- 1Department of Neuroscience, University of Minnesota, Minneapolis, MN, United States
- 2Medical Discovery Team on Addiction, University of Minnesota, Minneapolis, MN, United States
- 3Graduate Program in Neuroscience, University of Minnesota, Minneapolis, MN, United States
Addiction is a complex disease that impacts millions of people around the world. Clinically, addiction is formalized as substance use disorder (SUD), with three primary symptom categories: exaggerated substance use, social or lifestyle impairment, and risky substance use. Considerable efforts have been made to model features of these criteria in non-human animal research subjects, for insight into the underlying neurobiological mechanisms. Here we review evidence from rodent models of SUD-inspired criteria, focusing on the role of the striatal dopamine system. We identify distinct mesostriatal and nigrostriatal dopamine circuit functions in behavioral outcomes that are relevant to addictions and SUDs. This work suggests that striatal dopamine is essential for not only positive symptom features of SUDs, such as elevated intake and craving, but also for impairments in decision making that underlie compulsive behavior, reduced sociality, and risk taking. Understanding the functional heterogeneity of the dopamine system and related networks can offer insight into this complex symptomatology and may lead to more targeted treatments.
Introduction
Addiction is characterized by a transition from recreational drug use to compulsive, disordered use, punctuated by cycles of abstinence, withdrawal, craving, and relapse. Features of human drug use are complicated by social and political factors, including stigmatization, criminalization, and barriers to treatment access. Over the past 30 years, the prevailing scientific consensus has identified addiction as a chronic disease, codified as substance use disorder (SUD). SUDs are characterized by pharmacological effects of tolerance and withdrawal, as well as a core set of behavioral features defined by the Diagnostic and Statistical Manual of Mental Disorders (DSM-5). These can be grouped into three major categories: I. Impaired control of substance use; II. Social impairment; and III. Risky use of substance (Figure 1, top). Significant research efforts have been made to characterize the neurobiological and psychological underpinnings of these behavioral symptoms. The hope is that understanding the basic science behind these behaviors will lead to more effective treatments for SUD, and other psychiatric illnesses with comorbid symptoms (such as compulsive gambling, ADHD, and schizophrenia).
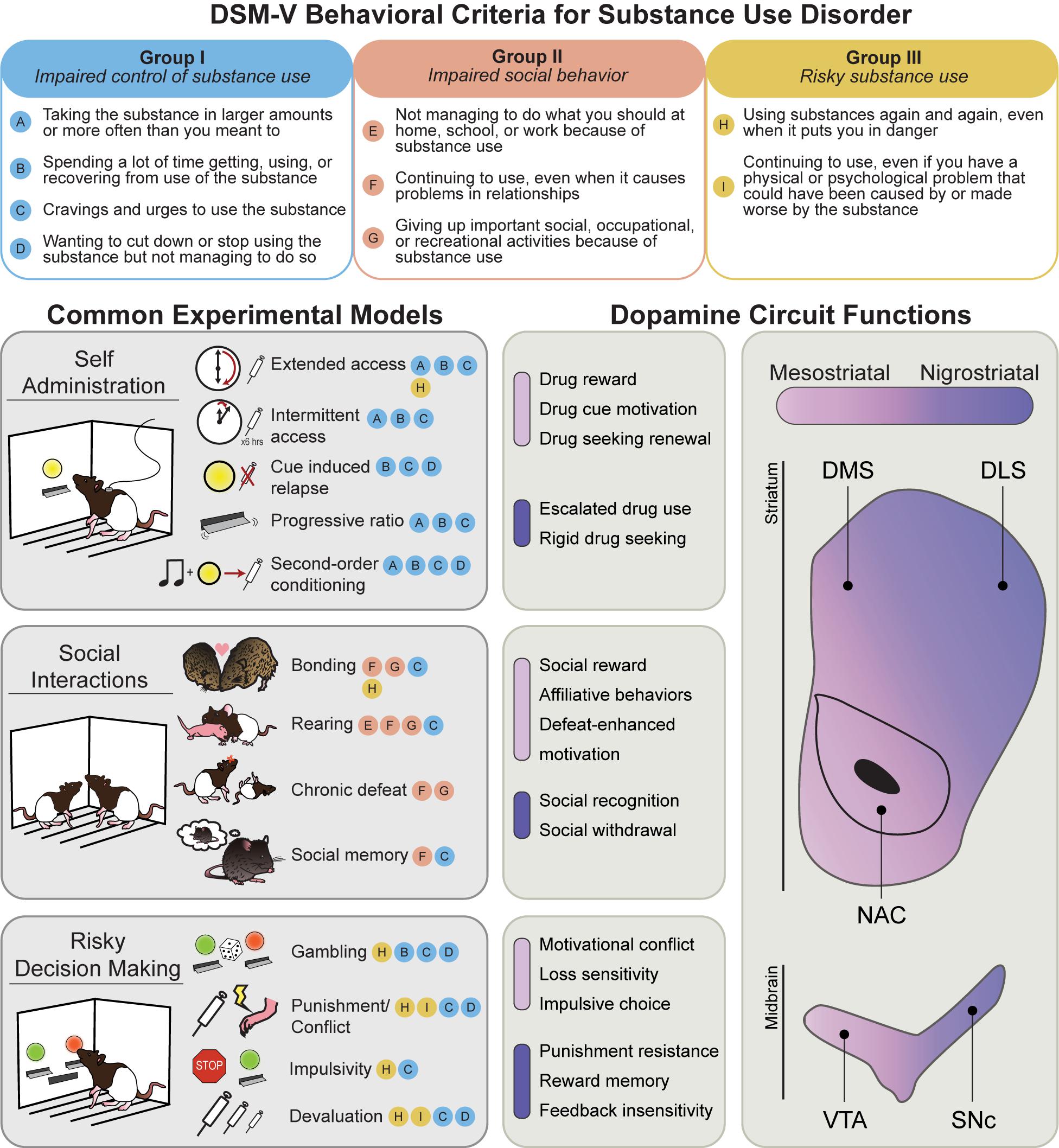
Figure 1. Behavioral models used to classify phenotypes of substance use disorder. (Top) The behavioral criteria of SUDs (circled letters) can be sorted into three main categories: impaired control of substance use (Group I), impaired social behavior (Group II), and risky substance use (Group III). (Left) Common rodent experimental models and the SUD criteria they are thought to best approximate. Note that most models capture multiple SUD features. (Right) Mesostriatal circuits (light purple), including dopamine projections from the ventral tegmental area (VTA) to the nucleus accumbens (NAC), and nigrostriatal circuits (dark purple), including dopamine projections to the dorsomedial (DMS) and dorsolateral striatum (DLS), have generally dissociable roles in different components of major SUD models. In the middle panels, the most clearly defined roles for these two systems in each SUD category are listed.
Research making use of non-human animals is essential to this effort. Leveraging convergent biology of reward learning and decision-making systems across species, addiction scientists have established a variety of animal models to investigate drug-related behaviors (Figure 1). While considerable debate exists surrounding the translational efficacy of individual models to the complexity of human addiction (for recent review, see Venniro et al., 2020), they nonetheless offer powerful experimental insight into neurobehavioral mechanisms that govern core features of drug use.
Among brain systems, dopamine (DA) circuits are a key modulator of behaviors associated with SUDs. Via several mechanisms, including direct excitation of DA neurons (nicotine, alcohol), blockade of terminal DA reuptake (amphetamine, cocaine), and DA neuron disinhibition (opioids and cannabinoids), nearly all drugs used by humans acutely increase signaling of DA within the striatum, and blocking DA receptors decreases the reinforcing effects of many drugs (Wise and Bozarth, 1987; Johnson and North, 1992; Nutt et al., 2015; Volkow et al., 2017; Nestler and Lüscher, 2019; Solinas et al., 2019; Wise and Robble, 2020). The connection between DA and drug use is further supported by in vivo measurements of drug-evoked DA release in human and non-human animal studies (Hernandez et al., 1987; Chiara and Imperato, 1988; Robinson et al., 1988; Pontieri et al., 1995; Ito et al., 2002; Porrino et al., 2004; Volkow et al., 2006; Belin and Everitt, 2008; Willuhn et al., 2012, 2014). Humans with a history of drug use, including those meeting DSM criteria for SUDs, have altered DA system transmission and function (Stewart, 2008; Volkow et al., 2009; Diana, 2011; Leyton and Vezina, 2014; Ikemoto et al., 2015; Leyton, 2017). As such, popular theories of addiction and compulsive behavior are built on the notion of altered activity in the DA system (Robinson and Berridge, 1993; Everitt and Robbins, 2005; Wise, 2009; Keiflin and Janak, 2015; Nestler and Lüscher, 2019). Advances in neuroscience research technology and theory surrounding addiction-like behaviors in animal models of reward seeking have afforded the opportunity to characterize the role of precisely defined brain circuits and regions in behavior. In this review, we will discuss current evidence for regional and circuit-specific functions within the DA system in different aspects of addiction-like behavior, in the context of animal studies derived from DSM criteria for the behavioral features of SUDs.
Dopamine Circuits
Most of the brain’s neurons are in two midbrain regions (Figure 1): the ventral tegmental area (VTA) and substantia nigra pars compacta (SNc). DA neurons in the VTA largely project to the ventral striatum, in particular, the nucleus accumbens (NAc) core and shell, comprising the mesostriatal pathway, and to other frontal targets in the pallidum, amygdala, and prefrontal cortex (Swanson, 1982; Ikemoto, 2007). Intermingled with DA neurons in the VTA are a substantial fraction of GABAergic and glutamatergic neurons (Olson and Nestler, 2007; Nair-Roberts et al., 2008; Bouarab et al., 2019). The SNc, in contrast, contains DA neurons that project almost exclusively to the dorsomedial (DMS) and dorsolateral (DLS) striatum, comprising the nigrostriatal pathway (Beckstead et al., 1979; Swanson, 1982; Fields et al., 2007; Ikemoto, 2007; Britt et al., 2012). At their targets in the striatum, DA neurons primarily contact GABAergic medium spiny neurons (MSNs) that contain excitatory type 1 (D1-MSNs), or inhibitory type 2 (D2-MSNs) DA receptors (Gerfen, 1984; Kupchik et al., 2015). Dopamine’s modulatory influence on striatal activity via these outputs is a predominant mechanism of behavioral control in reward learning and motivation. Notably, many drugs act in the striatum to increase DA release locally, via regionally specific terminal mechanisms (Collins and Saunders, 2020), which plays a key role in heterogeneous mechanisms of drug use, craving, and relapse behaviors that underlie features of SUDs (Koob and Bloom, 1988; Ahmed and Koob, 1998; Lobo et al., 2010; Thompson et al., 2010; Oliver et al., 2019).
Dopamine neurons across VTA and SNc circuits exhibit considerable heterogeneity with respect to behavioral function (Figure 1; Björklund and Dunnett, 2007; Lammel et al., 2014; Morales and Margolis, 2017; Cox and Witten, 2019; Collins and Saunders, 2020). In the classic framework, mesostriatal DA neurons contribute to learning and execution of goal-directed behaviors, while nigrostriatal DA, especially in the DLS, is involved in movement control and the execution of rigid, habitual actions (Haber et al., 2000; Hassani et al., 2001; Everitt, 2014; Burton et al., 2015; Saunders et al., 2018; Cox and Witten, 2019). From an extensive literature, deficits in VTA and SNc DA signaling typically impair learning and reward-directed behaviors, or movement planning, execution and vigor, respectively. Exaggerated VTA and SNc DA signaling, conversely, underlies compulsive motivation and behavioral inflexibility (Robinson and Berridge, 1993; Cardinal et al., 2002; Everitt and Robbins, 2005; Wise, 2005). In the context of Pavlovian learning, sensory cues associated with increased VTA DA neuron activity evoke approach behavior and acquire value that supports second-order conditioning of instrumental actions, which is critical for persistent and adaptive reward pursuit (Berridge, 2007; Flagel et al., 2011; Saunders and Robinson, 2012; Saunders et al., 2018). Nigrostriatal DA neurons, especially those projecting to the DMS, are important for linking instrumental actions with outcomes they produce (Yin et al., 2005). Further, activation of SNc DA neurons evokes movement, and their activity encodes movement initiation (Dodson et al., 2016; Coddington and Dudman, 2018; da Silva et al., 2018), suggesting they contribute more generally to movement invigoration. VTA DA neuron activity and release in the NAc is in contrast engaged when animals emit cue- or goal-directed movements (Carelli, 2004; Burton et al., 2015; Howe and Dombeck, 2016; Mohebi et al., 2019). As such, mesostriatal DA can be conceptualized as generating a motivational “pull” to cues and the rewards they predict, while nigrostriatal DA provides a “push” that underlies general behavioral invigoration or arousal (Bolles, 1967; Ostlund, 2019). Thus, while dissociable, normal activity in these parallel circuits is necessary for successful reward seeking and reward-based decision making (Arias-Carrión and Pǒppel, 2007; Aarts et al., 2011; Hsu et al., 2018; Le Heron et al., 2018, 2020; Cox and Witten, 2019; Collins and Saunders, 2020).
Via its roles in signaling expectation, value, and action invigoration, DA has strong influence on both goal-directed and habitual actions that result from reward learning. These fundamental behavioral classifications are each maladapted in addiction (Tiffany, 1990; Singer et al., 2018; Ostlund, 2019; Hogarth, 2020; Vandaele and Ahmed, 2021). Given that drugs impinge heavily on DA circuitry, functional, circuit-level differences within the DA system have important implications for the understanding of SUDs. In the following, we will review some of the ways in which mesostriatal and nigrostriatal DA pathways regulate behaviors associated with major SUD criteria. Notably, emerging work highlights that striatal DA is essential not only for features of SUDs characterized by exaggerated behavior, such as drug use and craving, but also for behavioral deficits, including impairments in decision making that underlie compulsive behavior, reduced sociality, and risk taking.
Category I – Impaired Control of Substance Use
A hallmark of SUDs is a progression to impaired control over drug use, associated with increased drug intake, craving, and relapse vulnerability. As such, a major DSM criterion includes behavioral features such as “taking the substance in larger amounts or more often,” “spending a lot of time getting, using, or recovering from use of the substance,” and “cravings and urges to use the substance.” Animal studies of these SUD features are among the most common, leveraging the power of drug self administration models. The self administration models that align best with Category I SUD criteria are shown in Figure 1. Rodents, like humans, will readily self administer most commonly used drugs, such as; opioids, alcohol, cocaine, amphetamine, nicotine, and cannabinoids. In widely used paradigms, rats and mice are trained to engage in behaviors (typically, lever presses, or nose port responses) to receive drug doses delivered intravenously, orally, or via inhalation. Drug-associated cues and contexts, as well as small “priming” drug doses, stress and pain play a central role in promoting and maintaining drug use and relapse (Stewart, 1984; Balster and Lukas, 1985; Goeders and Guerin, 1994; Shaham et al., 2003; Chaudhri et al., 2008; Spanagel, 2017). In relapse models, the resumption of drug seeking following abstinence can be used as a behavioral index of drug “craving”. Notably, drug craving assessed in animal models undergoes “incubation” in the weeks to months following abstinence from many drug types. That is, the longer it has been since the last drug exposure, the greater the probability and intensity of relapse (Grimm et al., 2001; Venniro et al., 2016). This sensitization of the relapse-inducing power of drug cues in particular results in a persistent threat of a return to drug use, a feature of SUDs that is especially difficult to treat.
A major development in rodent addiction models came when it was discovered that giving rats extended access to drugs promotes an escalation of intake, where more drug is taken in a shorter time period, mimicking a central tenet of human SUDs. This has been observed with many drugs, including cocaine, heroin, methamphetamine, alcohol, and nicotine (Ahmed and Koob, 1998; Ahmed et al., 2000; Roberts et al., 2000; Kitamura et al., 2006; O’Dell et al., 2007). Escalation of drug intake following extended access is associated with other addiction-like features, including increased motivation for drug, drug seeking in the face of high effort cost, and seeking despite negative consequences. This approach has since informed a large portion of preclinical addiction research, including attempts to create a DSM-inspired composite addiction phenotype that can be applied to rodents (Deroche-Gamonet et al., 2004; Robinson, 2004; Vanderschuren and Everitt, 2004; O’Neal et al., 2020). More recently, intermittent access drug self administration models have gained attention (Zimmer et al., 2012; Kawa et al., 2019a). In these paradigms, brief periods of drug availability are interspersed with longer drug unavailability periods. This intermittency promotes rapid, binge-like drug intake that may better approximate some human drug use patterns. Intermittent self administration of cocaine, alcohol, and opioids, despite resulting in much less total drug intake compared to extended access models, promotes escalation of intake and elevated drug craving (Simms et al., 2008; Zimmer et al., 2012; Calipari et al., 2013; Kawa et al., 2016; O’Neal et al., 2020; Fragale et al., 2021; Samaha et al., 2021). Binge-like self administration can also develop in rats given extended, continuous access to cocaine, and individual differences in binge patterns early in self administration training predict the intensity of future use (Tornatzky and Miczek, 2000; Belin et al., 2009). Finally, some rodent models have also incorporated a behavioral economics framework to quantify drugs as commodities, to examine choice elasticity and demand (Oleson and Roberts, 2009; Bentzley et al., 2013; Mohammadkhani et al., 2019). This approach is useful for standardization of core behavioral indices related to decision making and motivation, which could facilitate quantitative comparisons across different tasks and reward modalities.
Mesostriatal
Drugs act on DA circuits to promote synaptic plasticity that amplifies VTA activity and DA signaling in the NAc, even following a single exposure (Ungless et al., 2001; Mameli et al., 2009; Pascoli et al., 2012; Ungless and Grace, 2012; Ji et al., 2017; Morel et al., 2019; Thompson et al., 2021). Within the mesostriatal pathway, DA release evoked by drugs occurs via multiple mechanisms that impinge on VTA cells and their axon terminals (Ritz et al., 1987; Thomas et al., 2008; Volkow et al., 2009; Mark et al., 2011; Ford, 2014; Lammel et al., 2014). Given the central role that mesostriatal DA plays in reward learning and behavioral reinforcement (Fouriezos and Wise, 1976; Corbett and Wise, 1980; Witten et al., 2011; Steinberg et al., 2014), this system is key in the control of drug seeking, drug cue-evoked motivation, and craving. Manipulation of VTA DA neuron activity can regulate drug self administration in animal models. One way this has been studied is through manipulation of DA D2 autoreceptors. Activation of these receptors decreases activity of DA neurons and phasic DA release through a negative feedback loop (Schmitz et al., 2003), leading to changes in drug-taking behavior. Dopamine binding on VTA D2 receptors is negatively correlated with cocaine and amphetamine seeking and consumption (Buckholtz et al., 2010; Bello et al., 2011). Further, elevating VTA DA neuron activity via D2 knockdown increases cocaine self administration (Chen et al., 2018) and blocking the negative feedback activity of these receptors increases cocaine self administration (McCall et al., 2017). Additionally, rats with knockdown D2Rs will also work harder for sucrose and cocaine (de Jong et al., 2015). In line with this, reducing DA signaling in VTA neurons blunts cocaine self administration motivation, as measured by impaired behavior on a progressive ratio task (Ranaldi and Wise, 2001). These studies illustrate some ways that alterations in normal signaling with the mesostriatal pathway can alter motivation for drugs. Elevating VTA DA neuron activity can also promote impulsive choice behavior, for example, where rats prefer small, immediate rewards over larger rewards that require a longer waiting period (Bernosky-Smith et al., 2018), another component of impaired control over reward seeking behavior that is common in SUDs (de Wit, 2009; Dalley and Ersche, 2019).
Mesostriatal DA signaling is important for the escalation of drug intake. Repeated drug exposure, via passive administration or self administration, generally increases DA signaling in the NAc and produces exaggerated drug seeking motivation (Robinson and Berridge, 2001). Recent work, however, highlights how the pattern of drug intake can produce starkly different effects on mesostriatal DA circuits (reviewed in Samaha et al., 2021). Extended or long access to drug self administration, which produces escalation of drug intake, craving, and other addiction-like behaviors across a variety of drug types (Ahmed and Koob, 1998; Vanderschuren and Ahmed, 2013; Ahmed, 2018), is also associated with blunted drug-evoked NAc DA signaling, especially in cocaine use models (Mateo et al., 2005; Calipari et al., 2014; Willuhn et al., 2014; Siciliano et al., 2015). Intermittent, binge-like cocaine use, in contrast, sensitizes mesostriatal DA signals, relative to animals with a history of extended or continuous access, despite also producing strong escalation of intake and craving after much less total drug exposure (Kawa et al., 2019a). Intermittent drug exposure also selectively potentiates cocaine’s actions to inhibit DA transporter function, to facilitate elevated NAc DA signaling, relative to continuous or extended access (Calipari et al., 2013).
The distinction between the impact of extended versus intermittent access self administration on NAc DA signaling illustrates how DA circuits are sensitive to a number of features of drug experience, and careful consideration of the details of animal behavior models are critical for interpreting reported brain mechanism outcomes. For example, extended drug access may produce blunted NAc DA responses during well predicted and well learned drug-taking actions, an acute “hypodopaminergic” state that could promote greater drug taking to make up the reward deficit (Blum et al., 2000, 2015; Leyton and Vezina, 2014). Simultaneously, DA responses to drug-paired cues and unpredicted drug exposure can become sensitized, which underlies exaggerated cue-evoked drug seeking motivation, especially after a period of abstinence (Robinson and Berridge, 2001; Bradberry, 2007; Kawa et al., 2019b). Thus, it is possible for DA to be both down and upregulated in the context of SUD models, depending on specific DA circuits, task features, and when the signals are measured. Notably, humans use drugs in a variety of patterns, depending on the reason for use, the drug’s pharmacology and route of administration, and various other social and cultural factors (Gardner, 2011; Allain et al., 2015), so animal models featuring extended, continuous, and intermittent exposure are all likely important for capturing different features of addiction that reflect complex adaptations in the DA system.
Drug seeking responses maintained by the conditioned reinforcing value of cocaine-paired cues rely on VTA activity (McFarland and Kalivas, 2001; Shaham et al., 2003; Ciano and Everitt, 2004; Yun et al., 2004; Kufahl et al., 2009; Lüscher and Malenka, 2011; Mahler and Aston-Jones, 2012). This feature of mesostriatal control of addiction-like behavior is clear in models of relapse, which is often precipitated by exposure to a cue or location that was previously paired with drug delivery. VTA DA neurons mediate drug-cue induced relapse behaviors, and drug cues elicit VTA DA activity and DA release in the NAc with cocaine, alcohol, and other drugs (Ito et al., 2000; Phillips et al., 2003; Aragona et al., 2009; Ostlund et al., 2014; Wolf, 2016; Liu et al., 2020). Under periods of abstinence, VTA activity and NAC DA release facilitates relapse to cocaine, heroin, and alcohol in the presence of these cues (Shaham et al., 2003; Saunders et al., 2013; Corre et al., 2018; Mahler et al., 2019). Conversely, drug seeking is reduced by inactivation of the mesolimbic pathway (McFarland and Kalivas, 2001; Chaudhri et al., 2009; Saunders et al., 2013; Corre et al., 2018; Mahler et al., 2019; Valyear et al., 2020). These data fit within the framework of mesostriatal DA primarily controlling cue-guided or goal-directed drug seeking motivation.
Nigrostriatal
A crucial part of SUDs is that drug taking is no longer recreational, but can become habitual, characterized by inflexible drug-taking actions that are insensitive to feedback and changing contingencies. A central feature of the organization of dopamine-striatum circuitry is the transition from ventromedial signaling early in reward learning, when behaviors are primarily goal directed, to later signaling in dorsolateral striatum that accompanies the development of habit-like behaviors (Haber et al., 2000; Joel et al., 2002; Ikemoto, 2007; Burton et al., 2015). This transition is readily demonstrated across drug classes in animal models (Zapata et al., 2010; Clemens et al., 2014; Hodebourg et al., 2019; Zhou et al., 2019), where habit-like behaviors are associated with nigrostriatal activity. Notably, the nigrostriatal DA pathway is less directly activated by acute drug exposure in animals with limited drug use history, compared to the mesostriatal pathway (Mereu et al., 1987; Ito et al., 2002; Keath et al., 2007; Belin and Everitt, 2008; Murray et al., 2012; Willuhn et al., 2012, 2014). Drug use is thought to accelerate the transition to addiction-like behaviors via progressive engagement of the dorsolateral striatum. Evidence from rodent self-administration models supports this notion. Dopamine signaling in response to cocaine and cocaine-associated cues is initially strongest in the NAc as rats learn to self administer the drug. Over time, the DA response to cocaine delivery in the NAc weakens, and DLS DA signaling emerges (Ito et al., 2002; Willuhn et al., 2012, 2014). Further, the emergence of robust DLS DA signaling predicts the degree of escalation of drug use, and DA signaling and activity in the DLS is necessary for robust cocaine and alcohol self administration only after extended drug use (Belin and Everitt, 2008; Corbit et al., 2012; Murray et al., 2012; Willuhn et al., 2012, 2014). In line with this, DLS D1-MSN activity is associated with escalated methamphetamine self-administration (Oliver et al., 2019). Extended nicotine self administration is associated with exaggerated neural activity in the SNc and DLS (Clemens et al., 2014). DLS DA signaling is necessary for cocaine self administration maintained on second-order reinforcement schedules (Vanderschuren et al., 2005), which is thought to reflect the development of stimulus-response associations that are resistant to extinction. Further, within the DLS, well learned alcohol seeking actions are preferentially encoded over drug receipt (Fanelli et al., 2013), which is consistent with the notion of this system in mediating habitual or ritualistic features of drug taking (Everitt and Robbins, 2005). Together these results suggest that the nigrostriatal DA pathway is recruited to promote the escalation of drug use and rigid drug-intake patterns, which underlies the development of addiction-like states in SUDs.
An inability to change behavior in response to changing outcome value is proposed to be a key reason behind drug craving and the draw of drug-associated cues. Interestingly, SNc DA neurons have been shown to encode reward values over the long term, even when these rewards are no longer expected (Kim et al., 2015). This could suggest that even if tolerance to some of the pharmacological features of a drug is developed, SNc DA retains the drug taking “habit” via an inflexible memory of the reward when first experienced. Disordered memory could also impact relapse susceptibility. For example, inflating rewarding memories of drug use, or decreasing memories of negative experience, could make one more likely to use a drug even after a period of abstinence. Supporting this general notion, in one study where rat SNc DA neurons were chemically lesioned, lesioned rats performed worse on a task that delivered negative feedback for poor performance (Da Cunha et al., 2001). Exaggerated or otherwise altered nigrostriatal activity that accompanies drug exposure may produce a state of feedback insensitivity that promotes exaggerated behaviors in SUDs.
Category II – Impaired Social Behavior
The social and lifestyle consequences of SUDs are perhaps the most difficult to study in non-human animals, as this category includes behavioral features such as “continuing to use, even when it causes problems in relationships” and “giving up important social, occupational, or recreational activities because of substance use”. Common models of social behavior that align with SUD diagnostic criteria are shown in Figure 1. Recent modeling efforts have focused on elements of sociality that are readily measured in species like rodents, including social interaction and affiliative and rearing behaviors, and the effects of social experience on decision making. Importantly, the interaction between social experience and drug-related behaviors is bidirectional. Conspecific-based stressors, including disrupted parental care, social isolation, and social defeat or subordinate status, generally increase future self administration of amphetamine, cocaine, alcohol, and heroin (Schenk et al., 1987; Bardo et al., 2013). In contrast, positively valenced or rewarding social interactions can be protective against cocaine and heroin self administration, craving, and other addiction-like behaviors, even in rats with extensive drug-taking experience (Banks and Negus, 2017; Venniro et al., 2018, 2019). Social play is highly rewarding in most mammals and relies on normal function in striatal DA systems (Vanderschuren et al., 1997; Manduca et al., 2016). As such, social behavior can be disrupted with prenatal or adolescent drug exposure (Trezza et al., 2014; Achterberg et al., 2019). Further, isolation from social play, particularly during adolescence, can promote future drug use and decision-making deficits associated with addiction (McCutcheon and Marinelli, 2009; Lesscher et al., 2015). Like social exposure, other forms of environmental enrichment and access to other non-food rewards, such as an exercise wheel, can have protective effects against escalation of cocaine self administration (Zlebnik and Carroll, 2015). While much remains to identify neural mechanisms of these effects, this work potentially underscores the importance of prosocial, lifestyle, and community-based SUD treatments for humans (Higgins et al., 2003; Meyers et al., 2011; Stitzer et al., 2011).
Mesostriatal
Given its core role in reward processes, the mesostriatal pathway has a central role in social behavior. Social interaction increases mesostriatal DA signaling and NAc neurons are active during approach to both novel conspecifics and pair-bonded partners (Robinson et al., 2002; Gunaydin et al., 2014; Scribner et al., 2020). Mesostriatal DA neurons projecting to the NAc are necessary for normal social interaction behavior (Gunaydin et al., 2014). Inhibition of mesostriatal DA neurons can disrupt exploration of novel conspecifics (Bariselli et al., 2018) and stimulating these neurons can enhance social preference (Bariselli et al., 2016). Drug-evoked changes in VTA DA signaling and physiology can impact these social behaviors. For example, neonatal exposure to amphetamine increases VTA DA activity and decreases social behavior in adulthood (Fukushiro et al., 2015). This may be due to a D1-specific mechanism in the NAc, as blocking D1-like DA receptors in this region rescues impaired social bonding behavior in amphetamine-treated male prairie voles (Liu et al., 2010). Further, following repeated exposure to amphetamine, female prairie voles show decreased social bonding behavior, accompanied by decreased DA D2 receptor immunoreactivity and increased DA levels in the NAc. Notably, administering oxytocin can restore social bonding and NAc DA levels, suggesting an interaction between oxytocin and DA systems in social behavior and drug use (Young et al., 2014).
In addition to drugs affecting mesostriatal DA signaling and social behavior, social interactions can in turn alter drug taking and signaling as well. Social defeat stress has been shown to enhance the long-term potentiation of glutamatergic signaling in the VTA as well as potentiate cocaine conditioned place preference (Stelly et al., 2016). Further, increased cocaine use following social defeat stress can be mimicked by directly infusing corticotropin releasing factor into the VTA, which modulates DA neurons (Leonard et al., 2017). Maternal separation can also disrupt reward seeking and DA signaling in the mesostriatal pathway. For example, female, but not male mice subjected to maternal separation and social isolation show a decreased conditioned place preference for a palatable reward and a decreased level of D1 receptor mRNA in the NAc (Sasagawa et al., 2017). Social isolation can also reduce the total dendritic length of MSNs in the NAc (Wang et al., 2012), while increasing DA signaling (Hall et al., 1999; Yorgason et al., 2016). The ability to produce aberrant or exaggerated mesostriatal DA signaling is thus one mechanism by which social stressors can produce addiction vulnerability.
Nigrostriatal
Given its role in movement control, the function of nigrostriatal DA in the context of Parkinson’s disease (PD) models has driven most research investigations. Notably, while severe PD is primarily characterized by motor impairments, patients also experience cognitive and emotional deficits that affect social behavior. As such, some (Tadaiesky et al., 2008; Matheus et al., 2016) suggest this pathway could be critical for social impairments seen in people with SUD’s. Supporting this, in rats, nigrostriatal damage can increase depression-like symptoms and cognitive impairments in a social recognition test, as well as promote social withdrawal (Tadaiesky et al., 2008; Matheus et al., 2016). Interestingly, these effects were observed after an initial anhedonic response which mapped onto changes in dorsal striatal D1 and D2 receptor activity. Specifically, DA lesions increased the density of D1 and D2 receptors in the DLS after 7 days, which returned to control levels at 21 days when the anhedonic-like effects were no longer present and social withdrawal emerged. Cholinergic interneurons (ChIs) in the dorsal striatum, which can regulate DA release locally via terminal mechanisms (Collins and Saunders, 2020), may also play a role in regulating social behaviors in mild nigrostriatal lesioned mice. For example, inhibiting striatal ChIs reverses social memory impairments caused by DA depletion (Ztaou et al., 2018). While the connection between nigrostriatal DA and socially-based behavioral changes in the context of SUDs has not been characterized in animal models, this system is engaged by social experiences (Robinson et al., 2002). Lesions of the substantia nigra in general seem to reduce some social behaviors, including mate investigation and social grooming (Eison et al., 1977). Other studies have shown that rats with SNc lesions exhibit no difference on a social interaction test (Loiodice et al., 2019), however, so the connection between nigrostriatal DA signaling and normal social behaviors, independent of non-specific motor effects, requires more consideration.
Rearing conditions also affect the nigrostriatal dopaminergic pathway. Social enrichment can reverse the behavioral effects of nigrostriatal lesions in mice. Specifically, it slows the progressive nature of lesioning damage as well as reverses motor impairments (Goldberg et al., 2012). Social isolation increases DA release and uptake in the dorsal striatum in rats, via alterations in DA transporter function, which results in greater psychostimulant potency (Yorgason et al., 2016). Together these studies show that disruptions to the nigrostriatal dopaminergic pathway produce social and cognitive deficits and different social conditions can affect this pathway and in turn, drug reactivity.
Category III – Risky Substance Use
The DSM criteria for risky use of substances includes “using substances again and again, even when it puts you in danger” and “continuing to use, even when you know you have a physical or psychological problem that could have been caused or made worse by the substance”. Common decision-making tasks thought to capture these SUD criteria are shown in Figure 1. Recently, animal models of behaviors related to this SUD criterion have become more common, including “risky” choice assessment, conflict procedures, and punishment-resistant intake models (Venniro et al., 2020).
Risky substance use is associated with compulsivity, which in animal models is typically operationalized as a continuation of behavior despite negative consequences. This is measured in a few ways. For example, rodent tasks that approximate human gambling conditions can assess cost-benefit decision making, sensitivity to loss or punishment, and performance under conditions of uncertainty as metrics of risk taking (Orsini et al., 2015; Winstanley and Clark, 2016; Lüscher et al., 2020). Approach-avoidance paradigms impose a situation of motivational conflict on the research subject, between the urge to seek out a reward and avoid an aversive or costly stimulus (Oleson and Cheer, 2013). In related punishment-based models, reward seeking actions also result in the delivery of noxious or otherwise aversive stimulus, such as footshock, or a bitter taste. A history of escalated use of several drugs, including cocaine, alcohol, and opiates promotes punishment resistance (Vanderschuren and Everitt, 2004; Pelloux et al., 2007; Marchant et al., 2013; Hopf and Lesscher, 2014; Blackwood et al., 2020; Monroe and Radke, 2021; Domi et al., 2021). Notably, these tasks are particularly useful for assessing individual differences in addiction-like behavior, as only a subset of animal subjects will persist in drug seeking in the face of high cost (Shaham et al., 2003; Everitt and Robbins, 2005; Cooper et al., 2007; Vanderschuren et al., 2017; Giuliano et al., 2018).
Mesostriatal
Ventral tegmental area DA neuron stimulation, in the absence of other reward-related stimuli, can lead to compulsive-like behavior. When given the option to self stimulate VTA DA neurons in the face of a punishing footshock, a subset of mice will perseverate, enduring high shock levels (Pascoli et al., 2015). Mesostriatal DA signaling is also important for conflict-based behaviors. In rats, phasic DA signaling in the NAc encodes motivational conflict: cues signaling threats evoke greater DA release compared to neutral cues, and this signal correlates with successful behavioral avoidance (Oleson et al., 2012; Gentry et al., 2016). Further, blocking NAc DA abolishes, and increasing NAc DA potentiates, drug-seeking behavior in a task where rats were required to cross an electrified barrier to receive infusions of cocaine (Saunders et al., 2013). Notably, this effect was strongest in the subset of rats who were willing to experience the highest shock levels, suggesting a link between mesostriatal DA and individual differences in motivation in the face of adverse consequences.
Disruptions to the mesostriatal pathway modulate so-called risk-based decisions that incorporate cost-benefit probabilities (Orsini et al., 2015). For example, DA signaling within the NAc encodes risky decisions in gambling-inspired tasks (Sugam et al., 2012). Notably, subsets of VTA DA neurons have different roles in risky decision making (Verharen et al., 2018). Activation of the mesostriatal pathway reduces sensitivity to loss and punishment, while activating the VTA-PFC pathway promotes risky decisions when there is no loss present. Further, stimulating the VTA after a non-rewarded risky choice, which overrides the phasic dip in DA release that would normally occur, biases rats to choose a risky reward in the future and reduces sensitivity to reward omissions (Stopper et al., 2014).
Hyperdopaminergic states, such as those evoked by drugs, can lead to disordered decision making that favors risk taking, feedback insensitivity, and behavioral inflexibility (Stalnaker et al., 2009; Izquierdo et al., 2010; Groman et al., 2018). Drug exposure can affect future risk-based decisions, via alterations in mesostriatal DA. For example, adolescent alcohol exposure in rats reduces overall mesostriatal DA tone, but potentiates phasic DA release, an effect that positively correlates with risk preference, and is reversed when the DA signal is normalized (Schindler et al., 2016). Adolescent drug exposure selectively disrupts NAc DA encoding of costs (Nasrallah et al., 2011), suggesting that feedback insensitivity associated with addiction-like behavior relies on specific drug-induced mesostriatal adaptations. Interestingly, the role of mesostriatal DA in risky decision making may be somewhat sex dependent, as males exhibit decreased cue-induced risky choice behavior following VTA inhibition while females exhibit increased risky choice (Hynes et al., 2021). These results suggest that altering VTA DA activity leads to an impairment of decision making that is facilitated by, and could contribute to, risky drug use.
Outside of the striatum, VTA projections to the prefrontal cortex also play a major role in reward seeking in risky situations. During reward-seeking actions, risk of punishment diminishes synchrony between the VTA and PFC (Park and Moghaddam, 2017). Further, during learning, phasic activity in the PFC of rats encodes risky seeking actions but not safe taking actions or reward delivery, suggesting that the PFC is preferentially involved in the learning of punishment probability. This effect was also sex specific, with females exhibiting greater sensitivity to probabilistic punishment than males (Jacobs and Moghaddam, 2020). However, this effect may not be mediated by VTA-PFC DA projections per se, given some studies showing that these neurons do not exhibit differential activity under threat of punishment (Verharen et al., 2020).
Nigrostriatal
The role of nigrostriatal DA in habit-like actions underlies its connection to compulsive behaviors that contribute to risky drug use (Everitt and Robbins, 2005). One characteristic of habit-like behavior is an encoding of a stable reward value despite changes to the reward itself. Notably, a subset of DA neurons in the lateral SNC demonstrate a “sustain-type” firing pattern that is insensitive to changes in expected reward after extended learning (Kim et al., 2015). Further, DLS DA axon terminals don’t exhibit a clear decrease in activity when the actual reward is smaller than predicted, unlike terminals in dorsomedial and ventral striatum (Tsutsui-Kimura et al., 2020). This lack of feedback within the nigrostriatal pathway in learning could result in drug-taking behavior despite a negative consequence or in risky situations.
In an approach-avoidance decision making task, dopamine’s actions within the DMS have opposing effects on behavior, with D1-MSN activation facilitating approach and D2-MSN receptors suppress approach. In contrast, DLS DA manipulations don’t as clearly affect approach-avoidance behavior (Nguyen et al., 2019). However, after extended access to cocaine self administration, DLS inactivation selectively reduces self administration in the face of punishment, compared to unpunished use (Jonkman et al., 2012). Further, individual differences in the extent to which alcohol seeking engages activity in the DLS predicts susceptibility to punishment resistance (Giuliano et al., 2019), suggesting a specific role in compulsivity and threat-based feedback. DMS inactivation increases risky choice on a probabilistic discounting task in rats, suggesting that it in contrast can facilitate flexibility in reward prediction (Schumacher et al., 2021). Taken together, these results are consistent with the notion that SNc-DLS DA projections contribute to inflexible behavior, the SNc-DMS projections promote flexibility in goal-directed behavior, both of which are engaged during risk-reward decisions (Lerner et al., 2015; Vandaele and Ahmed, 2021). Recent evidence suggests that unlike other DA neurons, projections to the caudal “tail” portion of the dorsal striatum preferentially encode threatening stimuli and threat avoidance, relative to positively valenced stimuli (Menegas et al., 2018). This suggests they could have a critical role in risk-based decisions and compulsivity. Notably, the effect of a history of drug exposure on nigrostriatal and striatal tail function in conflict or avoidance tasks remains relatively unexplored. Nevertheless, the above data suggest that in SUD patients, dysregulation or imbalance of DA signaling across SNC output targets could promote risk insensitivity to underlie dangerous substance use.
Further insight into the connection between altered nigrostriatal DA signaling and compulsive behavior comes from PD patients receiving DA replacement therapy, which can result in impulse control disorders that lead to risky decision making. Levodopa treatment, for example, can be effective at restoring motor function associated with nigrostriatal DA degeneration in Parkinson’s, but a subset of patients experience an increase in addiction-like behaviors, including compulsive use of levodopa itself (Lawrence et al., 2003; Evans et al., 2006). These effects are partially mediated by the emergence of D1-receptor supersensitivity that results from nigrostriatal DA neuron degeneration (Gerfen, 2003). Notably, and consistent with the human PD phenomenon, a subset of parkinsonian rats display high sensitivity to DA replacement drugs, after a history of drug self administration (Engeln et al., 2013). More work on the link between Parkinson’s states and the behavioral effects of DA replacement in animal models will be useful in understanding nigrostriatal DA’s role in risky and disordered drug use (Cenci et al., 2015; Napier et al., 2020).
Other Considerations for Animal Models of Substance Use Disorder
Drug Type and Route of Administration
Given historical patterns in addiction research, much of the current conceptualization of SUD is based on behavioral modeling in a relatively narrow range of compounds, compared to the broad scope of drug types and routes of administration used by humans. Much of the work discussed here, for example, made use of stimulant drugs (primarily cocaine), although application of SUD animal models to non-stimulant drugs is becoming more common. This is an important consideration for future research, given that the majority of drug use in contemporary humans is of non-stimulant drugs, including opioids, cannabis, and alcohol (Substance Abuse and Mental Health Services Administration, 2019). More SUD-model research on a broader set of drugs is critical because not all drugs engage the same learning mechanisms to produce patterns of addiction-like behaviors. Nicotine, for example, is relatively weak as a primary reinforcer of drug self administration, compared to other drugs (Pontieri et al., 1996). Instead, nicotine may augment reward seeking to promote addiction-like behavior by potentiating the motivational value of other stimuli and actions via non-associative mechanisms (Donny et al., 2003; Chaudhri et al., 2006). This heterogeneity is underscored by the fact that while most self administered drugs increase DA release and act on striatal circuits, they do so to different degrees, and through different mechanisms that may produce unique signaling patterns with specific behavioral relevance (Wise and Bozarth, 1987; Johnson and North, 1992; Nutt et al., 2015; Volkow et al., 2017; Nestler and Lüscher, 2019; Solinas et al., 2019; Wise and Robble, 2020). Further complications come from the fact that some drugs used by humans, such as some hallucinogens, do not as reliably increase DA signaling, and are not readily self administered by rodents, making the application of SUD behavioral models described here difficult (Griffiths et al., 1979; Fantegrossi et al., 2008; Serra et al., 2015).
Drugs are taken by humans through different routes of administration, including most commonly via oral consumption, intravenous or subcutaneous injection, or inhalation. The route of delivery affects the pharmacological impact that drugs have on the brain and peripheral physiology, producing unique neurobiological changes and vulnerabilities to addiction-like behaviors (Jones, 1990; Gossop et al., 1992; Cone, 1998; Allain et al., 2015). Intravenous injection and smoking produce the fastest rise and highest drug concentration in the blood, which is associated with greater DA signaling and neural activity in reward circuits (Samaha et al., 2021). Notably, the large majority of drug self administration animal models have relied on intravenous or oral consumption drug delivery, which may obscure unique neurobiological and behavioral adaptations produced by other delivery routes. Smoked cannabis and nicotine are among the most consumed drugs by humans, for example, underscoring the need for SUD models that are amenable to inhalation exposure. Recent work has progressed on this front, with technology for vapor-based delivery of cannabis, cannabinoids, nicotine, and other drugs in rodents (Nguyen et al., 2016; Marusich et al., 2019; Freels et al., 2020).
Co-substance/Poly-Drug Use Models
Simultaneous or serial use of multiple drug types is a common feature of human behavior and is reflected in many SUD patients. Alcohol, nicotine, and cannabis are commonly used alongside drugs like cocaine and heroin, for example, and poly-use SUD patients experience worse treatment outcomes, compared to patients who primarily use a single drug (Leri et al., 2003; Mccabe et al., 2006; Substance Abuse and Mental Health Services Administration, 2019; Crummy et al., 2020; Compton et al., 2021). Despite this, animal models of SUDs have nearly exclusively made use of single-drug procedures, studying drug effects in isolation. Given the unique adaptations in the DA system produced by different drug types, this single-drug focus likely prevents understanding of unique brain changes associated with poly-drug use. From a treatment perspective, a given individual’s specific drug combination history could produce individualized SUD vulnerabilities that are not captured in classic models. Recently, more emphasis has gone to modeling poly-drug use in rodents (reviewed in Crummy et al., 2020). For example, rats will readily self administer some drug cocktails, including cocaine and heroin (Crombag and Shaham, 2002). This produces DA responses in the NAc that are greater than those evoked by either drug alone (Hemby et al., 1999). In line with this, exposure to both methamphetamine and morphine results in greater locomotor activity than either drug in isolation (Trujillo et al., 2011), and sequential self administration of alcohol and cocaine produces unique neuroadaptations in the NAc compared to cocaine alone (Stennett et al., 2020). Thus, some single-drug studies may actually produce below threshold neurobiological changes, resulting in failure to detect SUD-like features that are more common in poly-use humans. Other work has been done on drug combinations that are popular among humans, such as alcohol and nicotine. Access to both of these can have synergistic effects on reward-related behaviors in rodents, although individual preferences for one drug or the other may drive co-self administration competition (DeBaker et al., 2020; Angelyn et al., 2021). Poly-drug studies can also offer insight into unique, drug-specific pathways to addiction-like behavior and treatment. For example, social defeat stress more reliably produces escalation of speedball self administration, compared to heroin self administration (Cruz et al., 2011). Further, methadone treatment, commonly used in human opioid use patients, is effective at reducing both cocaine and heroin relapse in rat models (Leri et al., 2003). Notably, little is known about unique adaptations in nigrostriatal DA circuits associated with poly-drug use. If some drug combinations evoke greater DA release in the NAc compared to others, they may also produce exaggerated SNc DA activity, facilitating a more rapid transition to habit-like drug related behaviors.
Individual Differences in Substance Use Disorder Vulnerability
Variability in drug use profiles is highlighted by the fact that among all recreational drug users, only ∼20% progress to meet some DSM diagnostic criteria of SUD (Substance Abuse and Mental Health Services Administration, 2019). Furthermore, in the current framework, a SUD diagnosis requires the presence of only two or more criteria (Figure 1). While more severe cases of SUD typically involve several common criteria (American Psychiatric Association, 2013), mild to moderate cases can present with relatively divergent behavioral features. This creates challenges for treatment, as there is no singular “addiction phenotype”: the SUD of one person can look quite different from another person. Animal studies have underscored this by demonstrating that only a small fraction of rodents, when given access to drugs, will progress to develop multiple addiction-like behavioral criteria (Deroche-Gamonet et al., 2004; Robinson, 2004; Vanderschuren and Everitt, 2004; O’Neal et al., 2020). Further, and perhaps more striking, when given the choice between a drug reward and a non-drug reward, such as food or a mate, only a small fraction of rats choose the drug option (Lenoir et al., 2007; Cantin et al., 2010). This non-drug preference persists even in rats with a history of extended drug self administration (Caprioli et al., 2015), but can be overcome with high drug doses and in tasks that equate the rate of reinforcement for drug and non-drug rewards, at least for cocaine (Thomsen et al., 2013; Beckmann et al., 2019). This suggests that individual differences in drug metabolism may intersect with and impact decision making in the context of drug use, contributing to individual vulnerabilities to SUD. This represents a challenge for animal models, where the comparatively limited genetic diversity of research subjects may elide some variability factors. Recent work has made use of “heterogeneous stock” rodents – the product of multiple strain crossings - to increase genetic diversity and investigate individual differences in addiction-like behavior and reward learning in the context of drug use (Hughson et al., 2019; de Guglielmo et al., 2021; King et al., 2021; Sedighim et al., 2021). One such study identified a relationship between gut bacterial content and behavioral features of impulsivity and attention (Peterson et al., 2020). Thus, individualized peripheral systems such as the microbiome can impinge on central brain systems, including DA circuits, in ways that could produce unique SUD vulnerabilities (Lucerne et al., 2021).
Humans exhibit considerable individual differences in SUD characteristics as a function of sex, gender, age, and social and environmental demographics (Brady and Randall, 1999; Degenhardt et al., 2017; Substance Abuse and Mental Health Services Administration, 2019). The factors that promote exaggerated drug use in only a fraction of people are likely myriad, but individual differences in DA system activity and function play a key role (Piray et al., 2010; Saunders et al., 2013). For example, humans with genetic polymorphisms that result in elevated DA system activity show greater reward cue-evoked striatal activity and craving that could denote a predisposition toward exaggerated drug use (Wittmann et al., 2013; Ray et al., 2014). Consistent with this, in animal models, variability in DA signaling and expression of striatal DA receptors is associated with higher drug cue responsivity and relapse (Flagel et al., 2007; Verheij and Cools, 2008; Piray et al., 2010; Saunders et al., 2013; Klanker et al., 2015; Ferguson et al., 2020). Furthermore, at certain stages of the estrus cycle, female rodents have larger NAc DA signals in response to cocaine and cocaine-associated cues, which is thought to underlie their generally higher propensity for addiction-like behaviors (Becker and Cha, 1989; Becker, 1999; Calipari et al., 2017; Johnson et al., 2019).
Given the circuit-specific DA functions in reward learning and addiction-like behavior, some of which we have outlined here, a detailed appreciation of the anatomical locus of variability within DA systems in humans will be essential to forming a link to unique SUD-related vulnerabilities. This will require a better understanding of the DA system across multiple levels of analysis, from genetic and developmental trajectories to in vivo circuit connectivity and activity patterns. Critically, much work remains to better understand how the larger mesocorticostriatal network changes over time as both an antecedent and consequence of drug taking. A functional circuit diagram, coupled with computational approaches for modeling preclinical individual and sex-based differences in decision making strategies (Groman et al., 2019; Chen et al., 2021), will be important for determining the neurobehavioral mechanisms underlying unique vulnerabilities for different types of SUD.
Conclusion
Here we have reviewed evidence for overlapping, but distinct mesostriatal and nigrostriatal DA circuit functions in behavioral outcomes that are relevant to addictions and SUDs (summarized in Figure 1). Dopamine innervation to the striatum contributes to multiple, parallel functions in the context of addiction-like behavior, with the mesostriatal pathway providing a “pull” toward drug seeking by signaling drug and drug-associated stimulus value, especially early in the use cycle. The nigrostriatal pathway, and particularly DLS projecting DA neurons, in contrast are more important for generating the “push” toward exaggerated drug use by controlling rigid, feedback insensitive drug-seeking actions. Notably, as highlighted above, striatal DA is important not only for these positive symptom features of SUDs, including exaggerated seeking and craving, but also for impairments in decision making that underlie compulsive behavior, reduced sociality, and risk taking. Through the use of animal models, greater understanding of the functional heterogeneity of the DA system and related networks can offer insight into this complex symptomatology and may lead to more targeted treatments.
Author Contributions
CP and LE conceptualized and wrote the original manuscript draft, in consultation with BS. CP and BS generated Figure 1. All authors contributed to writing and editing the final manuscript and approved its submission.
Funding
This work was supported by National Institute on Drug Abuse grants T32 DA007234 (CP) and R00 DA042895 (BS).
Conflict of Interest
The authors declare that the research was conducted in the absence of any commercial or financial relationships that could be construed as a potential conflict of interest.
Publisher’s Note
All claims expressed in this article are solely those of the authors and do not necessarily represent those of their affiliated organizations, or those of the publisher, the editors and the reviewers. Any product that may be evaluated in this article, or claim that may be made by its manufacturer, is not guaranteed or endorsed by the publisher.
References
Aarts, E., van Holstein, M., and Cools, R. (2011). Striatal dopamine and the interface between motivation and cognition. Front. Psychol. 2:163. doi: 10.3389/fpsyg.2011.00163
Achterberg, E. J. M., van Swieten, M. M. H., Houwing, D. J., Trezza, V., and Vanderschuren, L. J. M. J. (2019). Opioid modulation of social play reward in juvenile rats. Neuropharmacology 159:107332. doi: 10.1016/j.neuropharm.2018.09.007
Ahmed, S. H. (2018). Trying to make sense of rodents’ drug choice behavior. Prog. Neuropsychopharmacol. Biol. Psychiatry 87, 3–10.
Ahmed, S. H., and Koob, G. F. (1998). Transition from moderate to excessive drug intake: change in hedonic set point. Science 282, 298–300. doi: 10.1126/science.282.5387.298
Ahmed, S. H., Walker, J. R., and Koob, G. F. (2000). Persistent increase in the motivation to take heroin in rats with a history of drug escalation. Neuropsychopharmacology 22, 413–421. doi: 10.1016/S0893-133X(99)00133-5
Allain, F., Minogianis, E.-A., Roberts, D. C. S., and Samaha, A.-N. (2015). How fast and how often: the pharmacokinetics of drug use are decisive in addiction. Neurosci. Biobehav. Rev. 56, 166–179. doi: 10.1016/j.neubiorev.2015.06.012
American Psychiatric Association (2013). Diagnostic and Statistical Manual of Mental Disorders, 5th Edn. American Psychiatric Association. doi: 10.1176/appi.books.9780890425596
Angelyn, H., Loney, G. C., and Meyer, P. J. (2021). Nicotine enhances goal-tracking in ethanol and food Pavlovian conditioned approach paradigms. Front. Neurosci. 15:561766. doi: 10.3389/fnins.2021.561766
Aragona, B. J., Day, J. J., Roitman, M. F., Cleaveland, N. A., Wightman, R. M., and Carelli, R. M. (2009). Regional specificity in the real-time development of phasic dopamine transmission patterns during acquisition of a cue–cocaine association in rats. Eur. J. Neurosci. 30, 1889–1899. doi: 10.1111/j.1460-9568.2009.07027.x
Arias-Carrión, O., and Pǒppel, E. (2007). Dopamine, learning, and reward-seeking behavior. Acta Neurobiol. Exp. 67, 481–488.
Balster, R. L., and Lukas, S. E. (1985). Review of self-administration. Drug Alcohol Depend. 14, 249–261. doi: 10.1016/0376-8716(85)90060-2
Banks, M. L., and Negus, S. S. (2017). Insights from preclinical choice models on treating drug addiction. Trends Pharmacol. Sci. 38, 181–194. doi: 10.1016/j.tips.2016.11.002
Bardo, M. T., Neisewander, J. L., and Kelly, T. H. (2013). Individual differences and social influences on the neurobehavioral pharmacology of abused drugs. Pharmacol. Rev. 65, 255–290. doi: 10.1124/pr.111.005124
Bariselli, S., Hörnberg, H., Prévost-Solié, C., Musardo, S., Hatstatt-Burklé, L., Scheiffele, P., et al. (2018). Role of VTA dopamine neurons and neuroligin 3 in sociability traits related to nonfamiliar conspecific interaction. Nat. Commun. 9:3173. doi: 10.1038/s41467-018-05382-3
Bariselli, S., Tzanoulinou, S., Glangetas, C., Prévost-Solié, C., Pucci, L., Viguié, J., et al. (2016). SHANK3 controls maturation of social reward circuits in the VTA. Nat. Neurosci. 19, 926–934. doi: 10.1038/nn.4319
Becker, J. B. (1999). Gender differences in dopaminergic function in striatum and nucleus accumbens. Pharmacol. Biochem. Behav. 64, 803–812. doi: 10.1016/s0091-3057(99)00168-9
Becker, J. B., and Cha, J.-H. (1989). Estrous cycle-dependent variation in amphetamine-induced behaviors and striatal dopamine release assessed with microdialysis. Behav. Brain Res. 35, 117–125. doi: 10.1016/S0166-4328(89)80112-3
Beckmann, J. S., Chow, J. J., and Hutsell, B. A. (2019). Cocaine-associated decision-making: toward isolating preference. Neuropharmacology 153, 142–152. doi: 10.1016/j.neuropharm.2019.03.025
Beckstead, R. M., Domesick, V. B., and Nauta, W. J. H. (1979). Efferent connections of the substantia nigra and ventral tegmental area in the rat. Brain Res. 175, 191–217. doi: 10.1016/0006-8993(79)91001-1
Belin, D., Balado, E., Piazza, P. V., and Deroche-Gamonet, V. (2009). Pattern of intake and drug craving predict the development of cocaine addiction-like behavior in rats. Biol. Psychiatry 65, 863–868. doi: 10.1016/j.biopsych.2008.05.031
Belin, D., and Everitt, B. J. (2008). Cocaine seeking habits depend upon dopamine-dependent serial connectivity linking the ventral with the dorsal striatum. Neuron 57, 432–441. doi: 10.1016/j.neuron.2007.12.019
Bello, E. P., Mateo, Y., Gelman, D. M., Noaín, D., Shin, J. H., Low, M. J., et al. (2011). Cocaine supersensitivity and enhanced motivation for reward in mice lacking dopamine D2 autoreceptors. Nat. Neurosci. 14, 1033–1038. doi: 10.1038/nn.2862
Bentzley, B. S., Fender, K. M., and Aston-Jones, G. (2013). The behavioral economics of drug self-administration: a review and new analytical approach for within-session procedures. Psychopharmacology 226, 113–125. doi: 10.1007/s00213-012-2899-2
Bernosky-Smith, K. A., Qiu, Y.-Y., Feja, M., Lee, Y. B., Loughlin, B., Li, J.-X., et al. (2018). Ventral tegmental area D2 receptor knockdown enhances choice impulsivity in a delay-discounting task in rats. Behav. Brain Res. 341, 129–134. doi: 10.1016/j.bbr.2017.12.029
Berridge, K. C. (2007). The debate over dopamine’s role in reward: the case for incentive salience. Psychopharmacology 191, 391–431. doi: 10.1007/s00213-006-0578-x
Björklund, A., and Dunnett, S. B. (2007). Dopamine neuron systems in the brain: an update. Trends Neurosci. 30, 194–202. doi: 10.1016/j.tins.2007.03.006
Blackwood, C. A., McCoy, M. T., Ladenheim, B., and Cadet, J. L. (2020). Escalated oxycodone self-administration and punishment: differential expression of opioid receptors and immediate early genes in the rat dorsal striatum and prefrontal cortex. Front. Neurosci. 13:1392. doi: 10.3389/fnins.2019.01392
Blum, K., Braverman, E. R., Holder, J. M., Lubar, J. F., Monastra, V. J., Miller, D., et al. (2000). Reward deficiency syndrome: a biogenetic model for the diagnosis and treatment of impulsive, addictive, and compulsive behaviors. J. Psychoactive Drugs 32(Suppl., i–iv), 1–112. doi: 10.1080/02791072.2000.10736099
Blum, K., Thanos, P. K., Oscar-Berman, M., Febo, M., Baron, D., Badgaiyan, R. D., et al. (2015). Dopamine in the brain: hypothesizing surfeit or deficit links to reward and addiction. J. Reward Defic. Syndr. 1, 95–104. doi: 10.17756/jrds.2015-016
Bouarab, C., Thompson, B., and Polter, A. M. (2019). VTA GABA neurons at the interface of stress and reward. Front. Neural Circuits 13:78. doi: 10.3389/fncir.2019.00078
Bradberry, C. W. (2007). Cocaine sensitization and dopamine mediation of cue effects in rodents, monkeys, and humans: areas of agreement, disagreement, and implications for addiction. Psychopharmacology 191, 705–717. doi: 10.1007/s00213-006-0561-6
Brady, K. T., and Randall, C. L. (1999). Gender differences in substance use disorders. Psychiatr. Clin. North Am. 22, 241–252. doi: 10.1016/S0193-953X(05)70074-5
Britt, J. P., Benaliouad, F., McDevitt, R. A., Stuber, G. D., Wise, R. A., and Bonci, A. (2012). Synaptic and behavioral profile of multiple glutamatergic inputs to the nucleus accumbens. Neuron 76, 790–803. doi: 10.1016/j.neuron.2012.09.040
Buckholtz, J. W., Treadway, M. T., Cowan, R. L., Woodward, N. D., Li, R., Ansari, M. S., et al. (2010). Dopaminergic network differences in human impulsivity. Science 329:532. doi: 10.1126/science.1185778
Burton, A. C., Nakamura, K., and Roesch, M. R. (2015). From ventral-medial to dorsal-lateral striatum: neural correlates of reward-guided decision-making. Neurobiol. Learn. Mem. 117, 51–59. doi: 10.1016/j.nlm.2014.05.003
Calipari, E. S., Ferris, M. J., and Jones, S. R. (2014). Extended access of cocaine self-administration results in tolerance to the dopamine-elevating and locomotor-stimulating effects of cocaine. J. Neurochem. 128, 224–232. doi: 10.1111/jnc.12452
Calipari, E. S., Ferris, M. J., Zimmer, B. A., Roberts, D. C., and Jones, S. R. (2013). Temporal pattern of cocaine intake determines tolerance vs sensitization of cocaine effects at the dopamine transporter. Neuropsychopharmacology 38, 2385–2392. doi: 10.1038/npp.2013.136
Calipari, E. S., Juarez, B., Morel, C., Walker, D. M., Cahill, M. E., Ribeiro, E., et al. (2017). Dopaminergic dynamics underlying sex-specific cocaine reward. Nat. Commun. 8:13877. doi: 10.1038/ncomms13877
Cantin, L., Lenoir, M., Augier, E., Vanhille, N., Dubreucq, S., Serre, F., et al. (2010). Cocaine is low on the value ladder of rats: possible evidence for resilience to addiction. PLoS One 5:e11592. doi: 10.1371/journal.pone.0011592
Caprioli, D., Zeric, T., Thorndike, E. B., and Venniro, M. (2015). Persistent palatable food preference in rats with a history of limited and extended access to methamphetamine self-administration. Addict. Biol. 20, 913–926. doi: 10.1111/adb.12220
Cardinal, R. N., Parkinson, J. A., Hall, J., and Everitt, B. J. (2002). Emotion and motivation: the role of the amygdala, ventral striatum, and prefrontal cortex. Neurosci. Biobehav. Rev. 26, 321–352. doi: 10.1016/S0149-7634(02)00007-6
Carelli, R. M. (2004). Nucleus accumbens cell firing and rapid dopamine signaling during goal-directed behaviors in rats. Neuropharmacology 47(Suppl. 1), 180–189. doi: 10.1016/j.neuropharm.2004.07.017
Cenci, M. A., Francardo, V., O’Sullivan, S. S., and Lindgren, H. S. (2015). Rodent models of impulsive–compulsive behaviors in Parkinson’s disease: How far have we reached? Neurobiol. Dis. 82, 561–573. doi: 10.1016/j.nbd.2015.08.026
Chaudhri, N., Caggiula, A. R., Donny, E. C., Palmatier, M. I., Liu, X., and Sved, A. F. (2006). Complex interactions between nicotine and nonpharmacological stimuli reveal multiple roles for nicotine in reinforcement. Psychopharmacology 184, 353–366. doi: 10.1007/s00213-005-0178-1
Chaudhri, N., Sahuque, L. L., and Janak, P. H. (2008). Context-induced relapse of conditioned behavioral responding to ethanol cues in rats. Biol. Psychiatry 64, 203–210. doi: 10.1016/j.biopsych.2008.03.007
Chaudhri, N., Sahuque, L. L., and Janak, P. H. (2009). Ethanol seeking triggered by environmental context is attenuated by blocking dopamine D1 receptors in the nucleus accumbens core and shell in rats. Psychopharmacology 207, 303–314. doi: 10.1007/s00213-009-1657-6
Chen, C. S., Ebitz, R. B., Bindas, S. R., Redish, A. D., Hayden, B. Y., and Grissom, N. M. (2021). Divergent strategies for learning in males and females. Curr. Biol. 31, 39–50.e4. doi: 10.1016/j.cub.2020.09.075
Chen, R., McIntosh, S., Hemby, S., Sun, H., Sexton, T., Thomas, M., et al. (2018). High and low doses of cocaine intake are differentially regulated by dopamine D2 receptors in the ventral tegmental area and the nucleus accumbens. Neurosci. Lett. 671, 133–139. doi: 10.1016/j.neulet.2018.02.026
Chiara, G. D., and Imperato, A. (1988). Drugs abused by humans preferentially increase synaptic dopamine concentrations in the mesolimbic system of freely moving rats. Proc. Natl. Acad. Sci. U.S.A. 85, 5274–5278. doi: 10.1073/pnas.85.14.5274
Ciano, P. D., and Everitt, B. J. (2004). Contribution of the ventral tegmental area to cocaine-seeking maintained by a drug-paired conditioned stimulus in rats. Eur. J. Neurosci. 19, 1661–1667. doi: 10.1111/j.1460-9568.2004.03232.x
Clemens, K. J., Castino, M. R., Cornish, J. L., Goodchild, A. K., and Holmes, N. M. (2014). Behavioral and neural substrates of habit formation in rats intravenously self-administering nicotine. Neuropsychopharmacology 39, 2584–2593. doi: 10.1038/npp.2014.111
Coddington, L. T., and Dudman, J. T. (2018). The timing of action determines reward prediction signals in identified midbrain dopamine neurons. Nat. Neurosci. 21, 1563–1573. doi: 10.1038/s41593-018-0245-7
Collins, A. L., and Saunders, B. T. (2020). Heterogeneity in striatal dopamine circuits: form and function in dynamic reward seeking. J. Neurosci. Res. 98, 1046–1069. doi: 10.1002/jnr.24587
Compton, W. M., Valentino, R. J., and DuPont, R. L. (2021). Polysubstance use in the U.S. opioid crisis. Mol. Psychiatry 26, 41–50. doi: 10.1038/s41380-020-00949-3
Cone, E. J. (1998). Recent discoveries in pharmacokinetics of drugs of abuse. Toxicol. Lett. 10, 97–101. doi: 10.1016/S0378-4274(98)00292-6
Cooper, A., Barnea-Ygael, N., Levy, D., Shaham, Y., and Zangen, A. (2007). A conflict rat model of cue-induced relapse to cocaine seeking. Psychopharmacology 194, 117–125. doi: 10.1007/s00213-007-0827-7
Corbett, D., and Wise, R. A. (1980). Intracranial self-stimulation in relation to the ascending dopaminergic systems of the midbrain: a moveable electrode mapping study. Brain Res. 185, 1–15. doi: 10.1016/0006-8993(80)90666-6
Corbit, L. H., Nie, H., and Janak, P. H. (2012). Habitual alcohol seeking: time course and the contribution of subregions of the dorsal striatum. Biol. Psychiatry 72, 389–395. doi: 10.1016/j.biopsych.2012.02.024
Corre, J., van Zessen, R., Loureiro, M., Patriarchi, T., Tian, L., Pascoli, V., et al. (2018). Dopamine neurons projecting to medial shell of the nucleus accumbens drive heroin reinforcement. eLife 7:e39945. doi: 10.7554/eLife.39945
Cox, J., and Witten, I. B. (2019). Striatal circuits for reward learning and decision-making. Nat. Rev. Neurosci. 20, 482–494. doi: 10.1038/s41583-019-0189-2
Crombag, H. S., and Shaham, Y. (2002). Renewal of drug seeking by contextual cues after prolonged extinction in rats. Behav. Neurosci. 116, 169–173. doi: 10.1037//0735-7044.116.1.169
Crummy, E. A., O’Neal, T. J., Baskin, B. M., and Ferguson, S. M. (2020). One is not enough: understanding and modeling polysubstance use. Front. Neurosci. 14:569. doi: 10.3389/fnins.2020.00569
Cruz, F. C., Quadros, I. M., Hogenelst, K., Planeta, C. S., and Miczek, K. A. (2011). Social defeat stress in rats: escalation of cocaine and “speedball” binge self-administration, but not heroin. Psychopharmacology 215, 165–175. doi: 10.1007/s00213-010-2139-6
Da Cunha, C., Gevaerd, M. S., Vital, M. A., Miyoshi, E., Andreatini, R., Silveira, R., et al. (2001). Memory disruption in rats with nigral lesions induced by MPTP: a model for early Parkinson’s disease amnesia. Behav. Brain Res. 124, 9–18. doi: 10.1016/s0166-4328(01)00211-x
da Silva, J. A., Tecuapetla, F., Paixão, V., and Costa, R. M. (2018). Dopamine neuron activity before action initiation gates and invigorates future movements. Nature 554, 244–248. doi: 10.1038/nature25457
Dalley, J. W., and Ersche, K. D. (2019). Neural circuitry and mechanisms of waiting impulsivity: relevance to addiction. Philos. Trans. R. Soc. Lond. B Biol. Sci. 374:20180145 doi: 10.1098/rstb.2018.0145
de Guglielmo, G., Carrette, L. L., Kallupi, M., Brennan, M., Boomhower, B., Maturin, L., et al. (2021). Characterization of cocaine addiction-like behavior in heterogeneous stock rats. bioRxiv [Preprint]. doi: 10.1101/2021.07.22.453410
de Jong, J. W., Roelofs, T. J. M., Mol, F. M. U., Hillen, A. E. J., Meijboom, K. E., Luijendijk, M. C. M., et al. (2015). Reducing ventral tegmental dopamine D2 receptor expression selectively boosts incentive motivation. Neuropsychopharmacology 40, 2085–2095. doi: 10.1038/npp.2015.60
de Wit, H. (2009). Impulsivity as a determinant and consequence of drug use: a review of underlying processes. Addict. Biol. 14, 22–31. doi: 10.1111/j.1369-1600.2008.00129.x
DeBaker, M. C., Robinson, J. M., Moen, J. K., Wickman, K., and Lee, A. M. (2020). Differential patterns of alcohol and nicotine intake: combined alcohol and nicotine binge consumption behaviors in mice. Alcohol 85, 57–64. doi: 10.1016/j.alcohol.2019.09.006
Degenhardt, L., Peacock, A., Colledge, S., Leung, J., Grebely, J., Vickerman, P., et al. (2017). Global prevalence of injecting drug use and sociodemographic characteristics and prevalence of HIV, HBV, and HCV in people who inject drugs: a multistage systematic review. Lancet Glob. Health 5, e1192–e1207. doi: 10.1016/S2214-109X(17)30375-3
Deroche-Gamonet, V., Belin, D., and Piazza, P. V. (2004). Evidence for addiction-like behavior in the rat. Science 305, 1014–1017. doi: 10.1126/science.1099020
Diana, M. (2011). The dopamine hypothesis of drug addiction and its potential therapeutic value. Front. Psychiatry 2:64. doi: 10.3389/fpsyt.2011.00064
Dodson, P. D., Dreyer, J. K., Jennings, K. A., Syed, E. C. J., Wade-Martins, R., Cragg, S. J., et al. (2016). Representation of spontaneous movement by dopaminergic neurons is cell-type selective and disrupted in parkinsonism. Proc. Natl. Acad. Sci. U.S.A. 113, E2180–E2188. doi: 10.1073/pnas.1515941113
Domi, E., Xu, L., Toivainen, S., Nordeman, A., Gobbo, F., Venniro, M. E., et al. (2021). A neural substrate of compulsive alcohol use. Sci. Adv. 7:eabg9045. doi: 10.1126/sciadv.abg9045
Donny, E. C., Chaudhri, N., Caggiula, A. R., Evans-Martin, F. F., Booth, S., Gharib, M. A., et al. (2003). Operant responding for a visual reinforcer in rats is enhanced by noncontingent nicotine: implications for nicotine self-administration and reinforcement. Psychopharmacology 169, 68–76. doi: 10.1007/s00213-003-1473-3
Eison, M. S., Stark, A. D., and Ellison, G. (1977). Opposed effects of locus coeruleus and substantia Nigra lesions on social behavior in rat colonies. Pharmacol. Biochem. Behav. 7, 87–90. doi: 10.1016/0091-3057(77)90016-8
Engeln, M., Ahmed, S. H., Vouillac, C., Tison, F., Bezard, E., and Fernagut, P.-O. (2013). Reinforcing properties of Pramipexole in normal and parkinsonian rats. Neurobiol. Dis. 49, 79–86. doi: 10.1016/j.nbd.2012.08.005
Evans, A. H., Pavese, N., Lawrence, A. D., Tai, Y. F., Appel, S., Doder, M., et al. (2006). Compulsive drug use linked to sensitized ventral striatal dopamine transmission. Ann. Neurol. 59, 852–858. doi: 10.1002/ana.20822
Everitt, B. J. (2014). Neural and psychological mechanisms underlying compulsive drug seeking habits and drug memories – indications for novel treatments of addiction. Eur. J. Neurosci. 40, 2163–2182. doi: 10.1111/ejn.12644
Everitt, B. J., and Robbins, T. W. (2005). Neural systems of reinforcement for drug addiction: from actions to habits to compulsion. Nat. Neurosci. 8, 1481–1489. doi: 10.1038/nn1579
Fanelli, R. R., Klein, J. T., Reese, R. M., and Robinson, D. L. (2013). Dorsomedial and dorsolateral striatum exhibit distinct phasic neuronal activity during alcohol self-administration in rats. Eur. J. Neurosci. 38, 2637–2648. doi: 10.1111/ejn.12271
Fantegrossi, W. E., Murnane, K. S., and Reissig, C. J. (2008). The behavioral pharmacology of hallucinogens. Biochem. Pharmacol. 75, 17–33. doi: 10.1016/j.bcp.2007.07.018
Ferguson, L. M., Ahrens, A. M., Longyear, L. G., and Aldridge, J. W. (2020). Neurons of the ventral tegmental area encode individual differences in motivational “wanting” for reward cues. J. Neurosci. 40, 8951–8963. doi: 10.1523/JNEUROSCI.2947-19.2020
Fields, H. L., Hjelmstad, G. O., Margolis, E. B., and Nicola, S. M. (2007). Ventral tegmental area neurons in learned appetitive behavior and positive reinforcement. Annu. Rev. Neurosci. 30, 289–316. doi: 10.1146/annurev.neuro.30.051606.094341
Flagel, S. B., Clark, J. J., Robinson, T. E., Mayo, L., Czuj, A., Willuhn, I., et al. (2011). A selective role for dopamine in reward learning. Nature 469, 53–57. doi: 10.1038/nature09588
Flagel, S. B., Watson, S. J., Robinson, T. E., and Akil, H. (2007). Individual differences in the propensity to approach signals vs goals promote different adaptations in the dopamine system of rats. Psychopharmacology 191, 599–607. doi: 10.1007/s00213-006-0535-8
Ford, C. P. (2014). The role of D2-autoreceptors in regulating dopamine neuron activity and transmission. Neuroscience 282, 13–22. doi: 10.1016/j.neuroscience.2014.01.025
Fouriezos, G., and Wise, R. A. (1976). Pimozide-induced extinction of intracranial self-stimulation: response patterns rule out motor or performance deficits. Brain Res. 103, 377–380. doi: 10.1016/0006-8993(76)90809-x
Fragale, J. E., James, M. H., and Aston-Jones, G. (2021). Intermittent self-administration of fentanyl induces a multifaceted addiction state associated with persistent changes in the orexin system. Addict. Biol. 26:e12946. doi: 10.1111/adb.12946
Freels, T. G., Baxter-Potter, L. N., Lugo, J. M., Glodosky, N. C., Wright, H. R., Baglot, S. L., et al. (2020). Vaporized cannabis extracts have reinforcing properties and support conditioned drug-seeking behavior in rats. J. Neurosci. 40, 1897–1908. doi: 10.1523/JNEUROSCI.2416-19.2020
Fukushiro, D. F., Olivera, A., Liu, Y., and Wang, Z. (2015). Neonatal exposure to amphetamine alters social affiliation and central dopamine activity in adult male prairie voles. Neuroscience 307, 109–116. doi: 10.1016/j.neuroscience.2015.08.051
Gardner, E. L. (2011). Addiction and brain reward and antireward pathways. Adv. Psychosom. Med. 30, 22–60. doi: 10.1159/000324065
Gentry, R. N., Lee, B., and Roesch, M. R. (2016). Phasic dopamine release in the rat nucleus accumbens predicts approach and avoidance performance. Nat. Commun. 7:13154. doi: 10.1038/ncomms13154
Gerfen, C. R. (1984). The neostriatal mosaic: compartmentalization of corticostriatal input and striatonigral output systems. Nature 311, 461–464. doi: 10.1038/311461a0
Gerfen, C. R. (2003). D1 dopamine receptor supersensitivity in the dopamine-depleted striatum animal model of Parkinson’s disease. Neuroscientist 9, 455–462. doi: 10.1177/1073858403255839
Giuliano, C., Belin, D., and Everitt, B. J. (2019). Compulsive alcohol seeking results from a failure to disengage dorsolateral striatal control over behavior. J. Neurosci. 39, 1744–1754. doi: 10.1523/JNEUROSCI.2615-18.2018
Giuliano, C., Peña-Oliver, Y., Goodlett, C. R., Cardinal, R. N., Robbins, T. W., Bullmore, E. T., et al. (2018). Evidence for a long-lasting compulsive alcohol seeking phenotype in rats. Neuropsychopharmacology 43, 728–738. doi: 10.1038/npp.2017.105
Goeders, N. E., and Guerin, G. F. (1994). Non-contingent electric footshock facilitates the acquisition of intravenous cocaine self-administration in rats. Psychopharmacology 114, 63–70. doi: 10.1007/BF02245445
Goldberg, N. R. S., Fields, V., Pflibsen, L., Salvatore, M. F., and Meshul, C. K. (2012). Social enrichment attenuates nigrostriatal lesioning and reverses motor impairment in a progressive 1-methyl-2-phenyl-1,2,3,6-tetrahydropyridine (MPTP) mouse model of Parkinson’s disease. Neurobiol. Dis. 45, 1051–1067. doi: 10.1016/j.nbd.2011.12.024
Gossop, M., Griffiths, P., Powis, B., and Strang, J. (1992). Severity of dependence and route of administration of heroin, cocaine and amphetamines. Br. J. Addict. 87, 1527–1536. doi: 10.1111/j.1360-0443.1992.tb02660.x
Griffiths, R. R., Brady, J. V., and Bradford, L. D. (1979). “Predicting the abuse liability of drugs with animal drug self-administration procedures: psychomotor stimulants and hallucinogens,” in Advances in Behavioral Pharmacology, Vol. 2, eds T. Thompson and P. B. Dews (Amsterdam: Elsevier), 163–208. doi: 10.1016/B978-0-12-004702-4.50010-2
Grimm, J. W., Hope, B. T., Wise, R. A., and Shaham, Y. (2001). Incubation of cocaine craving after withdrawal. Nature 412, 141–142. doi: 10.1038/35084134
Groman, S. M., Massi, B., Mathias, S. R., Lee, D., and Taylor, J. R. (2019). Model-free and model-based influences in addiction-related behaviors. Biol. Psychiatry 85, 936–945. doi: 10.1016/j.biopsych.2018.12.017
Groman, S. M., Rich, K. M., Smith, N. J., Lee, D., and Taylor, J. R. (2018). Chronic exposure to methamphetamine disrupts reinforcement-based decision making in rats. Neuropsychopharmacology 43, 770–780. doi: 10.1038/npp.2017.159
Gunaydin, L. A., Grosenick, L., Finkelstein, J. C., Kauvar, I. V., Fenno, L. E., Adhikari, A., et al. (2014). Natural neural projection dynamics underlying social behavior. Cell 157, 1535–1551. doi: 10.1016/j.cell.2014.05.017
Haber, S. N., Fudge, J. L., and McFarland, N. R. (2000). Striatonigrostriatal pathways in primates form an ascending spiral from the shell to the dorsolateral striatum. J. Neurosci. 20, 2369–2382. doi: 10.1523/JNEUROSCI.20-06-02369.2000
Hall, F. S., Wilkinson, L. S., Humby, T., and Robbins, T. W. (1999). Maternal deprivation of neonatal rats produces enduring changes in dopamine function. Synapse 32, 37–43.
Hassani, O. K., Cromwell, H. C., and Schultz, W. (2001). Influence of expectation of different rewards on behavior-related neuronal activity in the striatum. J. Neurophysiol. 85, 2477–2489. doi: 10.1152/jn.2001.85.6.2477
Hemby, S. E., Co, C., Dworkin, S. I., and Smith, J. E. (1999). Synergistic elevations in nucleus accumbens extracellular dopamine concentrations during self-administration of cocaine/heroin combinations (speedball) in rats. J. Pharmacol. Exp. Ther. 288, 274–280.
Hernandez, L., Lee, F., and Hoebel, B. G. (1987). Simultaneous microdialysis and amphetamine infusion in the nucleus accumbens and striatum of freely moving rats: increase in extracellular dopamine and serotonin. Brain Res. Bull. 19, 623–628. doi: 10.1016/0361-9230(87)90047-5
Higgins, S. T., Sigmon, S. C., Wong, C. J., Heil, S. H., Badger, G. J., Donham, R., et al. (2003). Community reinforcement therapy for cocaine-dependent outpatients. Arch. Gen. Psychiatry 60, 1043–1052. doi: 10.1001/archpsyc.60.9.1043
Hodebourg, R., Murray, J. E., Fouyssac, M., Puaud, M., Everitt, B. J., and Belin, D. (2019). Heroin seeking becomes dependent on dorsal striatal dopaminergic mechanisms and can be decreased by N-acetylcysteine. Eur. J. Neurosci. 50, 2036–2044. doi: 10.1111/ejn.13894
Hogarth, L. (2020). Addiction is driven by excessive goal-directed drug choice under negative affect: translational critique of habit and compulsion theory. Neuropsychopharmacology 45, 720–735. doi: 10.1038/s41386-020-0600-8
Hopf, F. W., and Lesscher, H. M. B. (2014). Rodent models for compulsive alcohol intake. Alcohol 48, 253–264. doi: 10.1016/j.alcohol.2014.03.001
Howe, M. W., and Dombeck, D. A. (2016). Rapid signalling in distinct dopaminergic axons during locomotion and reward. Nature 535, 505–510. doi: 10.1038/nature18942
Hsu, T. M., McCutcheon, J. E., and Roitman, M. F. (2018). Parallels and overlap: the integration of homeostatic signals by mesolimbic dopamine neurons. Front. Psychiatry 9:410. doi: 10.3389/fpsyt.2018.00410
Hughson, A. R., Horvath, A. P., Holl, K., Palmer, A. A., Solberg Woods, L. C., Robinson, T. E., et al. (2019). Incentive salience attribution, “sensation-seeking” and “novelty-seeking” are independent traits in a large sample of male and female heterogeneous stock rats. Sci. Rep. 9:2351. doi: 10.1038/s41598-019-39519-1
Hynes, T. J., Hrelja, K. M., Hathaway, B. A., Hounjet, C. D., Chernoff, C. S., Ebsary, S. A., et al. (2021). Dopamine neurons gate the intersection of cocaine use, decision making, and impulsivity. Addict. Biol. 26:e13022. doi: 10.1111/adb.13022
Ikemoto, S. (2007). Dopamine reward circuitry: two projection systems from the ventral midbrain to the nucleus accumbens-olfactory tubercle complex. Brain Res. Rev. 56, 27–78. doi: 10.1016/j.brainresrev.2007.05.004
Ikemoto, S., Yang, C., and Tan, A. (2015). Basal ganglia circuit loops, dopamine and motivation: a review and enquiry. Behav. Brain Res. 290, 17–31. doi: 10.1016/j.bbr.2015.04.018
Ito, R., Dalley, J. W., Howes, S. R., Robbins, T. W., and Everitt, B. J. (2000). Dissociation in conditioned dopamine release in the nucleus accumbens core and shell in response to cocaine cues and during cocaine-seeking behavior in rats. J. Neurosci. 20, 7489–7495. doi: 10.1523/JNEUROSCI.20-19-07489.2000
Ito, R., Dalley, J. W., Robbins, T. W., and Everitt, B. J. (2002). Dopamine release in the dorsal striatum during cocaine-seeking behavior under the control of a drug-associated cue. J. Neurosci. 22, 6247–6253. doi: 10.1523/JNEUROSCI.22-14-06247.2002
Izquierdo, A., Belcher, A. M., Scott, L., Cazares, V. A., Chen, J., O’Dell, S. J., et al. (2010). Reversal-specific learning impairments after a binge regimen of methamphetamine in rats: possible involvement of striatal dopamine. Neuropsychopharmacology 35, 505–514. doi: 10.1038/npp.2009.155
Jacobs, D. S., and Moghaddam, B. (2020). Prefrontal Cortex Representation of Learning of Punishment Probability During Reward-Motivated Actions. J. Neurosci. 40, 5063–5077. doi: 10.1523/JNEUROSCI.0310-20.2020
Ji, X., Saha, S., Kolpakova, J., Guildford, M., Tapper, A. R., and Martin, G. E. (2017). Dopamine receptors differentially control binge alcohol drinking-mediated synaptic plasticity of the core nucleus accumbens direct and indirect pathways. J. Neurosci. 37, 5463–5474. doi: 10.1523/JNEUROSCI.3845-16.2017
Joel, D., Niv, Y., and Ruppin, E. (2002). Actor-critic models of the basal ganglia: new anatomical and computational perspectives. Neural Netw. 15, 535–547. doi: 10.1016/s0893-6080(02)00047-3
Johnson, A. R., Thibeault, K. C., Lopez, A. J., Peck, E. G., Sands, L. P., Sanders, C. M., et al. (2019). Cues play a critical role in estrous cycle-dependent enhancement of cocaine reinforcement. Neuropsychopharmacology 44, 1189–1197. doi: 10.1038/s41386-019-0320-0
Johnson, S. W., and North, R. A. (1992). Opioids excite dopamine neurons by hyperpolarization of local interneurons. J. Neurosci. 12, 483–488.
Jonkman, S., Pelloux, Y., and Everitt, B. J. (2012). Differential Roles of the Dorsolateral and Midlateral Striatum in Punished Cocaine Seeking. J. Neurosci. 32, 4645–4650. doi: 10.1523/JNEUROSCI.0348-12.2012
Kawa, A. B., Allain, F., Robinson, T. E., and Samaha, A.-N. (2019a). The transition to cocaine addiction: the importance of pharmacokinetics for preclinical models. Psychopharmacology 236, 1145–1157. doi: 10.1007/s00213-019-5164-0
Kawa, A. B., Valenta, A. C., Kennedy, R. T., and Robinson, T. E. (2019b). Incentive and dopamine sensitization produced by intermittent but not long access cocaine self-administration. Eur. J. Neurosci. 50, 2663–2682. doi: 10.1111/ejn.14418
Kawa, A. B., Bentzley, B. S., and Robinson, T. E. (2016). Less is more: prolonged intermittent access cocaine self-administration produces incentive-sensitization and addiction-like behavior. Psychopharmacology 233, 3587–3602. doi: 10.1007/s00213-016-4393-8
Keath, J. R., Iacoviello, M. P., Barrett, L. E., Mansvelder, H. D., and McGehee, D. S. (2007). Differential modulation by nicotine of substantia nigra versus ventral tegmental area dopamine neurons. J. Neurophysiol. 98, 3388–3396. doi: 10.1152/jn.00760.2007
Keiflin, R., and Janak, P. H. (2015). Dopamine prediction errors in reward learning and addiction: from theory to neural circuitry. Neuron 88, 247–263. doi: 10.1016/j.neuron.2015.08.037
Kim, H. F., Ghazizadeh, A., and Hikosaka, O. (2015). Dopamine neurons encoding long-term memory of object value for habitual behavior. Cell 163, 1165–1175. doi: 10.1016/j.cell.2015.10.063
King, C. P., Tripi, J. A., Hughson, A. R., Horvath, A. P., Lamparelli, A. C., Holl, K. L., et al. (2021). Sensitivity to food and cocaine cues are independent traits in a large sample of heterogeneous stock rats. Sci. Rep. 11:2223. doi: 10.1038/s41598-020-80798-w
Kitamura, O., Wee, S., Specio, S. E., Koob, G. F., and Pulvirenti, L. (2006). Escalation of methamphetamine self-administration in rats: a dose-effect function. Psychopharmacology 186, 48–53. doi: 10.1007/s00213-006-0353-z
Klanker, M., Sandberg, T., Joosten, R., Willuhn, I., Feenstra, M., and Denys, D. (2015). Phasic dopamine release induced by positive feedback predicts individual differences in reversal learning. Neurobiol. Learn. Mem. 125, 135–145. doi: 10.1016/j.nlm.2015.08.011
Koob, G. F., and Bloom, F. E. (1988). Cellular and molecular mechanisms of drug dependence. Science 242, 715–723. doi: 10.1126/science.2903550
Kufahl, P. R., Zavala, A. R., Singh, A., Thiel, K. J., Dickey, E. D., Joyce, J. N., et al. (2009). C-Fos expression associated with reinstatement of cocaine-seeking behavior by response-contingent conditioned cues. Synapse 63, 823–835. doi: 10.1002/syn.20666
Kupchik, Y. M., Brown, R. M., Heinsbroek, J. A., Lobo, M. K., Schwartz, D. J., and Kalivas, P. W. (2015). Coding the direct/indirect pathways by D1 and D2 receptors is not valid for accumbens projections. Nat. Neurosci. 18, 1230–1232. doi: 10.1038/nn.4068
Lammel, S., Lim, B. K., and Malenka, R. C. (2014). Reward and aversion in a heterogeneous midbrain dopamine system. Neuropharmacology 76, 351–359. doi: 10.1016/j.neuropharm.2013.03.019
Lawrence, A. D., Evans, A. H., and Lees, A. J. (2003). Compulsive use of dopamine replacement therapy in Parkinson’s disease: reward systems gone awry? Lancet Neurol. 2, 595–604. doi: 10.1016/s1474-4422(03)00529-5
Le Heron, C., Kolling, N., Plant, O., Kienast, A., Janska, R., Ang, Y.-S., et al. (2020). Dopamine modulates dynamic decision-making during foraging. J. Neurosci. 40, 5273–5282. doi: 10.1523/JNEUROSCI.2586-19.2020
Le Heron, C., Plant, O., Manohar, S., Ang, Y.-S., Jackson, M., Lennox, G., et al. (2018). Distinct effects of apathy and dopamine on effort-based decision-making in Parkinson’s disease. Brain 141, 1455–1469. doi: 10.1093/brain/awy110
Lenoir, M., Serre, F., Cantin, L., and Ahmed, S. H. (2007). Intense sweetness surpasses cocaine reward. PLoS One 2:e698. doi: 10.1371/journal.pone.0000698
Leonard, M. Z., DeBold, J. F., and Miczek, K. A. (2017). Escalated cocaine “binges” in rats: enduring effects of social defeat stress or intra-VTA CRF. Psychopharmacology 234, 2823–2836. doi: 10.1007/s00213-017-4677-7
Leri, F., Bruneau, J., and Stewart, J. (2003). Understanding polydrug use: review of heroin and cocaine co-use. Addiction 98, 7–22. doi: 10.1046/j.1360-0443.2003.00236.x
Lerner, T. N., Shilyansky, C., Davidson, T. J., Evans, K. E., Beier, K. T., Zalocusky, K. A., et al. (2015). Intact-brain analyses reveal distinct information carried by SNc dopamine subcircuits. Cell 162, 635–647. doi: 10.1016/j.cell.2015.07.014
Lesscher, H. M. B., Spoelder, M., Rotte, M. D., Janssen, M. J., Hesseling, P., Lozeman-van’t Klooster, J. G., et al. (2015). Early social isolation augments alcohol consumption in rats. Behav. Pharmacol. 26, 673–680. doi: 10.1097/FBP.0000000000000165
Leyton, M. (2017). Altered dopamine transmission as a familial risk trait for addictions. Curr. Opin. Behav. Sci. 13, 130–138. doi: 10.1016/j.cobeha.2016.11.011
Leyton, M., and Vezina, P. (2014). Dopamine ups and downs in vulnerability to addictions: a neurodevelopmental model. Trends Pharmacol. Sci. 35, 268–276. doi: 10.1016/j.tips.2014.04.002
Liu, Y., Aragona, B. J., Young, K. A., Dietz, D. M., Kabbaj, M., Mazei-Robison, M., et al. (2010). Nucleus accumbens dopamine mediates amphetamine-induced impairment of social bonding in a monogamous rodent species. Proc. Natl. Acad. Sci. U.S.A. 107, 1217–1222. doi: 10.1073/pnas.0911998107
Liu, Y., Jean-Richard-Dit-Bressel, P., Yau, J. O.-Y., Willing, A., Prasad, A. A., Power, J. M., et al. (2020). The mesolimbic dopamine activity signatures of relapse to alcohol-seeking. J. Neurosci. 40, 6409–6427. doi: 10.1523/JNEUROSCI.0724-20.2020
Lobo, M. K., Covington, H. E., Chaudhury, D., Friedman, A. K., Sun, H., Damez-Werno, D., et al. (2010). Cell type-specific loss of BDNF signaling mimics optogenetic control of cocaine reward. Science 330, 385–390. doi: 10.1126/science.1188472
Loiodice, S., Wing Young, H., Rion, B., Méot, B., Montagne, P., Denibaud, A.-S., et al. (2019). Implication of nigral dopaminergic lesion and repeated L-dopa exposure in neuropsychiatric symptoms of Parkinson’s disease. Behav. Brain Res. 360, 120–127. doi: 10.1016/j.bbr.2018.12.007
Lucerne, K. E., Osman, A., Meckel, K. R., and Kiraly, D. D. (2021). Contributions of neuroimmune and gut-brain signaling to vulnerability of developing substance use disorders. Neuropharmacology 192:108598. doi: 10.1016/j.neuropharm.2021.108598
Lüscher, C., and Malenka, R. C. (2011). Drug-evoked synaptic plasticity in addiction: from molecular changes to circuit remodeling. Neuron 69, 650–663. doi: 10.1016/j.neuron.2011.01.017
Lüscher, C., Robbins, T. W., and Everitt, B. J. (2020). The transition to compulsion in addiction. Nat. Rev. Neurosci. 21, 247–263. doi: 10.1038/s41583-020-0289-z
Mahler, S. V., and Aston-Jones, G. S. (2012). Fos activation of selective afferents to ventral tegmental area during cue-induced reinstatement of cocaine seeking in rats. J. Neurosci. 32, 13309–13325. doi: 10.1523/JNEUROSCI.2277-12.2012
Mahler, S. V., Brodnik, Z. D., Cox, B. M., Buchta, W. C., Bentzley, B. S., Quintanilla, J., et al. (2019). Chemogenetic manipulations of ventral tegmental area dopamine neurons reveal multifaceted roles in cocaine abuse. J. Neurosci. 39, 503–518. doi: 10.1523/JNEUROSCI.0537-18.2018
Mameli, M., Halbout, B., Creton, C., Engblom, D., Parkitna, J. R., Spanagel, R., et al. (2009). Cocaine-evoked synaptic plasticity: persistence in the VTA triggers adaptations in the NAc. Nat. Neurosci. 12, 1036–1041. doi: 10.1038/nn.2367
Manduca, A., Servadio, M., Damsteegt, R., Campolongo, P., Vanderschuren, L. J., and Trezza, V. (2016). Dopaminergic Neurotransmission in the Nucleus Accumbens Modulates Social Play Behavior in Rats. Neuropsychopharmacology 41, 2215–2223. doi: 10.1038/npp.2016.22
Marchant, N. J., Khuc, T. N., Pickens, C. L., Bonci, A., and Shaham, Y. (2013). Context-induced relapse to alcohol seeking after punishment in a rat model. Biol. Psychiatry 73, 256–262. doi: 10.1016/j.biopsych.2012.07.007
Mark, G. P., Shabani, S., Dobbs, L. K., and Hansen, S. T. (2011). Cholinergic modulation of mesolimbic dopamine function and reward. Physiol. Behav. 104, 76–81. doi: 10.1016/j.physbeh.2011.04.052
Marusich, J. A., Wiley, J. L., Silinski, M. A. R., Thomas, B. F., Meredith, S. E., Gahl, R. F., et al. (2019). Comparison of cigarette, little cigar, and waterpipe tobacco smoke condensate and e-cigarette aerosol condensate in a self-administration model. Behav. Brain Res. 372:112061. doi: 10.1016/j.bbr.2019.112061
Mateo, Y., Lack, C. M., Morgan, D., Roberts, D. C. S., and Jones, S. R. (2005). Reduced dopamine terminal function and insensitivity to cocaine following cocaine binge self-administration and deprivation. Neuropsychopharmacology 30, 1455–1463. doi: 10.1038/sj.npp.1300687
Matheus, F. C., Rial, D., Real, J. I., Lemos, C., Takahashi, R. N., Bertoglio, L. J., et al. (2016). Temporal dissociation of striatum and prefrontal cortex uncouples Anhedonia and defense behaviors relevant to depression in 6-OHDA-lesioned rats. Mol. Neurobiol. 53, 3891–3899. doi: 10.1007/s12035-015-9330-z
Mccabe, S. E., Cranford, J. A., Morales, M., and Young, A. (2006). Simultaneous and concurrent polydrug use of alcohol and prescription drugs: prevalence, correlates, and consequences. J. Stud. Alcohol 67, 529–537. doi: 10.15288/jsa.2006.67.529
McCall, N. M., Kotecki, L., Dominguez-Lopez, S., Marron Fernandez de Velasco, E., Carlblom, N., Sharpe, A. L., et al. (2017). Selective ablation of GIRK channels in dopamine neurons alters behavioral effects of cocaine in mice. Neuropsychopharmacology 42, 707–715. doi: 10.1038/npp.2016.138
McCutcheon, J. E., and Marinelli, M. (2009). Age matters. Eur. J. Neurosci. 29, 997–1014. doi: 10.1111/j.1460-9568.2009.06648.x
McFarland, K., and Kalivas, P. W. (2001). The circuitry mediating cocaine-induced reinstatement of drug-seeking behavior. J. Neurosci. 21, 8655–8663. doi: 10.1523/JNEUROSCI.21-21-08655.2001
Menegas, W., Akiti, K., Amo, R., Uchida, N., and Watabe-Uchida, M. (2018). Dopamine neurons projecting to the posterior striatum reinforce avoidance of threatening stimuli. Nat. Neurosci. 21, 1421–1430. doi: 10.1038/s41593-018-0222-1
Mereu, G., Yoon, K. W., Boi, V., Gessa, G. L., Naes, L., and Westfall, T. C. (1987). Preferential stimulation of ventral tegmental area dopaminergic neurons by nicotine. Eur. J. Pharmacol. 141, 395–399. doi: 10.1016/0014-2999(87)90556-5
Meyers, R. J., Roozen, H. G., and Smith, J. E. (2011). The community reinforcement approach. Alcohol Res. Health 33, 380–388.
Mohammadkhani, A., Fragale, J. E., Pantazis, C. B., Bowrey, H. E., James, M. H., and Aston-Jones, G. (2019). Orexin-1 receptor signaling in ventral pallidum regulates motivation for the opioid remifentanil. J. Neurosci. 39, 9831–9840. doi: 10.1523/JNEUROSCI.0255-19.2019
Mohebi, A., Pettibone, J. R., Hamid, A. A., Wong, J.-M. T., Vinson, L. T., Patriarchi, T., et al. (2019). Dissociable dopamine dynamics for learning and motivation. Nature 570, 65–70. doi: 10.1038/s41586-019-1235-y
Monroe, S. C., and Radke, A. K. (2021). Aversion-resistant fentanyl self-administration in mice. Psychopharmacology 238, 699–710. doi: 10.1007/s00213-020-05722-6
Morales, M., and Margolis, E. B. (2017). Ventral tegmental area: cellular heterogeneity, connectivity and behaviour. Nat. Rev. Neurosci. 18, 73–85. doi: 10.1038/nrn.2016.165
Morel, C., Montgomery, S., and Han, M.-H. (2019). Nicotine and alcohol: the role of midbrain dopaminergic neurons in drug reinforcement. Eur. J. Neurosci. 50, 2180–2200. doi: 10.1111/ejn.14160
Murray, J. E., Belin, D., and Everitt, B. J. (2012). Double dissociation of the dorsomedial and dorsolateral striatal control over the acquisition and performance of cocaine seeking. Neuropsychopharmacology 37, 2456–2466. doi: 10.1038/npp.2012.104
Nair-Roberts, R. G., Chatelain-Badie, S. D., Benson, E., White-Cooper, H., Bolam, J. P., and Ungless, M. A. (2008). Stereological estimates of dopaminergic, GABAergic and glutamatergic neurons in the ventral tegmental area, substantia nigra and retrorubral field in the rat. Neuroscience 152, 1024–1031. doi: 10.1016/j.neuroscience.2008.01.046
Napier, T. C., Kirby, A., and Persons, A. L. (2020). The role of dopamine pharmacotherapy and addiction-like behaviors in Parkinson’s disease. Prog. Neuropsychopharmacol. Biol. Psychiatry 102:109942. doi: 10.1016/j.pnpbp.2020.109942
Nasrallah, N. A., Clark, J. J., Collins, A. L., Akers, C. A., Phillips, P. E., and Bernstein, I. L. (2011). Risk preference following adolescent alcohol use is associated with corrupted encoding of costs but not rewards by mesolimbic dopamine. Proc. Natl. Acad. Sci. U.S.A. 108, 5466–5471. doi: 10.1073/pnas.1017732108
Nestler, E. J., and Lüscher, C. (2019). The molecular basis of drug addiction: linking epigenetic to synaptic and circuit mechanisms. Neuron 102, 48–59. doi: 10.1016/j.neuron.2019.01.016
Nguyen, D., Alushaj, E., Erb, S., and Ito, R. (2019). Dissociative effects of dorsomedial striatum D1 and D2 receptor antagonism in the regulation of anxiety and learned approach-avoidance conflict decision-making. Neuropharmacology 146, 222–230. doi: 10.1016/j.neuropharm.2018.11.040
Nguyen, J. D., Aarde, S. M., Vandewater, S. A., Grant, Y., Stouffer, D. G., Parsons, L. H., et al. (2016). Inhaled delivery of Δ9-tetrahydrocannabinol (THC) to rats by e-cigarette vapor technology. Neuropharmacology 109, 112–120. doi: 10.1016/j.neuropharm.2016.05.021
Nutt, D. J., Lingford-Hughes, A., Erritzoe, D., and Stokes, P. R. A. (2015). The dopamine theory of addiction: 40 years of highs and lows. Nat. Rev. Neurosci. 16, 305–312. doi: 10.1038/nrn3939
O’Dell, L. E., Chen, S. A., Smith, R. T., Specio, S. E., Balster, R. L., Paterson, N. E., et al. (2007). Extended access to nicotine self-administration leads to dependence: circadian measures, withdrawal measures, and extinction behavior in rats. J. Pharmacol. Exp. Ther. 320, 180–193. doi: 10.1124/jpet.106.105270
Oleson, E. B., and Cheer, J. F. (2013). On the role of subsecond dopamine release in conditioned avoidance. Front. Neurosci. 7:96. doi: 10.3389/fnins.2013.00096
Oleson, E. B., Gentry, R. N., Chioma, V. C., and Cheer, J. F. (2012). Subsecond dopamine release in the nucleus accumbens predicts conditioned punishment and its successful avoidance. J. Neurosci. 32, 14804–14808. doi: 10.1523/JNEUROSCI.3087-12.2012
Oleson, E. B., and Roberts, D. C. (2009). Behavioral economic assessment of price and cocaine consumption following self-administration histories that produce escalation of either final ratios or intake. Neuropsychopharmacology 34, 796–804. doi: 10.1038/npp.2008.195
Oliver, R. J., Purohit, D. C., Kharidia, K. M., and Mandyam, C. D. (2019). Transient Chemogenetic inhibition of D1-MSNs in the dorsal striatum enhances methamphetamine self-administration. Brain Sci. 9:330. doi: 10.3390/brainsci9110330
Olson, V. G., and Nestler, E. J. (2007). Topographical organization of GABAergic neurons within the ventral tegmental area of the rat. Synapse 61, 87–95. doi: 10.1002/syn.20345
O’Neal, T. J., Nooney, M. N., Thien, K., and Ferguson, S. M. (2020). Chemogenetic modulation of accumbens direct or indirect pathways bidirectionally alters reinstatement of heroin-seeking in high- but not low-risk rats. Neuropsychopharmacology 45, 1251–1262. doi: 10.1038/s41386-019-0571-9
Orsini, C. A., Moorman, D. E., Young, J. W., Setlow, B., and Floresco, S. B. (2015). Neural mechanisms regulating different forms of risk-related decision-making: insights from animal models. Neurosci. Biobehav. Rev. 58, 147–167. doi: 10.1016/j.neubiorev.2015.04.009
Ostlund, S. B. (2019). The push and pull of dopamine in cue-reward learning. Learn. Behav. 47, 273–274. doi: 10.3758/s13420-018-0370-x
Ostlund, S. B., LeBlanc, K. H., Kosheleff, A. R., Wassum, K. M., and Maidment, N. T. (2014). Phasic mesolimbic dopamine signaling encodes the facilitation of incentive motivation produced by repeated cocaine exposure. Neuropsychopharmacology 39, 2441–2449. doi: 10.1038/npp.2014.96
Park, J., and Moghaddam, B. (2017). Risk of punishment influences discrete and coordinated encoding of reward-guided actions by prefrontal cortex and VTA neurons. eLife 6:e30056. doi: 10.7554/eLife.30056
Pascoli, V., Terrier, J., Hiver, A., and Lüscher, C. (2015). Sufficiency of mesolimbic dopamine neuron stimulation for the progression to addiction. Neuron 88, 1054–1066. doi: 10.1016/j.neuron.2015.10.017
Pascoli, V., Turiault, M., and Lüscher, C. (2012). Reversal of cocaine-evoked synaptic potentiation resets drug-induced adaptive behaviour. Nature 481, 71–75. doi: 10.1038/nature10709
Pelloux, Y., Everitt, B. J., and Dickinson, A. (2007). Compulsive drug seeking by rats under punishment: effects of drug taking history. Psychopharmacology 194, 127–137. doi: 10.1007/s00213-007-0805-0
Peterson, V. L., Richards, J. B., Meyer, P. J., Cabrera-Rubio, R., Tripi, J. A., King, C. P., et al. (2020). Sex-dependent associations between addiction-related behaviors and the microbiome in outbred rats. EBioMedicine 55:102769. doi: 10.1016/j.ebiom.2020.102769
Phillips, P. E. M., Stuber, G. D., Heien, M. L. A. V., Wightman, R. M., and Carelli, R. M. (2003). Subsecond dopamine release promotes cocaine seeking. Nature 422, 614–618. doi: 10.1038/nature01476
Piray, P., Keramati, M. M., Dezfouli, A., Lucas, C., and Mokri, A. (2010). Individual differences in nucleus accumbens dopamine receptors predict development of addiction-like behavior: a computational approach. Neural Comput. 22, 2334–2368. doi: 10.1162/NECO_a_00009
Pontieri, F. E., Tanda, G., and Chiara, G. D. (1995). Intravenous cocaine, morphine, and amphetamine preferentially increase extracellular dopamine in the “shell” as compared with the “core” of the rat nucleus accumbens. Proc. Natl. Acad. Sci. U.S.A. 92, 12304–12308. doi: 10.1073/pnas.92.26.12304
Pontieri, F. E., Tanda, G., Orzi, F., and Chiara, G. D. (1996). Effects of nicotine on the nucleus accumbens and similarity to those of addictive drugs. Nature 382, 255–257. doi: 10.1038/382255a0
Porrino, L. J., Lyons, D., Smith, H. R., Daunais, J. B., and Nader, M. A. (2004). Cocaine self-administration produces a progressive involvement of limbic, association, and sensorimotor striatal domains. J. Neurosci. 24, 3554–3562. doi: 10.1523/JNEUROSCI.5578-03.2004
Ranaldi, R., and Wise, R. A. (2001). Blockade of D1 dopamine receptors in the ventral tegmental area decreases cocaine reward: possible role for dendritically released dopamine. J. Neurosci. 21, 5841–5846. doi: 10.1523/JNEUROSCI.21-15-05841.2001
Ray, L. A., Courtney, K. E., Hutchison, K. E., MacKillop, J., Galvan, A., and Ghahremani, D. G. (2014). Initial evidence that Oprm1 genotype moderates ventral and dorsal striatum functional connectivity during alcohol cues. Alcohol. Clin. Exp. Res. 38, 78–89. doi: 10.1111/acer.12136
Ritz, M. C., Lamb, R. J., Goldberg, S. R., and Kuhar, M. J. (1987). Cocaine receptors on dopamine transporters are related to self-administration of cocaine. Science 237, 1219–1223. doi: 10.1126/science.2820058
Roberts, A. J., Heyser, C. J., Cole, M., Griffin, P., and Koob, G. F. (2000). Excessive ethanol drinking following a history of dependence: animal model of allostasis. Neuropsychopharmacology 22, 581–594. doi: 10.1016/S0893-133X(99)00167-0
Robinson, D. L., Heien, M. L. A. V., and Wightman, R. M. (2002). Frequency of dopamine concentration transients increases in dorsal and ventral striatum of male rats during introduction of conspecifics. J. Neurosci. 22, 10477–10486. doi: 10.1523/JNEUROSCI.22-23-10477.2002
Robinson, T. E., and Berridge, K. C. (1993). The neural basis of drug craving: an incentive-sensitization theory of addiction. Brain Res. Brain Res. Rev. 18, 247–291. doi: 10.1016/0165-0173(93)90013-p
Robinson, T. E., and Berridge, K. C. (2001). Incentive-sensitization and addiction. Addiction 96, 103–114. doi: 10.1046/j.1360-0443.2001.9611038.x
Robinson, T. E., Jurson, P. A., Bennett, J. A., and Bentgen, K. M. (1988). Persistent sensitization of dopamine neurotransmission in ventral striatum (nucleus accumbens) produced by prior experience with (+)-amphetamine: a microdialysis study in freely moving rats. Brain Res. 462, 211–222. doi: 10.1016/0006-8993(88)90549-5
Samaha, A.-N., Khoo, S. Y.-S., Ferrario, C. R., and Robinson, T. E. (2021). Dopamine “ups and downs” in addiction revisited. Trends Neurosci. 44, 516–526. doi: 10.1016/j.tins.2021.03.003
Sasagawa, T., Horii-Hayashi, N., Okuda, A., Hashimoto, T., Azuma, C., and Nishi, M. (2017). Long-term effects of maternal separation coupled with social isolation on reward seeking and changes in dopamine D1 receptor expression in the nucleus accumbens via DNA methylation in mice. Neurosci. Lett. 641, 33–39. doi: 10.1016/j.neulet.2017.01.025
Saunders, B. T., Richard, J. M., Margolis, E. B., and Janak, P. H. (2018). Dopamine neurons create Pavlovian conditioned stimuli with circuit-defined motivational properties. Nat. Neurosci. 21, 1072–1083. doi: 10.1038/s41593-018-0191-4
Saunders, B. T., and Robinson, T. E. (2012). The role of dopamine in the accumbens core in the expression of Pavlovian conditioned responses. Eur. J. Neurosci. 36, 2521–2532. doi: 10.1111/j.1460-9568.2012.08217.x
Saunders, B. T., Yager, L. M., and Robinson, T. E. (2013). Cue-evoked cocaine “craving”: role of dopamine in the accumbens core. J. Neurosci. 33, 13989–14000. doi: 10.1523/JNEUROSCI.0450-13.2013
Schenk, S., Lacelle, G., Gorman, K., and Amit, Z. (1987). Cocaine self-administration in rats influenced by environmental conditions: Implications for the etiology of drug abuse. Neurosci. Lett. 81, 227–231. doi: 10.1016/0304-3940(87)91003-2
Schindler, A. G., Soden, M. E., Zweifel, L. S., and Clark, J. J. (2016). Reversal of alcohol-induced dysregulation in dopamine network dynamics may rescue maladaptive decision-making. J. Neurosci. 36, 3698–3708. doi: 10.1523/JNEUROSCI.4394-15.2016
Schmitz, Y., Benoit-Marand, M., Gonon, F., and Sulzer, D. (2003). Presynaptic regulation of dopaminergic neurotransmission. J. Neurochem. 87, 273–289. doi: 10.1046/j.1471-4159.2003.02050.x
Schumacher, J. D., van Holstein, M., Bagrodia, V., Le Bouder, H. B., and Floresco, S. B. (2021). Dorsomedial striatal contributions to different forms of risk/reward decision making. Neurobiol. Learn. Mem. 178:107369. doi: 10.1016/j.nlm.2020.107369
Scribner, J. L., Vance, E. A., Protter, D. S. W., Sheeran, W. M., Saslow, E., Cameron, R. T., et al. (2020). A neuronal signature for monogamous reunion. Proc. Natl. Acad. Sci. U.S.A. 117, 11076–11084. doi: 10.1073/pnas.1917287117
Sedighim, S., Carrette, L. L., Venniro, M., Shaham, Y., de Guglielmo, G., and George, O. (2021). Individual differences in addiction-like behaviors and choice between cocaine versus food in Heterogeneous Stock rats. Psychopharmacology. doi: 10.1007/s00213-021-05961-1 [Epub ahead of print].
Serra, V., Fattore, L., Scherma, M., Collu, R., Spano, M. S., Fratta, W., et al. (2015). Behavioural and neurochemical assessment of salvinorin A abuse potential in the rat. Psychopharmacology 232, 91–100. doi: 10.1007/s00213-014-3641-z
Shaham, Y., Shalev, U., Lu, L., de Wit, H., and Stewart, J. (2003). The reinstatement model of drug relapse: history, methodology and major findings. Psychopharmacology 168, 3–20. doi: 10.1007/s00213-002-1224-x
Siciliano, C. A., Ferris, M. J., and Jones, S. R. (2015). Cocaine self-administration disrupts mesolimbic dopamine circuit function and attenuates dopaminergic responsiveness to cocaine. Eur. J. Neurosci. 42, 2091–2096. doi: 10.1111/ejn.12970
Simms, J. A., Steensland, P., Medina, B., Abernathy, K. E., Chandler, L. J., Wise, R., et al. (2008). Intermittent access to 20% ethanol induces high ethanol consumption in Long-Evans and Wistar rats. Alcohol. Clin. Exp. Res. 32, 1816–1823. doi: 10.1111/j.1530-0277.2008.00753.x
Singer, B. F., Fadanelli, M., Kawa, A. B., and Robinson, T. E. (2018). Are cocaine-seeking “Habits” necessary for the development of addiction-like behavior in rats? J. Neurosci. 38, 60–73. doi: 10.1523/JNEUROSCI.2458-17.2017
Solinas, M., Belujon, P., Fernagut, P. O., Jaber, M., and Thiriet, N. (2019). Dopamine and addiction: what have we learned from 40 years of research. J. Neural Transm. 126, 481–516. doi: 10.1007/s00702-018-1957-2
Stalnaker, T. A., Takahashi, Y., Roesch, M. R., and Schoenbaum, G. (2009). Neural substrates of cognitive inflexibility after chronic cocaine exposure. Neuropharmacology 56, 63–72. doi: 10.1016/j.neuropharm.2008.07.019
Steinberg, E. E., Boivin, J. R., Saunders, B. T., Witten, I. B., Deisseroth, K., and Janak, P. H. (2014). Positive reinforcement mediated by midbrain dopamine neurons requires D1 and D2 receptor activation in the nucleus accumbens. PLoS One 9:e94771. doi: 10.1371/journal.pone.0094771
Stelly, C. E., Pomrenze, M. B., Cook, J. B., and Morikawa, H. (2016). Repeated social defeat stress enhances glutamatergic synaptic plasticity in the VTA and cocaine place conditioning. eLife 5:e15448. doi: 10.7554/eLife.15448
Stennett, B. A., Padovan-Hernandez, Y., and Knackstedt, L. A. (2020). Sequential cocaine-alcohol self-administration produces adaptations in rat nucleus accumbens core glutamate homeostasis that are distinct from those produced by cocaine self-administration alone. Neuropsychopharmacology 45, 441–450. doi: 10.1038/s41386-019-0452-2
Stewart, J. (1984). Reinstatement of heroin and cocaine self-administration behavior in the rat by intracerebral application of morphine in the ventral tegmental area. Pharmacol. Biochem. Behav. 20, 917–923. doi: 10.1016/0091-3057(84)90017-0
Stewart, J. (2008). Psychological and neural mechanisms of relapse. Philos. Trans. R. Soc. Lond. B Biol. Sci. 363, 3147–3158. doi: 10.1098/rstb.2008.0084
Stitzer, M. L., Jones, H. E., Tuten, M., and Wong, C. (2011). “Community reinforcement approach and contingency management interventions for substance abuse,” in Handbook of Motivational Counseling: Goal-Based Approaches to Assessment and Intervention with Addiction and other Problems, 2nd Edn, eds M. Cox and E. Klinger (Hoboken, NJ: Wiley Blackwell), 549–569. doi: 10.1002/9780470979952.ch23
Stopper, C. M., Tse, M. T. L., Montes, D. R., Wiedman, C. R., and Floresco, S. B. (2014). Overriding phasic dopamine signals redirects action selection during risk/reward decision making. Neuron 84, 177–189. doi: 10.1016/j.neuron.2014.08.033
Substance Abuse and Mental Health Services Administration (2019). Key substance use and Mental Health Indicators in the United States: Results from the 2018 National Survey on Drug Use and Health. Rockville, MD: Substance Abuse and Mental Health Services Administration.
Sugam, J. A., Day, J. J., Wightman, R. M., and Carelli, R. M. (2012). Phasic nucleus accumbens dopamine encodes risk-based decision-making behavior. Biol. Psychiatry 71, 199–205. doi: 10.1016/j.biopsych.2011.09.029
Swanson, L. W. (1982). The projections of the ventral tegmental area and adjacent regions: a combined fluorescent retrograde tracer and immunofluorescence study in the rat. Brain Res. Bull. 9, 321–353. doi: 10.1016/0361-9230(82)90145-9
Tadaiesky, M. T., Dombrowski, P. A., Figueiredo, C. P., Cargnin-Ferreira, E., Da Cunha, C., and Takahashi, R. N. (2008). Emotional, cognitive and neurochemical alterations in a premotor stage model of Parkinson’s disease. Neuroscience 156, 830–840. doi: 10.1016/j.neuroscience.2008.08.035
Thomas, M. J., Kalivas, P. W., and Shaham, Y. (2008). Neuroplasticity in the mesolimbic dopamine system and cocaine addiction. Br. J. Pharmacol. 154, 327–342. doi: 10.1038/bjp.2008.77
Thompson, B. L., Oscar-Berman, M., and Kaplan, G. B. (2021). Opioid-induced structural and functional plasticity of medium-spiny neurons in the nucleus accumbens. Neurosci. Biobehav. Rev. 120, 417–430. doi: 10.1016/j.neubiorev.2020.10.015
Thompson, D., Martini, L., and Whistler, J. L. (2010). Altered ratio of D1 and D2 dopamine receptors in mouse striatum is associated with behavioral sensitization to cocaine. PLoS One 5:e11038. doi: 10.1371/journal.pone.0011038
Thomsen, M., Barrett, A. C., Negus, S. S., and Caine, S. B. (2013). Cocaine versus food choice procedure in rats: environmental manipulations and effects of amphetamine. J. Exp. Anal. Behav. 99, 211–233. doi: 10.1002/jeab.15
Tiffany, S. T. (1990). A cognitive model of drug urges and drug-use behavior: role of automatic and nonautomatic processes. Psychol. Rev. 97, 147–168. doi: 10.1037/0033-295x.97.2.147
Tornatzky, W., and Miczek, K. A. (2000). Cocaine self-administration “binges”: transition from behavioral and autonomic regulation toward homeostatic dysregulation in rats. Psychopharmacology 148, 289–298. doi: 10.1007/s002130050053
Trezza, V., Baarendse, P. J. J., and Vanderschuren, L. J. M. J. (2014). On the interaction between drugs of abuse and adolescent social behavior. Psychopharmacology 231, 1715–1729. doi: 10.1007/s00213-014-3471-z
Trujillo, K. A., Smith, M. L., and Guaderrama, M. M. (2011). Powerful behavioral interactions between methamphetamine and morphine. Pharmacol. Biochem. Behav. 99, 451–458. doi: 10.1016/j.pbb.2011.04.014
Tsutsui-Kimura, I., Matsumoto, H., Akiti, K., Yamada, M. M., Uchida, N., and Watabe-Uchida, M. (2020). Distinct temporal difference error signals in dopamine axons in three regions of the striatum in a decision-making task. eLife 9:e62390. doi: 10.7554/eLife.62390
Ungless, M. A., and Grace, A. A. (2012). Are you or aren’t you? Challenges associated with physiologically identifying dopamine neurons. Trends Neurosci. 35, 422–430. doi: 10.1016/j.tins.2012.02.003
Ungless, M. A., Whistler, J. L., Malenka, R. C., and Bonci, A. (2001). Single cocaine exposure in vivo induces long-term potentiation in dopamine neurons. Nature 411, 583–587. doi: 10.1038/35079077
Valyear, M. D., Glovaci, I., Zaari, A., Lahlou, S., Trujillo-Pisanty, I., Andrew Chapman, C., et al. (2020). Dissociable mesolimbic dopamine circuits control responding triggered by alcohol-predictive discrete cues and contexts. Nat. Commun. 11:3764. doi: 10.1038/s41467-020-17543-4
Vandaele, Y., and Ahmed, S. H. (2021). Habit, choice, and addiction. Neuropsychopharmacology 46, 689–698. doi: 10.1038/s41386-020-00899-y
Vanderschuren, L. J., Minnaard, A. M., Smeets, J. A., and Lesscher, H. M. (2017). Punishment models of addictive behavior. Curr. Opin. Behav. Sci. 13, 77–84. doi: 10.1016/j.cobeha.2016.10.007
Vanderschuren, L. J. M. J., and Ahmed, S. H. (2013). Animal studies of addictive behavior. Cold Spring Harb. Perspect. Med. 3:a011932. doi: 10.1101/cshperspect.a011932
Vanderschuren, L. J. M. J., Ciano, P. D., and Everitt, B. J. (2005). Involvement of the dorsal striatum in cue-controlled cocaine seeking. J. Neurosci. 25, 8665–8670. doi: 10.1523/JNEUROSCI.0925-05.2005
Vanderschuren, L. J. M. J., and Everitt, B. J. (2004). Drug seeking becomes compulsive after prolonged cocaine self-administration. Science 305, 1017–1019. doi: 10.1126/science.1098975
Vanderschuren, L. J. M. J., Niesink, R. J. M., and Van Pee, J. M. (1997). The neurobiology of social play behavior in rats. Neurosci. Biobehav. Rev. 21, 309–326. doi: 10.1016/S0149-7634(96)00020-6
Venniro, M., Banks, M. L., Heilig, M., Epstein, D. H., and Shaham, Y. (2020). Improving translation of animal models of addiction and relapse by reverse translation. Nat. Rev. Neurosci. 21, 625–643. doi: 10.1038/s41583-020-0378-z
Venniro, M., Caprioli, D., and Shaham, Y. (2016). Animal models of drug relapse and craving: from drug priming-induced reinstatement to incubation of craving after voluntary abstinence. Prog. Brain Res. 224, 25–52. doi: 10.1016/bs.pbr.2015.08.004
Venniro, M., Russell, T. I., Zhang, M., and Shaham, Y. (2019). Operant social reward decreases incubation of heroin craving in male and female rats. Biol. Psychiatry 86, 848–856. doi: 10.1016/j.biopsych.2019.05.018
Venniro, M., Zhang, M., Caprioli, D., Hoots, J. K., Golden, S. A., Heins, C., et al. (2018). Volitional social interaction prevents drug addiction in rat models. Nat. Neurosci. 21, 1520–1529. doi: 10.1038/s41593-018-0246-6
Verharen, J. P. H., de Jong, J. W., Roelofs, T. J. M., Huffels, C. F. M., van Zessen, R., Luijendijk, M. C. M., et al. (2018). A neuronal mechanism underlying decision-making deficits during hyperdopaminergic states. Nat. Commun. 9:731. doi: 10.1038/s41467-018-03087-1
Verharen, J. P. H., Luijendijk, M. C. M., Vanderschuren, L. J. M. J., and Adan, R. A. H. (2020). Dopaminergic contributions to behavioral control under threat of punishment in rats. Psychopharmacology 237, 1769–1782. doi: 10.1007/s00213-020-05497-w
Verheij, M. M. M., and Cools, A. R. (2008). Twenty years of dopamine research: individual differences in the response of accumbal dopamine to environmental and pharmacological challenges. Eur. J. Pharmacol. 585, 228–244. doi: 10.1016/j.ejphar.2008.02.084
Volkow, N. D., Fowler, J. S., Wang, G. J., Baler, R., and Telang, F. (2009). Imaging dopamine’s role in drug abuse and addiction. Neuropharmacology 56(Suppl. 1), 3–8. doi: 10.1016/j.neuropharm.2008.05.022
Volkow, N. D., Wang, G.-J., Telang, F., Fowler, J. S., Logan, J., Childress, A.-R., et al. (2006). Cocaine cues and dopamine in dorsal striatum: mechanism of craving in cocaine addiction. J. Neurosci. 26, 6583–6588. doi: 10.1523/JNEUROSCI.1544-06.2006
Volkow, N. D., Wise, R. A., and Baler, R. (2017). The dopamine motive system: implications for drug and food addiction. Nat. Rev. Neurosci. 18, 741–752. doi: 10.1038/nrn.2017.130
Wang, Y.-C., Ho, U.-C., Ko, M.-C., Liao, C.-C., and Lee, L.-J. (2012). Differential neuronal changes in medial prefrontal cortex, basolateral amygdala and nucleus accumbens after postweaning social isolation. Brain Struct. Funct. 217, 337–351. doi: 10.1007/s00429-011-0355-4
Willuhn, I., Burgeno, L. M., Everitt, B. J., and Phillips, P. E. M. (2012). Hierarchical recruitment of phasic dopamine signaling in the striatum during the progression of cocaine use. Proc. Natl. Acad. Sci. U.S.A. 109, 20703–20708. doi: 10.1073/pnas.1213460109
Willuhn, I., Burgeno, L. M., Groblewski, P. A., and Phillips, P. E. M. (2014). Excessive cocaine use results from decreased phasic dopamine signaling in the striatum. Nat. Neurosci. 17, 704–709. doi: 10.1038/nn.3694
Winstanley, C. A., and Clark, L. (2016). Translational models of gambling-related decision-making. Curr. Top. Behav. Neurosci. 28, 93–120. doi: 10.1007/7854_2015_5014
Wise, R. A. (2005). Forebrain substrates of reward and motivation. J. Comp. Neurol. 493, 115–121. doi: 10.1002/cne.20689
Wise, R. A. (2009). Roles for nigrostriatal—Not just mesocorticolimbic—Dopamine in reward and addiction. Trends Neurosci. 32, 517–524. doi: 10.1016/j.tins.2009.06.004
Wise, R. A., and Bozarth, M. A. (1987). A psychomotor stimulant theory of addiction. Psychol. Rev. 94, 469–492.
Wise, R. A., and Robble, M. A. (2020). Dopamine and addiction. Annu. Rev. Psychol. 71, 79–106. doi: 10.1146/annurev-psych-010418-103337
Witten, I. B., Steinberg, E. E., Lee, S. Y., Davidson, T. J., Zalocusky, K. A., Brodsky, M., et al. (2011). Recombinase-driver rat lines: tools, techniques, and optogenetic application to dopamine-mediated reinforcement. Neuron 72, 721–733. doi: 10.1016/j.neuron.2011.10.028
Wittmann, B. C., Tan, G. C., Lisman, J. E., Dolan, R. J., and Düzel, E. (2013). DAT genotype modulates striatal processing and long-term memory for items associated with reward and punishment. Neuropsychologia 51, 2184–2193. doi: 10.1016/j.neuropsychologia.2013.07.018
Wolf, M. E. (2016). Synaptic mechanisms underlying persistent cocaine craving. Nat. Rev. Neurosci. 17, 351–365. doi: 10.1038/nrn.2016.39
Yin, H. H., Ostlund, S. B., Knowlton, B. J., and Balleine, B. W. (2005). The role of the dorsomedial striatum in instrumental conditioning. Eur. J. Neurosci. 22, 513–523. doi: 10.1111/j.1460-9568.2005.04218.x
Yorgason, J. T., Calipari, E. S., Ferris, M. J., Karkhanis, A. N., Fordahl, S. C., Weiner, J. L., et al. (2016). Social isolation rearing increases dopamine uptake and psychostimulant potency in the striatum. Neuropharmacology 101, 471–479. doi: 10.1016/j.neuropharm.2015.10.025
Young, K. A., Liu, Y., Gobrogge, K. L., Wang, H., and Wang, Z. (2014). Oxytocin reverses amphetamine-induced deficits in social bonding: Evidence for an interaction with nucleus accumbens dopamine. J. Neurosci. 34, 8499–8506. doi: 10.1523/JNEUROSCI.4275-13.2014
Yun, I. A., Wakabayashi, K. T., Fields, H. L., and Nicola, S. M. (2004). The ventral tegmental area is required for the behavioral and nucleus accumbens neuronal firing responses to incentive cues. J. Neurosci. 24, 2923–2933. doi: 10.1523/JNEUROSCI.5282-03.2004
Zapata, A., Minney, V. L., and Shippenberg, T. S. (2010). Shift from goal-directed to habitual cocaine seeking after prolonged experience in rats. J. Neurosci. 30, 15457–15463. doi: 10.1523/JNEUROSCI.4072-10.2010
Zhou, Z., Liu, X., Chen, S., Zhang, Z., Liu, Y., Montardy, Q., et al. (2019). A VTA GABAergic neural circuit mediates visually evoked innate defensive responses. Neuron 103, 473–488.e6. doi: 10.1016/j.neuron.2019.05.027
Zimmer, B. A., Oleson, E. B., and Roberts, D. C. (2012). The motivation to self-administer is increased after a history of spiking brain levels of cocaine. Neuropsychopharmacology 37, 1901–1910. doi: 10.1038/npp.2012.37
Zlebnik, N. E., and Carroll, M. E. (2015). Prevention of the incubation of cocaine seeking by aerobic exercise in female rats. Psychopharmacology 232, 3507–3513. doi: 10.1007/s00213-015-3999-6
Keywords: dopamine, striatum, addiction, substance use disorder, animal model, nigrostriatal, mesostriatal
Citation: Poisson CL, Engel L and Saunders BT (2021) Dopamine Circuit Mechanisms of Addiction-Like Behaviors. Front. Neural Circuits 15:752420. doi: 10.3389/fncir.2021.752420
Received: 03 August 2021; Accepted: 08 October 2021;
Published: 09 November 2021.
Edited by:
Talia Newcombe Lerner, Northwestern University, United StatesReviewed by:
Marco Venniro, University of Maryland, Baltimore, United StatesBarbara Juarez, University of Washington, United States
Donna J. Calu, University of Maryland, Baltimore, United States
Susan Ferguson, University of Washington, United States
Copyright © 2021 Poisson, Engel and Saunders. This is an open-access article distributed under the terms of the Creative Commons Attribution License (CC BY). The use, distribution or reproduction in other forums is permitted, provided the original author(s) and the copyright owner(s) are credited and that the original publication in this journal is cited, in accordance with accepted academic practice. No use, distribution or reproduction is permitted which does not comply with these terms.
*Correspondence: Benjamin T. Saunders, YnRzQHVtbi5lZHU=