- Department of Molecular Genetics, Institute of Cellular Physiology, National Autonomous University of Mexico, Mexico City, Mexico
The circadian clock synchronizes the temporal activity of physiological processes with geophysical time. At the molecular level circadian rhythms arise from negative feedback loops between activator and repressor transcription factors whose opposite and rhythmic activity at gene promoters sustains cyclic transcription. Additional epigenetic mechanisms driving rhythmic transcription involve dynamic remodeling of the proximal and distal chromatin environment of cyclic genes around the day. In this context, previous studies reported that thousands of enhancer elements display rhythmic activity throughout the 24 h and more recently, 3C-based technologies have shown that circadian genes establish static and rhythmic contacts with enhancers. However, the precise mechanisms by which the clock modulates gene topology are yet to be fully characterized and at the frontier of chronobiology. Here we review evidence of the proximal and long-distance epigenetic mechanisms controlling circadian transcription in health and disease.
Proximal control of circadian gene expression
Temporal control of physiology at short time scales such as the circadian cycle, requires resonance between environmental cues carrying the geophysical time and the expression of genes in time and space. Much of our understanding of the circadian rhythms in transcription relies on the cell-autonomous transcriptional-translational feedback loop (TTFL) mechanism that sustains circadian transcription at promoters of circadian genes (Rosbash, 2009).
In mammals, the cell-autonomous circadian clock consists of interlocking TTFLs. In the main loop, the transcription factors (TFs), BMAL1, and CLOCK form an activator complex that occupies gene promoters containing E-box elements (Gekakis et al., 1998). This event drives the expression of the repressors Period (Per1, Per2, and Per3) and Cryptochrome (Cry1 and Cry2) and hundreds of cell-type-specific rhythmic genes called clock-controlled genes (CCGs). After being transcribed, the messengers of Per and Cry leave the nucleus to access the cytosol for protein synthesis. Afterwards, PER and CRY proteins suffer posttranslational modifications that enable them to interact, forming a repressive complex that, a later stage of the cycle, comes back to the nucleus to arrest the BMAL-CLOCK complex activity, consequently interrupting their-own transcription (Lee et al., 2001). This feedback loop exhibits an almost 24-h periodicity, driving circadian rhythms in transcription.
The BMAL1-CLOCK complex also controls the expression of the orphan nuclear receptors REV-ERBα/β, and RORα/β/γ that cooperatively form a second loop (Partch et al., 2014). With opposite effects on gene expression, these TFs compete for the same RORE motif at Bmal1 and Clock gene promoters (Preitner et al., 2002). Therefore, their actions are influenced by the relative abundance of their protein products throughout the 24 h of the day. In the mouse liver, REV-ERB-α binds to the Bmal1 gene promoter around ZT10 (ZT stands for Zeitgeber Time, ZT0 indicates the beginning of the light phase, and ZT12 indicates the beginning of the dark phase) and decreases its transcriptional activity by recruiting the repressive complex HDAC3-NCoR (Preitner et al., 2002; Yin and Lazar., 2005). In contrast, at the opposite time of the day (ZT22), RORα binds the same gene promoter and increases its transcriptional activity (Preitner et al., 2002). Overall, the opposite effects of these nuclear receptors at the same regulatory elements strengthen the TTFL mechanism by controlling the rhythmic transcription of Bmal1.
In addition, the CCGs Dbp and Nfil3 (also known as E4bp4) coordinate a third transcriptional feedback loop. Like REV-ERBs and RORs, DBP, and NFIL3 compete for the same D-box motif at thousands of regulatory elements across the genome and induce opposite transcriptional effects at contrasting times of the day (Mitsui et al., 2001; Yoshitane et al., 2019). In the mouse liver, DBP gene targets encompass many enzymes and regulators involved in xenobiotic detoxification and drug metabolism (Gachon et al., 2006). Altogether, the interconnected activities of these feedback loops drive circadian rhythms in the transcription of hundreds of CCGs that cover a continuum of peak times throughout the day.
Microarray and RNA-seq studies in mice tissues have identified that 5%–25% of the protein-coding transcriptome in the body correspond to CCGs (Zhang et al., 2014). Although the core clock machinery is identical in all cells of the body, circadian transcriptomes are tissue-specific and present little overlap (Mure et al., 2018) suggesting that additional tissue-specific mechanisms contribute to rhythmic gene transcription. In this context recent evidence suggests that the synergistic actions of tissue-specific TFs with the clock machinery at regulatory sequences and chromatin loops connecting circadian genes with tissue-specific enhancers, are essential factors for the control of tissue-specific transcriptomes (Yeung et al., 2018; Beytebiere et al., 2019).
Distal control of circadian gene expression
Enhancers
Enhancers are short (0.2–1.5 kb) DNA sequences that increase the transcription fire rate of their target gene promoter. This class of regulatory elements functions as command centers for signal transduction systems that sense, integrate and transmit regulatory cues to gene-promoters to orchestrate appropriate transcriptional programs in time and space (Panigrahi and O'Malley, 2021). For this task enhancers contain clusters of general and cell type-specific transcription factor binding motifs that enable them to be active in specific cell types at different times (Plank and Dean., 2014).
In the 80 s, the Schaffner laboratory described the first enhancer as a DNA sequence contained in the SV-40 virus that dramatically boosted the rabbit β-globin gene expression in reporter assays (Banerji et al., 1981; Schaffner, 2015). Soon after, the same group discovered the first mammalian enhancer inside an intron of the mouse immunoglobulin heavy chain gene (Banerji et al., 1983). Since then, millions of enhancers have been identified in mammalian genomes and our understanding of their epigenetic features and their mechanisms of action has progressed significantly. However many questions regarding the molecular selectivity of enhancers remain open and are at the heart of the gene regulation field.
Advances in high-throughput DNA sequencing technologies have enabled the precise annotation of active enhancer elements in different organisms based on their epigenomic features at genomic scale. These features include residence in cell type-specific DNase I hypersensitive sites (DHSs) flanked by nucleosomes containing H3K4me1 and H3K27ac histone marks and enrichment of cofactors like p300 and MED1 (Gross and Garrard, 1988; Heintzman et al., 2009; Andersson and Sandelin, 2020). In addition, recent works have described that active enhancers exhibit bi-directional transcription by the RNA polymerase II machinery leading to the production of enhancer RNAs (eRNAs), whose role in gene regulation is still under investigation. (de Santa et al., 2010). Even though enhancers can be hundreds or thousands of kb from their target genes, these elements engage in physical contact with them forming chromatin-protein complexes through chromatin looping. Protein-protein interactions between compatible TFs and architectural proteins located at the enhancer-promoter anchors have been found to mediate these chromatin loops in a cell type-specific manner (Wang et al., 2021). Additionally, studying the tridimensional organization of the genome has provided essential insights into enhancer biology and their selectivity (revised in Schoenfelder and Fraser, 2019).
Circadian enhancers
Pioneer studies performing H3K27ac ChIP-seq experiments throughout the 24 h in the mouse liver identified a set of functional enhancers exhibiting circadian rhythms in their activity (Koike et al., 2012; Vollmers et al., 2012). Although these seminal reports restricted the assignment of enhancer target genes based on their linear proximity in the genome, they provided novel insights into the participation of enhancers in controlling circadian gene transcription.
According to work by Koike et al. (2012) the H3K27ac histone mark peaks globally around the middle of the night, in phase-coherence with the peaks of the RNA Pol II occupancy and the pre-messenger RNA signal. This evidence is consistent with DNase I hypersensitivity experiments around the clock, showing that the DHSs globally peak in phase with the RNA Pol II loading and the H3K27ac mark (Sobel et al., 2017). These findings collectively suggest a genome-wide regulation of circadian transcription and additional work will be needed to confirm this hypothesis.
Another work measuring the H3K27ac deposition throughout the 24 h in mouse liver shows that roughly 30% of oscillating enhancers were located at less than 200 kb of oscillating genes peaking in synchrony with them. Furthermore, oscillating genes positioned near circadian enhancers displayed higher amplitude than oscillating genes located far from these elements, contributing to the characterization of enhancers in boosting rhythms in gene transcription (Vollmers et al., 2012). In addition, this study also identified rhythmic enhancers located inside clusters of circadian genes coordinating essential liver physiological processes. Interestingly, the peak activity of these regulatory elements measured through the accumulation of H3K27ac, also temporally correlates with the circadian expression of the gene cluster, emphasizing their role in the temporal control of physiology (Vollmers et al., 2012).
Additional work measuring nascent transcription around the clock in mouse liver identified that 30% of functional enhancers display circadian rhythms in their transcriptional activity producing rhythmic eRNAs (Fang et al., 2014). In contrast with the mRNAs of circadian genes, circadian eRNAs show a non-homogeneous distribution of their peak production over the 24 h with most circadian enhancers peaking around dawn (Fang et al., 2014). This evidence suggests that there are different regulatory requirements depending on the circadian gene transcriptional phase. Additionally, this study identified core-clock and tissue-specific TFs enriched in every enhancer group. Consistent with the core clock’s peak binding at the whole-genome scale (Koike et al., 2012), BMAL1, CLOCK, and NPAS2 occupied the ZT6-ZT9 enhancers, the D-box binding factor E4BP4 bound the ZT9-ZT15 enhancers and the nuclear receptors REV-ERB-α and ROR-α occupied the ZT21-ZT24 enhancers. Although FOXA1 and HNF4A did not display any preference for a specific group, their motifs were present in all enhancers. Moreover, the binding motifs for the ETS TF were enriched in the ZT0-ZT3 group, shedding light on their possible role in circadian biology in the mouse liver (Fang et al., 2014). In line with this, it has been identified that roughly 30% of BMAL1 binding sites in the mouse genome, map to oscillating enhancers (Rey et al., 2011; Vollmers et al., 2012). Also, experiments performed in Rev-erb-alpha −/− knockout mice demonstrate that REV-ERB-alpha regulates the expression of hundreds of genes by controlling the activity of their in-phase neighbor enhancer (Fang et al., 2014).
Collectively, these findings uncover circadian rhythms in enhancer activity. However, the precise mechanisms by which the molecular clock generates circadian rhythms in gene transcription through controlling enhancer activity are not yet completely understood.
Circadian rhythms in the three-dimensional organization of the genome
Using circular chromosome conformation capture on chip (4C on-chip) on DEX-synchronized mouse embryonic fibroblast (MEFs), Aguilar-Arnal et al. (2013) identified rhythmic contacts in trans between the Dbp gene and large regions of chromosomes (∼130 kb). Interestingly, the highest frequency of these interactions coincides with the peak of the Dbp mRNA, and Bmal 1 deficient MEFs lost the rhythmicity of these contacts (Aguilar-Arnal et al., 2013).
In line with this, high-resolution chromosome conformation capture experiments (4C-seq) on mouse livers collected at opposite phases of the day (ZT08 and ZT20) placing the bait at the transcriptional start site (TSS) of the Cry1 gene, identified that the Cry1 gene promoter exhibits a rhythmic spatial contact with a 26 kb downstream intronic enhancer.
This interaction was detected at ZT20 in phase-coherence with Cry1 messenger RNA peak production, and genetic ablation of Bmal1 abolished their rhythmicity.
Furthermore, the Cry1 intronic enhancer contained RORE motifs, and the deletion of this element shortened the spontaneous mouse locomotor activity period and reduced the transcriptional burst frequency of the Cry1 promoter. This finding sheds light on the role of non-coding DNA regulatory elements in the temporal control of physiology (Mermet et al., 2018).
Structural proteins such as CTCF, YY1, the cohesin complex and tissue-specific transcription factors bring distant regulatory elements into close spatial proximity through chromatin looping (Nora et al., 2017; Rao et al., 2017; Weintraub et al., 2017). The emergent data showing that dynamic enhancer-promoter contacts lose their rhythmicity in clock deficient animals suggests that components of the core clock machinery also could modulate chromatin looping. In this context, a report using high-throughput chromosome conformation capture (Hi-C) experiments on mouse livers at opposite times of the day identified rhythmic chromatin loops connecting functional REV-ERB-α binding sites with gene promoters at ZT22, which is the opposite phase of the global REV-ERB-α binding at ZT10. Gene ablation and overexpression experiments further confirmed that REV-ERB-α opposes chromatin loops by recruiting the NCoR-HDAC3 complex and avoiding BRD4 and MED1 occupancy (Kim et al., 2018).
In contrast, another report using circular chromosome conformation capture (4C-seq) experiments at opposite circadian times (CT6 vs. CT18, the Circadian Time is a standard marker of time that is based upon the free-running period of an oscillation or rhythm. By convention, circadian time 0 (CT0) is defined as the initiation of the stimulus or activity), identified a stable hub of interactions connecting a BMAL1 bound super-enhancer with the CCG promoters of Rev-erb-α, Med24 and Thra (Xu et al., 2016). This suggests that in addition to modulating the frequency of regulatory interactions at the most appropriate time of the day the clock can take advantage of a pre-established chromatin conformation to boost rhythms in gene transcription.
A global interrogation of gene promoter topology around the clock by promoter capture Hi-C (P-CHi-C) and Hi-C experiments, provided new insights into the dynamics of chromatin loops throughout the 24 h at different genomic scales (Furlan-Magaril et al., 2021). Consistent with previous evidence, this study shows that circadian gene promoters engage in rhythmic and stable promoter-promoter and promoter-enhancer interactions throughout the day. In this context, circadian gene promoters peaking at roughly the same time of the day preferentially engage in physical contact, forming promoter-promoter contacts (Furlan-Magaril et al., 2021). This finding is illustrated in a time-resolved 4C-seq experiment focused on the locus of the CCG gene Por, which encodes the enzyme cytochrome P450 oxidoreductase, an important enzyme in the metabolism of steroid hormones and xenobiotics. Por gene establishes a stable chromatin hub connecting with other CCG genes including Rhbdd2, Tmem120a, Styxl1, and Mdh2 all peaking in synchrony towards the end of the day (Mermet et al., 2021).
In addition, circadian gene promoters also establish rhythmic and stable contacts with regulatory enhancers throughout the 24 h. Like in promoter-promoter interactions, circadian promoters preferentially established interactions with fluctuating-active enhancers in synchrony with them (Furlan-Magaril et al., 2021).
Moreover, consistent with previous findings, circadian gene promoters recruited their maximal number of contacts at roughly the same time as their peak in pre-mRNA production. Remarkably, the core-clock and clock-controlled gene promoters presented significant differences in the dynamics of their interactomes. While the core-clock gene promoters establish fewer interactions with enhancers, these are more dynamic. In contrast, the clock-controlled gene promoters establish more interactions, and a significant fraction of these remain stable throughout the 24 h (Furlan-Magaril et al., 2021).
High-resolution experiments illustrate this finding by showing that the Bmal1 promoter establishes rhythmic contacts with 40 and 75 kb downstream enhancers around ZT22, the time of the day at which Bmal1 pre-mRNA reaches its maximal production. In contrast, the promoter of the CCG Nampt establishes contacts with 50 and 125 kb upstream enhancers that remain stable during the day. However, the activity of these elements was rhythmic, peaking at ZT10 in phase-coherence with the peak of the Nampt pre-mRNA (Figure 1) (Mermet et al., 2021).
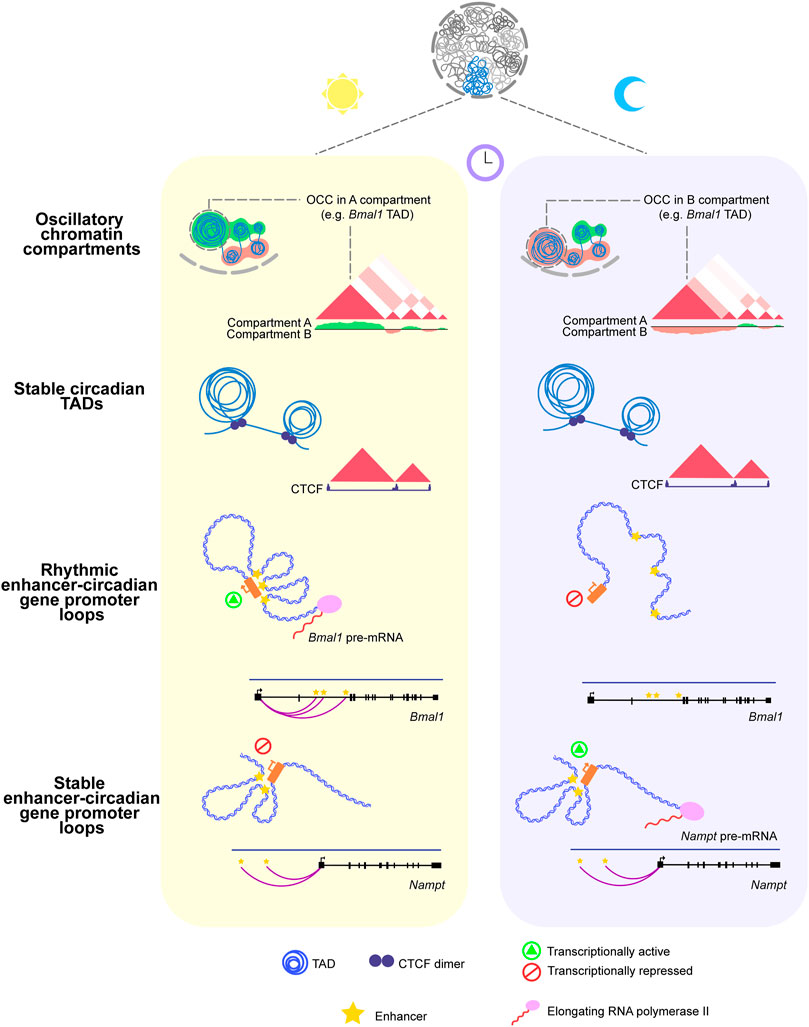
FIGURE 1. Circadian rhythms in the three-dimensional organization of the genome. Upper panel: Oscillatory Chromatin Compartments switch their assignment (A,B) between the onset of the light (ZT0) and dark (ZT12) phases of the day. Middle panel: Circadian TADs remain stable throughout the 24 h of the day. Also, the occupancy of CTCF at their boundaries remains stable over the 24 h. Lower panel: The core-clock gene Bmal1 promoter establishes rhythmic contacts with regulatory elements in phase-coherence with the maximal production of its pre-mRNA. The clock-controlled gene Nampt promoter engages in physical contact with two upstream enhancers that remain stable around the day, even though its pre-mRNA peaks at the dark phase.
Different proportions of stable and dynamic chromatin loops connecting gene promoters with their regulatory elements have also been identified in other biological contexts, such as terminal differentiation of human somatic cells and during Drosophila melanogaster embryogenesis (Ghavi-Helm et al., 2014; Rubin et al., 2017). Thus the combination of dynamic and pre-established chromatin loops might be a conserved mechanism that primes gene promoters for activation at a later stage of development, differentiation, or time of the day. Single molecule resolution experiments will be important to discriminate between dynamic states in just a single allele versus a population of them.
In mammals, the genome is partitioned into megabase-sized structures called Topologically Associating Domains (TADs), whose boundaries contain insulator sequences segregating the interactions inside the domain from the neighbor TADs (Dixon et al., 2012). By facilitating interactions between genes and their regulatory elements in the same domain, TADs provide a framework for the communication between regulatory elements (Lupiañez et al., 2015; Symmons et al., 2016).
Recent studies performing Hi-C experiments around the clock have identified TADs containing circadian genes (cTADs) (Figure 1) (Kim et al., 2018; Furlan-Magaril et al., 2021). Consistent with previous findings, cTAD boundaries and the occupancy of the architectural protein CTCF at them remain stable during the day (Kim et al., 2018; Furlan-Magaril et al., 2021). In addition, circadian genes residing inside the same cTAD display their peak pre-mRNA accumulation at roughly the same time of the day; this finding illustrates the role of TADs in isolating temporal transcriptional programs (Furlan-Magaril et al., 2021).
At a larger scale (∼100 Mb), the genome is organized into active A and inactive B compartments. High throughput chromosome conformation capture (Hi-C) experiments around the clock showed that 17% of the genome switches its compartment assignment between open and closed chromatin states during the day. A large fraction of these oscillatory chromatin compartments (OCCs) switched between open to closed (A to B) chromatin states at ZT12 while remaining in A for the rest of the day (Figure 1) (Furlan-Magaril et al., 2021).
Furthermore, 70% of cTADs reside within OCCs and switch their compartment assignment throughout the 24 h. A detailed inspection of the Npas2 gene TAD exemplifies this finding by showing that the domain switches from transcriptionally active to repressive at ZT12, in synchrony with the nadir of the Npas2 pre-mRNA (Furlan-Magaril et al., 2021). These findings are consistent with studies interrogating chromosome conformation in other cellular processes such as cell differentiation and organism development, showing that while TADs remain invariable, chromatin compartments are more plastic and dynamic (Dixon et al., 2015; Bonev et al., 2017; Rubin et al., 2017).
Collectively, these evidences show circadian rhythms in different layers of genome organization.
Distal regulation of circadian transcription in metabolic disease
Metabolic diseases represent a disruption of the complex link between metabolism and circadian rhythms. In the murine liver, nutritional challenges such as those imposed by the high-fat diet (HFD) lead to a global remodeling of the circadian transcriptome. In this scenario, some oscillating genes lose their rhythmicity; others present phase delays or phase advances, and strikingly, a subset of non-oscillating genes acquire oscillations in their transcriptional activity (Eckel-Mahan et al., 2013).
A study measuring nascent transcription around the clock in the mouse liver of obese mice shows a remodeling in the activity of thousands of circadian enhancers (Guan et al., 2018). Interestingly, a significant fraction of these elements peaked around ZT10 and were driven by the master regulators SREBP and PPAR-α, whose activity became rhythmic in obese mice. The daily activity of enhancers in obesity induces the temporal coexistence of gene expression programs enriched for the de novo lipogenesis (DNL) and fatty acid oxidation pathways (FAO) that in normal mice are segregated at opposite times of the day (Guan et al., 2018).
Recent work interrogating the effects of nutritional challenges on the dynamics of chromosome conformation has furthered our understanding of the long-range epigenetic mechanisms behind transcriptional remodeling in obesity. According to, Qin et al. (2020) while compartments and TADs were insensitive to nutritional challenges, chromatin loops showed two modulation mechanisms: the activation of pre-established chromatin loops and, to a lesser extent, the newly created enhancer-promoter contacts. Additional experiments revealed that the HNF4A receptor increased its occupancy at the enhancers of upregulated genes. In addition, other recent studies have uncovered the role of this receptor in regulating the activity of the liver clock (Qu et al., 2018, Qu et al., 2021). In this regard HNF4A emerges as a candidate for mediating aberrant enhancer-promoter contacts and an attractive target for pharmacological modification in obesity.
Closing remarks
The evidence reviewed here exposes the function of distal regulatory elements in controlling circadian gene transcription together with rhythmic fluctuations in genome spatial organization at different scales. Whether components of the molecular clock, tissue-specific TFs, architectural proteins, or a combination drive the rhythms in chromatin conformation will have to be further characterized. Future experiments using genetic and pharmacological approaches will illuminate essential mechanisms by which the clock controls genome topology. This knowledge will contribute to better understand the mechanisms behind transcriptional programs remodeling in diseases with circadian-misalignment such as obesity, to identify new therapeutic targets.
Author contributions
MF-M, AR-F, and LT-H conceptualize and defined the content of the mini review. AR-F wrote the manuscript with help and contributions from MF-M and LT-H designed and executed the figure and commented on the manuscript.
Funding
AR-F and LT-H are supported by CONACyT scholarships (708191 and 1101439 respectively). This work is supported by CONACyT grant numbers 15758 and 303068.
Conflict of interest
The authors declare that the research was conducted in the absence of any commercial or financial relationships that could be construed as a potential conflict of interest.
Publisher’s note
All claims expressed in this article are solely those of the authors and do not necessarily represent those of their affiliated organizations, or those of the publisher, the editors and the reviewers. Any product that may be evaluated in this article, or claim that may be made by its manufacturer, is not guaranteed or endorsed by the publisher.
References
Aguilar-Arnal, L., Hakim, O., Patel, V. R., Baldi, P., Hager, G. L., and Sassone-Corsi, P. (2013). Cycles in spatial and temporal chromosomal organization driven by the circadian clock. Nat. Struct. Mol. Biol. 20, 1206–1213. doi:10.1038/nsmb.2667
Andersson, R., and Sandelin, A. (2020). Determinants of enhancer and promoter activities of regulatory elements. Nat. Rev. Genet. 21, 71–87. doi:10.1038/s41576-019-0173-8
Banerji, J., Olson, L., and Schaffner, W. (1983). A lymphocyte-specific cellular enhancer is located downstream of the joining region in immunoglobulin heavy chain genes. Cell 33, 729–740. doi:10.1016/0092-8674(83)90015-6
Banerji, J., Rusconi, S., and Schaffner, W. (1981). Expression of a β-globin gene is enhanced by remote SV40 DNA sequences. Cell 27, 299–308. doi:10.1016/0092-8674(81)90413-X
Beytebiere, J. R., Trott, A. J., Greenwell, B. J., Osborne, C. A., Vitet, H., Spence, J., et al. (2019). Tissue-specific BMAL1 cistromes reveal that rhythmic transcription is associated with rhythmic enhancer–enhancer interactions. Genes Dev. 33, 294–309. doi:10.1101/gad.322198.118
Bonev, B., Mendelson Cohen, N., Szabo, Q., Fritsch, L., Papadopoulos, G. L., Lubling, Y., et al. (2017). Multiscale 3D genome rewiring during mouse neural development. Cell 171, 557–572. doi:10.1016/j.cell.2017.09.043
de Santa, F., Barozzi, I., Mietton, F., Ghisletti, S., Polletti, S., Tusi, B. K., et al. (2010). A large fraction of extragenic RNA Pol II transcription sites overlap enhancers. PLoS Biol. 8, e1000384. doi:10.1371/journal.pbio.1000384
Dixon, J. R., Jung, I., Selvaraj, S., Shen, Y., Antosiewicz-Bourget, J. E., Lee, A. Y., et al. (2015). Chromatin architecture reorganization during stem cell differentiation. Nature 518, 331–336. doi:10.1038/nature14222
Dixon, J. R., Selvaraj, S., Yue, F., Kim, A., Li, Y., Shen, Y., et al. (2012). Topological domains in mammalian genomes identified by analysis of chromatin interactions. Nature 485, 376–380. doi:10.1038/nature11082
Eckel-Mahan, K. L., Patel, V. R., de Mateo, S., Orozco-Solis, R., Ceglia, N. J., Sahar, S., et al. (2013). Reprogramming of the circadian clock by nutritional challenge. Cell 155, 1464–1478. doi:10.1016/j.cell.2013.11.034
Fang, B., Everett, L. J., Jager, J., Briggs, E., Armour, S. M., Feng, D., et al. (2014). Circadian enhancers coordinate multiple phases of rhythmic gene transcription in vivo. Cell 159, 1140–1152. doi:10.1016/j.cell.2014.10.022
Furlan-Magaril, M., Ando-Kuri, M., Arzate-Mejía, R. G., Morf, J., Cairns, J., Román-Figueroa, A., et al. (2021). The global and promoter-centric 3D genome organization temporally resolved during a circadian cycle. Genome Biol. 22, 162. doi:10.1186/s13059-021-02374-3
Gachon, F., Olela, F. F., Schaad, O., Descombes, P., and Schibler, U. (2006). The circadian PAR-domain basic leucine zipper transcription factors DBP, TEF, and HLF modulate basal and inducible xenobiotic detoxification. Cell Metab. 4, 25–36. doi:10.1016/j.cmet.2006.04.015
Gekakis, N., Staknis, D., Nguyen, H. B., Davis, F. C., Wilsbacner, L. D., King, D. P., et al. (1998). Role of the CLOCK protein in the mammalian circadian mechanism. Science 280, 1564–1569. doi:10.1126/science.280.5369.1564
Ghavi-Helm, Y., Klein, F. A., Pakozdi, T., Ciglar, L., Noordermeer, D., Huber, W., et al. (2014). Enhancer loops appear stable during development and are associated with paused polymerase. Nature 512, 96–100. doi:10.1038/nature13417
Gross, D. S., and Garrard, W. T. (1988). Nuclease hypersensitive sites in chromatin. Annu. Rev. Biochem. 57, 159–197. doi:10.1146/annurev.bi.57.070188.001111
Guan, D., Xiong, Y., Borck, P. C., Jang, C., Doulias, P. T., Papazyan, R., et al. (2018). Diet-induced circadian enhancer remodeling synchronizes opposing hepatic lipid metabolic processes. Cell 174, 831–842. doi:10.1016/j.cell.2018.06.031
Heintzman, N. D., Hon, G. C., Hawkins, R. D., Kheradpour, P., Stark, A., Harp, L. F., et al. (2009). Histone modifications at human enhancers reflect global cell-type-specific gene expression. Nature 459, 108–112. doi:10.1038/nature07829
Kim, Y. H., Marhon, S. A., Zhang, Y., Steger, D. J., Won, K. J., and Lazar, M. A. (2018). Rev-erbα dynamically modulates chromatin looping to control circadian gene transcription. Science 359, 1274–1277. doi:10.1126/science.aao6891
Koike, N., Yoo, S. H., Huang, H. C., Kumar, V., Lee, C., Kim, T. K., et al. (2012). Transcriptional architecture and chromatin landscape of the core circadian clock in mammals. Science 338, 349–354. doi:10.1126/science.1226339
Lee, C., Etchegaray, J. P., Cagampang, F. R. A., Loudon, A. S. I., and Reppert, S. M. (2001). Posttranslational mechanisms regulate the mammalian circadian clock. Cell 107, 855–867. doi:10.1016/S0092-8674(01)00610-9
Lupiáñez, D. G., Kraft, K., Heinrich, V., Krawitz, P., Brancati, F., Klopocki, E., et al. (2015). Disruptions of topological chromatin domains cause pathogenic rewiring of gene-enhancer interactions. Cell 161, 1012–1025. doi:10.1016/j.cell.2015.04.004
Mermet, J., Yeung, J., Hurni, C., Mauvoisin, D., Gustafson, K., Jouffe, C., et al. (2018). Clock-dependent chromatin topology modulates circadian transcription and behavior. Genes Dev. 32, 347–358. doi:10.1101/gad.312397.118
Mermet, J., Yeung, J., and Naef, F. (2021). Oscillating and stable genome topologies underlie hepatic physiological rhythms during the circadian cycle. PLoS Genet. 17, e1009350. doi:10.1371/JOURNAL.PGEN.1009350
Mitsui, S., Yamaguchi, S., Matsuo, T., Ishida, Y., and Okamura, H. (2001). Antagonistic role of E4BP4 and PAR proteins in the circadian oscillatory mechanism. Genes Dev. 15, 995–1006. doi:10.1101/gad.873501
Mure, L. S., Le, H. D., Benegiamo, G., Chang, M. W., Rios, L., Jillani, N., et al. (2018). Diurnal transcriptome atlas of a primate across major neural and peripheral tissues. Science 359, eaao0318. doi:10.1126/science.aao0318
Nora, E. P., Goloborodko, A., Valton, A. L., Gibcus, J. H., Uebersohn, A., Abdennur, N., et al. (2017). Targeted degradation of CTCF decouples local insulation of chromosome domains from genomic compartmentalization. Cell 169, 930–944. doi:10.1016/j.cell.2017.05.004
Panigrahi, A., and O’Malley, B. W. (2021). Mechanisms of enhancer action: The known and the unknown. Genome Biol. 22, 108. doi:10.1186/s13059-021-02322-1
Partch, C. L., Green, C. B., and Takahashi, J. S. (2014). Molecular architecture of the mammalian circadian clock. Trends Cell Biol. 24, 90–99. doi:10.1016/j.tcb.2013.07.002
Plank, J. L., and Dean, A. (2014). Enhancer function: Mechanistic and genome-wide insights come together. Mol. Cell 55, 5–14. doi:10.1016/j.molcel.2014.06.015
Preitner, N., Damiola, F., Zakany, J., Duboule, D., Albrecht, U., Schibler, U., et al. (2002). The orphan nuclear receptor REV-ERBalpha controls circadian transcription within the positive limb of the mammalian circadian oscillator. Cell 110, 251–260. doi:10.1016/s0092-8674(02)00825-5
Qin, Y., Grimm, S. A., Roberts, J. D., Chrysovergis, K., and Wade, P. A. (2020). Alterations in promoter interaction landscape and transcriptional network underlying metabolic adaptation to diet. Nat. Commun. 11, 962. doi:10.1038/s41467-020-14796-x
Qu, M., Duffy, T., Hirota, T., and Kay, S. A. (2018). Nuclear receptor HNF4A transrepresses CLOCK: BMAL1 and modulates tissue-specific circadian networks. Proc. Natl. Acad. Sci. U. S. A. 115, E12305–E12312. doi:10.1073/pnas.1816411115
Qu, M., Qu, H., Jia, Z., and Kay, S. A. (2021). HNF4A defines tissue-specific circadian rhythms by beaconing BMAL1:CLOCK chromatin binding and shaping the rhythmic chromatin landscape. Nat. Commun. 12, 6350. doi:10.1038/s41467-021-26567-3
Rao, S. S. P., Huang, S. C., Glenn St Hilaire, B., Engreitz, J. M., Perez, E. M., Kieffer-Kwon, K. R., et al. (2017). Cohesin loss eliminates all loop domains. Cell 171, 305–320. doi:10.1016/j.cell.2017.09.026
Rey, G., Cesbron, F., Rougemont, J., Reinke, H., Brunner, M., and Naef, F. (2011). Genome-wide and phase-specific DNA-binding rhythms of BMAL1 control circadian output functions in mouse liver. PLoS Biol. 9, e1000595. doi:10.1371/journal.pbio.1000595
Rosbash, M. (2009). The implications of multiple circadian clock origins. PLoS Biol. 7, e62. doi:10.1371/journal.pbio.1000062
Rubin, A. J., Barajas, B. C., Furlan-Magaril, M., Lopez-Pajares, V., Mumbach, M. R., Howard, I., et al. (2017). Lineage-specific dynamic and pre-established enhancer-promoter contacts cooperate in terminal differentiation. Nat. Genet. 49, 1522–1528. doi:10.1038/ng.3935
Schaffner, W. (2015). Enhancers, enhancers - from their discovery to today’s universe of transcription enhancers. Biol. Chem. 396, 311–327. doi:10.1515/hsz-2014-0303
Schoenfelder, S., and Fraser, P. (2019). Long-range enhancer–promoter contacts in gene expression control. Nat. Rev. Genet. 20, 437–455. doi:10.1038/s41576-019-0128-0
Sobel, J. A., Krier, I., Andersin, T., Raghav, S., Canella, D., Gilardi, F., et al. (2017). Transcriptional regulatory logic of the diurnal cycle in the mouse liver. PLoS Biol. 15, e2001069. doi:10.1371/journal.pbio.2001069
Symmons, O., Pan, L., Remeseiro, S., Aktas, T., Klein, F., Huber, W., et al. (2016). The shh topological domain facilitates the action of remote enhancers by reducing the effects of genomic distances. Dev. Cell 39, 529–543. doi:10.1016/j.devcel.2016.10.015
Vollmers, C., Schmitz, R. J., Nathanson, J., Yeo, G., Ecker, J. R., and Panda, S. (2012). Circadian oscillations of protein-coding and regulatory RNAs in a highly dynamic mammalian liver epigenome. Cell Metab. 16, 833–845. doi:10.1016/j.cmet.2012.11.004
Wang, H., Huang, B., and Wang, J. (2021). Predict long-range enhancer regulation based on protein-protein interactions between transcription factors. Nucleic Acids Res. 49, 10347–10368. doi:10.1093/nar/gkab841
Weintraub, A. S., Li, C. H., Zamudio, A. v., Sigova, A. A., Hannett, N. M., Day, D. S., et al. (2017). YY1 is a structural regulator of enhancer-promoter loops. Cell 171, 1573–1588. doi:10.1016/j.cell.2017.11.008
Xu, Y., Guo, W., Li, P., Zhang, Y., Zhao, M., Fan, Z., et al. (2016). Long-range chromosome interactions mediated by cohesin shape circadian gene expression. PLoS Genet. 12, e1005992. doi:10.1371/journal.pgen.1005992
Yeung, J., Mermet, J., Jouffe, C., Marquis, J., Charpagne, A., Gachon, F., et al. (2018). Transcription factor activity rhythms and tissue-specific chromatin interactions explain circadian gene expression across organs. Genome Res. 28, 182–191. doi:10.1101/gr.222430.117
Yin, L., and Lazar, M. A. (2005). The orphan nuclear receptor Rev-erbalpha recruits the N-CoR/histone deacetylase 3 corepressor to regulate the circadian Bmal1 gene. Mol. Endocrinol. 19, 1452–1459. doi:10.1210/me.2005-0057
Yoshitane, H., Asano, Y., Sagami, A., Sakai, S., Suzuki, Y., Okamura, H., et al. (2019). Functional D-box sequences reset the circadian clock and drive mRNA rhythms. Commun. Biol. 2, 300. doi:10.1038/s42003-019-0522-3
Keywords: circadian rhythms, clock controlled genes, chromatin, enhancers, 3D genome, gene expression
Citation: Román-Figueroa A, Tenorio-Hernández L and Furlan-Magaril M (2022) Distal and proximal control of rhythmic gene transcription. Front. Syst. Biol. 2:1026424. doi: 10.3389/fsysb.2022.1026424
Received: 23 August 2022; Accepted: 04 October 2022;
Published: 18 October 2022.
Edited by:
Argyris Papantonis, University Medical Center Göttingen, GermanyReviewed by:
Jianrong Wang, Michigan State University, United StatesKevin Van Bortle, University of Illinois at Urbana-Champaign, United States
Copyright © 2022 Román-Figueroa, Tenorio-Hernández and Furlan-Magaril. This is an open-access article distributed under the terms of the Creative Commons Attribution License (CC BY). The use, distribution or reproduction in other forums is permitted, provided the original author(s) and the copyright owner(s) are credited and that the original publication in this journal is cited, in accordance with accepted academic practice. No use, distribution or reproduction is permitted which does not comply with these terms.
*Correspondence: Mayra Furlan-Magaril, mfurlan@ifc.unam.mx