Lüddecke et al., 2022), parasitic mites that transmit scrub typhus and cause pruritic dermatitis (e.g., trombiculiasis), and ticks that are vectors of debilitating, even fatal pathogens (e.g., tick-borne encephalitis virus (TBEV), Anaplasma spp., Borrelia spp., and Babesia spp.) (Knap et al., 2009; de la Fuente et al., 2017). Some arachnids are also considered as agricultural pests (Cornman et al., 2010; Grbić et al., 2011). Most arachnid species, of course, are neutral to humans, and constitute thousands of unique biological forms that are ecologically important within known and unknown networks. Others may hold potentially beneficial biological secrets yet to be uncovered. All of them are simply beautiful products of evolution over hundreds of millions of years.
Given the enormous diversity of arachnids, their ubiquity in most ecosystems, the recent developments in biological sciences, and the biodiversity declines in the era of habitat destruction and global climate changes, I hereby identify the seven grand challenges in the field of arachnid science.
Grand challenge 1: Grasp the arachnid species diversity
The arachnid species diversity is overwhelming, with taxonomists having only discovered and described a small portion. For example, while about 50 thousand spider species are catalogued (WSC, 2022), the true spider species diversity is estimated to exceed 120 thousand (Agnarsson et al., 2013). Although arachnids also contain some species-depauperate lineages such as Uraraneida, Palpigradi, Thelyphonida, Schizomida and Ricinulei, whose species diversity will not exceed tens or hundreds, the taxonomic incompleteness noted above in spiders may be even more extreme in other arachnids. About 10 thousand species of oribatid mites are described, yet estimates of their true diversity range from 50 to 100 thousand (Schatz and Behan-Pelletier, 2008). By 2010, taxonomy had catalogued roughly 92 thousand extant arachnid species (Adis and Harvey, 2000) and today this number exceeds 110 thousand. However, if the above established measures of taxonomic completeness (42% in spiders and perhaps less than 10% in Acari) are typical of all arachnids, then, by extrapolation, the true species diversity of Arachnida must lie between 262 thousand and 1.1 million. Other approximations are even higher: estimates for species richness in Acari alone are 1 to 1.5 million (Krantz and Walter, 2009; Walter and Proctor, 2013). Arachnids are a truly mega-diverse lineage.
With only a fraction of the true arachnid diversity catalogued and considering the rate of habitat destruction worldwide, taxonomy is obviously not going to detect all species in our lifetimes. Taxonomic efforts need to be dramatically accelerated, and immediately (Agnarsson and Kuntner, 2007). Rapid species discovery now uses massive sampling and DNA barcoding (Cao et al., 2016). However, accelerating dissemination should not be achieved at the expense of taxonomy’s rigor. Arachnological taxonomic practices have recently been scrutinized with some focus on spiders (Bond et al., 2021) and the results are not encouraging with much left to be desired in terms of integrating data sources, data availability, and repeatability. These authors called for higher taxonomic standards though specific recommendations: i) All taxonomic works should clearly state the species concept being applied; ii) All data should be made electronically accessible; iii) Descriptions of new species based on singleton specimen data that are described outside a clear context should be discouraged; and iv) Taxonomy should become integrative (Bond et al., 2021). These recommendations, and the general calls for the importance of taxonomy, should be adopted by the arachnological community in order to provide scientific rigor in untangling species diversity.
Grand challenge 2: Standardize arachnid systematics research
Phylogenetic progress in arachnids has shifted from predominantly morphological cladistics in the 1990s, through targeted use of a selected few genes during the first two decades of the century, towards the use of subgenomic and transcriptomic data in the past years (Agnarsson et al., 2013; Bond et al., 2014; Fernández et al., 2014; Fernández and Giribet, 2015; Starrett et al., 2017; van Dam et al., 2019). Even though arachnology has decisively ventured into the genomic era, many regions of the arachnid tree of life remain unresolved and the field still lacks a consensus on the phylogenetic definition of many higher taxa.
Take the limits of Arachnida as an example, and the results are conflicting (Sharma et al., 2021). While morphological evidence for arachnid monophyly (i.e. Arachnida traditionally excluding Xiphosura) has once been deemed solid (Shultz, 2001; Coddington et al., 2004), most molecular studies do not recover it (Ballesteros et al., 2022; Ban et al., 2022), nor do they recover some of the classical groups within Arachnida (Ballesteros et al., 2019; Ballesteros and Sharma, 2019). Monophyly and phylogenetic placement of several arachnid lineages continue to be ambiguous due to long branch attraction artifacts (Ontano et al., 2022). Additionally, a divide seems to persist between students of fossils and morphologies on the one hand and the proponents of genomics on the other, hampering a total evidence resolution of the problem.
Intermediate level phylogenies at, say, family and genus levels, have likewise failed to stabilize despite considerable phylogenetic efforts in capturing more and more taxa and data (Hormiga and Griswold, 2014). Clearly, better datasets are paramount, but what should one emphasize given the limited data generation and computational capability? Genome scale phylogenetic analyses are possible and could be the systematists’ ultimate goal, but may not yet be feasible in multidiverse non-model lineages such as arachnids due to taxon sampling constraints. Thus, immediate phylogenetic efforts should focus on reduced representation sequencing, e.g. anchored hybrid enrichment (Lemmon et al., 2012) or the ultraconserved elements (Faircloth et al., 2012). An increasing number of studies on arachnids are demonstrating that these techniques are able to resolve both deep and shallow divergencies (Hamilton et al., 2016; Maddison et al., 2017; Starrett et al., 2017; Kuntner et al., 2019; Kulkarni et al., 2020; Xu et al., 2021; Chamberland et al., 2022; Li et al., 2022). These data should continue to be accumulated with the ultimate goal of openness and compatibility of datasets.
Phylogenetic instability aside, there is also a need for consensual classifications that would provide nomenclatural stability. Because the International Code of Zoological Nomenclature does not mandate the ranks above the genus level, systematic practice should adopt clear classification criteria, examples being monophyly, information content, diagnosability, stability, and lineage age (Kuntner et al., 2019). Arachnids are an old phylogenetic entity with numerous clades of widely varying ages and compositions, but this fact should not preclude systematists from creating order from chaos.
Grand challenge 3: Interpret arachnid trait evolution through omics approaches
Since 2010, when the first arachnid genome was published, 34 additional genomes have been sequenced and assembled (Table 1; Google Scholar search on 17 October 2022), and this pace is accelerating exponentially (Figure 1). Recent analyses demonstrate the power of large-scale comparative genomics in uncovering the links between the genotype and phenotype in arthropods (Thomas et al., 2020).
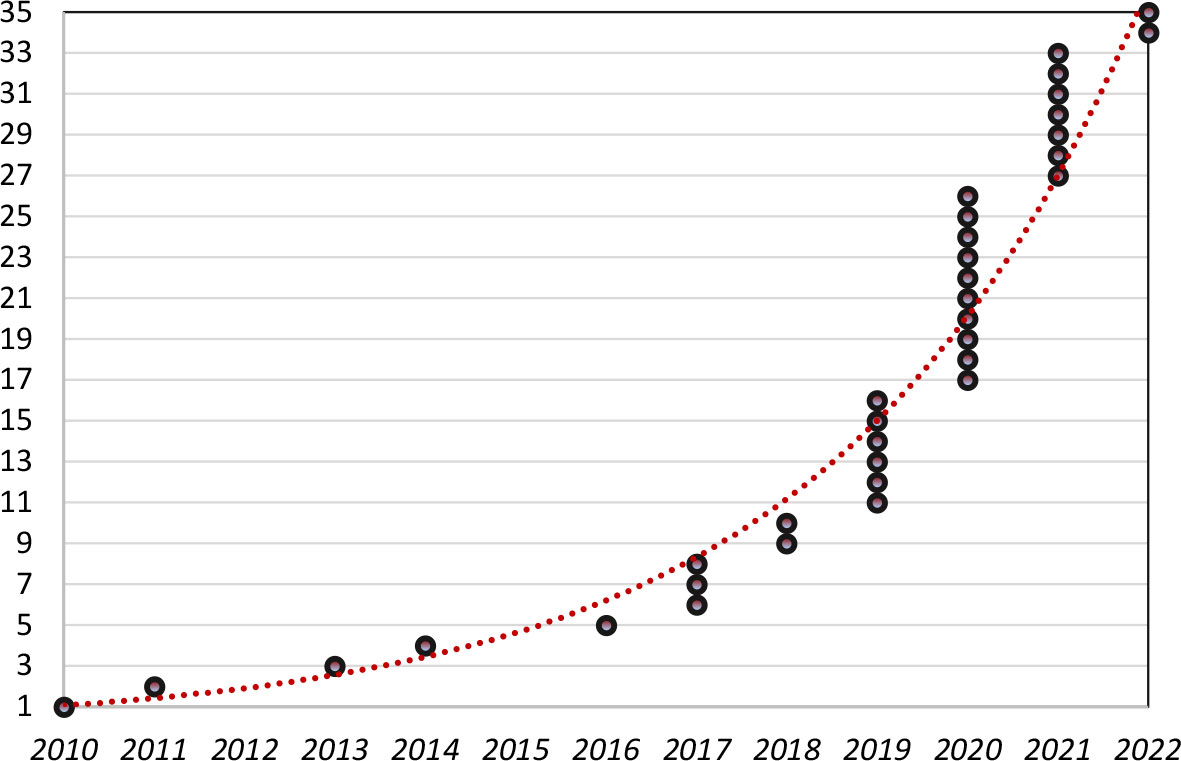
Figure 1 Cumulative numbers of arachnid genomes per year of publication (Table 1).
Comparative arachnid genomics is just taking flight given the diversity of arachnid karyotypes (Král et al., 2019) and genome sizes [Table 1; see also (Cerca et al., 2021)]: these range from the smallest known arthropod genome at 90 megabases (Mbp) in the mite Tetranychus urticae (Grbić et al., 2011) to a sizeable 4.29 gigabases (Gb) in the velvet spider Stegodyphus dumicola (Liu et al., 2019). The currently limited number of arachnid genomes reveal high rates of methylation in spiders and scorpions, perhaps suggesting epigenetic control of large portions of arachnid genes (Thomas et al., 2020). Current evidence furthermore points towards rampant gene duplication and loss in arachnids, with at least one whole genome duplication event at the branch subtending Arachnopulmonata, a clade that unites spiders and amblypygids (Schwager et al., 2017; Harper et al., 2021) as well as additional whole genome duplications in horseshoe crabs (Shingate et al., 2020; Nong et al., 2021). Nuclear genome sequencing in ticks has revealed expansions of gene families associated with vector-host interactions (Gulia-Nuss et al., 2016). Evidence has been found for ancient lateral gene transfer events to arachnids from bacteria or fungi (Dong et al., 2018). Genomic and transcriptomic studies have so far focused on annotating gene families that encode for morphological development (Hoy et al., 2016; Zhang et al., 2019; Harper et al., 2021), for venom neurotoxins (Cao et al., 2013; Yu et al., 2019), for silks (Babb et al., 2017; Garb et al., 2019; Kono et al., 2021; Babb et al., 2022), for dietary characteristics (Grbić et al., 2011; Hoy et al., 2016; Fan et al., 2021), for enzymes that cause allergies in vertebrates (Dong et al., 2018), for candidate genes for sex determination (Hoy et al., 2016; Miller et al., 2022), and for genes important for the innate immune system (Shingate et al., 2020). Large scale comparative genomics has also started to understand the genetic basis for tick-borne diseases (Jia et al., 2020).
Rapid genomic progress in the recent years as depicted in Figure 1 predicts hundreds and thousands of newly sequenced arachnid genomes in the coming years and decades, respectively. The new genomic data will facilitate more accurate interpretations of genetic architectures of arachnid traits and augment our understanding of the genetics of extreme arachnid diversity, ecological specialization, parasitism, disease transmission, metabolism, and biomaterials (Garb et al., 2018). Comparative genomics in itself, however, will not suffice to understand trait evolution. For this, a systems biology approach combining genomics, transcriptomics, and proteomics is best (Wilson and Daly, 2018; Mans, 2020). Such omics insights, combined with species interactions and ecology, will pave the way to discover novel biotechnological, biomedical, and pest control applications of arachnid bioproducts, such as venoms, silks, and allergy causing biomolecules.
Grand challenge 4: Facilitate biotechnological applications of arachnid molecules and biomaterials
Arachnids produce biomolecules and biomaterials that show applied potential in industry and biomedicine. Tick saliva, for example, contains proteins, peptides, lipid derivatives, and non-coding RNAs, that combined act to inhibit vertebrate immune reactions (Chmelař et al., 2019; Aounallah et al., 2020). These bioproducts could be genetically modified to fit numerous therapeutic requirements, perhaps as drugs for human immune diseases. Other arachnid biomolecules show antimicrobial potential (Leannec-Rialland et al., 2021).
Silks are natural materials that have independently evolved multiple times in arthropods (Craig, 1997). Most research has focused on understanding and exploiting spider silks. All spiders have abdominal silk glands (up to seven types in a single species) that produce silks for different uses (Sethy and Ahi, 2022). The glands connect with the spinnerets where a biologically unique spinning process solidifies the silken gland products (Vollrath and Knight, 2001). Dragline silk is used by all spiders—even those that do not spin prey-capture webs—, as a safety line. Spun out of the major ampullate glands (Ayoub et al., 2007), it is the toughest silk type known (Agnarsson et al., 2010; Blackledge et al., 2011) and therefore forms those architectural elements of orb webs that absorb the kinetic energy of impacting prey. Orbweaving spiders have also evolved glands specific for other silks (Vollrath and Knight, 2001; Lewis, 2006) such as minor ampullate glands that produce auxiliary spirals, flagelliform silk glands that produce extremely stretchy capture spirals, piriform silk glands that produce cement silk, aciniform silk glands that produce attack wrapping and eggsac insulation silk, tubuliform and cylindrical silk glands that produce silks for eggsac coating, and finally, aggregate silk glands that produce viscous glue (Sethy and Ahi, 2022).
Classical knowledge suggested a one-to-one correspondence between the seven silk glands in spiders and the different genes that encode spider silk proteins (spidroins). Genomic studies have already refuted this simplistic presumption. Babb et al. (2017) annotated 28 spidroin genes in Trichonephila clavipes and found their expression in several silk glands to be complex, as well as sex-specific (Correa-Garhwal et al., 2021). The genomes and transcriptomes of bark spiders (genus Caerostris) reveal a repertoire of no fewer than 31 spidroins (Garb et al., 2019; Kono et al., 2021; Babb et al., 2022). In comparison, studies of the genomes of other spiders have annotated fewer spidroins: 11 in Araneus ventricosus (Kono et al., 2019), 10 in Uloborus diversus (Miller et al., 2022), 16 in a non-web-building wolf spider (Yu et al., 2019).
Beyond spiders, other arachnids can produce silks. The silk fibers of the spider mite Tetranychus urticae, for example, have diameters on the nanometer scale, representing the thinnest natural silk fiber produced by arthropods (Lozano-Pérez et al., 2020). Combined with a Young’s modulus almost double that of spider Trichonephila clavipes silk, the spider mite silks show great potential in pharmacological and biomedical application (Lozano-Pérez et al., 2020).
Understanding the genetic basis for arachnid silk material properties over a larger taxonomic breath is essential for genetic modification and biotechnological use of silks. In fact, this grand challenge has already begun in a study that sequenced over a thousand spider species over their phylogenetic breath in order to map their spidroins and link their genetics with silk properties (Arakawa et al., 2022). This study launched the Spider Silkome Database (https://spider-silkome.org/), a new, vast resource that could help to identify applications for silks of a particular species, gland, and sex. The field is moving towards the possibility of genetic modification of silks of desired properties.
Applicative potentials of genetically modified arachnid silks are numerous. Being biodegradable and purportedly neutral to the human immune system, silks could encapsulate drugs and deliver them to targeted tissues (Leal-Egaña and Scheibel, 2010; Omenetto and Kaplan, 2010). Further potential medical applications are self-absorbing wound stitches (Omenetto and Kaplan, 2010), scaffolding for tissue engineering, and artificial nerve construction (Schacht and Scheibel, 2014). Electric conductivity, antibiotic, and insecticidal properties are among the least understood silk properties with great potential (Marques et al., 2004; Salles et al., 2006; Ortega-Jimenez and Dudley, 2013). Finally, being tough yet light, synthetic silks could replace nylon and rubber materials in the industries, and perhaps replace most plastic products thereby lowering humanity’s burden on ecosystems.
Grand challenge 5: Utilize arachnids as models in ecological and biogeographic research
Biodiversity inventories routinely sample arachnids. Works focusing on spider communities in tropical and temperate ecosystems have fine-tuned the sampling and the analytical protocols (Coddington et al., 1996; Scharff et al., 2003; Cardoso, 2009; Cardoso et al., 2009; Malumbres-Olarte et al., 2016), and explained that rare species in tropical inventories are artefacts of undersampling (Coddington et al., 2009). These techniques should be extended to other arachnid groups such as mites, ticks, scorpions, and harvestmen for a bigger picture of arachnid alpha and beta diversity.
The ease of sequencing has brought forth protocols to define food webs from predator gut contents, and arachnids are excellent model predators for such ecological application (Krehenwinkel et al., 2017). Less invasive sampling techniques are also being developed, one example being spider webs that can potentially monitor overall biotic diversity in ecosystems. Functioning as an extended phenotype targeting specific or nonspecific prey (Blackledge et al., 2011; Blamires et al., 2018), and being suspended in air or on substrates (Eberhard, 2020), spider webs passively filter organisms other than the spider host and its prey. Gregorič et al. (2022) demonstrated that spider webs harbor environmental DNA (eDNA) of hundreds or thousands of animals, fungi, bacteria, and most likely, viruses. Given the web architecture diversity, and the durability of silks, future studies should design ecological experiments where spider webs serve as non-invasive samplers of the total eDNA in ecosystems. Alternatively, spider webs could be used for more targeted monitoring of environmentally or medically important species of pests, parasites or pathogens.
Given the immense diversity of arachnid forms, ecologies, life histories, and evolutionary imprints, arachnology has had little trouble selecting suitable model species or clades to address some of the fundamental biogeographic questions relating to dispersal, vicariance, and extinction. As examples, biogeographic patterns in hooded tick-spiders (Ricinulei), the harvestmen lineage Cyphophthalmi, as well as in certain ground-burrowing lineages of spiders (Mesothelae and Mygalomorphae) seem to be heavily imprinted by vicariant events (Boyer et al., 2007; Opatova et al., 2013; Fernández and Giribet, 2015; Xu et al., 2015). The biogeographic patterns in these lineages are often interpreted as direct consequences of historic tectonic events, or events such as mountain uplifts, sea level fluctuations or river formation (Xu et al., 2016; Xu et al., 2018). In contrast, numerous groups of spiders that regularly disperse aerially and thus more easily maintain gene flow over bodies of land or water have been more informative about speciation on oceanic islands (Gillespie, 2002; Hormiga et al., 2003; Kuntner and Agnarsson, 2011; Casquet et al., 2015). Arachnids also play prominently in subterranean biology research (Arnedo et al., 2007; Harms et al., 2018; Mammola et al., 2020).
Considering how different biological traits affect the evolutionary imprints of organisms, the most powerful approaches to biogeography would overlay as many different lineages as possible over the same geographic area, and seek for congruence over conflicts amongst datasets. One such massive sampling in the Caribbean archipelago (http://www.islandbiogeography.org/) offered reconstructed biogeographic histories of numerous arachnid clades of varying dispersal propensities (Dziki et al., 2015; Agnarsson et al., 2016; Čandek et al., 2019; Tong et al., 2019; Čandek et al., 2020a; Chamberland et al., 2020; Crews and Esposito, 2020; Čandek et al., 2021; Shapiro et al., 2022). Such multi-clade studies can powerfully test the ultimate biogeographic hypotheses specific to a particular archipelago. Among the grand field challenges, therefore, is to perform similar massive field samplings along other major global biogeographic hotspots to address fundamental biogeographical questions.
At the same time, next-generation biogeography should improve on the accuracy of analytical tools. Kuntner and Turk (2022) argue that among the elements needed for historical biogeographic inference, the estimations of organismal dispersal probabilities are particularly vague, representing the bottle neck in historical biogeography. Their appeal for dispersal probability estimations that are better informed and biologically meaningful has so far only been tested on spiders (Turk et al., 2020; Turk et al., 2021; Magalhaes and Ramirez (2022)). If the better devised estimations of dispersal probability are accepted by this community, then perhaps arachnids will be established as the model lineage of choice for applications of the next-generation biogeography (Kuntner and Turk, 2022).
Grand challenge 6: Disentangle evolutionary drivers of arachnid diversity
What arachnid traits, or what extrinsic factors may predict, or even drive, the extreme diversity of certain lineages? In spiders, comparative research points towards a correlative link between small male body size and extreme species diversity: Čandek et al. (2020b) tested the predictive power of 22 variables deriving from morphological, genetic, geographic, ecological, and behavioral landscapes on species richness of 45 spider genera. Within their sample, no variable that described spider-specific biologies predicted species richness, but one biologically universal trait—small male body size—did. Comparative research on other arachnid groups should test whether this finding is generally valid for arachnids, or even broader, for arthropods.
Research shows that body size of an average spider female is 6.9 mm and of a male is 5.6 mm (Kuntner and Coddington, 2020). This overall modest sexual size dimorphism has repeatedly evolved to extremes in orb-weaving spiders, with females in some species overgrowing males 10 or even 14-fold. If size differences are measured in mass, they are even more dramatic: while attached on the host, ‘gluttonous’ female ixodid ticks increase their mass 100 times of the original size, while the males remain comparatively tiny (Sojka et al., 2013). In spiders, Nephila females on average outweigh their male partners 125 times (Kuntner et al., 2012b). This female biased, extreme sexual size dimorphism (eSSD) in arachnids is the greatest among all terrestrial life.
The evolution of eSSD in spiders is explained through the differential equilibrium model, whereby sex-specific selection pressures, relating to the processes of both natural and sexual selection, act additively or antagonistically to sum up the overall selection (Kuntner and Coddington, 2020). Once the genetic correlation between the sexes is broken, eSSD emerges through runaway selection. Whether this or similar evolutionary models can be more broadly generalized to apply to body sizes in arachnids is among the field’s grand challenges. But it is possible that, rather than a driver of diversity, extreme phenotypes such as eSSD may in fact be evolutionary dead ends, driving lineages towards extinction (Kuntner and Coddington, 2020). A similar phenomenon has been detected in the evolution of sociality in otherwise solitary and cannibalistic spiders (Agnarsson et al., 2006).
Much remains to be learnt from variation in body size among arachnids, particularly how it is inherited and how plastic versus canalized body sizes are and in what context (Walzer and Schausberger, 2014; Turk et al., 2018; Quiñones-Lebrón et al., 2021). Body sizes affect other phenotypic traits, notably behaviors. eSSD, for example, may be linked to the levels of sexual conflict that arises when male and female interests in the mating context diverge (Parker, 1979; Chapman et al., 2003; Tregenza et al., 2006). At least in arachnids, this field is somewhat controversial as it explains behavioral and evolutionary patterns though intersexual arms race (Kuntner et al., 2009a) rather than cryptic female choice (Eberhard, 2004; Peretti and Aisenberg, 2015).
Behavioral literature on spiders has ample examples of the imprints of sexual conflict. For example, sexual conflict is the best explanation for the occurrence of sexual cannibalism (Elgar, 1991; Elgar and Schneider, 2004; Kralj-Fišer et al., 2012; Kralj-Fišer et al., 2013; Schneider, 2014; Kralj-Fišer et al., 2016), and for evolution of opportunistic mating (Uhl et al., 2015; Sentenská et al., 2021). Further adaptations that could be interpreted as sexually conflicted are male self-sacrifice and male spontaneous death (Andrade, 1996; Welke and Schneider, 2010; Schwartz et al., 2013; Schwartz et al., 2014; Neumann and Schneider, 2020), genital mutilation and plugging (Uhl et al., 2007; Kuntner et al., 2009b; Uhl et al., 2010; Kuntner et al., 2012a), emasculation (Lee et al., 2012; Kuntner et al., 2015b; Kuntner et al., 2015a), remote copulation (Li et al., 2012), mate guarding, binding, or biting and other coercive acts (Zhang et al., 2011; Sentenská et al., 2020), male strategic allocation of sperm depending on levels of perceived cannibalism (Zhang et al., 2022b), and finally, males catapulting to safety to avoid being cannibalized (Zhang et al., 2022a). Whether or not these adaptations are merely spider specific or indicative of broader evolutionary patterns in arachnids is yet to be established.
Among the field grand challenges, thus, is a more precise evaluation to what extent the emergence of arachnid phenotypes is shaped by classical selection processes, and under what conditions, if any, sexual conflict needs to be invoked.
Grand challenge 7: Define effective conservation measures for arachnids in the light of global changes
Impediments to arachnid conservation mirror those for most invertebrates, and so do the proposed solutions (Cardoso et al., 2011). Arachnid science should attempt to redefine the role of arachnids in biological conservation given their genetic and functional diversity, as well as their known and unknown roles in ecological webs. The conservation measures should be assessed repeatedly in the light of global changes. Namely, research on arachnids and insects reveals that changes in temperature affect all behavioral interactions in a mating context (Leith et al., 2021). As a result of urbanization, anthropogenic noise also impacts negatively the functioning of arthropod communication by altering their soundscape (Classen-Rodríguez et al., 2021), and, likely, their vibroscape (e.g. Šturm et al., 2021). The effects of human degradation of the planet are thus directly manifested in the communities of arachnids, which are known to employ complex multimodal communication (Hebets et al., 2006). Finally, arachnid species are predicted to either go locally extinct or shift their ecological and geographical optima in response to global changes (Gobbi et al., 2006; Kuntner et al., 2014; Krehenwinkel et al., 2015; Mammola et al., 2019). These shifts will create new species communities with unknown consequences.
Among the field’s challenges is to define appropriate and effective conservation measures for arachnids by integrating data from (phylogenetic) diversity, physiology, ecology, biogeography, and global change biology.
Conclusions
This perspective identifies the seven grand challenges in arachnid science: 1. Grasp the arachnid species diversity; 2. Standardize arachnid systematics research; 3. Interpret arachnid trait evolution through omics approaches; 4. Facilitate biotechnological applications of arachnid molecules and biomaterials; 5. Utilize arachnids as models in ecological and biogeographic research; 6. Disentangle evolutionary drivers of arachnid diversity; 7. Define effective conservation measures for arachnids in the light of global changes. Additional grand challenges in arachnid science will be defined, more specific to arachnid diversity and conservation, to arachnid morphology and systematics to arachnid micriobiota and vector-borne diseases, and to arachnid ecology and behavior. Frontiers in Arachnid Science is a new, open access venue to unite for the first time the scientific advances in all of arachnid science. It aims to become the main scientific forum for publishing high quality research bearing on arachnids and their chelicerate cousins. It will advance the basic knowledge on arachnid biology and their roles in ecosystems, strive to better understand and preserve their genetic, phenotypic, ecological, and functional diversity, and move towards applications of arachnid evolutionary novelties.
Author contributions
The author confirms being the sole contributor of this work and has approved it for publication.
Funding
This work was supported in part by the Slovenian Research Agency (Javna agencija za raziskovalno dejavnost Republike Slovenije) (research core funding P1-0255).
Acknowledgments
I thank Ingi Agnarsson, Alejandro Cabezas-Cruz, Peter Schausberger, Matjaž Gregorič, Simona Kralj-Fišer, and Eva Turk for helpful comments or suggestions.
Conflict of interest
The author declares that the research was conducted in the absence of any commercial or financial relationships that could be construed as a potential conflict of interest.
Publisher’s note
All claims expressed in this article are solely those of the authors and do not necessarily represent those of their affiliated organizations, or those of the publisher, the editors and the reviewers. Any product that may be evaluated in this article, or claim that may be made by its manufacturer, is not guaranteed or endorsed by the publisher.
References
Adis J., Harvey M. S. (2000). How many Arachnida and Myriapoda are there world-wide and in Amazonia? Stud. Neotrop. Fauna. Environ. 35, 139–141. doi: 10.1076/0165-0521
Agnarsson I., Avilés L., Coddington J. A., Maddison W. P. (2006). Sociality in theridiid spiders: repeated origins of an evolutionary dead end. Evol. (N. Y). 60, 2342–2351.
Agnarsson I., Coddington J. A., Kuntner M. (2013). “Systematics: progress in the study of spider diversity and evolution,” in Spider research in the 21st century: Trends and perspectives. Ed. Penney D. (Manchester: Siri Scientific Press), 58–111.
Agnarsson I., Kuntner M. (2007). Taxonomy in a changing world: Seeking solutions for a science in crisis. Syst. Biol. 56, 531–539. doi: 10.1080/10635150701424546
Agnarsson I., Kuntner M., Blackledge T. A. (2010). Bioprospecting finds the toughest biological material: Extraordinary silk from a giant riverine orb spider. PloS One 5, 1–8. doi: 10.1371/journal.pone.0011234
Agnarsson I., LeQuier S. M., Kuntner M., Cheng R.-C., Coddington J. A., Binford G. J. (2016). Phylogeography of a good Caribbean disperser: Argiope argentata (Araneae, Araneidae) and a new ‘cryptic’ species from Cuba. Zookeys 2016, 25–44. doi: 10.3897/zookeys.625.8729
Andrade M. C. B. (1996). Sexual selection for male sacrifice in the Australian redback spider. Science 271, 70–72. doi: 10.1126/science.271.5245.70
Aounallah H., Bensaoud C., M’ghirbi Y., Faria F., Chmelar̆ J., Kotsyfakis M. (2020). Tick salivary compounds for targeted immunomodulatory therapy. Front. Immunol. 11. doi: 10.3389/fimmu.2020.583845
Arakawa K., Kono N., Malay A. D., Tateishi A., Ifuku N., Masunaga H., et al. (2022). 1000 spider silkomes: Linking sequences to silk physical properties. Sci. Adv. 8, 6043. doi: 10.1126/sciadv.abo6043
Arnedo M. A., Oromí P., Múrria C., Macías-Hernández N., Ribera C. (2007). The dark side of an island radiation: Systematics and evolution of troglobitic spiders of the genus Dysdera Latreille (Araneae: Dysderidae) in the Canary Islands. Invertebr. Syst. 21, 623. doi: 10.1071/IS07015
Ayoub N. A., Garb J. E., Tinghitella R. M., Collin M. A., Hayashi C. Y. (2007). Blueprint for a high-performance biomaterial: Full-length spider dragline silk genes. PloS One 6, e514. doi: 10.1371/journal.pone.0000514
Babb P. L., Gregorič M., Lahens N. F., Nicholson D. N., Hayashi C. Y., Higgins L., et al. (2022). Characterization of the genome and silk-gland transcriptomes of Darwin’s bark spider (Caerostris darwini). PloS One 17, e0268660. doi: 10.1371/journal.pone.0268660
Babb P. L., Lahens N. F., Correa-Garhwal S. M., Nicholson D. N., Kim E. J., Hogenesch J. B., et al. (2017). The Nephila clavipes genome highlights the diversity of spider silk genes and their complex expression. Nat. Genet. 49, 895–903. doi: 10.1038/ng.3852
Ballesteros J. A., Santibáñez-López C. E. S., Kováč L., Gavish-Regev E., Sharma P. P. (2019). Ordered phylogenomic subsampling enables diagnosis of systematic errors in the placement of the enigmatic arachnid order Palpigradi. Proc. R. Soc. B.: Biol. Sci. 286. doi: 10.1098/rspb.2019.2426
Ballesteros J. A., Santibáñez-López C. E., Baker C. M., Benavides L. R., Cunha T. J., Gainett G., et al. (2022). Comprehensive species sampling and sophisticated algorithmic approaches refute the monophyly of Arachnida. Mol. Biol. Evol. 39. doi: 10.1093/molbev/msac021
Ballesteros J. A., Sharma P. P. (2019). A critical appraisal of the placement of Xiphosura (Chelicerata) with account of known sources of phylogenetic error. Syst. Biol. 68, 896–917. doi: 10.1093/sysbio/syz011
Ban X. C., Shao Z. K., Wu L. J., Sun J. T., Xue X. F. (2022). Highly diversified mitochondrial genomes provide new evidence for interordinal relationships in the Arachnida. Cladistics 38, 452–464. doi: 10.1111/cla.12504
Blackledge T. A., Kuntner M., Agnarsson I. (2011). The form and function of spider orb webs: Evolution from silk to ecosystems. Adv. In. Insect Phys. 41, 175–262. doi: 10.1016/B978-0-12-415919-8.00004-5
Blamires S. J., Martens P. J., Kasumovic M. M. (2018). Fitness consequences of plasticity in an extended phenotype. J. Exp. Biol. 221, jeb167288. doi: 10.1242/jeb.167288
Bond J. E., Garrison N. L., Hamilton C. A., Godwin R. L., Hedin M., Agnarsson I. (2014). Phylogenomics resolves a spider backbone phylogeny and rejects a prevailing paradigm for orb web evolution. Curr. Biol. 24, 1765–1771. doi: 10.1016/j.cub.2014.06.034
Bond J. E., Godwin R. L., Colby J. D., Newton L. G., Zahnle X. J., Agnarsson I., et al. (2021). Improving taxonomic practices and enhancing its extensibility–an example from araneology. Diversity (Basel). 14, 1–15. doi: 10.3390/d14010005
Boyer S. L., Clouse R. M., Benavides L. R., Sharma P., Schwendinger P. J., Karunarathna I., et al. (2007). Biogeography of the world: A case study from cyphophthalmid Opiliones, a globally distributed group of arachnids. J. Biogeogr. 34, 2070–2085. doi: 10.1111/j.1365-2699.2007.01755.x
Čandek K., Agnarsson I., Binford G. J., Kuntner M. (2019). Biogeography of the Caribbean Cyrtognatha spiders. Sci. Rep. 9, 397. doi: 10.1038/s41598-018-36590-y
Čandek K., Agnarsson I., Binford G. J., Kuntner M. (2020a). Caribbean golden orbweaving spiders maintain gene flow with north America. Zool. Scr. 49, 210–221. doi: 10.1111/zsc.12405
Čandek K., Agnarsson I., Binford G. J., Kuntner M. (2021). Biogeography of long-jawed spiders reveals multiple colonization of the Caribbean. Diversity (Basel). 13, 1–18. doi: 10.3390/d13120622
Čandek K., Pristovšek Čandek U., Kuntner M. (2020b). Machine learning approaches identify male body size as the most accurate predictor of species richness. BMC Biol. 18, 105. doi: 10.1186/s12915-020-00835-y
Cao X., Liu J., Chen J., Zheng G., Kuntner M., Agnarsson I. (2016). Rapid dissemination of taxonomic discoveries based on DNA barcoding and morphology. Sci. Rep. 6, 37066. doi: 10.1038/srep37066
Cao Z., Yu Y., Wu Y., Hao P., Di Z., He Y., et al. (2013). The genome of Mesobuthus martensii reveals a unique adaptation model of arthropods. Nat. Commun. 4, 2602. doi: 10.1038/ncomms3602
Cardoso P. (2009). Standardization and optimization of arthropod inventories-the case of Iberian spiders. Biodivers. Conserv. 18, 3949–3962. doi: 10.1007/s10531-009-9690-7
Cardoso P., Erwin T. L., Borges P. A. V., New T. R. (2011). The seven impediments in invertebrate conservation and how to overcome them. Biol. Conserv. 144, 2647–2655. doi: 10.1016/j.biocon.2011.07.024
Cardoso P., Henriques S. S., Gaspar C., Crespo L. C., Carvalho R., Schmidt J. B., et al. (2009). Species richness and composition assessment of spiders in a Mediterranean scrubland. J. Insect Conserv. 13, 45–55. doi: 10.1007/s10841-007-9116-3
Casquet J., Bourgeois Y. X. C., Cruaud C., Gavory F., Gillespie R. G., Thébaud C. (2015). Community assembly on remote islands: A comparison of Hawaiian and Mascarene spiders. J. Biogeogr. 42, 39–50. doi: 10.1111/jbi.12391
Cerca J., Armstrong E. E., Vizueta J., Fernández R., Dimitrov D., Petersen B., et al. (2021). The Tetragnatha kauaiensis genome sheds light on the origins of genomic novelty in spiders. Genome Biol. Evol. 13, 1–17. doi: 10.1093/gbe/evab262
Chamberland L., Agnarsson I., Quayle I. L., Ruddy T., Starrett J., Bond J. E. (2022). Biogeography and eye size evolution of the ogre-faced spiders. Sci. Rep. 12, 17769. doi: 10.1038/s41598-022-22157-5
Chamberland L., Salgado-Roa F. C., Basco A., Crastz-Flores A., Binford G. J., Agnarsson I. (2020). Phylogeography of the widespread Caribbean spiny orb weaver Gasteracantha cancriformis. PeerJ 2020, 1–27. doi: 10.7717/peerj.8976
Chapman T., Arnqvist G., Bangham J., Rowe L. (2003). Sexual conflict. Trends Ecol. Evol. 18, 41–47. doi: 10.1016/S0169-5347(02)00004-6
Chmelař J., Kotál J., Kovaříková A., Kotsyfakis M. (2019). The use of tick salivary proteins as novel therapeutics. Front. Physiol. 10. doi: 10.3389/fphys.2019.00812
Classen-Rodríguez L., Tinghitella R., Fowler-Finn K. (2021). Anthropogenic noise affects insect and arachnid behavior, thus changing interactions within and between species. Curr. Opin. Insect Sci. 47, 142–153. doi: 10.1016/j.cois.2021.06.005
Coddington J. A., Agnarsson I., Miller J. A., Kuntner M., Hormiga G. (2009). Undersampling bias: The null hypothesis for singleton species in tropical arthropod surveys. J. Anim. Ecol. 78, 573–584. doi: 10.1111/j.1365-2656.2009.01525.x
Coddington J. A., Giribet G., Harvey M. S., Prendini L., Walter D. E. (2004). “Arachnida,” in Assembling the tree of life, vol. 296–318. .
Coddington J., Young L. H., Coyle F. (1996). Estimating spider species richness in a southern Appalachian cove hardwood forest. J. Arachnol. 24, 111–128. doi: 10.2307/3705945
Cornman S. R., Schatz M. C., Johnston S. J., Chen Y.-P., Pettis J., Hunt G., et al. (2010). Genomic survey of the ectoparasitic mite Varroa destructor, a major pest of the honey bee Apis mellifera. BMC Genomics 11, 1–15. doi: 10.1186/1471-2164-11-602
Correa-Garhwal S. M., Babb P. L., Voight B. F., Hayashi C. Y. (2021). Golden orb-weaving spider (Trichonephila clavipes) silk genes with sex-biased expression and atypical architectures. G3.: Genes. Genomes. Genet. 11, 1. doi: 10.1093/G3JOURNAL/JKAA039
Craig C. L. (1997). Evolution of arthropod silks. Annu. Rev. Entomol. 42, 231–267. doi: 10.1146/annurev.ento.42.1.231
Crews S. C., Esposito L. A. (2020). Towards a synthesis of the Caribbean biogeography of terrestrial arthropods. BMC Evol. Biol. 20, 1–27. doi: 10.1186/s12862-019-1576-z
de la Fuente J., Contreras M., Estrada-Peña A., Cabezas-Cruz A. (2017). Targeting a global health problem: Vaccine design and challenges for the control of tick-borne diseases. Vaccine 35, 5089–5094. doi: 10.1016/j.vaccine.2017.07.097
Dong X., Chaisiri K., Xia D., Armstrong S. D., Fang Y., Donnelly M. J., et al. (2018). Genomes of trombidid mites reveal novel predicted allergens and laterally transferred genes associated with secondary metabolism. Gigascience 7, 1–33. doi: 10.1093/gigascience/giy127
Dziki A., Binford G. J., Coddington J. A., Agnarsson I. (2015). Spintharus flavidus in the Caribbean–a 30 million year biogeographical history and radiation of a ‘widespread species.’. PeerJ 3, e1422. doi: 10.7717/peerj.1422
Eberhard W. G. (2004). Male-female conflict and genitalia: Failure to confirm predictions in insects and spiders. Biol. Rev. Camb. Philos. Soc. 79, 121–186. doi: 10.1017/S1464793103006237
Elgar M. A. (1991). Sexual cannibalism, size dimorphism, and courtship in orb-weaving spiders (Araneidae). Evolution 45, 444–448. doi: 10.2307/2409679
Elgar M. A., Schneider J. M. (2004). Evolutionary significance of sexual cannibalism. Adv. Study. Behav. 34, 135–163. doi: 10.1016/S0065-3454(04)34004-0
Faircloth B. C., McCormack J. E., Crawford N. G., Harvey M. G., Brumfield R. T., Glenn T. C. (2012). Ultraconserved elements anchor thousands of genetic markers spanning multiple evolutionary timescales. Syst. Biol. 61, 717–726. doi: 10.1093/sysbio/sys004
Fan Z., Yuan T., Liu P., Wang L. Y., Jin J. F., Zhang F., et al. (2021). A chromosome-level genome of the spider Trichonephila antipodiana reveals the genetic basis of its polyphagy and evidence of an ancient whole-genome duplication event. Gigascience 10, 1–15. doi: 10.1093/gigascience/giab016
Fernández R., Giribet G. (2015). Unnoticed in the tropics: Phylogenomic resolution of the poorly known arachnid order Ricinulei (Arachnida). R. Soc. Open Sci. 2, 150065. doi: 10.1098/rsos.150065
Fernández R., Hormiga G., Giribet G. (2014). Phylogenomic analysis of spiders reveals nonmonophyly of orb weavers. Curr. Biol. 24, 1772–1777. doi: 10.1016/j.cub.2014.06.035
Garb J. E., Haney R. A., Schwager E. E., Gregorič M., Kuntner M., Agnarsson I., et al. (2019). The transcriptome of Darwin’s bark spider silk glands predicts proteins contributing to dragline silk toughness. Commun. Biol. 2, 275. doi: 10.1038/s42003-019-0496-1
Garb J. E., Sharma P. P., Ayoub N. A. (2018). Recent progress and prospects for advancing arachnid genomics. Curr. Opin. Insect Sci. 25, 51–57. doi: 10.1016/j.cois.2017.11.005
Gillespie R. G. (2002). Biogeography of spiders on remote oceanic islands of the Pacific: archipelagoes as stepping stones? J. Biogeogr. 29, 655–662. doi: 10.1046/j.1365-2699.2002.00714.x
Gobbi M., Fontaneto D., de Bernardi F. (2006). Influence of climate changes on animal communities in space and time: The case of spider assemblages along an alpine glacier foreland. Glob. Chang. Biol. 12, 1985–1992. doi: 10.1111/j.1365-2486.2006.01236.x
Grbić M., van Leeuwen T., Clark R. M., Rombauts S., Rouzé P., Grbić V., et al. (2011). The genome of Tetranychus urticae reveals herbivorous pest adaptations. Nature 479, 487–492. doi: 10.1038/nature10640
Gregorič M., Kutnjak D., Bačnik K., Gostinčar C., Pecman A., Ravnikar M., et al. (2022). Spider webs as eDNA samplers: Biodiversity assessment across the tree of life. Mol. Ecol. Resour. 22, 2534–2545. doi: 10.1111/1755-0998.13629
Gulia-Nuss M., Nuss A. B., Meyer J. M., Sonenshine D. E., Roe R. M., Waterhouse R. M., et al. (2016). Genomic insights into the Ixodes scapularis tick vector of Lyme disease. Nat. Commun. 7, 10507. doi: 10.1038/ncomms10507
Hamilton C. A., Lemmon A. R., Lemmon E. M., Bond J. E. (2016). Expanding anchored hybrid enrichment to resolve both deep and shallow relationships within the spider tree of life. BMC Evol. Biol. 16, 212. doi: 10.1186/s12862-016-0769-y
Harms D., Curran M. K., Klesser R., Finston T. L., Halse S. A. (2018). Speciation patterns in complex subterranean environments: A case study using short-tailed whipscorpions (Schizomida: Hubbardiidae). Biol. J. Linn. Soc. 125, 355–367. doi: 10.1093/biolinnean/bly102
Harper A., Gonzalez L. B., Schönauer A., Janssen R., Seiter M., Holzem M., et al. (2021). Widespread retention of ohnologs in key developmental gene families following whole-genome duplication in arachnopulmonates. G3.: Genes. Genomes. Genet. 11, 12. doi: 10.1093/g3journal/jkab299
Harvey M. S. (2002). The neglected cousins: What do we know about the smaller arachnid orders? J. Arachnol. 30, 357–372. doi: 10.1636/0161-8202
Hebets E. A., Cuasay K., Rivlin P. K. (2006). The role of visual ornamentation in female choice of a multimodal male courtship display. Ethology 112, 1062–1070. doi: 10.1111/j.1439-0310.2006.01274.x
Hormiga G., Arnedo M., Gillespie R. G. (2003). Speciation on a conveyor belt: Sequential colonization of the Hawaiian Islands by Orsonwelles spiders (Araneae, Linyphiidae). Syst. Biol. 52, 70–88. doi: 10.1080/10635150390132786
Hormiga G., Griswold C. E. (2014). Systematics, phylogeny, and evolution of orb-weaving spiders. Annu. Rev. Entomol. 59, 487–512. doi: 10.1146/annurev-ento-011613-162046
Hoy M. A., Waterhouse R. M., Wu K., Estep A. S., Ioannidis P., Palmer W. J., et al. (2016). Genome sequencing of the phytoseiid predatory mite Metaseiulus occidentalis reveals completely atomized Hox genes and superdynamic intron evolution. Genome Biol. Evol. 8, 1762–1775. doi: 10.1093/gbe/evw048
Jia N., Wang J., Shi W., Du L., Sun Y., Zhan W., et al. (2020). Large-scale comparative analyses of tick genomes elucidate their genetic diversity and vector capacities. Cell 182, 1328–1340.e13. doi: 10.1016/j.cell.2020.07.023
Knap N., Durmiši E., Saksida A., Korva M., Petrovec M., Avšič-Županc T. (2009). Influence of climatic factors on dynamics of questing Ixodes ricinus ticks in Slovenia. Vet. Parasitol. 164, 275–281. doi: 10.1016/j.vetpar.2009.06.001
Kono N., Nakamura H., Ohtoshi R., Moran D. A. P., Shinohara A., Yoshida Y., et al. (2019). Orb-weaving spider Araneus ventricosus genome elucidates the spidroin gene catalogue. Sci. Rep. 9, 1–13. doi: 10.1038/s41598-019-44775-2
Kono N., Ohtoshi R., Malay A. D., Mori M., Masunaga H., Yoshida Y., et al. (2021). Darwin’s bark spider shares a spidroin repertoire with Caerostris extrusa but achieves extraordinary silk toughness through gene expression. Open Biol. 11, 210242. doi: 10.1098/rsob.210242
Král J., Forman M., Kořínková T., Lerma A. C. R., Haddad C. R., Musilová J., et al. (2019). Insights into the karyotype and genome evolution of haplogyne spiders indicate a polyploid origin of lineage with holokinetic chromosomes. Sci. Rep. 9, 3001. doi: 10.1038/s41598-019-39034-3
Kralj-Fišer S., Čandek K., Lokovšek T., Čelik T., Cheng R.-C., Elgar M. A., et al. (2016). Mate choice and sexual size dimorphism, not personality, explain female aggression and sexual cannibalism in raft spiders. Anim. Behav. 111, 49–55. doi: 10.1016/j.anbehav.2015.10.013
Kralj-Fišer S., Schneider J. M., Justinek Ž., Kalin S., Gregorič M., Pekár S., et al. (2012). Mate quality, not aggressive spillover, explains sexual cannibalism in a size-dimorphic spider. Behav. Ecol. Sociobiol. 66, 145–151. doi: 10.1007/s00265-011-1262-7
Kralj-Fišer S., Schneider J. M., Kuntner M. (2013). Challenging the aggressive spillover hypothesis: Is pre-copulatory sexual cannibalism a part of a behavioural syndrome? Ethology 119, 615–623. doi: 10.1111/eth.12111
Krantz G., Walter D. E. (2009). A manual of acarology. 3rd edition. Eds. Krantz G., Walter D. E. (Lubbock, Texas: Texas Tech University Press).
Krehenwinkel H., Kennedy S., Pekár S., Gillespie R. G. (2017). A cost-efficient and simple protocol to enrich prey DNA from extractions of predatory arthropods for large-scale gut content analysis by Illumina sequencing. Methods Ecol. Evol. 8, 126–134. doi: 10.1111/2041-210X.12647
Krehenwinkel H., Rödder D., Tautz D. (2015). Eco-genomic analysis of the poleward range expansion of the wasp spider Argiope bruennichi shows rapid adaptation and genomic admixture. Glob. Chang. Biol. 21, 4320–4332. doi: 10.1111/gcb.13042
Kulkarni S., Wood H., Lloyd M., Hormiga G. (2020). Spider-specific probe set for ultraconserved elements offers new perspectives on the evolutionary history of spiders (Arachnida, Araneae). Mol. Ecol. Resour. 20, 185–203. doi: 10.1111/1755-0998.13099
Kuntner M., Agnarsson I. (2011). Biogeography and diversification of hermit spiders on Indian Ocean islands (Nephilidae: Nephilengys). Mol. Phylogenet. Evol. 59, 477–488. doi: 10.1016/j.ympev.2011.02.002
Kuntner M., Agnarsson I., Li D. (2015a). The eunuch phenomenon: Adaptive evolution of genital emasculation in sexually dimorphic spiders. Biol. Rev. 90, 279–296. doi: 10.1111/brv.12109
Kuntner M., Coddington J. A. (2020). Sexual size dimorphism: Evolution and perils of extreme phenotypes in spiders. Annu. Rev. Entomol. 65, 57–80. doi: 10.1146/annurev-ento-011019-025032
Kuntner M., Coddington J. A., Schneider J. M. (2009a). Intersexual arms race? Genital coevolution in nephilid spiders (Araneae, Nephilidae). Evolution 63, 1451–1463. doi: 10.1111/j.1558-5646.2009.00634.x
Kuntner M., Gregoric M., Zhang S., Kralj-Fiser S., Li D. (2012a). Mating plugs in polyandrous giants: Which sex produces them, when, how and why? PloS One 7, e40939. doi: 10.1371/journal.pone.0040939
Kuntner M., Hamilton C. A., Cheng R.-C., Gregorič M., Lupše N., Lokovšek T., et al. (2019). Golden orbweavers ignore biological rules: Phylogenomic and comparative analyses unravel a complex evolution of sexual size dimorphism. Syst. Biol. 68, 555–572. doi: 10.1093/sysbio/syy082
Kuntner M., Kralj-Fišer S., Schneider J. M., Li D. (2009b). Mate plugging via genital mutilation in nephilid spiders: An evolutionary hypothesis. J. Zool. 277, 257–266. doi: 10.1111/j.1469-7998.2008.00533.x
Kuntner M., Năpăruş M., Li D., Coddington J. A. (2014). Phylogeny predicts future habitat shifts due to climate change. PloS One 9, e98907. doi: 10.1371/journal.pone.0098907
Kuntner M., Pristovšek U., Cheng R.-C., Li D., Zhang S., Tso I.-M., et al. (2015b). Eunuch supremacy: evolution of post-mating spider emasculation. Behav. Ecol. Sociobiol. 69, 117–126. doi: 10.1007/s00265-014-1824-6
Kuntner M., Turk E. (2022). Towards better-informed dispersal probabilities in historical biogeography: Arachnids as a model lineage. Front Arachnid Sci. doi: 10.3389/frchs.2022.1058676
Kuntner M., Zhang S., Gregorič M., Li D. (2012b). Nephila female gigantism attained through post-maturity molting. J. Arachnol. 40, 345–347. doi: 10.1636/B12-03.1
Leal-Egaña A., Scheibel T. (2010). Silk-based materials for biomedical applications. Biotechnol. Appl. Biochem. 55, 155–167. doi: 10.1042/BA20090229
Leannec-Rialland V., Cabezas-Cruz A., Atanasova V., Chereau S., Ponts N., Tonk M., et al. (2021). Tick defensin γ-core reduces Fusarium graminearum growth and abrogates mycotoxins production with high efficiency. Sci. Rep. 11, 7962. doi: 10.1038/s41598-021-86904-w
Lee Q. Q., Oh J., Kralj-Fiser S., Kuntner M., Li D. (2012). Emasculation: gloves-off strategy enhances eunuch spider endurance. Biol. Lett. 8, 733–735. doi: 10.1098/rsbl.2012.0285
Leith N. T., Macchiano A., Moore M. P., Fowler-Finn K. D. (2021). Temperature impacts all behavioral interactions during insect and arachnid reproduction. Curr. Opin. Insect Sci. 45, 106–114. doi: 10.1016/j.cois.2021.03.005
Lemmon A. R., Emme S. A., Lemmon E. M. (2012). Anchored hybrid enrichment for massively high-throughput phylogenomics. Syst. Biol. 61, 727–744. doi: 10.1093/sysbio/sys049
Lewis R. V. (2006). Spider silk: Ancient ideas for new biomaterials. Chem. Rev. 106, 3762–3774. doi: 10.1021/cr010194g
Li D., Oh J., Kralj-Fišer S., Kuntner M. (2012). Remote copulation: Male adaptation to female cannibalism. Biol. Lett. 8, 512–515. doi: 10.1098/rsbl.2011.1202
Li Y. Y., Tsai J. M., Wu C. Y., Chiu Y. F., Li H. Y., Warrit N., et al. (2022). In silico assessment of probe-capturing strategies and effectiveness in the spider sub-lineage Araneoidea (Order: Araneae). Diversity (Basel). 14, 184. doi: 10.3390/d14030184
Liu S., Aagaard A., Bechsgaard J., Bilde T. (2019). DNA methylation patterns in the social spider, Stegodyphus dumicola. Genes (Basel). 10, 137. doi: 10.3390/genes10020137
Lozano-Pérez A. A., Pagán A., Zhurov V., Hudson S. D., Hutter J. L., Pruneri V., et al. (2020). The silk of gorse spider mite Tetranychus lintearius represents a novel natural source of nanoparticles and biomaterials. Sci. Rep. 10, 18471. doi: 10.1038/s41598-020-74766-7
Lüddecke T., Herzig V., Reumont B. M., Vilcinskas A. (2022). The biology and evolution of spider venoms. Biol. Rev. 97, 163–178. doi: 10.1111/brv.12793
Maddison W. P., Evans S. C., Hamilton C. A., Bond J. E., Lemmon A. R., Lemmon E. M. (2017). A genome-wide phylogeny of jumping spiders (Araneae, Salticidae), using anchored hybrid enrichment. Zookeys 2017, 89–101. doi: 10.3897/zookeys.695.13852
Magalhaes I. L. F., Ramírez M. J. (2022). Phylogeny and biogeography of the ancient spider family Filistatidae (Araneae) is consistent both with long-distance dispersal and vicariance following continental drift. Cladistics 38, 538–62. doi: 10.1111/cla.12505
Malumbres-Olarte J., Scharff N., Pape T., Coddington J. A., Cardoso P. (2016). Gauging megadiversity with optimized and standardized sampling protocols: A case for tropical forest spiders. Ecol. Evol 7, 494–506. doi: 10.1002/ece3.2626
Mammola S., Arnedo M. A., Fišer C., Cardoso P., Dejanaz A. J., Marco I. (2020). Environmental filtering and convergent evolution determine the ecological specialization of subterranean spiders. Funct. Ecol. 34, 1064–1077. doi: 10.1111/1365-2435.13527
Mammola S., Piano E., Cardoso P., Vernon P., Domínguez-Villar D., Culver D. C., et al. (2019). Climate change going deep: The effects of global climatic alterations on cave ecosystems. Anthropocene. Rev. 6, 98–116. doi: 10.1177/2053019619851594
Mans B. J. (2020). Quantitative visions of reality at the tick-host interface: Biochemistry, genomics, proteomics, and transcriptomics as measures of complete inventories of the tick sialoverse. Front. Cell Infect. Microbiol. 10. doi: 10.3389/fcimb.2020.574405
Marques M. R., Mendes M. A., Tormena C. F., Souza B. M., Ribeiro S. P., Rittner R., et al. (2004). Structure determination of an organometallic 1-(Diazenylaryl) ethanol: A novel toxin subclass from the web of the spider Nephila clavipes. Chem. Biodivers. 1, 830–838. doi: 10.1002/cbdv.200490065
Miller A. J., Zimin A. V., Gordus A. (2022). Chromosome-level genome and chromosomes in Uloborus diversus. doi: 10.1101/2022.06.14.495972
Neumann R., Schneider J. M. (2020). Males sacrifice their legs to pacify aggressive females in a sexually cannibalistic spider. Anim. Behav. 159, 59–67. doi: 10.1016/j.anbehav.2019.11.003
Nong W., Qu Z., Li Y., Barton-Owen T., Wong A. Y. P., Yip H. Y., et al. (2021). Horseshoe crab genomes reveal the evolution of genes and microRNAs after three rounds of whole genome duplication. Commun. Biol. 4, 83. doi: 10.1038/s42003-020-01637-2
Omenetto F. G., Kaplan D. L. (2010). New opportunities for an ancient material. Science 329, 528–531. doi: 10.1126/science.1188936
Ontano A. Z., Steiner H. G., Sharma P. P. (2022). How many long branch orders occur in Chelicerata? Opposing effects of Palpigradi and Opilioacariformes on phylogenetic stability. Mol. Phylogenet. Evol. 168, 107378. doi: 10.1016/j.ympev.2021.107378
Opatova V., Bond J. E., Arnedo M. A. (2013). Ancient origins of the Mediterranean trap-door spiders of the family Ctenizidae (Araneae, mygalomorphae). Mol. Phylogenet. Evol. 69, 1135–1145. doi: 10.1016/j.ympev.2013.08.002
Ortega-Jimenez V. M., Dudley R. (2013). Spider web deformation induced by electrostatically charged insects. Sci. Rep. 3, 1–4. doi: 10.1038/srep02108
Parker G. (1979). “Sexual selection and sexual conflict,” in Sexual selection and reproductive competition in insects (New York: Academic Press), 123–166. doi: 10.1016/B978-0-12-108750-0.50010-0
Peretti A. V., Aisenberg A. (2015). Cryptic female choice in arthropods: Patterns, mechanisms and prospects. (Heidelberg: Springer-Verlag) 1–509. doi: 10.1007/978-3-319-17894-3
Purcell J., Pruitt J. N. (2019). Are personalities genetically determined? Inferences from subsocial spiders. BMC Genomics 20, 867. doi: 10.1186/s12864-019-6172-5
Quiñones-Lebrón S. G., Kuntner M., Kralj-Fišer S. (2021). The effect of genetics, diet, and social environment on adult male size in a sexually dimorphic spider. Evol. Ecol. 35, 217–234. doi: 10.1007/s10682-020-10097-3
Salles H. C., Volsi E. C. F. R., Marques M. R., Souza B. M., dos Santos L. D., Tormena C. F., et al. (2006). The venomous secrets of the web droplets from the viscid spiral of the orb-weaver spider Nephila clavipes (Araneae, Tetragnatidae). Chem. Biodivers. 3, 727–741. doi: 10.1002/cbdv.200690075
Sánchez-Herrero J. F., Frías-López C., Escuer P., Hinojosa-Alvarez S., Arnedo M. A., Sánchez-Gracia A., et al. (2019). The draft genome sequence of the spider Dysdera silvatica (Araneae, Dysderidae): A valuable resource for functional and evolutionary genomic studies in chelicerates. Gigascience 8, 1–9. doi: 10.1093/gigascience/giz099
Sanggaard K. W., Bechsgaard J. S., Fang X., Duan J., Dyrlund T. F., Gupta V., et al. (2014). Spider genomes provide insight into composition and evolution of venom and silk. Nat. Commun. 5, 3765. doi: 10.1038/ncomms4765
Schacht K., Scheibel T. (2014). Processing of recombinant spider silk proteins into tailor-made materials for biomaterials applications. Curr. Opin. Biotechnol. 29, 62–69. doi: 10.1016/j.copbio.2014.02.015
Scharff N., Coddington J. A., Griswold C. E., Hormiga G., de Bjørn P. (2003). When to quit? Estimating spider species richness in a northern European deciduous forest. J. Arachnol. 31, 246–273. doi: 10.1636/0161-8202
Schatz H., Behan-Pelletier V. (2008). Global diversity of oribatids (Oribatida: Acari: Arachnida). Hydrobiologia 595, 323–328. doi: 10.1007/s10750-007-9027-z
Schneider J. M. (2014). Sexual cannibalism as a manifestation of sexual conflict. Cold Spring Harb. Perspect. Biol. 6, a017731. doi: 10.1101/cshperspect.a017731
Schwager E. E., Sharma P. P., Clarke T., Leite D. J., Wierschin T., Pechmann M., et al. (2017). The house spider genome reveals an ancient whole-genome duplication during arachnid evolution. BMC Biol. 15, 62. doi: 10.1186/s12915-017-0399-x
Schwartz S. K., Wagner W. E., Hebets E. A. (2013). Spontaneous male death and monogyny in the dark fishing spider. Biol. Lett. 9, 20130113. doi: 10.1098/rsbl.2013.0113
Schwartz S. K., Wagner W. E., Hebets E. A. (2014). Obligate male death and sexual cannibalism in dark fishing spiders. Anim. Behav. 93, 151–156. doi: 10.1016/j.anbehav.2014.04.037
Sentenská L., Neumann A., Lubin Y., Uhl G. (2021). Functional morphology of immature mating in a widow spider. Front. Zool. 18, 1–18. doi: 10.1186/s12983-021-00404-1
Sentenská L., Šedo O., Pekár S. (2020). Biting and binding: an exclusive coercive mating strategy of males in a philodromid spider. Anim. Behav. 168, 59–68. doi: 10.1016/j.anbehav.2020.08.001
Sethy T. R., Ahi J. (2022). Spider silk and the silk of egg sacs with its astonishing concealed attributes: A review. J. Natural Fibers. 00, 1–15. doi: 10.1080/15440478.2022.2025986
Shapiro L., Binford G. J., Agnarsson I. (2022). Single-island endemism despite repeated dispersal in Caribbean Micrathena (Araneae: Araneidae): An updated phylogeographic analysis. Diversity (Basel). 14, 1–26. doi: 10.3390/d14020128
Sharma P. P., Ballesteros J. A., Santibáñez-López C. E. (2021). What is an “arachnid”? Consensus, consilience, and confirmation bias in the phylogenetics of Chelicerata. Diversity (Basel). 13, 1–33. doi: 10.3390/d13110568
Sheffer M. M., Hoppe A., Krehenwinkel H., Uhl G., Kuss A. W., Jensen L., et al. (2021). Chromosome-level reference genome of the European wasp spider Argiope bruennichi: A resource for studies on range expansion and evolutionary adaptation. Gigascience 10, 1–12. doi: 10.1093/gigascience/giaa148
Shingate P., Ravi V., Prasad A., Tay B. H., Garg K. M., Chattopadhyay B., et al. (2020). Chromosome-level assembly of the horseshoe crab genome provides insights into its genome evolution. Nat. Commun. 11, 2322. doi: 10.1038/s41467-020-16180-1
Shultz J. W. (2001). Gross muscular anatomy of Limulus polyphemus (Xiphosura, Chelicerata) and its bearing on evolution in the Arachnida. J. Arachnol. 29, 283–303. doi: 10.1636/0161-8202
Sojka D., Franta Z., Horn M., Caffrey C. R., Mareš M., Kopáček P. (2013). New insights into the machinery of blood digestion by ticks. Trends Parasitol. 29, 276–285. doi: 10.1016/j.pt.2013.04.002
Starrett J., Derkarabetian S., Hedin M., Bryson R. W., McCormack J. E., Faircloth B. C. (2017). High phylogenetic utility of an ultraconserved element probe set designed for Arachnida. Mol. Ecol. Resour. 17, 812–823. doi: 10.1111/1755-0998.12621
Šturm R., Rexhepi B., López Díez J. J., Blejec A., Polajnar J., Sueur J., et al. (2021). Hay meadow vibroscape and interactions within insect vibrational community. iScience 24, 103070. doi: 10.1016/j.isci.2021.103070
Thomas G. W. C., Dohmen E., Hughes D. S. T., Murali S. C., Poelchau M., Glastad K., et al. (2020). Gene content evolution in the arthropods. Genome Biol. 21, 15. doi: 10.1186/s13059-019-1925-7
Tong Y., Binford G., Rheims C. A., Kuntner M., Liu J., Agnarsson I. (2019). Huntsmen of the Caribbean: Multiple tests of the GAARlandia hypothesis. Mol. Phylogenet. Evol. 130, 259–268. doi: 10.1016/j.ympev.2018.09.017
Tregenza T., Wedell N., Chapman T. (2006). Introduction. Sexual conflict: A new paradigm? Philos. Trans. R. Soc. Lond. B. Biol. Sci. 361, 229–234. doi: 10.1098/rstb.2005.1796
Turk E., Bond J. E., Cheng R.-C., Čandek K., Hamilton C. A., Gregorič M., et al. (2021). A natural colonisation of Asia: Phylogenomic and biogeographic history of coin spiders (Araneae: Nephilidae: Herennia). Diversity (Basel). 13, 515. doi: 10.3390/d13110515
Turk E., Čandek K., Kralj-Fišer S., Kuntner M. (2020). Biogeographical history of golden orbweavers: Chronology of a global conquest. J. Biogeogr. 47, 1333–1344. doi: 10.1111/jbi.13838
Turk E., Kuntner M., Kralj-Fišer S. (2018). Cross-sex genetic correlation does not extend to sexual size dimorphism in spiders. Sci. Nat. 105, 1. doi: 10.1007/s00114-017-1529-6
Uhl G., Nessler S. H., Schneider J. (2007). Copulatory mechanism in a sexually cannibalistic spider with genital mutilation (Araneae: Araneidae: Argiope bruennichi). Zoology 110, 398–408. doi: 10.1016/j.zool.2007.07.003
Uhl G., Nessler S. H., Schneider J. M. (2010). Securing paternity in spiders? A review on occurrence and effects of mating plugs and male genital mutilation. Genetica 138, 75–104. doi: 10.1007/s10709-009-9388-5
Uhl G., Zimmer S. M., Renner D., Schneider J. M. (2015). Exploiting a moment of weakness: Male spiders escape sexual cannibalism by copulating with moulting females. Sci. Rep. 5, 16928. doi: 10.1038/srep16928
van Dam M. H., Trautwein M., Spicer G. S., Esposito L. (2019). Advancing mite phylogenomics: Designing ultraconserved elements for Acari phylogeny. Mol. Ecol. Resour. 19, 465–475. doi: 10.1111/1755-0998.12962
Vollrath F., Knight D. P. (2001). Liquid crystalline spinning of spider silk. Nature 410, 541–548. doi: 10.1038/35069000
Walter D. E., Proctor H. C. (2013). Mites: Ecology, evolution & behaviour (Dordrecht: Springer Netherlands). doi: 10.1007/978-94-007-7164-2
Walzer A., Schausberger P. (2014) Canalization of body size matters for lifetime reproductive success of male predatory mites (Acari: Phytoseiidae). Biol. J. Linn. Soc. 111, 889–899. doi: 10.1111/bij.12235
Wang B., Dunlop J. A., Selden P. A., Garwood R. J., Shear W. A., Müller P., et al. (2018). Cretaceous arachnid Chimerarachne yingi gen. et sp. nov. illuminates spider origins. Nat. Ecol. Evol. 2, 614–622. doi: 10.1038/s41559-017-0449-3
Welke K. W., Schneider J. M. (2010). Males of the orb-web spider Argiope bruennichi sacrifice themselves to unrelated females. Biol. Lett. 6, 585–588. doi: 10.1098/rsbl.2010.0214
Xu X., Kuntner M., Liu F., Chen J., Li D. (2018). Formation of rivers and mountains drives diversification of primitively segmented spiders in continental East Asia. J. Biogeogr. 45, 2080–2091. doi: 10.1111/jbi.13403
Xu X., Liu F., Cheng R. C., Chen J., Xu X., Zhang Z., et al. (2015). Extant primitively segmented spiders have recently diversified from an ancient lineage. Proc. R. Soc. B.: Biol. Sci. 282, 20142486. doi: 10.1098/rspb.2014.2486
Xu X., Liu F., Chen J., Ono H., Agnarsson I., Li D., et al. (2016). Pre-Pleistocene geological events shaping diversification and distribution of primitively segmented spiders on East Asian margins. J. Biogeogr. 43, 1004–1019. doi: 10.1111/jbi.12687
Xu X., Su Y.-C., Ho S. Y. W., Kuntner M., Ono H., Liu F., et al. (2021). Phylogenomic analysis of ultraconserved elements resolves the evolutionary and biogeographic history of segmented trapdoor spiders. Syst. Biol. 70, 1110–1122. doi: 10.1093/sysbio/syaa098
Yu N., Li J., Liu M., Huang L., Bao H., Yang Z., et al (2019) Genome sequencing and neurotoxin diversity of a wandering spider Pardosa pseudoannulata (pond wolf spider) bioRxiv preprint doi: 10.1101/747147
Zhang Y. X., Chen X., Wang J. P., Zhang Z. Q., Wei H., Yu H. Y., et al. (2019). Genomic insights into mite phylogeny, fitness, development, and reproduction. BMC Genomics 20, 954. doi: 10.1186/s12864-019-6281-1
Zhang S., Kuntner M., Li D. (2011). Mate binding: male adaptation to sexual conflict in the golden orb-web spider (Nephilidae: Nephila pilipes). Anim. Behav. 82, 1299–1304. doi: 10.1016/j.anbehav.2011.09.010
Zhang S., Liu Y., Ma Y., Wang H., Zhao Y., Kuntner M., et al. (2022a). Male spiders avoid sexual cannibalism with a catapult mechanism. Curr. Biol. 32, R354–R355. doi: 10.1016/j.cub.2022.03.051
Keywords: diversity, systematics, omics, evolutionary novelty, biomaterials, arachnid biogeography, arachnid evolution, arachnid conservation
Citation: Kuntner M (2022) The seven grand challenges in arachnid science. Front. Arachn. Sci. 1:1082700. doi: 10.3389/frchs.2022.1082700
Received: 28 October 2022; Accepted: 14 November 2022;
Published: 07 December 2022.
Edited by:
Peter Schausberger, University of Vienna, AustriaCopyright © 2022 Kuntner. This is an open-access article distributed under the terms of the Creative Commons Attribution License (CC BY). The use, distribution or reproduction in other forums is permitted, provided the original author(s) and the copyright owner(s) are credited and that the original publication in this journal is cited, in accordance with accepted academic practice. No use, distribution or reproduction is permitted which does not comply with these terms.
*Correspondence: Matjaž Kuntner, bWF0amF6Lmt1bnRuZXJAbmliLnNp