- 1State Key Laboratory of Integrated Management of Pest Insects and Rodents, Institute of Zoology, Chinese Academy of Sciences, Beijing, China
- 2University of Chinese Academy of Sciences, Beijing, China
Huddling as social thermoregulatory behavior is commonly used by small mammals to reduce heat loss and energy expenditure in the cold. Our study aimed to determine the effect of huddling behavior on energy conservation, thermogenesis, core body temperature (Tb) regulation and body composition in Brandt's voles (Lasiopodomys brandtii). Adult captive-bred female Brandt's voles (n = 124) (~50 g) in 31 cages with 4 individuals each were exposed to cool (23 ± 1°C) and cold (4 ± 1°C) ambient temperatures (Ta) and were allowed to huddle or were physically separated. The cold huddling (Cold-H) groups significantly reduced food intake by 29% and saved digestible energy 156.99 kJ/day compared with cold separated groups (Cold-S); in cool huddling groups (Cool-H) the reduction in food intake was 26% and digestible energy was saved by 105.19 kJ/day in comparison to the separated groups (Cool-S). Resting metabolic rate (RMR) of huddling groups was 35.7 and 37.2% lower than in separated groups at cold and cool Tas, respectively. Maximum non-shivering thermogenesis (NSTmax) of huddling voles was not affected by Ta, but in Cold-S voles it was significantly increased in comparison to Cool-S. Huddling groups decreased wet thermal conductance by 39% compared with separated groups in the cold, but not in the cool Ta. Unexpectedly, huddling voles significantly decreased Tb by 0.25 – 0.50°C at each Ta. Nevertheless, activity of Cold-H voles was higher than in Cold-S voles. Thus, huddling is energetically highly effective because of reduced metabolic rate, thermogenic capacity and relaxed Tb regulation despite the increase of activity. Therefore, Brandt's voles can remain active and maintain their body condition without increased energetic costs during cold exposure. This study highlights the ecological significance of huddling behavior for maintenance of individual fitness at low costs, and thus survival of population during severe winter in small mammals.
Introduction
Winter is a stressful period for mammals when the majority ceases reproduction and allocates nutrients and fuel for the maintenance of the organism. Small mammals generally are more strongly affected than large mammals because energy requirements per unit of body mass are high due to the large surface area to volume ratio. Therefore, when environmental stressors persist for prolonged periods and available resources are limited small species will be challenged. To a large extent because of this, living in seasonal cold environment requires individual adjustments in morphology and physiology and also cooperative behavior by groups for communal nest sharing and storage of food (Wolff and Lidicker, 1981). Among cooperative behaviors huddling is an important social thermoregulatory behavior for group living species.
Huddling as an active and close aggregation of animals is used by many endotherms to reduce heat loss and lower energy expenditure and possibly allowing them to reallocate the saved energy to other functions such as growth or reproduction (Gilbert et al., 2010). Energetic advantages of huddling increase with lowered Ta, increased group size and are mainly due to a reduced surface to volume ratio (Vickery and Millar, 1984; Canals et al., 1997; Nuñez-Villegas et al., 2014). The benefits of huddling in energy conservation (Putaala et al., 1995; Scantlebury et al., 2006; Kotze et al., 2008), local environment heating (Hayes et al., 1992; Nowack and Geiser, 2016) and survival (Sealander, 1952) have been studied in several species. In cold environments some small homeothermic mammals slightly lowered their core Tb (Chi and Wang, 2011; Nieminen et al., 2013). Yet huddling reduces the heat loss, its effect on core Tb regulation in huddling animals remains controversial (Andrews et al., 1987; Boix-Hinzen and Lovegrove, 1998; Fortin et al., 2000). Moreover, cold-exposed group of 4 mice exhibited a substantial increase in total huddling and a decrease in total activity relative to warm-acclimated group of mice only during the dark phase of 24 h period (Batchelder et al., 1983). Whether the activity pattern in huddling animals is affected by longer period of acclimation remains unexplored. To the extent of our knowledge, direct evaluation for maximum capacity for non-shivering thermogenesis (NSTmax) has not been examined in small mammals living in group during adaptation to low temperature. Few studies only mentioned that mice living in group developed less brown adipose tissue in the cold (Heldmaier, 1975) or the activity of uncoupling protein 1 was suppressed due to increased housing mice density (Himms-Hagen and Villemure, 1992).
Brandt's voles (Lasiopodomys brandtii) are small non-hibernating herbivorous rodents that are widely distributed in the dry steppe zone of Mongolia, the southeast of Baikal region of Russia and the Inner Mongolian grasslands of Northern China (Zhang and Wang, 1998; Avirmed, 2003). Their habitat is characterized by extreme continental climatic condition with long cold and dry winters and deep frozen soil. Brandt's voles live in family groups in complex burrow systems. The physiological mechanisms of individual Brandt's vole to the cold acclimation have been studied (Li and Wang, 2005; Zhang and Wang, 2006; Tang et al., 2009; Zhang et al., 2015). For example, individual Brandt's voles exposed to cold increase their resting metabolic rate (RMR), energy intake and uncoupling protein content in brown fat (Zhang and Wang, 2006). To date, there is no information on their adaptive strategies when they are in groups. Therefore, our study aimed to determine the significance of huddling behavior in group of Brandt's voles on energy conservation, NSTmax, Tb regulation, activity pattern, and body composition as a function of Ta. We predicted that (i) huddling would reduce energy intake, (ii) change body composition, (iii) reduce energy expenditure, (iv) reduce NSTmax, (v) keep Tb higher, (vi) and reduce activity in comparison to separated voles in the cold.
Materials and Methods
Experimental Design and Animals
Adult female voles with a body mass of 28–70 g and about 4 months old from a breeding colony at Institute of Zoology, CAS were examined. They were housed under laboratory condition with light regime 16L: 8D h s (light on from 4:00 to 20:00) and Ta 23 ± 1°C. For experiments we preferred to use 4 sibling voles, but also substituted the lacking siblings by up to 2 voles of similar age; animals had 3 weeks to acclimate to the cage (42 × 27 × 20 cm) and cage mates. Individual voles were dyed for identification. The cages had 4 equal compartments separated by stainless steel walls with small holes (6 mm) and connected by passageways. For huddling groups the passageways were opened in order to provide free movement of voles; passageways were closed for separated groups, but they had possibilities of olfactory, visual and vocal contacts. To reveal the effects of huddling we compared 4 experimental groups in different grouping conditions (huddling and separated) and Tas (cold 4 ± 1°C and cool 23 ± 1°C) for 4 weeks of the experimental period. The group size (4 voles in each cage) used in this experiment was chosen to ensure compact groups in which most animals remained inactive in a huddle. There were 8 cages for Cool-H, 9 cages for Cool-S, 7 cages for Cold-H and 7 cages for Cold-S groups, respectively. In the experiment we used 31 cages with a total of 124 voles. During the experimental period voles were provided with standard rabbit pellet chow (Beijing KeAo Bioscience Co.) and water ad libitum and wood shavings as bedding except period of food intake measurements. The study was carried out in accordance with recommendations of the Animal Care and Use Committee of the Institute of Zoology, the Chinese Academy of Sciences. The protocol was approved by the Animal Care and Use Committee of the Institute of Zoology, Chinese Academy of Sciences (IOZ16042).
Body Mass and Food Intake
Body mass of voles was measured before experiment and once in every week during the experiment by using an electronic balance (Sartorius Model BL 1500, ± 0.1 g). Food intake was measured 3 times by groups, once before the experiment as baseline when all cages were in the same Ta (23°C) and huddled together and next 2 measurements in the second and third week during the treatment. Each food intake measurement lasted for 3 consecutive days and animals were provided with ad libitum food and 5 g tissue paper in group voles and 3 g tissue paper for each separated voles for absorbing urine. The food given to the voles was weighed and dry mass calculated according to the measured water content of samples. Food intake was calculated by subtracting the uneaten from the total offered food. Energy contents of the food and feces were determined with a Parr 1281 oxygen bomb calorimeter (Parr Instrument Company, Moline, Illinois, USA). Gross energy intake (GEI), digestible energy intake (DEI) and apparent digestibility were calculated based on (Grodzinski and Wunder, 1975; Liu et al., 2003):
RMR and NSTmax
RMR was measured by groups of voles both huddling (4 voles in a transparent plastic chamber with a volume of 5.8L) and separated (4 voles in the same chamber but separated by double-layered dividing meshes) for 3 h at their acclimation Tas 4 and 23°C in open-circuit respirometry system (TSE labmaster, Germany). The air flow rate was 3L/min. The open circuitry respirometry system monitored 4 metabolic chambers in one running of the measurement. Three metabolic chambers were used for oxygen consumption of group voles and one blank chamber used for a baseline in sequence, but all sampled in every 6 min. We took the average of consecutive, stable and minimum 3 readings of oxygen consumption as the RMR at least after 1 h acclimation to the chamber.
The capacity for NST was determined individually at Ta 25°C 2 days after the RMR measurements. The volume of transparent plastic chamber was 2.7 L, and flow rate was 1 L/min. We took voles from their acclimation Ta room and injected norepinephrine (NE) subcutaneously (Shanghai Harvest Pharmaceutical Co. Ltd) to induce the maximum NST. The dosage of NE was calculated by the formula NE (mg/kg) = 2.53W−0.4 for Brandt's voles (Wang and Wang, 2006). The NSTmax was estimated as the stable 4 highest consecutive 3–min readings of oxygen consumption after 15–20 min of injection during 1 h measurements.
Wet Thermal Conductance (C)
Group wet thermal conductance was determined by formula C = RMR/ (Tb-Ta) (Scholander et al., 1950; McNab, 1980). However, as we did not measure Tb directly during RMR, we assumed that the averaged daytime Tb derived from transponder data from each group at end of the acclimation period were equivalent to the Tb during RMR measurements because voles were exposed to same Ta.
Core Body Temperature (Tb) and Locomotor Activity (Activity)
Tb and activity were recorded via intraperitoneally implanted transponders (G2 E-Mitter, to ± 0.01°C, STARR life sciences). Prior to implantation surgery we calibrated the transponders in water bath to the nearest 0.1°C against a precision mercury thermometer. Before the surgery the transponder and surgical apparatus were sterilized in a 75% by volume alcohol solution for 30 min. Animals were anesthetized by injection of pentobarbital sodium (1%) with a dose of 50 mg/kg. After sterilizing the skin with an iodophor (Nanjing modern sanitation & anti-epidemic products Co.Ltd) we made a small (~1 cm) incision along the midline of the abdominal skin and muscles ~ 1 cm caudal to the diaphragm to open the abdomen. After insertion of the transponders, the wound was closed with absorbable PGA surgical suture (Jinhuan Model R413, 4/0) and sterilized with iodophor again. During surgery, a temperature controlled blanket (Temp control II 908100 by TSE systems) was used to prevent hypothermia until animal had recovered from anesthesia about 2 h after the injection. After surgery voles were kept in a cage in 23°C room individually for 2 days, then transferred to the original cage; 10 days were allowed for recovery before experiments began. For Tb and activity recordings, all receivers were connected to a computer using the VitalView software. Data of Tb and activity were collected at 6-min intervals throughout the experiment (Chi and Wang, 2011).
Body Composition
All voles were sacrificed with CO2 asphyxiation between 08:00 and 11:00 at end of the 4 weeks. We weighed (Mettler PE360 to 0.001 g) the wet masses of interscapular brown adipose tissue (iBAT), liver and retroperitoneal and epigonadal white adipose tissues (WAT), gonads and total gut mass with content. After removing the visceral organs and iBAT, the body mass including WAT of above organs was weighed, then dried in an oven until mass was constant and weighed again to determine body dry mass. Total body fat was extracted from the dried carcass with WAT by petroleum ether extraction in a Soxtec apparatus (Avanti 2055, Foss, Sweden).
Statistics
We used the software SPSS 17.0 for statistical analyses. Body mass and food intake were converted to percentage in order to show their patterns during the experimental period. For the statistical analyses data on body mass were arcsine transformed and analyzed by two-way ANOVA (Ta and grouping condition) at each time point and repeated measures.
Group food intake was analyzed by two-way ANCOVA (temperature and grouping condition) with body mass as covariate and repeated measures of ANCOVA. RMR by a group of voles and NSTmax by individual were analyzed by two-way ANCOVA with body mass as covariate. Dry and wet carcass mass, retroperitoneal and epigonadal WATs, total body fat, iBAT, liver, gonad and gut mass with content were analyzed by two-way ANCOVA with body mass as covariate.
Data of core Tb and activity were averaged for each day as well as daytime and nighttime during the experimental period. For statistical analyses, we used only last 2 weeks of data when all voles' physiological acclimation was completed and became stable. Core Tb, activity and wet thermal conductance were analyzed by two-way ANOVA; differences in Tb and activity between daytime and nighttime were determined by t-test. Pearson's correlation was carried out to examine the relationship between core Tb and activity by hourly means of last 4 days. Significant group differences were further evaluated using least significant difference post hoc tests. All values were expressed as mean ± SE and P < 0.05 was considered to be statistically significant.
Results
Body Mass and Food Intake
All groups increased their body mass [repeated measure, F(4, 432) = 29.559, P < 0.001] during the course of experiment, but there was no difference among groups during the entire experiment [by temperature F(1, 108) = 1.179, P = 0.28 and grouping condition F(1, 108) = 0.278, P = 0.599] (Figure 1A).
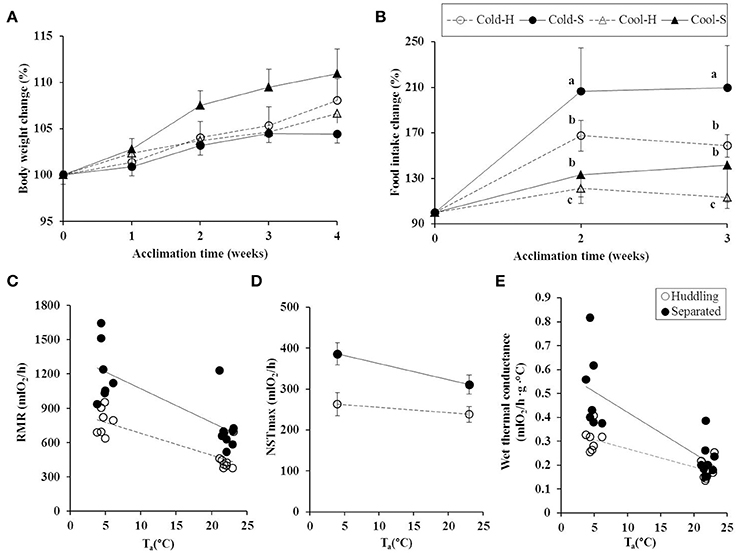
Figure 1. Body mass changes (A), group food intake (B), group RMR (C), NSTmax (D), and group wet thermal conductance (E) in huddling and separated Brandt's voles at cool and cold Tas. (A): Cool-S, cool separated voles (n = 32); Cool-H, cool huddling voles (n = 28); Cold-S, cold separated voles (n = 25) and Cold-H, cold huddling voles (n = 27); (B): Cool-S group (n = 8); Cool-H group (n = 7); Cold-S group (n = 6) and Cold-H group (n = 6); (C): Cold-H group (n = 7); Cold-S group (n = 7); Cool-H group (n = 8) and Cool-S group (n = 9); (D): Cold-H voles (n = 7); Cold-S voles (n = 7); Cool-H voles (n = 8); Cool-S voles (n = 9); (E): Cold-H group (n = 7); Cold-S group (n = 7); Cool-H group (n = 8) and Cool-S group (n = 9). Values are means ± SE. Different small letters indicate significance (P < 0.05).
Prior to experiment the voles from all groups were kept in same Ta (23°C) and could huddle with cagemates. Baseline food intake during this time did not differ among groups [F(3, 22) = 0.275, P = 0.843]. After 2 weeks under experimental conditions the group food intake was significantly affected by Ta [F(1, 22) = 148.180, P < 0.001] and grouping condition [F(1, 22) = 67.892, P < 0.001]. The Cold-S groups showed the highest food intake in comparison to other groups, the Cold-H groups increased food intake to an intermediate level between the Cold-S and cool groups, but was not significantly different from Cool-S group (LSD post hoc test P = 0.509). Repeated measure analysis showed that cold groups increased food intake at the second measure and then kept it stable [Cold-S F(2, 10) = 35.024, P < 0.001; Cold-H F(2, 10) = 35.549, P < 0.001], while cool groups did not show any significant changes over time [Cool-S F(2, 14) = 1.065, P = 0.371; Cool-H F(2, 12) = 2.043, P = 0.172] (Figure 1B). When body mass adjusted food and gross energy intakes of different groups are considered, huddling groups significantly reduced food intake by 13.19 g/day (29%) and 7.61 g/day (26%), and also saved 156.99 kJ/day and 105.19 kJ/day digestible energy per day in cold and cool Tas at last measurement in comparison to separated groups, respectively (Table 1).

Table 1. Body mass adjusted food intake, gross energy intake, digestible energy intake, and digestibility in groups of Brandt's voles under different Ta and grouping conditions.
RMR and NSTmax
At the end of experiment the separated groups had higher group RMR (Cold-S: 1220.28 ± 99.79 ml O2/h; Cool-S: 714.66 ± 68.07 ml O2/h) than huddling groups (Cold-H: 784.33 ± 44.78 ml O2/h; Cool-H: 448.66 ± 36.48 ml O2/h) (grouping condition [F(1, 26) = 22.07, P < 0.001]. The group RMR was increased by low Ta [F(1, 26) = 37.347, P < 0.001], but there was no interaction between Ta and grouping condition on RMR [F(1, 26) = 1.353, P = 0.255]. Huddling groups decreased RMR by 35.7% and 37.2% in cold and cool Tas, respectively (Figure 1C). The RMR of Cold-H group was intermediate between Cold-S and Cool-H groups (LSD P = 0.001), but were not significantly different from Cool-S group (LSD P = 0.433).
Separated voles had higher NSTmax (Cold-S: 386.48 ± 27.07 mlO2/h; Cool-S: 311.58 ± 23.40 mlO2/h) than huddling voles (Cold-H: 263.55 ± 28.55 mlO2/h; Cool-H: 238.06 ± 18.72 mlO2/h) (grouping condition [F(1, 24) = 8.458, P = 0.008]. NSTmax was not changed by Ta [F(1, 24) = 3.815, P = 0.063] or the interaction between Ta and grouping condition [F(1, 24) = 0.928, P = 0.345]. NSTmax in huddling voles was significantly lower by 31% at cold and 23.5% at cool Tas (Figure 1D). Cold-H voles did not increase the NSTmax and maintained it at values similar to the voles in the cool.
Wet Thermal Conductance (C)
Cold-exposed groups either huddling or separated had higher group wet thermal conductance than cool-exposed groups [F(1.27) = 40.989, P < 0.001]. Huddling decreased group wet thermal conductance in cold Ta [F(1.27) = 13.176, P = 0.001], while huddling in cool Ta did not change (Figure 1E). Cold exposure increased heat loss, but Cold-H groups were able to reduce it by 39% to an intermediate level between Cool-H and Cold-S.
Core Tb and Activity
Both daytime and nighttime average Tbs of voles fluctuated over the time of the experiment [F(29.377) = 5.161, P < 0.001] and [F(29.377) = 3.675, P < 0.001, respectively] (Figure 2A).
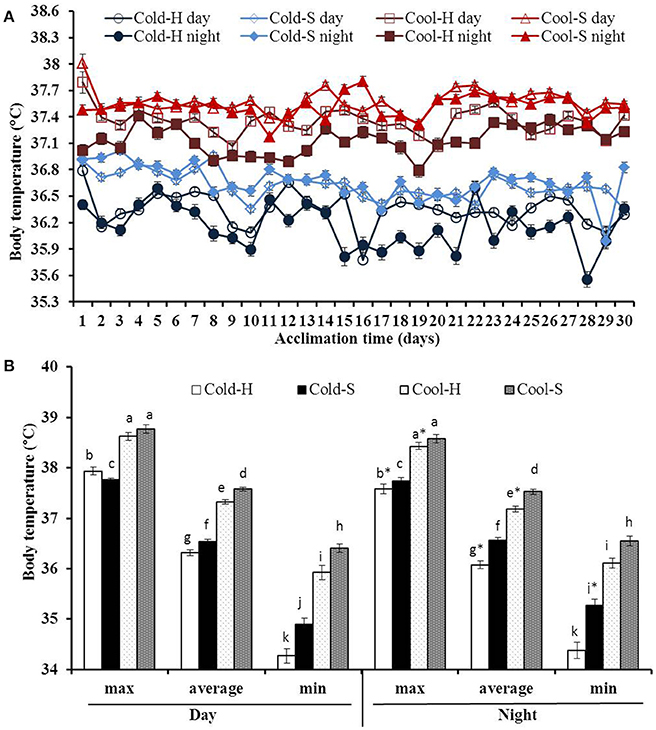
Figure 2. Daytime and nighttime average Tbs during acclimation (A) and differences in maximum, average and minimum Tb (B) of voles from different experimental groups. Cool-H (n = 4); Cool-S (n = 5); Cold-H (n = 4); Cold-S (n = 4). Values are means ± SE. *(star) indicates differences between daytime and nighttime values. Different small letters indicate the significant differences among experimental groups.
Tb was also significantly different among groups for daytime (Cool-S: 37.57 ± 0.04°C; Cool-H: 37.32 ± 0.05°C; Cold-S: 36.53 ± 0.04°C; Cold-H: 36.31 ± 0.06°C) [F(3.55) = 349.908, P < 0.001] and nighttime (Cool-S: 37.52 ± 0.05°C; Cool-H: 37.18 ± 0.06°C; Cold-S: 36.56 ± 0.05°C; Cold-H: 36.06 ± 0.08°C) [F(3.55) = 153.260, P < 0.001]. Huddling voles significantly decreased their nighttime average Tb by 0.14°C (in cool) and 0.25°C (in cold) in comparison to their daytime average Tb values (Cool-H: t = 2.547, P = 0.017, Cold-H: t = 3.292, P = 0.003) while separated voles did not show such differences (Figure 2B).
Maximum Tb of voles in the cool at daytime was higher than that of voles in the cold [F(1.52) = 58.491, P < 0.001) with interaction between Ta and grouping condition [F(1.52) = 11.844, P = 0.001]. At nighttime these values were significantly lowered in most groups except Cold-S voles. Minimum Tb was significantly increased only in Cold-S voles at night while there were no changes in other groups (Figure 2B).
Large fluctuations in daytime average activity were found especially in Cold-H and Cool-H voles with time [F(29.377) = 5.714, P < 0.001] relative to nighttime average activity with time [F(29.377) = 1.745, P < 0.05] (Figure 3A). Cold-H voles decreased their activity during the first week, then increased it to the level of Cool-H voles and had similar pattern until end of experiment (Mean difference = 0.71, P = 0.933) whereas Cold-S voles reduced activity until end of experiment.
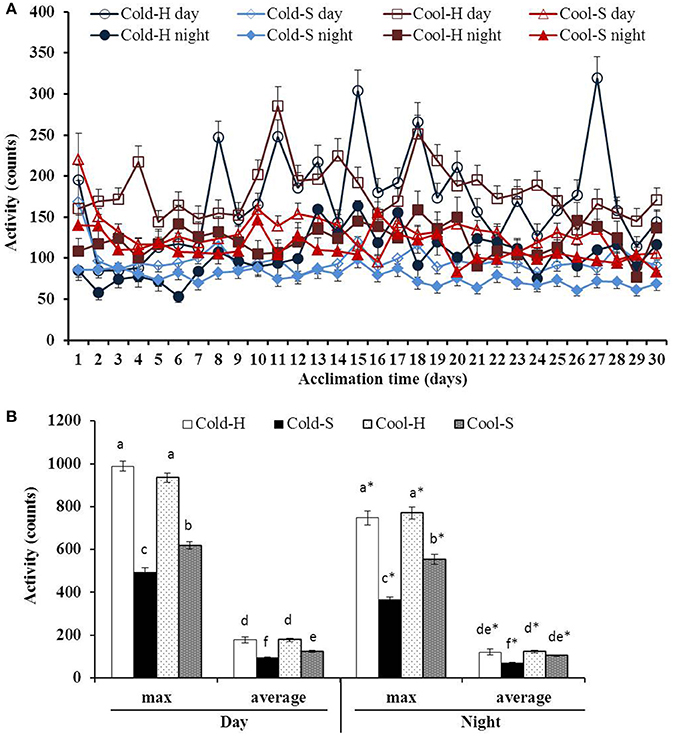
Figure 3. Daytime and nighttime activities during acclimation (A) and differences in maximum and average activity (B) of voles from different experimental groups. Different letters indicate significance among groups; *(star) indicates differences between daytime and nighttime values.
The activity of huddling voles was higher than that of separated voles at both daytime [F(1.52) = 59.912, P < 0.001] and nighttime [F(1.52) = 20.192, P < 0.001]. Daytime average activity of voles in all groups was significantly higher than that of their nighttime activity (Cool-S: t = 3.610, P = 0.001; Cool-H: t = 5.571, P < 0.001; Cold-S: t = 8.048, P < 0.001; Cold-H: t = 2.844, P = 0.009) same as maximum activity (Figure 3B). Cold-H voles increased their activity by 1.7 fold in comparison to Cold-S voles. Pearson correlation on core Tb and activity was positive in all experimental groups (Cool-S: day r = 0.727, P < 0.001, night r = 0.866 P < 0.001; Cool-H: day r = 0.634, P < 0.001, night r = 0.882, P < 0.001; Cold-S: day r = 0.479, P < 0.001, night r = 0.518, P < 0.001; Cold-H: day r = 0.641, P < 0.001, night r = 0.457, P < 0.001).
Body Composition
Cold-exposed voles had higher iBAT mass than cool-exposed voles [F(1, 57) = 6.611, P = 0.013] and separated voles had higher iBAT mass than huddling voles [F(1, 57) = 5.836, P = 0.019]. iBAT mass of Cold-S voles was higher than in voles from other groups [F(3, 61) = 6.810, P = 0.001]. Total gut wet mass of cold-exposed voles was significantly higher than that in cool exposed voles [F(1, 57) = 47.443, P < 0.001], but there was no difference by grouping conditions [F(1, 57) = 2.808, P = 0.099]. Wet liver mass of huddling voles was significantly lower than that in separated voles [F(1, 57) = 9.674, P = 0.003] at cool Ta, whereas such differences was not significant in cold groups. Total body fat of huddling voles was significantly lower than that in separated voles [F(1.57) = 6.618, P = 0.013], but there was no significant difference between cold and cool groups [F(1.57) = 2.689, P = 0.107]. Both wet and dry carcass masses of huddling voles were lower than that in separated voles [wet mass, F(1, 57) = 8.479, P = 0.005; dry mass, F(1, 57) = 7.795, P = 0.007] and cold exposure reduced carcass mass compared with cool condition [wet mass, F(1, 57) = 19.327, P < 0.001 and dry mass, F(1, 57) = 4.053, P = 0.049]. There were no differences in body water content, retroperitoneal and epigonadal WATs, and gonad masses among groups (Table 2).
Discussion
Huddling is social thermoregulatory behavior important to the adaptation to low ambient temperature for reducing heat loss, energy expenditure and maintaining body temperature of animals to survive the cold (Bustamante et al., 2002; Gilbert et al., 2010). Our study shows that huddling substantially affects thermal energetics, thermal conductance, body composition, NSTmax and iBAT mass of voles and results in a reduction of Tb and an increase of activity. These effects of huddling were stronger at low Ta.
Changes in Body Mass, Energy Intake and Body Composition
The voles of all groups increased body mass during the experiment regardless of Ta or grouping condition. Cold exposure increased food intake of cold groups more than cool exposed groups to reduce energetic challenges as for individuals exposed to cold (Zhang and Wang, 2006). Huddling groups reduced food intake by 29% in cold and 26% in cool as compared with separated groups and their digestible energy savings reached to 156.99 and 105.19 kJ/day respectively supporting our prediction. Because the 23°C was below lower critical temperature of thermoneutral zone (TNZ) (27.5°C−32.5°C) of Brandt's voles (Li and Huang, 1994), the Cool-H voles increased their metabolism as the Tb and Ta differential increased. For instance, non-reproductive Mongolian gerbil individuals increased their energy intake with decreased Ta from 50.3 ± 3.7 kJ/day at 30°C (within TNZ 26–38°C) to 70.2 ± 3.9 kJ/day at 21°C and 109.3 ± 6.7 kJ/day at 10°C (Yang et al., 2013). While mice under standard conditions (21°C) display energy expenditure 3.1 times higher basal metabolism (Fischer et al., 2018). For the group housing at TNZ, only few studies focused on mice because of their biomedical research significance (Gordon et al., 1998, 2014; Maher et al., 2015). Both singly and group housed mice preferred relatively warm Tas ~ 29°C during the light phase in temperature gradient tests higher than Ta of 22°C in animal facilities (Gordon et al., 1998). Group of 3 mice housed in the thermocline (floor temperatures 23–39°C) remained at warm end and had significantly smaller livers and kidneys and increase in tail length compared to group of mice in the isothermal runaway (22°C) as well as the cage controls (22°C). But within their TNZ of 30°C the inclusion of cagemates did not influence most physiological parameters in mice (Maher et al., 2015). This is reasonable because both single and group housed mice have thermal comfort at their TNZ. Obviously, when Ta decreases, effect of huddling in energy conservation is apparent. Reduced food intake in huddling groups of Brandt's voles was consistent with previous studies in several small rodent species (Prychodko, 1958; Springer et al., 1981; Kauffman et al., 2003; Nuñez-Villegas et al., 2014) at low Ta. Except for the reduction in iBAT, huddling voles also had lower carcass masses and total fat, but increased the gut masses. Therefore, the decrease in overall carcass mass of huddling voles may be associated with decrease of their metabolic rate. Moreover, huddling remodels gut microbiota to affect host's energy metabolism during cold exposure (Zhang et al., in press).
Changes in RMR, NSTmax and Wet Thermal Conductance
Endotherms living in cold environment maintain normothermic and energy balance through an activation of mechanisms that increase the heat production and conservation (Liu et al., 2009). The metabolic rate and NST for thermoregulation are increased during cold exposure in Brandt's voles (Li et al., 2001; Zhang and Wang, 2006), prairie voles (Microtus ochrogaster) (Wunder et al., 1977) and root voles (Wang et al., 2006). As expected, cold-exposed voles increased RMR. However, the group RMR of huddling groups was lower by 35.7% and 37.2% at 4°C and 23°C of Ta, respectively than in separated groups. The reduction of RMR in huddling group of Brandt's voles was within the range 8–53% of reduction in studies of huddling in many other animals (Gilbert et al., 2010). Furthermore, we importantly found that NSTmax was not increased in Cold-H voles and remained at the same level as for voles from cool groups while it was significantly increased in Cold-S voles. The low NSTmax in Cold-H voles was relative to their lower iBAT masses. It has also been shown that a pair of mice exposed to cold developed less BAT mass than single mice (Heldmaier, 1975) and the thermogenic state of BAT mitochondria and the content of total uncoupling protein were reduced by number of mice in group (Himms-Hagen and Villemure, 1992). Through decrease in exposed body surface area and increase in local heating huddling voles do not need to increase thermogenesis and thus are able to reduce thermoregulatory costs.
With regard to heat loss at low Ta the rate of heat loss in Brandt's voles is increased in comparison to that at 23°C due to high thermal conductance and surface to volume ratio. The group wet thermal conductance of Cold-H group was lower than that of Cold-S group, but higher than in cool groups. Huddling groups reduced their group thermal conductance through reduced surface area and decreased their fur thickness in contact zones and thus transferred heat between each other with less loss to the environment. Interestingly, we observed that some separated voles (not huddling) molted and quickly refreshed the pelage at beginning of the experiment especially around the neck and interscapular area to improve their insulation at cold Ta.
Changes in Core Tb and Activity
Maintenance of high constant Tb is an important feature of mammals and birds. Endothermic animals expend great quantities of energy to regulate and maintain constant internal thermal conditions and functional processes over wide range of Tas (Bennett and Ruben, 1979). Surprisingly and to the contrary our prediction, Cold-H voles had the lowest daytime (36.31 ± 0.06°C) and nighttime (36.06 ± 0.08°C) Tbs than that of other groups. To our knowledge, this decrease of core Tb in huddling Brandt's voles is the first observation especially among small adult homeothermic rodent species. A decrease of Tb has been recorded only in huddling birds. Greater snow goose goslings (Chen caerulescens atlantica) growing in arctic environments lowered Tb by 0.3 ± 0.5°C during huddling (Fortin et al., 2000), and Emperor penguin (Aptenodytes forsteri) decreased Tb by 0.9°C during huddling in the Antarctic winter (Gilbert et al., 2007). In contrast, Tb was increased in huddling deer mice (Peromyscus maniculatus) (Andrews and Belknap, 1986) and townsend voles (Microtus townsendii) (Andrews et al., 1987). Moreover, a recent study on hamsters (Phodopus sungorus) showed that radiant heat exposure by heat lamp (mimic to basking) at low Ta reduced core Tb, metabolic rate and thermal conductance (Geiser et al., 2016). Similarly in our study, huddling decreased Tb and RMR in Brandt's voles in the cold. This Tb decrease is probably explained by the fact that inside huddle bout the voles relaxed endothermic thermoregulation and maintenance of high normothermic Tb setpoint. Thus, the decrease of Tb contributed to reduction of heat loss by decrease in differential between Tb and Ta. It is likely linked to the heat exchange among huddling voles, local surrounding heating (Hayes et al., 1992; Gilbert et al., 2012) and increased local surface Tb at contact zones between animals (Nuñez-Villegas et al., 2014; Bautista et al., 2017).
Interestingly, the activity of Cold-H voles was higher in comparison to Cold-S voles and opposite to our prediction. As Brandt's voles are diurnal animal, daytime Tb and activity of Cold-H voles were higher than at nighttime. Therefore, it seems that this species does not need to reduce the activity to save energy, but keep the activity same as Cool-H voles. Thus, energetic advantages achieved by reduction in RMR, NSTmax and Tb during huddling allowed Cold-H voles to increase their activity. However, Tb and activity were correlated in all groups in present study. The explanation that high activity is to increase Tb is not supported because the huddling voles had higher activity, but lower Tb. The study involving 8 species showed that the temperature rhythm is not a byproduct of the activity rhythm, because Tb during the active phase of the daily cycle was higher than Tb during the inactive phase irrespective of the activity level prevailing during each phase (Refinetti, 1999). Gebczynski and Taylor (2004) have reported that NST plays more important role than activity in shaping of circadian rhythm of Tb. In our study Cold-H voles did not increase the mass of iBAT, but rather increased their activity significantly by 1.7 fold than in Cold-S voles. Girardier et al. (1995) showed that obese rats with atrophied BAT and lean rats with active BAT increased their moving distance by 3.4 fold and 1.4 fold at cold exposure, respectively. Thus activity-associated thermogenesis was the predominant thermogenic source for obese rats whereas such a correlation was not found in lean rats. In addition, balancing the activity-induced increase in Tb with lower Tb minima allows exercised animals in cold environments to benefit from both maintained activity and any energy savings afforded by lower Tbs (Glanville and Seebacher, 2010). Moreover, in the wild the Brandt's voles huddling in winter chamber need to move for feeding to a storage chambers located on the periphery of burrow system. The increased activity in huddling voles may also be related to disturbance of other voles in order to occupy a better position in a huddle.
Conclusion
Our study shows that huddling in Brandt's voles is an important cooperative behavior to reduce metabolic cost for thermoregulation. The pronounced effects of huddling are achieved not only by a decrease in energetics but also involved decreases in NSTmax and iBAT mass, and importantly a decrease of core Tb. The energetic benefits of huddling are likely more extensive than the cost for the increase of activity. Therefore, Brandt's voles can remain active for a longer period and maintain their body condition without increased energetic costs during cold exposure. This study highlights the ecological significance of huddling behavior for maintenance of individual fitness at low costs, and thus survival of population during severe winter in small mammals.
Author Contributions
GS, X-YZ, and D-HW conceived and designed the study. GS and Q-SC conducted the experiment. GS, X-YZ, and Q-SC analyzed the data. GS drafted the manuscript which discussed and improved by X-YZ and D-HW. All authors read and approved the final manuscript.
Funding
This study was supported by grants from the National Natural Scientific Foundation of China (No 31772461, 31770440 and 31470474) to X-YZ and D-HW. GS was supported by CAS-TWAS President's Fellowship Programme.
Conflict of Interest Statement
The authors declare that the research was conducted in the absence of any commercial or financial relationships that could be construed as a potential conflict of interest.
Acknowledgments
We are grateful to the reviewers for valuable comments and suggestions to our manuscript. We thank Professor Fritz Geiser, University of New England, Australia for constructive comments on the original draft. We also thank all members of Animal Physiological Ecology Group, Institute of Zoology of the Chinese Academy of Sciences for valuable suggestion and discussion in the experiment.
References
Andrews, R. V., and Belknap, R. W. (1986). Bioenergetic benefits of huddling by deer mice (Peromyscus maniculatus). Comp. Biochem. Physiol. A. 85, 775–778. doi: 10.1016/0300-9629(86)90294-X
Andrews, R. V., Phillips, D., and Makihara, D. (1987). Metabolic and thermoregulatory consequences of social behaviors between Microtus townsendii. Comp. Biochem. Physiol. A. 87, 345–348. doi: 10.1016/0300-9629(87)90133-2
Batchelder, P., Kinney, R. O., Demlow, L., and Lynch, C. B. (1983). Effects of temperature and social interactions on huddling behavior in Mus musculus. Physiol. Behav. 31, 97–102. doi: 10.1016/0031-9384(83)90102-6
Bautista, A., Zepeda, J. A., Reyes-Meza, V., Feron, C., Rodel, H. G., and Hudson, R. (2017). Body mass modulates huddling dynamics and body temperature profiles in rabbit pups. Physiol. Behav. 179, 184–190. doi: 10.1016/j.physbeh.2017.06.005
Bennett, A. F., and Ruben, J. A. (1979). Endothermy and activity in vertebrates. Science 206, 649–654. doi: 10.1126/science.493968
Boix-Hinzen, C., and Lovegrove, B. G. (1998). Circadian metabolic and thermoregulatory patterns of red-billed woodhoopoes (Phoeniculus purpureus): the influence of huddling. J. Zool. 244, 33–41. doi: 10.1111/j.1469-7998.1998.tb00004.x
Bustamante, D. M., Nespolo, R. F., Rezende, E. L., and Bozinovic, F. (2002). Dynamic thermal balance in the leaf -eared mouse: the interplay among ambient temperature, body size, and behavior. Physiol. Biochem. Zool. 75, 396–404. doi: 10.1086/342253
Canals, M., Rosenmann, M., and Bozinovic, F. (1997). Geometrical aspects of the energetic effectiveness of huddling in small mammals. Acta Theriol. 42, 321–328. doi: 10.4098/AT.arch.97-32
Chi, Q. S., and Wang, D. H. (2011). Thermal physiology and energetics in male desert hamsters (Phodopus roborovskii) during cold acclimation. J. Comp. Physiol. B. 181, 91–103. doi: 10.1007/s00360-010-0506-6
Fischer, A. W., Cannon, B., and Nedergaard, J. (2018). Optimal housing temperatures for mice to mimic the thermal environment of humans: an experimental study. Mol. Metab. 7, 161–170. doi: 10.1016/j.molmet.2017.10.009
Fortin, D., Gauthier, G., and Larochelle, J. (2000). Body temperature and resting behavior of Greater snow goose goslings in the high Arctic. Condor 102, 163–171. doi: 10.1650/0010-5422(2000)102[0163:BTARBO]2.0.CO;2
Gebczynski, A. K., and Taylor, J. R. E. (2004). Daily variation of body temperature, locomotor activity and maximum nonshivering thermogenesis in two species of small rodents. J. Therm. Biol. 29, 123–131. doi: 10.1016/j.jtherbio.2004.01.001
Geiser, F., Gasch, K., Bieber, C., Stalder, G. L., Gerritsmann, H., and Ruf, T. (2016). Basking hamsters reduce resting metabolism, body temperature and energy costs during rewarming from torpor. J. Exp. Biol. 219, 2166–2172. doi: 10.1242/jeb.137828
Gilbert, C., Maho, Y. L., Perret, M., and Ancel, A. (2007). Body temperature changes induced by huddling in breeding male emperor penguins. Am. J. Physiol. Regul. Integr. Comp. Physiol. 292, R176–R185. doi: 10.1152/ajpregu.00912.2005
Gilbert, C., McCafferty, D. J., Giroud, S., Ancel, A., and Blanc, S. (2012). Private heat for public warmth: how huddling shapes individual thermogenic responses of rabbit pups. PLoS ONE 7:e33553. doi: 10.1371/journal.pone.0033553
Gilbert, C., McCafferty, D., Maho, Y. L., Martrette, J. M., Giroud, S., Blanc, S., et al. (2010). One for all and all for one: the energetic benefits of huddling in endotherms. Biol. Rev. Camb. Philos. Soc. 85, 545–569. doi: 10.1111/j.1469-185X.2009.00115.x
Glanville, E. J., and Seebacher, F. (2010). Advantage to lower body temperatures for a small mammal (Rattus fuscipes) experiencing chronic cold. J. Mammal. 91, 1197–1204. doi: 10.1644/10-MAMM-A-003.1
Girardier, L., Clark, M. G., and Seydoux, J. (1995). Thermogenesis associated with spontaneous activity - an important component of thermoregulatory needs in rats. J. Physiol. 488, 779–787. doi: 10.1113/jphysiol.1995.sp021009
Gordon, C. J., Aydin, C., Repasky, E. A., Kokolus, K. M., Dheyongera, G., and Johnstone, A. F. (2014). Behaviorally mediated, warm adaptation: a physiological strategy when mice behaviorally thermoregulate. J. Therm. Biol. 44, 41–46. doi: 10.1016/j.jtherbio.2014.06.006
Gordon, C. J., Becker, P., and Ali, J. S. (1998). Behavioral thermoregulatory responses of single- and group-housed mice. Physiol. Behav. 65, 255–262. doi: 10.1016/S0031-9384(98)00148-6
Grodzinski, W., and Wunder, B. (1975). “Ecological energetics of small mammals,” in Small Mammals: Their Productivity and Population Dynamics, eds F. B. Golley, K. Petrusewicz and L. Ryszkowski (Cambridge: Cambridge University Press), 173–204.
Hayes, J. P., Speakman, J. R., and Racey, P. A. (1992). The contributions of local heating and reducing exposed surface area to the energetic benefits of huddling by short-tailed field voles (Microtus agrestis). Physiol. Zool. 65, 742–762. doi: 10.1086/physzool.65.4.30158537
Heldmaier, G. (1975). The influence of the social thermoregulation on the cold-adaptive growth of BAT in hairless and furred mice. Pflugers Arch. 355, 261–266. doi: 10.1007/BF00583688
Himms-Hagen, J., and Villemure, C. (1992). Number of mice per cage influences uncoupling protein content of brown adipose tissue. Exp. Biol. Med. 200, 502–506. doi: 10.3181/00379727-200-43461
Kauffman, A. S., Paul, M. J., Butler, M. P., and Zucker, I. (2003). Huddling, locomotor, and nest-building behaviors of furred and furless Siberian hamsters. Physiol. Behav. 79, 247–256. doi: 10.1016/S0031-9384(03)00115-X
Kotze, J., Bennett, N. C., and Scantlebury, M. (2008). The energetics of huddling in two species of mole-rat (Rodentia: Bathyergidae). Physiol. Behav. 93, 215–221. doi: 10.1016/j.physbeh.2007.08.016
Li, Q. F., and Huang, C. X. (1994). Characteristics of the resting metabolic rate of Brandt's voles (Microtus brandtii). Acta Theriol Sin. 14, 217–220.
Li, Q., Sun, R., Huang, C., Wang, Z., Liu, X., Hou, J., et al. (2001). Cold adaptive thermogenesis in small mammals from different geographical zones of China. Comp. Biochem. Physiol. A. 129, 949–961. doi: 10.1016/S1095-6433(01)00357-9
Li, X. S., and Wang, D. H. (2005). Regulation of body weight and thermogenesis in seasonally acclimatized Brandt's voles (Microtus brandti). Horm. Behav. 48, 321–328. doi: 10.1016/j.yhbeh.2005.04.004
Liu, H., Wang, D. H., and Wang, Z. W. (2003). Energy requirements during reproduction in female Brandt's voles (Microtus brandtii). J. Mammal. 84, 1410–1416. doi: 10.1644/BRG-030
Liu, J. S., Yang, M., Sun, R. Y., and Wang, D. H. (2009). Adaptive thermogenesis in Brandt's vole (Lasiopodomys brandtii) during cold and warm acclimation. J. Therm. Biol. 34, 60–69. doi: 10.1016/j.jtherbio.2008.11.001
Maher, R. L., Barbash, S. M., Lynch, D. V., and Swoap, S. J. (2015). Group housing and nest building only slightly ameliorate the cold stress of typical housing in female C57BL/6J mice. Am. J. Physiol. Regul. Integr. Comp. Physiol. 308, R1070–R1079. doi: 10.1152/ajpregu.00407.2014
McNab, B. K. (1980). On estimating thermal conductance in endotherms. Physiol. Zool. 53, 145–156. doi: 10.1086/physzool.53.2.30152577
Nieminen, P., Hohtola, E., and Mustonen, A. M. (2013). Body temperature rhythms in Microtus voles during feeding, food deprivation, and winter acclimatization. J. Mammal. 94, 591–600. doi: 10.1644/12-MAMM-A-219.1
Nowack, J., and Geiser, F. (2016). Friends with benefits: the role of huddling in mixed groups of torpid and normothermic animals. J. Exp. Biol. 219, 590–596. doi: 10.1242/jeb.128926
Nuñez-Villegas, M., Bozinovic, F., and Sabat, P. (2014). Interplay between group size, huddling behavior and basal metabolism: an experimental approach in the social degu. J. Exp. Biol. 217, 997–1002. doi: 10.1242/jeb.096164
Prychodko, W. (1958). Effect of aggregation of laboratory mice (Mus musculus) on food intake at different temperatures. Ecology 39, 500–503. doi: 10.2307/1931760
Putaala, A., Hohtola, E., and Hissa, R. (1995). The effect of group size on metabolism in huddling grey partridge (Perdix perdix). Comp. Biochem. Physiol. B. 111, 243–247. doi: 10.1016/0305-0491(94)00246-Q
Refinetti, R. (1999). Relationship between the daily rhythms of locomotor activity and body temperature in eight mammalian species. Am. J. Physiol. Regul. Integr. Comp. Physiol. 277, R1493–R1500. doi: 10.1152/ajpregu.1999.277.5.R1493
Scantlebury, M., Bennett, N. C., Speakman, J. R., Pillay, N., and Schradin, C. (2006). Huddling in groups leads to daily energy savings in free-living African four-striped grass mice (Rhabdomys pumilio). Funct. Ecol. 20, 166–173. doi: 10.1111/j.1365-2435.2006.01074.x
Scholander, P. F., Hock, R., Walters, V., Johnson, F., and Irving, L. (1950). Heat regulation in some arctic and tropical mammals and birds. Biol. Bull. 99, 237–258. doi: 10.2307/1538741
Sealander, J. A. (1952). The relationship of nest protection and huddling to survival of Peromyscus at low temperature. Ecology 33, 63–71. doi: 10.2307/1931252
Springer, S. D., Gregory, P. A., and Barrett, G. W. (1981). Importance of social grouping on bioenergetics of the Golden mouse, Ochrotomys nuttall. J. Mammal. 62, 628–630. doi: 10.2307/1380411
Tang, G. B., Cui, J. C., and Wang, D. H. (2009). Role of hypoleptinemia during cold adaptation in Brandt's voles (Lasiopodomys brandtii). Am. J. Physiol. Regul. Integr. Comp. Physiol. 297, R1293–R1301. doi: 10.1152/ajpregu.00185.2009
Vickery, W. L., and Millar, J. S. (1984). The energetics of huddling by endotherms. Oikos 43, 88–93. doi: 10.2307/3544249
Wang, J. M., Zhang, Y. M., and Wang, D. H. (2006). Seasonal regulations of energetics, serum concentrations of leptin, and uncoupling protein 1 content of brown adipose tissue in root voles (Microtus oeconomus) from the Qinghai-Tibetan plateau. J. Comp. Physiol. B Biochem. Syst. Environ. Physiol. 176, 663–671. doi: 10.1007/s00360-006-0089-4
Wang, J., and Wang, D. (2006). Comparison of nonshivering thermogenesis induced by dosages of norepinephrine from 3 allometric equations in Brandt's voles (Lasiopodomys brandtii). Acta Theriol Sin. 26, 84–88. doi: 10.3969/j.issn.1000-1050.2006.01.015
Wolff, J. O., and Lidicker, W. Z. (1981). Communal winter nesting and food sharing in Taiga voles. Behav. Ecol. Sociobiol. 9, 237–240. doi: 10.1007/BF00299877
Wunder, B. A., Dobkin, D. S., and Gettinger, R. D. (1977). Shifts of thermogenesis in the prairie vole (Microtus ochrogaster). Oecologia 29, 11–26. doi: 10.1007/BF00345359
Yang, D. B., Li, L., Wang, L. P., Chi, Q. S., Hambly, C., Wang, D. H., et al. (2013). Limits to sustained energy intake. XIX. A test of the heat dissipation limitation hypothesis in Mongolian gerbils (Meriones unguiculatus). J. Exp. Biol. 216, 3358–3368. doi: 10.1242/jeb.085233
Zhang, Q., Lin, Y., Zhang, X. Y., and Wang, D. H. (2015). Cold exposure inhibits hypothalamic kiss-1 gene expression, serum leptin concentration, and delays reproductive development in male Brandt's vole (Lasiopodomys brandtii). Int. J. Biometeorol. 59, 679–691. doi: 10.1007/s00484-014-0879-4
Zhang, X. Y., Sukhchuluun, G., Bo, T. B., Chi, Q. S., Yang, J. J., Chen, B. (in press). Huddling remodels gut microbiota to reduce energy requirements in a small mammal species during cold exposure. Microbiome. doi: 10.1186/s40168-018-0473-9, et al.
Keywords: huddling, Brandt's voles, energetics, thermogenesis, core body temperature, activity
Citation: Sukhchuluun G, Zhang X-Y, Chi Q-S and Wang D-H (2018) Huddling Conserves Energy, Decreases Core Body Temperature, but Increases Activity in Brandt's Voles (Lasiopodomys brandtii). Front. Physiol. 9:563. doi: 10.3389/fphys.2018.00563
Received: 11 November 2017; Accepted: 30 April 2018;
Published: 18 May 2018.
Edited by:
David C. Randall, University of Kentucky College of Medicine, United StatesReviewed by:
James Todd Pearson, National Cerebral and Cardiovascular Center, JapanChristopher J. Madden, Oregon Health & Science University, United States
Copyright © 2018 Sukhchuluun, Zhang, Chi and Wang. This is an open-access article distributed under the terms of the Creative Commons Attribution License (CC BY). The use, distribution or reproduction in other forums is permitted, provided the original author(s) and the copyright owner are credited and that the original publication in this journal is cited, in accordance with accepted academic practice. No use, distribution or reproduction is permitted which does not comply with these terms.
*Correspondence: Xue-Ying Zhang, emhhbmd4eUBpb3ouYWMuY24=
De-Hua Wang, d2FuZ2RoQGlvei5hYy5jbg==