- 1Future Cities Laboratory, Singapore ETH-Centre, Singapore, Singapore
- 2Applied Computing and Mechanics Laboratory (IMAC), School of Architecture, Civil and Environmental Engineering (ENAC), Civil Engineering Institute, Swiss Federal Institute of Technology, Lausanne, Switzerland
- 3Department of Architecture, Karlsruhe Institute of Technology, Karlsruhe, Germany
Bamboo fibers with high mechanical properties can be a sustainable alternative to synthetic fibers for application in fiber reinforced polymer composites. The first aim of this study is to evaluate the dependence of mechanical properties of Dendrocalamus asper, known as bamboo Petung from Indonesia, on physical properties of the culm, including culm diameter, wall thickness, height, moisture content and specific density. Correlations between mechanical properties including tensile strength, modulus of rupture and modulus of elasticity in flexure and tension and culm physical properties have been studied. The results demonstrate that specific density is directly correlated with all these mechanical properties of bamboo while the moisture content values are correlated only with value of modules of rupture. Although wall thicknesses value of the culm are correlated with all of the mechanical properties studied, the culm diameter was only correlated with modulus of rupture and modulus of elasticity in flexure. Therefore, measurements of the culm geometry and specific density of raw bamboo have the potential for rapid, non-destructive evaluations of the quality of the bamboo, particularly in nurseries and forests where there is limited access to testing facilities. The second aim of this study is to evaluate whether such tests allow for an evaluation of the mechanical potential of the bamboo for production of high performance bamboo fiber reinforced polymer composites. Use of these formulas is illustrated through a case study of bamboo composite reinforcement for structural concrete. Pull-out tests and beam testing using this composite successfully validate the usefulness of this strategy for sustainable construction.
Introduction
The demand for construction materials is increasing worldwide as populations and their aspirations are growing. Developing countries in Africa and Asia are struggling to meet this demand due to missing infrastructures and industries. To satisfy the demands for housing and infrastructure for the increasing population in new cities, countries, such as Indonesia, Thailand, Vietnam, and Myanmar have to rely on mostly imported building materials, for example sand, cement, steel and also timber for construction. However, in the long-term, this could be problematic since these construction materials are either finite for local supply (sand or timber) or they are only available through import (copper, iron ore, steel, or other metals). Without alternatives, many nations may no longer be able to satisfy the rising demands for construction materials. Fortunately, proposals are emerging to replace timber and steel with renewable, low-cost and sustainable forms of construction materials that are found locally in developing regions.
Fiber reinforced composite materials have enabled much industrial innovation. Currently composites reinforced by glass fibers and carbon fibers are being used extensively for many structural applications. However, there are economic and environmental challenges. Most of the synthetic fibers are difficult to recycle and are produced from chemicals made from refined petroleum. Furthermore, their fabrication procedures are energy-intensive. Composite materials based on synthetic inorganic fibers are thus expensive and environmentally unsuitable. A promising alternative is to employ natural fibers instead of the synthetic inorganic fibers. Advantages, in comparison with synthetic fibers, are their abundance, renewability, biodegradability and lower cost. Among various natural fibers, bamboo has shown to be a sustainable yet affordable alternative.
Bamboo is one of the locally available natural materials that has gained attention in recent years for fabrication of new category of sustainable bamboo-fiber-reinforced composite materials. Bamboo is a fast growing, inexpensive and available natural resource in most developing countries and it has outstanding material qualities. The cultivation and industrial processing of bamboo offers a huge potential for a new generation of building materials fabricated through embedding natural bamboo fibers into a resin matrix for applications in architecture and construction (Faruk et al., 2014; Hebel et al., 2014; Yu et al., 2014; Javadian et al., 2016; Javadian, 2017; Rahman et al., 2017; Archila et al., 2018).
Bamboo is a natural hierarchical cellular material which has good mechanical properties, including tensile and flexural strength, along its fiber direction. Since bamboo is a functionally graded natural composite the interfaces between its different ingredients including the fibers, parenchyma cells, and lignin matrix can have significant impact on its mechanical properties (Wegst and Ashby, 2004). The hierarchical microstructure of bamboo arises from the vascular bundles in the parenchyma matrix being surrounded by supporting cellulose fibers. These fibers provide the main mechanical properties of bamboo. Furthermore, the cellulose fibers act as reinforcement to strengthen the lignin matrix, similarly to fiber reinforced polymer matrix composites. This structure creates the crystalline and amorphous regions within the microstructure of bamboo where linear chains of glucose with hydrogen bonds form the crystalline regions while irregular hydrogen bonds create amorphous regions (Gibson, 2012; Youssefian and Rahbar, 2015).
Bamboo has higher mechanical properties along its fiber direction than across it. The unique microstructural properties of natural bamboo with respect to its mechanical properties make it a suitable renewable material for composites in high performance applications.
Usually, the density of bamboo is higher on the outer surface and decreases toward the inner layers of the wall cross section (Lakkad and Patel, 1981; Murphy and Alvin, 1992; Ray et al., 2004; Zou et al., 2009; Wahab et al., 2010; Kaur et al., 2016). Therefore, the outer layers of bamboo culms are supposed to have better mechanical properties (Liese, 1985; Lo et al., 2008; Yu et al., 2008). However, to date, no comprehensive and systematic studies of properties of bamboo in terms of density and culm geometry—including wall thickness, culm diameter and culm height—have been found. The identification of sections with higher fiber densities and therefore possible superior quality in terms of physical and mechanical properties has the potential to have a significant impact on the performance of fabricated composite materials.
Previous studies of bamboo Guadua angustifolia from Columbia have shown that the top portion of the culm has the highest strength and modulus of elasticity compared with lower portions, since top portion of the culm has higher density (Correal et al., 2010). However, this study did not include top culm sections and thus, correlations of the culm position with mechanical and physical properties have not been studied.
Similar studies were carried out in Bangladesh on mechanical properties, moisture content and density of four indigenous bamboo species in relationship to the respective culm height (Kamruzzaman et al., 2008). In this study Bambusa balcooa, Bambusa tulda, Bambusa salarkhanii and Melocanna baccifera were tested to determine the modulus of elasticity and the modulus of rupture. However, no correlation between the culm height and the mechanical properties has been found.
Bamboo Gigantochloa levis (buluh beting) of Malaysia was studied for the variation of density, modulus of rupture and modulus of elasticity with height and age of the culm (Nordahlia et al., 2012). The study showed that although the modulus of rupture did not change significantly with the culm height, the modulus of elasticity increased with increases in culm height. However, no information has been provided on values for tensile strength and modulus of elasticity in bending along with their effects on wall thickness or culm height.
Wakchaure and Kute have studied moisture content, specific gravity, water absorption, dimensional changes, tensile and compressive strength of bamboo Dendrocalamus strictus from India at several heights (Wakchaure and Kute, 2012). They found no significant difference between bottom and middle portions for tensile, compressive strength and modulus of elasticity. Moisture content decreased from bottom to top sections while specific density increased. Unfortunately, the effect of culm wall thickness and culm diameter on the physical and mechanical properties has not been studied.
Moisture content (MC) is an important property of raw bamboo, especially in building and construction applications and for composite fabrication. MC may adversely affect the bonding strength of bamboo fibers in composite products and bamboo laminates as has been shown in studies carried out by Okubo et al. (2004), Chen et al. (2009), Kushwaha and Kumar (2009). Therefore, the MC is expected to have a major impact on the performance and service life of new bamboo composite materials.
In addition to MC influence on mechanical properties of raw bamboo, such as tensile strength and flexural strength, it also affects geometrical properties of raw bamboo, such as dimensional stability. Although several studies have included the effect of water absorption on dimensional stability of raw bamboo and bamboo composite specimens, they did not examine the correlation between water absorption of green bamboo and its mechanical properties (Rowel and Norimoto, 1988; Nugroho and Ando, 2000, 2001). Rapid moisture changes can result in severe shrinkage or expansion of bamboo layers, which may, especially in laminates or composites, result in layer-bond failure (Lee et al., 1996; Zaidon et al., 2004; Malanit et al., 2011). Therefore, it is important to determine the MC of various sections of raw bamboo and classify the MC according to the location within the culm length before processing the raw bamboo fibers into composites or laminates.
The specific density (SD) is the oven-dry weight of a given volume of raw bamboo divided by the weight of an equal volume of water. SD values are closely related to MC values. A standard method for measuring SD and MC is needed to ensure that results are comparable with other studies. The SD of raw bamboo is a potential indicator of properties of bamboo-based products, such as laminates and bamboo composite materials and therefore, it is important to measure both SD and MC values and relate them to the mechanical properties of raw bamboo.
As the fiber density changes over the wall thickness, SD values will differ from the outer to inner section of the wall cross section. Therefore, for any application of raw bamboo, it is important to know which part of the wall cross section is processed and what are the corresponding MC and SD of that part. Measuring MC and SD values to correlate them with values for wall thickness and mechanical properties provides an affordable and valuable method for selecting the best bamboo sections for the production of bamboo-based products having pre-defined qualities.
Research into the mechanical properties of hierarchical structures of raw bamboo should lead to better control of fabrication and quality of the novel bamboo-based composites. Since bamboo is a grass, which reaches its full height of 20–30 m within an extremely short period of only a few months, the mechanical properties along culm lengths may vary substantially (Liese, 1998).
Similar variation of properties may be encountered in all three principle directions e.g., longitudinal, radial and tangential (Liese, 1987). Studies have been carried out on various species of bamboo to investigate differences in mechanical properties (Limaye, 1952; Liese and Jackson, 1985; Rao et al., 1988; Hidalgo-Lopez, 2003; Janssen, 2012). However, neither the effects of wall thickness nor culm geometry on the mechanical properties was investigated or correlated to bamboo's natural hierarchical structure.
Some studies investigated the mechanical properties of laminates and composites made from Dendrocalamus asper (Malanit et al., 2009, 2011; Febrianto et al., 2012). The results show that composites and boards made from Dendrocalamus asper have high mechanical properties compared with commercial products made from wood. However, these studies also did not take into account variations of mechanical properties of various sections of Dendrocalamus asper and various culm diameters.
In this paper, a comprehensive and systematic study of Moisture Content (MC), Specific Density (SD), Tensile Strength (TS) along the fiber direction, modulus of Elasticity in tension (Et), flexural strength or Modulus of Rupture (MOR), and modulus of Elasticity in flexure (Ef) is presented. These properties are then correlated with the respective culm geometry of bamboo to have a better understanding of its hierarchical structure which then can be considered for synthesis of novel bamboo-fiber-reinforced composite materials fabricated from bamboo Dendrocalamus asper. Finally, the use of these relationships is examined through a case study involving the development of a bamboo composite for use in reinforced concrete. Mechanical test results are then used to validate this new approach.
Materials and Methods
Bamboo Species
Dendrocalamus asper or Petung Putih bamboo was selected from a bamboo forest on the Java island of Indonesia. This bamboo is widely available in Java and mostly being used for construction of small housing in local villages. The Dendrocalamus asper from Java had an average culm length of 15 m. The outer diameter of the selected culms were between 80 and 150 mm. The selected culms had wall thicknesses between 6 and 20 mm. The initial MC of the culms ranged between 12 and 15%. The culms were cut into three sections and labeled as top, middle and bottom. Each section was 5 m in length. Samples for this study were obtained only from the middle and bottom sections as the top portion of the culms were not available for this study.
Sample Preparation
Fifteen culms of 15 m length were chosen for this study. The bottom and middle sections were eventually divided into five subsections with a length of 1 m. The 1 m section was then split lengthwise and samples of varying thicknesses were cut randomly for the physical and mechanical tests. The subsections have been classified into seven groups according to culm diameter and wall thickness as shown in Table 1.
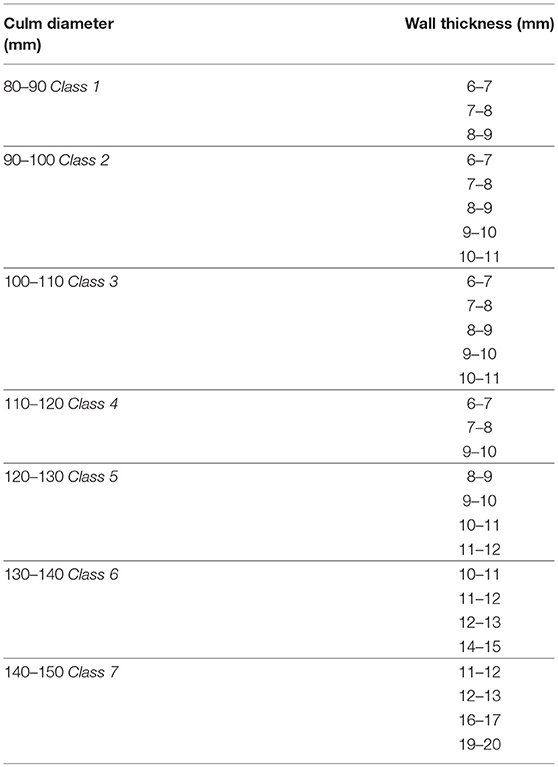
Table 1. Classification of the samples used in this study according to culm diameter and wall thickness.
Sections with bigger diameters typically have larger wall thicknesses as compared with sections of smaller diameter. For class 6 and 7, the samples with larger wall thicknesses of up to 20 mm were used for testing to evaluate the effect of thicker than 15 mm wall section on culm properties.
Moisture Content
The MC was measured for samples taken from the 1 m subsections. From each subsection, 10 samples were prepared. The ASTM D4442-07 standard test method for direct moisture content determination of wood and wood-based materials was followed (ASTM International, 2015). The sample size was (10) mm × (10) mm × (thickness of the section). Once the samples were cut from the culms, they were weighed using a Shimadzu BL320H balance with an accuracy of 0.001 g. The samples were then dried using a convection oven that could maintain 103°C for 24 h. MC was calculated from Equation (1):
where, A is the original weight in gram and B is the dried weight in grams.
Specific Density
Samples for SD measurement were prepared according to ASTM D2395-14 standard test method for density and specific gravity of wood and wood-based materials (ASTM International, 2014a). From each subsection, 10 samples were prepared randomly. For each sample, the width, the length and the thickness were determined for the volume (V) calculation. The initial mass (m) of each sample was measured with the Shimadzu BL320H balance with an accuracy of 0.001 g. The density (ρ) and SD were calculated using the following formulae:
where K = 1,000 mm3/g, (m) is in grams and (V) is in mm3.
Tensile Strength Along the Fiber
The tensile strength of the samples was measured with reference to the ASTM D143-09 standard test method for small clear specimens of timber using a Shimadzu AG-IC 100 kN tensile-testing machine (ASTM International, 2014b). Samples were cut from the 1 m sections of bamboo culms and were chosen from various radial locations along the sections and then prepared into dog-bone shapes. The average width and length of the sample grips was 25 and 50 mm, respectively. The average gauge length was 130 mm.
The samples prepared from thick culms were first split into sections with the same thickness along the length. Subsequently each section was papered according to ASTM D143-09 into dog-bone shapes and tested.
Average values from the tensile tests of the two sections were then used for analysis and evaluation. Five samples were taken from the internodes of 1 m subsections. The loading rate was set to 1 mm/min. All tests were carried out at room temperature and 65% relative humidity. The tensile strength (σt) was calculated by measuring the ultimate load at failure of the test (Fult) and then dividing it by the cross section of the sample across the gauge length (A). The following formula was used to determine the tensile strength.
Modulus of Elasticity in Tension (Et)
The modulus of elasticity in tension was measured using a Shimadzu AG-IC 100 kN machine according to the ASTM D143-09 standard test method for small clear specimens of timber (ASTM International, 2014b). Dog-bone shape tensile strength samples were used for this test. The gauge length was adjusted for modulus of elasticity test to 80 mm and the grip width and length remain unchanged. An Epsilon axial extensometer with a gauge length of 80 mm was used to measure the sample deformation during the test. The loading rate was set to 1 mm/min. Load-deformation curves were obtained from each test to measure the modulus of elasticity in tension. The modulus of elasticity has been calculated from the slope of the initial linear portion of the stress-strain curve derived from the load-deformation curves.
Modulus of Rupture (MOR)
MOR or flexural strength was measured according to ASTM D3043-00(2011) standard test method for structural panels in flexure (ASTM International, 2011). A two-point flexural test was carried out in this study. The advantage of a two-point flexure test over a center-point flexure test is that a larger area of the sample is subjected to peak stress—unlike the center-point flexure test, where the peak stress is applied to an isolated location. Therefore, the probability that any crack or flaw exists between two loading supports will be higher and the results will be more reliable in a two-point flexure test. Five Samples without nodes were prepared from 1 m subsections of each culm. The loading rate was calculated according to ASTM D3043 with respect to sample thickness and width.
Modulus of Elasticity in Flexure (Ef)
The modulus of elasticity in flexure was measured by obtaining the load-deformation curve in the flexural strength test. An Epsilon extensometer with a travel gauge of 25 mm was used to measure the mid-span deflection of the samples during the flexural strength test. The measurement and calculation of the modulus of elasticity were carried out according to ASTM D3043-00(2011) at room temperature and 65% relative humidity. Multiple comparisons between several wall thicknesses and culm diameters were conducted in this study.
Statistical Analysis
Statistical analysis was performed on the data obtained in this study by using SPSS version 22 (SPSS Inc., Chicago, IL). Pearson's correlation coefficients (r) were calculated to find the relationship between the culm geometry, SD, MC and the mechanical properties of bamboo. Three levels of correlation were defined (i.e., strong, r > 0.5; moderately strong, 0.3 < r < 0.5; and weak, r < 0.3). To further examine the relationships between the culm geometry and mechanical properties of hierarchical structure of natural bamboo, stepwise multiple linear regressions were performed. The model performance was assessed with an adjusted r2 value, which represents the percentage of the variations that are described by independent variables. The r2 in general is a statistical parameter to demonstrate that the results of the study are close to the model obtained through multiple regression analysis. The values of r2 are normally between 0 and 1; however, if the values of r2 are closer to 1, it indicates that the model obtained can represent more of the data points.
Results and Discussion
Moisture Content (MC)
The moisture content has been measured at two relative humidity conditions: one at 20°C with 65% relative humidity and one at 45°C with 80% relative humidity. The results for the samples from various categories of bamboo Dendrocalamus asper are shown in Table 2.
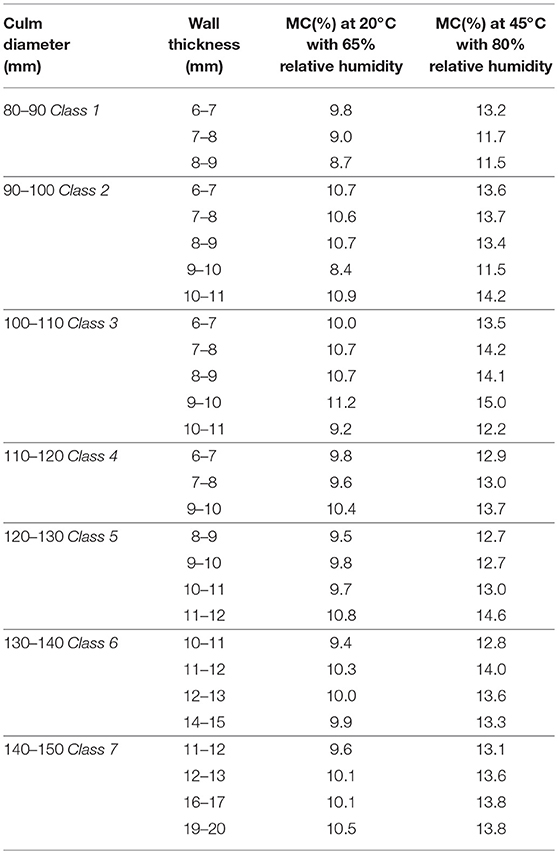
Table 2. Moisture content of bamboo Petung at two relative humidity conditions for different classes.
At a relative humidity of 80%, the MC increases for all classes equally. This condition has been achieved after 6 days for wall thicknesses of more than 13 mm and after only 3 days for wall thickness of < 13 mm. The increase in MC for all classes are in the range of 25–35%. The change in MC for class 4–7 is not significant at 80% relative humidity condition. Figure 1 shows the comparison of average MC values together with the error bars for each class.
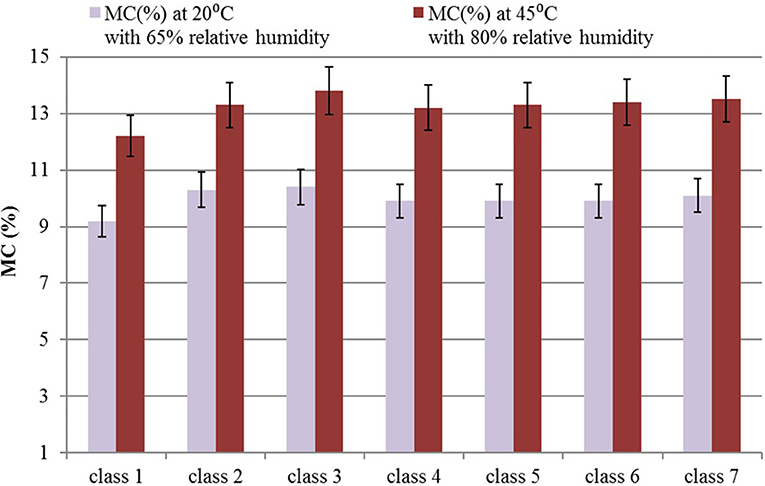
Figure 1. Average MC comparison for all classes of bamboo petung under two relative humidity conditions with error bars at two standard deviations.
Although, the average MC under both relative humidity conditions for class 4–7 does not vary considerably, for class 1–3 the MC increases with increasing culm diameter. Culms with 100 mm or less in diameter and with thinner wall sections have lower percentage of lignin matrix and higher presence of cellulose fibers compared with culms larger than 100 mm in diameter, as shown in other studies (Alvin and Murphy, 1988; Murphy and Alvin, 1992; Mohmod et al., 1993).
Since bamboo has a hierarchical structure, its lignin matrix establishes hydrogen bonds with water, thus large culms are more stable when exposed to relative humidity variations compared with small culms with thinner wall sections. The thinner wall section in culms with smaller diameter has a higher fiber density and therefore, a lower percentage of lignin matrix when compared with large culms (Zou et al., 2009). As a result, the relative humidity variation will have a greater impact on MC of small culms with thin wall sections compared with large culms with thick wall sections. Despite the trend observed in MC variation with culm diameter and culm wall thickness, the differences in MC values of various classes of bamboo Petung for each relative humidity condition are not substantial.
For processing the raw bamboo culms into sections suitable for bamboo-based composite fabrication, it was essential to carefully analyze the hierarchical structure of natural bamboo with respect to the change in MC with various culm diameters and wall thicknesses. The average MC of the raw bamboo culms selected for processing should fall below 10% to reduce the effect of excessive delamination or long-term environmental impacts through the degradation of the final composite product. By measuring the moisture content of chosen bamboo culms for composite production, a preliminary estimation of the required time to achieve a certain MC percentage suitable for processing of the raw bamboo and fabrication of composite became possible.
Specific Density (SD)
The results of SD measurement are presented in Table 3 for various classes of bamboo culms.
One-way ANOVA (Analysis of Variance) test shows that there is no significant difference between SD values of wall thicknesses within class 1–3. SD for class 5–7 decreases with increasing culm diameter. The common wall thickness category between class 5, 6, and 7 is 11–12 mm. The SD for this category of wall thickness and for class 5, 6, and 7 was 0.741, 0.738, and 0.735, respectively. With increasing culm diameter for culms with diameter of 120–150 mm, the SD decreases.
The reduction in SD of larger culms is attributed to fiber density characteristics. Larger culm diameters with thicker walls are usually found at the bottom of the culm where the fiber density is lower. Generally, bamboo culms have higher fiber density at top parts where the fibers are closely packed as has been shown by other studies on microstructure of bamboo culm of different species (Alvin and Murphy, 1988; Ray et al., 2004). As a result, the SD will be lower in the bottom parts where the culm diameter and wall thickness are much greater as compared to the middle and top parts.
Tensile Strength Along the Fiber
The results of the tensile strength tests of the bamboo Petung samples along the fiber direction are provided in Table 4. The maximum tensile strength of class 1 samples is 295 MPa for a wall thickness of 7–8 mm. In the same class, wall thickness categories of 6–7 mm and 8–9 mm have similar tensile strength. In class 2, the samples with a wall thickness of 7–8 mm have the highest tensile strength of 298 MPa. Other categories of wall thickness have similar tensile properties and there is no significant difference between the values.
As Figure 2 displays, there is no significant difference between average tensile strength of class 1–3 samples. However, the average tensile strength for class 4–7 decreases with increasing culm diameter. A relationship between the culm diameter, specific density and tensile strength is revealed when comparing the results from SD and tensile strength measurement. For class 1–3, there is no significant change in SD and tensile strength while increasing the culm diameter. For class 4–7, by increasing the culm diameter, both tensile strength and SD decreases.
For culm diameters above 110 mm the tensile strength is influenced by the fiber density of bamboo. Larger culms are likely to have lower cellulose fibers and higher lignin content. Therefore, the tensile strength of the raw bamboo, which mainly comes from the tensile capacity of the cellulose fibers, is largely reduced. This is in line with the trend observed for the SD of bamboo Petung. As mentioned earlier, SD is principally influenced by the fiber density, therefore declining the fiber density, results in lower SD as shown also in previous study (Ray et al., 2004). The correlation between SD, tensile strength and fiber density is important when selecting the bamboo culms for composite processing. Being able to distinguish culms with diverse tensile strengths by measuring only their SD is a valuable method for choosing the most appropriate culms for bamboo composite materials.
Modulus of Elasticity in Tension (Et)
The modulus of elasticity in tension of bamboo Petung was measured for different classes of bamboo Petung with varying culm diameters and wall thicknesses according to ASTM D143-14. The results are summarized in Table 5.
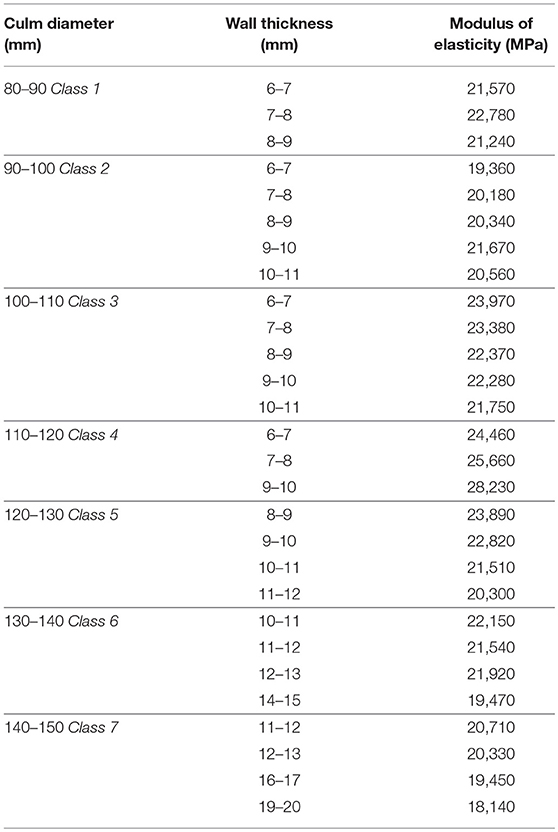
Table 5. Modulus of elasticity in tension of bamboo petung for different culm diamters and wall thicknesses.
The modulus of elasticity of bamboo is a measure of the stiffness of the bamboo matrix and its resistance to elastic deformation. The highest modulus of elasticity is observed for class 4 samples with 9 mm to 10 mm wall thickness with 28,230 MPa while the lowest modulus of elasticity is found for class 7 samples with wall thicknesses of 19 mm to 20 mm at 18,140 MPa.
The one-way ANOVA test showed no significant difference between modulus of elasticity of various wall thicknesses within class 1 samples. The modulus of elasticity of the samples in class 4 has shown an increase compared with the samples from class 1, 2 and 3. In class 4, the modulus of elasticity increases with increasing wall thickness.
Within the seven classes of bamboo Petung, class 4 exhibits the highest average modulus of elasticity. In classes 5 to 7, the modulus of elasticity reduces with increasing wall thickness. Similar trends are observed for class 6 and 7 samples. This is in line with the trend observed for tensile strength of the samples from class 5 to class 7 where an increasing wall thickness lowered the tensile strength. Comparing Table 5 to Table 4 reveals that for class 5, 6, and 7, both tensile strength and modulus of elasticity decrease with increasing wall thickness.
As mentioned earlier, the high tensile capacity of bamboo is largely influenced by the tensile capacity of the cellulose fibers within the natural hierarchical structure of bamboo. This is also true for the modulus of elasticity of the bamboo. The modulus of elasticity may be estimated through taking the sum of the modulus of the cellulose fibers and the modulus of the lignin matrix weighted by their volumetric fractions. Culms with diameters of <110 mm, have nearly similar volumetric ratios of cellulose fibers and lignin, therefore they have shown similar modulus of elasticity in all categories of wall thicknesses.
By increasing the culm diameter, the wall thickness also increases. With increasing wall thickness in larger culms, the volumetric ratio of cellulose fibers to lignin is also reduced as has been observed in other studies (Alvin and Murphy, 1988; Murphy and Alvin, 1992). As a result, a higher percentage of lignin as compared with the cellulose fibers is expected in thicker wall sections. This leads to lower modulus of elasticity of larger bamboo culms compared to smaller culms in which the volumetric ratio of cellulose fibers to lignin is higher.
Modulus of Rupture (MOR)
Table 6 summarizes the results of the MOR tests for varying wall thicknesses and culm diameters of bamboo Petung. Class 1 samples have the highest MOR with 209 MPa while class 7 samples have the lowest MOR with 121 MPa. For class 1 samples, increasing the wall thickness from 6 to 9 mm results in the reduction of the MOR from 209 to 198 MPa. For class 2 and 3 samples, no significant relationship is found between the wall thickness and the MOR. In class 4 samples, increasing the wall thickness from 6 to 10 mm, reduces the MOR from 166 to 155 MPa, which corresponds to a reduction of 6.7%. For class 5, the MOR for wall thickness of 10–11 mm is the lowest with 149 MPa. The MOR in class 5 is in the same range as for wall thickness of 9–12 mm with a standard deviation of 5%. For class 6 samples, similar trend is observed as compared with the classes 1, 4, and 5 of bamboo Petung.
The standard deviation of these samples in class 6 was < 4%. The MOR for class 7 samples is reduced with an increase in wall thickness. Wall thicknesses between 19 and 20 mm had the lowest MOR of 121 MPa. Figure 3 shows the average MOR for seven classes of bamboo Petung.
Culms with larger diameters have thicker walls particularly at bottom sections. The thicker wall thickness leads to a higher percentage of lignin and lower proportion of cellulose fibers. As observed earlier regarding the tensile capacity and its relationship with the fiber density, similar conclusions can be made concerning MOR. The cellulose fibers are densely packed at the top sections of the hierarchical structure of bamboo culms where a smaller diameter prevails. The MOR increases with decreasing culm diameter. Except for class 2 samples, the MOR decreases with increasing wall thickness within a class. This underlines the importance of the fiber density on the mechanical properties of raw bamboo. Cellulose fibers contribute to high mechanical properties of natural bamboo. The cellulose fiber density is higher at the outer layer of the wall sections and at the top portions of the culms. Therefore, the MOR increases with increasing fiber content and decreasing lignin content at the surroundings of the fibers.
Modulus of Elasticity in Flexure (Ef)
The modulus of elasticity in flexure is taken from the load deflection curve that has been obtained with the help of an extensometer. The effect of wall thickness and culm diameter on the modulus of elasticity in flexure is studied for all seven classes of bamboo Petung.
Table 7 presents the result of this test for the range of wall thicknesses and culm diameters that were tested according to ASTM D3043-00(2011).
The highest modulus of elasticity of 14,279 MPa was observed for class 2 samples with a wall thickness in the range of 9–10 mm. The lowest modulus of elasticity of 9,375 MPa was observed in samples of class 7 with wall thicknesses between 19 and 20 mm. This finding is comparable to the results of MOR tests where class 7 samples show the lowest MOR of all samples. When the results of various wall thicknesses were compared, random variations in modulus of elasticity with increasing the wall thickness were observed. However, as displayed in Table 7, bamboo Petung shows a decrease in the average modulus of elasticity in flexure with increasing culm diameter from 80 to 150 mm. Samples with culm diameters of <120 mm show less significant changes in the modulus of elasticity with varying the culm diameters. Nevertheless, for samples with culm diameters of 120 mm and larger, the modulus of elasticity drops with increasing the culm diameter.
The effect of the culm diameter on the modulus of elasticity in flexure is similar to MOR. With increasing culm diameter, the average modulus of elasticity decreases. This observation can be attributed to the culm hierarchical microstructure. With increasing culm diameter, mainly at the bottom and middle sections of bamboo, the fiber density decreases due to the higher lignin content as compared with the fiber content.
As described earlier, the top sections of a culm exhibit higher fiber densities as compared with the bottom sections. Such high fiber density is responsible for the strong mechanical features of the bamboo culm—especially the modulus of elasticity, MOR and the tensile strength.
Within one class of bamboo, the change in modulus of elasticity with varying wall thicknesses is not linear for all the samples. This is due to the spatially varying microstructure of the bamboo culm walls. Samples tested in this study have been randomly collected at different cross sections and varying height locations. Therefore, the variation in modulus of elasticity with wall thickness was expected from culm to culm.
Comparing the mechanical properties of bamboo Petung with available local timber species that are commonly used in structural applications in Indonesia, demonstrates the superior properties that bamboo Petung offers as compared with timber. Table 8 presents the range of specific density, tensile strength along the fiber, modulus of elasticity in tension and MOR of timber species used commonly in structural applications as well as composite products (Green et al., 1999). Timber species commonly used in Indonesia are Balau, Sumatran Pine and Indonesian Rosewood.

Table 8. Comparison of properties between common timber species in Indonesia and bamboo Petung (Green et al., 1999).
The mean tensile strength of bamboo Petung is higher than Balau, Sumatran Pine, and Indonesian Rosewood. In terms of modulus of elasticity, bamboo Petung is stiffer than all timber species specified in Table 8 except for the top range of Balau, which is close to the modulus of elasticity of the bamboo Petung. The Indonesian Rosewood has a low modulus of elasticity compared with bamboo Petung and other common timber species in Indonesia. Balau has the highest range of MOR among the common timber species. However, bamboo Petung has higher values of MOR compared with all timber species. Sections of bamboo Petung with the lowest mechanical properties are still superior to some of the most common structural grade timber species found in Indonesia as shown in Table 8.
Correlation Studies and Statistical Modeling of Physical and Mechanical Properties
To measure the strength of any possible relationship between mechanical properties, culm diameter, wall thickness, specific density and moisture content, Pearson's correlation coefficients (r) are calculated. Table 9 summarizes the correlation coefficients for only statistically significant correlations with a p-value of < 0.05 by a two-tailed t-test between mechanical and physical properties measured in this study. As it is shown in Table 9, wall thickness of the culms and specific density (SD) have moderate to strong negative and positive correlations with all the mechanical properties, respectively. Culm diameter shows strong negative correlation with all the mechanical properties except modulus of elasticity in tension (Et). This finding is in accordance with the results of the modulus of elasticity tests presented in Table 5. Moisture Content (MC) has only moderately negative correlation with Modulus of Rupture (MOR) in this study. Therefore, it is not viable to estimate mechanical properties including tensile strength and modulus of elasticity by only measuring the MC of the bamboo culm sections. The highest Pearson correlation coefficient is observed between tensile strength and wall thickness (r = −0.742) of the bamboo Dendrocalamus asper. Therefore, larger culm diameters would show lower tensile strength.
Among all the mechanical properties measured in this study, only Modulus of Rupture (MOR) showed strong correlation with the physical properties under study [culm geometry, Moisture Content (MC) and Specific Density (SD)]. The Pearson correlation coefficients show that by increasing either of the culm diameter, wall thickness and MC, MOR reduces and increasing the Specific Density (SD) will have a positive impact on MOR. As it is expected, Specific Density (SD) has positive correlation with all the mechanical properties. This is in agreement with previous studies carried out on other bamboo species regarding the effect of density on mechanical properties (Lakkad and Patel, 1981; Lo et al., 2004). SD represents the fiber density of the culm cross sections. Therefore, the higher the fiber density of bamboo cross sections, the larger is the SD and as a result, those sections show better mechanical properties. Furthermore, mathematical models and equations were suggested for estimating the mechanical properties of bamboo Petung by only measuring the culm diameter and wall thickness. Table 10 displays linear-model parameter values created with the data obtained in this study. In Table 14, all mechanical properties have units of MPa while D and t are in mm and MC is in percentage. The empirical relations between MOR, Ef, Et, CS, TS, and culm physical properties are developed and summarized here. These equations should be considered to provide only preliminary estimation of the mechanical properties for bamboo Dendrocalamus asper. For other species of bamboo and bamboo from other regions around the world, the model coefficients and constants could differ.
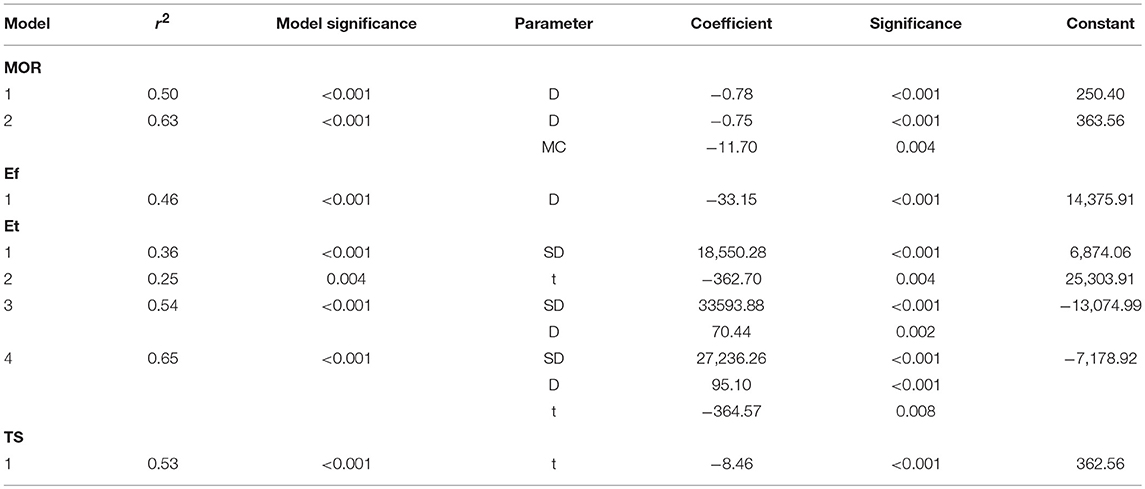
Table 10. Multiple linear regression models for mechanical properties of bamboo Dendrocalamus asper (bamboo Petung).
Furthermore, SD of the bamboo culms can also be estimated through measuring only the culm diameter and wall thickness by using Equation 9.
Application of Bamboo for Composite Fabrication for Reinforced Concrete
Concrete is currently being used widely around the world for major projects in building and construction industry. However, concrete has a major drawback; it has low tensile strength. Therefore, when it is used in applications where it has to sustain tensile forces, large cracks and premature failure are unavoidable.
To overcome this limitation, reinforcement bars with high tensile strength are used in structural concrete. Currently steel reinforcement is being used in a wide range of structural concrete buildings and infrastructure. However, a challenge associated with using steel reinforcement in concrete is the corrosion and the corrosion-related degradation of the reinforced concrete member. Corrosion of the steel reinforcement in concrete is initiated by either concrete carbonation or exposure of the concrete element to chloride ions as discussed in various works (Slater, 1983; Macias and Andrade, 1987). Therefore, in the presence of oxygen and moisture, the corrosion of rebar results in dissolution of iron in the form of ferrous hydroxide [Fe(OH2)] which subsequently forms a layer of rust surrounding the reinforcement bar. As a result of rust occupying a volume larger than the reinforcement bar, large tensile forces are generated in the concrete in the form of tensile stress. The tensile forces initiate the cracking of the concrete layers around the steel bars in the form of concrete delamination, or the debonding of the reinforcement bars from the concrete (Bertolini et al., 2013).
Alternative reinforcement materials including Fiber Reinforced Polymers (FRP) that do not corrode since the polymer matrix protects the fibers. They have comparable mechanical properties to steel reinforcement. There has been much interest in the application of natural fibers in the production of FRP composites to replace synthetic fibers, such as glass and carbon fibers. Natural fibers are widely available in nature and therefore require relatively low energy for production. When natural fibers are used in FRP composite production, they can result in high-performance composites which could potentially replace all, if not many, of the synthetic composite materials at lower prices for applications in the building and construction sector, where weight savings have significant impact on lowering energy consumption and overall cost of the building or infrastructure.
The application of natural fiber reinforced polymer composites in the building and construction industry has been successful in recent years, but mainly as non-structural elements applied as an insulation element for structural members, for floor and wall covers, in door and window frames, for fitting elements, such as door and window handles and for fencing. Among the various high-strength natural materials, bamboo is considered one of the oldest natural construction materials used in buildings particularly in South America, Africa and, in particular, in Southeast Asia.
The variation in the properties of bamboo FRP composites fabricated by various groups around the world is mainly associated with the species of bamboo, the length of bamboo fiber used in fabrication, fiber orientation, the type of epoxy/resin used and the type of treatment carried out on raw bamboo fibers (Ichhaporia, 2008). So far, no investigation has been carried out on utilizing bamboo for the fabrication of FRP composites for structural and load-bearing elements in the construction and building sector. Much of the work on bamboo FRP composites is dedicated to non-load-bearing elements, e.g., fencing or flooring in buildings where the structural properties and mechanical capacities are far lower than for any structural member, such as beams and columns (Jindal, 1986; Nugroho and Ando, 2000; Okubo et al., 2004). This research aims to fill this gap by providing a novel approach to enhance the properties of bamboo FRP composites through new processing and fabricating techniques for bamboo FRP composites and subsequently, through using the new material as reinforcing for structural-concrete elements.
Bamboo Composite Fabrication Using Material Correlation Relationships
In this study bamboo Dendrocalamus asper or Petung bamboo was used for fabrication of the high performance bamboo-fiber-reinforced polymer composite. In a detailed study carried out recently by the research team, patented processing tools were developed to process bamboo culms into bamboo fiber bundles of varying thicknesses, width and length (Hebel et al., 2014; Hebel and Heisel, 2016; Javadian et al., 2016; Javadian, 2017).
The processed bamboo fibers were first dried, in an air-circulated oven at 80°C until the moisture content was < 10%. The moisture content was measured according to the ASTM D4442-07 standard test method. Subsequently processed bamboo fibers were sorted according to their thickness. The raw bamboo fiber bundles used in the study represented an average fiber collection from upper, middle and lower sections of the bamboo culm in nearly equal ratios.
Before processing the bamboo culms into fiber bundles, tensile and flexural properties of the raw materials were assessed only based on the correlation relationships established in section Correlation Studies and Statistical Modeling of Physical and Mechanical Properties assuming no testing devices available. The average culm diameter and wall thickness of bamboo used in this study were 90 and 8 mm, respectively. By using the material property relationships, the respective mechanical properties of bamboo culms can be found as below;
These values were used as the basis for the fabrication of bamboo-fiber-reinforced polymer composite in this study. Further evaluation of these numbers were carried out by measuring the mechanical properties of the final bamboo composite samples and comparing the results with the raw bamboo properties found based on the material relationships.
A two-component epoxy system with a resin and a hardener was employed as matrix. Once the resin and hardener of the epoxy system were mixed, each bamboo fiber bundle was impregnated with the epoxy matrix and aligned along the fiber direction. The impregnated fiber bundles were stacked on one another to form a layered structure. Subsequently, the impregnated bamboo fiber bundles were subjected to different pressures (between 15 and 25 MPa) and temperatures (between 80 and 140°C) at various press/hold times to achieve densely compressed composites. The boards were finally post-cured for another 48 h at a temperature of 55°C and then prepared into the suitable shapes to measure their mechanical properties. The post-curing curing time was to ensure that the optimal cross-link networks were fully developed at the recommended temperature by providing the essential energy to give the epoxy molecules the flexibility needed to move, and to fully form the networks within the microstructural cross sections of the epoxy matrix. The average specific density of the bamboo composite reinforcements was 1.33. This procedure provides sufficient environmental protection for the fibers, thus ensuring that their properties do not degrade over time (Javadian, 2017).
Figure 4 shows a bamboo composite reinforcing bar after it was removed from the hot-press machine.
Tensile properties of the bamboo composite sample, including tensile strength and modulus of elasticity in tension, were measured according to ASTM D3039-08, “Standard Test Method for Tensile Properties of Polymer Matrix Composite Materials” while flexural properties, including Modulus of Rupture (MOR) and modulus of elasticity in flexure, were measured according to ASTM D7264, “Standard Test Method for Flexural Properties of Polymer Matrix Composite Materials through a four-point bending test.” All the tests were carried out by using a Shimadzu AG-IC 100 kN machine. At least five specimens have been tested for each mechanical property and results exceeding a 10% standard deviation range, which was statistically set as confidence interval, were discarded. Table 11 displays the mechanical properties of bamboo composite samples fabricated in this study.
As shown in Table 11 the average mechanical properties of bamboo composite samples are higher than the average mechanical properties of raw bamboo fiber bundles. The results show that the novel techniques for processing the bamboo into fiber bundles together with new production methods used in this study improved the mechanical properties of the final bamboo composite. This has also been observed by Hebel et al. (2014), Javadian (2017), and Rahman et al. (2017). When the modulus of elasticity in flexure of the bamboo composite board is compared to the raw bamboo properties, an improvement of up to two times the raw bamboo modulus of elasticity in flexure is observed. Similarly, MOR, tensile strength and modulus of elasticity in tension of the bamboo composite boards are enhanced compared with the raw material by 30, 2, and 39%, respectively.
The correlation relationships helped to save the time needed for preliminary testing of the raw material before composite fabrication. Furthermore, through this study it is demonstrated that by employing novel techniques based on only mechanical processes, naturally available raw materials (such as bamboo) can be turned into high performance composite materials with applications in building industry for reinforcing structural concrete.
Reinforced Concrete Design Using Bamboo Composite Reinforcement
There are two types of reinforcement used in reinforcing the concrete beams: longitudinal and transverse (shear) reinforcement. The longitudinal reinforcements are placed parallel to the long axis of the beam to provide the required tensile capacity, while shear reinforcement is used to provide sufficient shear strength perpendicular to the long axis of the concrete beam.
All the bamboo composite reinforcement produced in this study have square cross sections of 10 × 10 mm. The square cross section is the result of the production process of the bamboo composite materials as explained earlier. The most common reinforcement currently being used for structural concrete have round cross sections with and without ribs on the surface, including steel and Glass Fiber Reinforced Polymer reinforcement (GFRP) systems. However, in this study, for simplicity only square cross sections are studied (Javadian, 2017). According to American Concrete Institute (ACI) 318 “Building Code Requirements for Structural Concrete and Commentary” (American Concrete Institute, 2008), to provide sufficient confinement to the longitudinal reinforcement of the beam, the shear reinforcement has a closed-loop shape in which it stays intact before failure is initiated from the longitudinal tension reinforcement. Furthermore, by having closed loop shape the failure of the concrete beam does not begin by the failure of the shear reinforcement. Instead, failure of the longitudinal reinforcement is observed. Figure 5 displays the bamboo composite reinforcement system developed in this study for reinforcing concrete beam samples.
The bent portion of the shear reinforcement has lower mechanical properties compared with the straight parts of the shear reinforcements. Earlier study on the different types of Fiber Reinforced Polymer (FRP) shear reinforcements, including Glass Fiber Reinforced Polymer (GFRP) reinforcements, had shown a tensile strength reduction of up to 45% of the strength parallel to the fibers' direction for the bent sections, due to the localized stress concentration as a result of the curvature which introduced radial stresses within the bent portions (Javadian, 2017).
In an earlier study carried out by the research team, bonding mechanism of bamboo composite reinforcement system to the surrounding concrete matrix was investigated in detail (Javadian et al., 2016). A sufficient bond mechanism between concrete and bamboo composite reinforcements has contributed to a higher ultimate load-bearing capacity of the reinforced concrete member. It was shown that by providing an interfacial microstructure (coating system) which ensured smooth tensile stress transfer between concrete and reinforcement system the maximum mechanical capacities of the bamboo composite reinforcement could be activated thus resulting in a higher ultimate load-bearing capacity compare to non-coated reinforcement.
A series of pull-out tests were designed to find a suitable technique which enhanced the bonding between the two materials. To enhance the bond mechanism between the bamboo composite reinforcement and concrete matrix, four types of coatings and two bonding lengths of 200 mm (20 × thickness) and 100 mm (10 × thickness) were considered in the earlier study. A waterproof vapor barrier membrane system, a bio-based epoxy resin system, a two-part epoxy resin general coating and a two-part epoxy resin based surfacing system with and without sand particles were among the coatings used to investigate the bond mechanism. The average bond strength of bamboo composite reinforcement coated with waterproof vapor barrier membrane system and sand particles with an embedment length of 200 mm was similar to the bond strength of the plain Glass Fiber Reinforced Polymer (GFRP) reinforcement in normal strength concrete. Therefore, to evaluate the bamboo composite reinforcement within concrete beam samples, firstly they were coated with a coating and secondly an embedment length of 20 times the thickness of bamboo composite refinement was included as part of the beam design (Javadian et al., 2016).
The coating applied to the surface of the bamboo-composite reinforcement provides long-term resistance to alkaline environments and water ingress from the concrete matrix. Therefore, in concrete having an alkaline environment, the addition of coating on the surface of reinforcement bars provides supplementary protection for the reinforcement (in addition to the epoxy matrix) against long-term degradation and ensures the required bonding with the concrete matrix.
The American Concrete Institute's (ACI) guide for the design and construction of structural concrete reinforced with Fiber Reinforced Polymer (FRP) bars (ACI 440.1R-15) was used as the primary guideline during the design and evaluation of the bamboo composite reinforced concrete beams in this study (American Concrete Institute, 2015). ACI 440.1R-15 has provided the necessary design guides for the application of FRP materials as reinforcement in concrete to justify the lower ductility of the FRP (e.g., GFRP) reinforced concrete elements as compared with steel reinforced concrete members. The size of the bamboo composite reinforcement and the concrete beam in this study were designed in a way such that the loading capacity of the testing machine was not exceeded. All the longitudinal reinforcement in this study had similar cross-sectional dimensions of 10 × 10 mm, while the thickness of the shear reinforcement was 6 mm. Figure 6 displays the schematic view of the concrete-beam cross-section reinforced with bamboo composite reinforcement.
In this study, all bamboo composite reinforced concrete beams had cross sections of 160 × 160 mm and total length of 1,300 mm while their loading span (L) was kept at 1,050 mm according to four-point (or so-called third-point loading) flexural test set-up. The four-point loading set-up allowed for a zero shear zone along the middle section of the bamboo composite reinforced concrete beam. The zero shear zone permits the elimination of the shear reinforcement in this study, thus the longitudinal reinforcement is loaded completely in tension and flexure and the calculation of the ultimate load-bearing capacity of the concrete beams became simplified. The longitudinal reinforcement had cross sections of 10 × 10 mm. A total of 15 concrete beams with a compressive strength of 20 MPa were prepared and tested in this study. The arrangement of the reinforcement and the loading distance are summarized in Table 12.
A total of three design scenarios were considered in this study by either changing the number of bottom reinforcement or number and spacing of the shear reinforcement as displayed in Table 12. For each design scenario, five samples were prepared and tested. Two reinforcement bars were used as top compression reinforcement for all the beams tested in this study. The concrete beams were tested until failure and for each test, ultimate failure load, ultimate flexural capacity (MOR), load corresponding to first crack, and flexural capacity at the time of first crack were obtained. Table 13 contains the results of the flexural tests.
Figure 7 displays one of the beams tested in this study after ultimate failure. To evaluate the results obtained in this section on the ultimate failure load with respect to the recommendations and calculations specified in ACI 440.1R-15, a series of computations based on ACI 440.1R-15 were carried out to estimate the failure load.
Table 14 shows the comparison of the cracking loads, nominal and design ultimate failure loads between the values measured during the tests and design values obtained according to ACI 440.1R-15 standard recommendations. The values presented for the experimental results were the average values obtained for each beam series shown in Table 13.

Table 14. Comparison between ACI 440.1R-15 design values and experimental results obtained in this study.
The bamboo composite reinforcement showed better initial cracking load and much higher ultimate load-bearing capacity compared to the design values obtained through calculations according to ACI 440.1R-15. The estimated design cracking loads based on ACI 440.1R-15 were lower than the values obtained by testing the bamboo composite reinforced concrete beams. The cracking loads measured during the four-point flexural test of the beams on average was 2–5 times larger than the design values of the ACI 440.1R-15 standard, confirming the superior performance of the bamboo composite reinforcement in comparison to the estimates according to the ACI standard. Beam samples with only two bamboo composite reinforcement bars at the tension side of the concrete beam cross section failed mainly due to the rupture of the reinforcement, while beam samples with 4 bamboo composite tensile reinforcement bars had a tendency to fail due to concrete crushing at the compression side of the beam. In both cases, the bamboo-composite reinforcement performed well, showing that it is a suitable alternative to steel and GFRP reinforcement for concrete structures in terms of mechanical capacity and technical feasibility.
Conclusion
Bamboo Dendrocalamus asper locally known as bamboo Petung from Indonesia was selected to correlate its mechanical properties with physical properties of the culm including culm geometry, specific density and moisture content for composite fabrication for use in structural concrete. Based on the results obtained from the first part of this study, the following conclusions are relevant:
• Physical properties of the bamboo culm can be used to estimate the mechanical potential of bamboo for use in production of novel bamboo-based composite material applications in the building and construction sector.
• Mechanical properties of bamboo sections often decrease with increasing the wall thickness of the culm. This is associated with the reduced volumetric ratio of cellulose fibers to lignin as culm diameter increases.
• This study provides a simple method that allows estimation of the mechanical properties of bamboo through non-destructive measurement of only wall thickness and diameter. This capability is particularly useful in contexts of nurseries and in forests where there is limited access to testing facilities.
These findings are then used for the selection process of the raw bamboo for structural composite production when specific mechanical properties are required. A case study and independent mechanical testing of the novel bamboo-based composite reinforcement in concrete successfully validates the relationships proposed in this paper. Further work involves investigation of compressive and shear strength of bamboo, such as Dendrocalamus asper and evaluating the dependence on culm geometry including culm diameter, wall thickness and height. Further studies on microstructural analysis of bamboo-based composite reinforcement and the correlation with mechanical properties of bamboo will also be carried out.
Author Contributions
AJ designed and performed the experiments. AJ and NS derived the models and analyzed the data. AJ and NS wrote the manuscript in consultation with IS and DH. IS was involved in planning and supervised the work. DH contributed to the implementation of the research. All authors discussed the results and commented on the manuscript.
Funding
The research was conducted at the Future Cities Laboratory at the Singapore-ETH Centre, which was established collaboratively between ETH Zurich and Singapore's National Research Foundation (FI 370074016) under its Campus for Research Excellence and Technological Enterprise programme.
Conflict of Interest Statement
The authors declare that the research was conducted in the absence of any commercial or financial relationships that could be construed as a potential conflict of interest.
Acknowledgments
The authors would like to gratefully acknowledge the support of Sawiris Foundation for Social Development and Singapore-MIT Alliance for Research and Technology Innovation Center in Singapore.
References
Alvin, K., and Murphy, R. (1988). Variation in fibre and parenchyma wall thickness in culms of the bamboo Sinobambusa tootsik. IAWA J. 9, 353–361. doi: 10.1163/22941932-90001095
American Concrete Institute (2008). Building Code Requirements for Structural Concrete (ACI 318–08) and Commentary. Farmington Hills, MI: American Concrete Institute.
American Concrete Institute (2015). ACI 440.1R-15 Guide for the Design and Construction of Structural Concrete Reinforced with Fiber-Reinforced Polymer Bars. Farmington Hills, MI: ACI Committee 440.
Archila, H., Kaminski, S., Trujillo, D., Zea Escamilla, E., and Harries, K. A. (2018). Bamboo reinforced concrete: a critical review. Mat. Struc. 51:102. doi: 10.1617/s11527-018-1228-6
ASTM International (2011). Standard Test Methods for Structural Panels in Flexure. ASTM D3043–00(2011). West Conshohocken, PA: ASTM International.
ASTM International (2014a). Standard Test Methods for Density and Specific Gravity (Relative Density) of Wood and Wood-Based Materials. ASTM D2395-14e1. West Conshohocken, PA: ASTM International.
ASTM International (2014b). Standard Test Methods for Small Clear Specimens of Timber. ASTM D143-14. West Conshohocken, PA: ASTM International.
ASTM International (2015). Standard Test Methods for Direct Moisture Content Measurements of Wood and Wood-Base Materials. ASTM D4442-15. West Conshohocken, PA: ASTM International.
Bertolini, L., Elsener, B., Pedeferri, P., Redaelli, E., and Polder, R. B. (2013). Corrosion of Steel in Concrete: Prevention, Diagnosis, Repair. Weinheim: John Wiley & Sons.
Chen, H., Miao, M., and Ding, X. (2009). Influence of moisture absorption on the interfacial strength of bamboo/vinyl ester composites. Compos. Part A. Appl. S. 40, 2013–2019. doi: 10.1016/j.compositesa.2009.09.003
Correal, D., Francisco, J., and Arbeláez, C. (2010). Influence of age and height position on Colombian Guadua angustifolia bamboo mechanical properties. Maderas. Ciencia Tecnol. 12, 105–113. doi: 10.4067/S0718-221X2010000200005
Faruk, O., Bledzki, A. K., Fink, H. P., and Sain, M. (2014). Progress report on natural fiber reinforced composites. Macromol. Mater. Eng. 299, 9–26. doi: 10.1002/mame.201300008
Febrianto, F., Hidayat, W., Bakar, E. S., Kwon, G.-J., Kwon, J.-H., Hong, S.-I., et al. (2012). Properties of oriented strand board made from Betung bamboo (Dendrocalamus asper (Schultes. f) Backer ex Heyne). Wood Sci. Technol. 46, 53–62. doi: 10.1007/s00226-010-0385-8
Gibson, L. J. (2012). The hierarchical structure and mechanics of plant materials. J. R. Soc Interface 9, 2749–2766. doi: 10.1098/rsif.2012.0341
Green, D. W., Winandy, J. E., and Kretschmann, D. E. (1999). “Wood handbook: mechanical properties of wood,” in General Technical Report FPL-GTR-113, ed F. S. Department of Agriculture, Forest Product Laboratory (Madison, WI: United States Department of Agriculture), 4-1–4-44.
Hebel, D., and Heisel, F. (2016). Bamboo Composite Material for Structural Applications and Method of Fabricating the Same.
Hebel, D. E., Javadian, A., Heisel, F., Schlesier, K., Griebel, D., and Wielopolski, M. (2014). Process-controlled optimization of the tensile strength of bamboo fiber composites for structural applications. Compos. Part B Eng. 67, 125–131. doi: 10.1016/j.compositesb.2014.06.032
Javadian, A. (2017). Composite Bamboo and its Application as Reinforcement in Structural Concrete. Zurich: ETH Zurich.
Javadian, A., Wielopolski, M., Smith, I. F., and Hebel, D. E. (2016). Bond-behavior study of newly developed bamboo-composite reinforcement in concrete. Constr. Build Mater. 122, 110–117. doi: 10.1016/j.conbuildmat.2016.06.084
Jindal, U. (1986). Development and testing of bamboo-fibres reinforced plastic composites. J. Compos. Mater. 20, 19–29. doi: 10.1177/002199838602000102
Kamruzzaman, M., Saha, S., Bose, A., and Islam, M. (2008). Effects of age and height on physical and mechanical properties of bamboo. J. Trop. For Sci. 211–217.
Kaur, P. J., Kardam, V., Pant, K., Naik, S., and Satya, S. (2016). Characterization of commercially important Asian bamboo species. Eur. J. Wood Wood Prod. 74, 137–139. doi: 10.1007/s00107-015-0977-y
Kushwaha, P. K., and Kumar, R. (2009). Studies on water absorption of bamboo-polyester composites: effect of silane treatment of mercerized bamboo. Polym. Plast. Technol. Eng. 49, 45–52. doi: 10.1080/03602550903283026
Lakkad, S., and Patel, J. (1981). Mechanical properties of bamboo, a natural composite. Fibre Sci. Technol. 14, 319–322. doi: 10.1016/0015-0568(81)90023-3
Lee, A. W., Bai, X., and Peralta, P. N. (1996). Physical and mechanical properties of strandboard made from moso bamboo. Forest Prod. J. 46:84.
Liese, W. (1985). “Anatomy and properties of bamboo,” in International Bamboo Workshop (Hangzhou), 196–208.
Liese, W., and Jackson, A. (1985). Bamboos Biology, Silvics, Properties, Utilization. Eschborn: Deutsche Gesellschaft für Technische Zusammenarbeit (GTZ).
Lo, T. Y., Cui, H., and Leung, H. (2004). The effect of fiber density on strength capacity of bamboo. Mater. Lett. 58, 2595–2598. doi: 10.1016/j.matlet.2004.03.029
Lo, T. Y., Cui, H., Tang, P., and Leung, H. (2008). Strength analysis of bamboo by microscopic investigation of bamboo fibre. Constr. Build Mater. 22, 1532–1535. doi: 10.1016/j.conbuildmat.2007.03.031
Macias, A., and Andrade, C. (1987). Corrosion of galvanized steel reinforcements in alkaline solutions: Part 1: electrochemical results. Br. Corr. J. 22, 113–118. doi: 10.1179/000705987798271631
Malanit, P., Barbu, M., and Frühwald, A. (2009). The gluability and bonding quality of an asian bamboo (“dendrocalamus asper”) for the production of composite lumber. J. Trop. For. Sci. 21, 361–368.
Malanit, P., Barbu, M. C., and Frühwald, A. (2011). Physical and mechanical properties of oriented strand lumber made from an Asian bamboo (Dendrocalamus asper Backer). Eur. J. Wood Wood Prod. 69, 27–36. doi: 10.1007/s00107-009-0394-1
Mohmod, A. L., Amin, A. H., Kasim, J., and Jusuh, M. Z. (1993). Effects of anatomical characteristics on the physical and mechanical properties of Bambusa blumeana. J. Trop. For. Sci. 6, 159–170.
Murphy, R., and Alvin, K. (1992). Variation in fibre wall structure in bamboo. IAWA J. 13, 403–410. doi: 10.1163/22941932-90001296
Nordahlia, A., Anwar, U., Hamdan, H., Zaidon, A., Paridah, M., and Razak, O. A. (2012). Effects of age and height on selected properties of Malaysian bamboo (Gigantochloa levis). J. Trop. For Sci. 102–109.
Nugroho, N., and Ando, N. (2000). Development of structural composite products made from bamboo I: fundamental properties of bamboo zephyr board. J. Wood Sci. 46, 68–74. doi: 10.1007/BF00779556
Nugroho, N., and Ando, N. (2001). Development of structural composite products made from bamboo II: fundamental properties of laminated bamboo lumber. J. Wood Sci. 47, 237–242. doi: 10.1007/BF01171228
Okubo, K., Fujii, T., and Yamamoto, Y. (2004). Development of bamboo-based polymer composites and their mechanical properties. Compos. Part A. Appl. S. 35, 377–383. doi: 10.1016/j.compositesa.2003.09.017
Rahman, N., Shing, L. W., Simon, L., Philipp, M., Alireza, J., Ling, C. S., et al. (2017). Enhanced bamboo composite with protective coating for structural concrete application. Energy Procedia 143, 167–172. doi: 10.1016/j.egypro.2017.12.666
Rao, I. R., Gnanaharan, R., and Sastry, C. B. (1988). “Bamboos. current research. proceedings of the international bamboo workshop, Cochin, India, 14–18 Nov. 1988,” in: Bamboos. Current Research (Cochin: Kerala Forest Research Institute), 217–290.
Ray, A. K., Das, S. K., Mondal, S., and Ramachandrarao, P. (2004). Microstructural characterization of bamboo. J. Mater. Sci. 39, 1055–1060. doi: 10.1023/B:JMSC.0000012943.27090.8f
Rowel, R., and Norimoto, M. (1988). Dimensional stability of bamboo particleboards made from acetylated particles. Mokuzai Gakkaishi 34, 627–629.
Slater, J. E. (1983). Corrosion of Metals in Association with Concrete: A Manual Sponsored by ASTM Subcommittee G01. 14 on Corrosion of Reinforcing Steel, and Metal Properties Council. Philadelphia, PA: ASTM International.
Wahab, R., Mustapa, M., Sulaiman, O., Mohamed, A., Hassan, A., and Khalid, I. (2010). Anatomical and physical properties of cultivated two-and four-year-old Bambusa vulgaris. Sains Malays. 39, 571–579. Available online at: http://www.ukm.my/jsm/
Wakchaure, M., and Kute, S. (2012). Effect of moisture content on physical and mechanical properties of bamboo. Asian J. Civ. Eng. (Build Hous). 13, 753–763.
Wegst, U., and Ashby, M. (2004). The mechanical efficiency of natural materials. Philos. Magazine 84, 2167–2186. doi: 10.1080/14786430410001680935
Youssefian, S., and Rahbar, N. (2015). Molecular origin of strength and stiffness in bamboo fibrils. Sci. Rep. 5:11116. doi: 10.1038/srep11116
Yu, H., Jiang, Z., Hse, C., and Shupe, T. (2008). Selected physical and mechanical properties of moso bamboo (Phyllostachys pubescens). J. Trop. For. Sci. 258–263.
Yu, Y., Wang, H., Lu, F., Tian, G., and Lin, J. (2014). Bamboo fibers for composite applications: a mechanical and morphological investigation. J. Mater. Sci. 49, 2559–2566. doi: 10.1007/s10853-013-7951-z
Zaidon, A., Paridah, M., Sari, C., Razak, W., and Yuziah, M. (2004). Bonding characteristics of Gigantochloa scortechinii. J. Bamboo Rattan 3, 57–65. doi: 10.1163/156915904772875644
Keywords: bamboo, natural fiber reinforced polymer, mechanical properties, culm geometry, correlation study, statistical analysis
Citation: Javadian A, Smith IFC, Saeidi N and Hebel DE (2019) Mechanical Properties of Bamboo Through Measurement of Culm Physical Properties for Composite Fabrication of Structural Concrete Reinforcement. Front. Mater. 6:15. doi: 10.3389/fmats.2019.00015
Received: 22 October 2018; Accepted: 25 January 2019;
Published: 13 February 2019.
Edited by:
Fernando Fraternali, University of Salerno, ItalyReviewed by:
Enrico Radi, University of Modena, ItalyAnastasiia O. Krushynska, University of Trento, Italy
Copyright © 2019 Javadian, Smith, Saeidi and Hebel. This is an open-access article distributed under the terms of the Creative Commons Attribution License (CC BY). The use, distribution or reproduction in other forums is permitted, provided the original author(s) and the copyright owner(s) are credited and that the original publication in this journal is cited, in accordance with accepted academic practice. No use, distribution or reproduction is permitted which does not comply with these terms.
*Correspondence: Alireza Javadian, amF2YWRpYW5AYXJjaC5ldGh6LmNo