- Department of Endocrinology and Metabolism, China National Research Center for Metabolic Diseases, Ruijin Hospital, Shanghai Jiao Tong University School of Medicine, Shanghai, China
Obesity has become epidemic worldwide, which triggers several obesity-associated complications. Obesity is characterized by excess fat storage mainly in the visceral white adipose tissue (vWAT), subcutaneous WAT (sWAT), and other tissues. Myriad studies have demonstrated the crucial role of canonical Wnt/β-catenin cascade in the development of organs and physiological homeostasis, whereas recent studies show that genetic variations/mutations in the Wnt/β-catenin pathway are associated with human metabolic diseases. In this review, we highlight the regulation of updated Wnt/β-catenin signaling in obesity, especially the distinctly depot-specific roles between subcutaneous and visceral adipose tissue under high-fed diet stimulation and WAT browning process.
Introduction
Worldwide obesity has more than doubled since 1980; 39% of adults aged 18 years and older were overweight in 2016, and 13% were obese throughout the world (World Health Organisation [WHO], 2017). The main reasons for the continuous rise in obesity seem to be well-known, increased food intake and decreased exercise, which result in energy consumption that exceeds energy expenditure and predispose the body to more fat accumulation.
Wnt/β-catenin signaling controls several developmental processes in early stages of life and the homeostasis of mature tissues via regulating cell proliferation (Alonso and Fuchs, 2003), differentiation (Hu et al., 2016), and genetic stability (Alberici and Fodde, 2006; Rawson et al., 2011; Hoffmeyer et al., 2012), as well as via maintenance of adult precursor cells in a pluripotent state (Sato et al., 2009). Aberration or mutation in this signaling is associated with a wide range of diseases in humans, including cancer (Porfiri et al., 1997; de La Coste et al., 1998; Anastas and Moon, 2013), osteoporosis (Gong et al., 2001; Little et al., 2002b; Loh et al., 2015), neurodegeneration (Okerlund and Cheyette, 2011; Berwick and Harvey, 2012; Inestrosa et al., 2012) and cardiometabolic disorders (Schinner, 2009). The significance of aberrant Wnt/β-catenin signaling has led to substantial efforts in the future development of therapeutic methods in metabolic disorders, including obesity. However, this field is much rough due to the enormously complex structure and a multitude of new factors involved in this pathway.
White adipose tissues (WATs) are distributed almost in the entire body and more biologically relevant with obesity. However, brown adipose tissues (BATs) principally function in the neonatal period to combat extrauterine coldness, and recent research has shown that adult humans also possess functional BAT and its mass shows a negative correlation with adiposity and body mass index (BMI) (Cypess et al., 2009, 2013; Saito et al., 2009; van Marken Lichtenbelt et al., 2009; Lidell et al., 2013; Orava et al., 2013; Chondronikola et al., 2014). Several studies have demonstrated the crucial role of Wnt/β-catenin signaling in the development of WAT and BAT, thus targeting Wnt/β-catenin pathway to combat obesity is promising.
Here we systematically reviewed literature about Wnt/β-catenin signaling and obesity, and its precise role in the browning process of depot-specific white adipocytes, to provide information about therapeutic approaches for different subgroups of the obese population.
Association Between Obesity and Different Adipose Depots
Overview of Obesity and Adipose Tissues
The prevalence of chronic diseases, including cancer, diabetes, and cardiovascular diseases, has been markedly increasing almost worldwide. Reducing obesity should be given priority over prevention and treatment of these chronic diseases (Heymsfield et al., 2017). The obesity crisis has led to focus on adipose tissue, which is composed mainly of adipocytes and mesenchymal cells. White and brown adipocytes in mammals have different function, morphology, and molecular features. White adipocytes are designed for energy storage in the form of fatty acids, whereas the brown adipocytes burn substrates, including fatty acids and glucose, dissipate energy, and generate heat through the process of adaptive non-shivering thermogenesis in response to various stimuli (Wang and Seale, 2016). WAT is highly plastic and dynamic, accounting for 5% to approximately 60% of body weight in different individuals, and it can change in mass in the same individual (Guo et al., 1999; Droyvold et al., 2006). Although excessive accumulation of WAT is the key feature of adiposity, obesity is clinically defined by a BMI over 30 kg/m2, which does not take fat content into account (World Health Organisation [WHO], 2017). Waist circumference is another index to define obesity, with a cutoff in Caucasians of 85 cm for females and 90 cm for males and in Asians 80 cm for females and 85 cm for males, which could also not distinct sWAT and vWAT proportion despite the metabolic discrepancy between them. Waist-to-hip ratio adjusted for BMI provides a surrogate measure of abdominal adiposity, which can partly reveal the association of vWAT and metabolic diseases (Emdin et al., 2017). WAT can expand via both hyperplasia and hypertrophy, and recent studies suggest that adipocyte hyperplasia is of importance to human obesity in addition to the well-known hypertrophy theory (Spalding et al., 2008; Arner et al., 2013). However, intrinsic mechanisms that lead to the increase in fat mass in response to positive energy imbalance are unknown; some details will be discussed herein.
Differences Between sWAT and vWAT Depots
Adipose tissue comprises various discrete depots, such as inguinal, interscapular, perigonadal, retroperitoneal, and mesenteric depots, which are placed in defined positions throughout the body. There are two major divisions of WAT, vWAT, and sWAT (Cinti, 2005). vWAT and sWAT depots are simply separated based on gross anatomical location, inside or outside of the abdominal cavity. However, this gross classification indicates a variety of crucial features, including developmental lineage (Chau et al., 2014; Krueger et al., 2014; Sanchez-Gurmaches and Guertin, 2014), gene expression (Grove et al., 2010; Karastergiou and Fried, 2013; Cohen et al., 2014), adipokine secretion profiles (Shi et al., 2009), microenviroments (Jeffery et al., 2016), and metabolic characteristics (Tran et al., 2008; Karastergiou and Fried, 2013; Cohen et al., 2014).
The current prevailing view shows that sWAT and vWAT depots have different contributions to cardiometabolic disease (Emdin et al., 2017). In humans, high gluteofemoral sWAT content, which is more pervasive in premenopausal females, called “pear-shaped”, might protect against certain aspects of metabolic dysfunction (Snijder et al., 2003; Tanko et al., 2003; Manolopoulos et al., 2010; Palmer and Clegg, 2015). Conversely, high vWAT and deep abdominal sWAT depots, also known as “apple-shaped”, are thought to be correlated with increased risk of hyperlipidemia, diabetes, and cardiovascular disease (Kelley et al., 2000; Wang et al., 2005; Nicklas et al., 2006). In rodents, inguinal (posterior) sWAT is correlated with improved metabolism (Tran et al., 2008), similar to human gluteofemoral sWAT, whereas mouse perigonadal vWAT is correlated with insulin resistance (Foster et al., 2010). Most studies have demonstrated that an increased vWAT content leads to a marked degree of inflammation with macrophage accumulation (Weisberg et al., 2003), as well as adversely reduced expression of genes associated with adipogenesis and metabolism in vWAT (Nadler et al., 2000; Voigt et al., 2013; Choi et al., 2015). Hence, it has become prevalent to term sWAT depot as “good fat” and vWAT depot as “bad fat” (Berry et al., 2014). The factors that contribute to this discrepancy are still unknown.
In mice, sWAT primitive organs are formed by cells expressing adipogenic markers in comparatively late embryogenesis, and there is no obvious lipid loading of white adipocytes until birth (Birsoy et al., 2011). The visceral perigonadal WAT (pWAT) depot becomes populated after birth, with the first determined adipocyte precursors appearing at postnatal day 4 (P4) and lipid fulling in developing pWAT overt at P7 (Han et al., 2011). Moreover, recent research shows that six vWAT depots arise mainly from Wt1-expressing cells late in gestation but no sWAT or BAT from Wt1-expressing cells; these results indicate a major ontogenetic difference between vWAT and sWAT (Chau et al., 2014).
Recent progress in the identification and isolation of adipocyte precursor cells (APs, in adipose tissue, APs consist of adipocyte progenitors and preadipocytes) in vivo allows for direct investigation of cellular and molecular mechanisms (Rodeheffer et al., 2008; Tang et al., 2008; Lee et al., 2012; Berry and Rodeheffer, 2013; Jiang et al., 2014; Vishvanath et al., 2016). Increasing evidence suggests that APs derived from different WAT depots are distinct populations and differ in their inherent properties (Tchkonia et al., 2005, 2006; Macotela et al., 2012). The functional difference of these APs depots depends on cellular circulation, innervation, and anatomic constraints contributing to pathophysiological variation of depot-specific related metabolic homeostasis (Tchkonia et al., 2007, 2013). Of note, Tchkonia and colleagues suppose that differences between sWAT APs (S-APs) and vWAT APs (V-APs) are at least partly cell autonomous via in vitro APs isolated experiments (Tchkonia et al., 2013). However, a recent report indicates that instead of cell-intrinsic mechanisms, the activation of APs is regulated by the adipose depot-specific microenvironment in vivo (Jeffery et al., 2016). This finding highlights that APs are plastic, and both local and systemic signals could influence their differentiation potential dependent or independent of depot origin.
A variety of advances highlight the notion that definable and distinct developmental signaling cascades, including Wnt pathway, might underlie the developmental and functional discrepancy between sWAT and vWAT. Wnt signaling is critically important for health and disease, which also regulates adipose tissue in a depot-specific manner (Longo et al., 2004; Zeve et al., 2012). Therefore, understanding how depot-specific WAT mass is developmentally and functionally regulated by Wnt/β-catenin pathway in vivo is required.
Overview of Wnt/β-Catenin Signaling Cascade
Development and Structure of Canonical Wnt/β-Catenin Signaling
The Wnt/β-catenin signaling (often referred to as “canonical” signaling) was first identified in 1982 via the discovery of the Wnt1 gene (originally named Int1) in mice (Nusse and Varmus, 1982). Then the fly Wingless (wg) gene, a homolog of Wnt1, was cloned and shown that it controls proper wing formation (Baker, 1987). Epistasis experiments delineated the significance of this developmental signaling in Drosophila (Siegfried et al., 1992; Noordermeer et al., 1994; Peifer et al., 1994), and injection of mouse Int1 into early frog embryos caused body axis duplication in Xenopus (McMahon and Moon, 1989), both providing the highly conserved nature of the signaling, generally referred to as the canonical Wnt/β-catenin signaling. By 1996, major gaps in Wnt/β-catenin signaling were occluded with the identification of Wnt nuclear effectors, TCF/lymphoid enhancer factor (LEF) transcription factors (Behrens et al., 1996; Molenaar et al., 1996), and Wnt receptors, Frizzleds (Bhanot et al., 1996), which function with lipoprotein receptor-related proteins (LRPs)/Arrow as coreceptors (Wehrli et al., 2000).
It is worth noting that the Wnt/β-catenin signaling is different from most other pathways. Here, we discuss the key structure of this signaling cascade via referring to several recent reviews for more details (Clevers and Nusse, 2012; Niehrs, 2012; Nusse and Varmus, 2012; Kahn, 2014; Jiang and Cong, 2016). Wnt proteins (Wnts) are cysteine-rich, secreted glycoproteins, about 40 kDa in size; they predominantly act as close-range ligands in a concentration-dependent manner to activate receptor-mediated signaling, for instance, in adult stem cell niches (Sato et al., 2011; Strand and Micchelli, 2011; Clevers and Nusse, 2012). The hallmark of this signaling pathway is that it regulates the transcriptional activity of the cofactor β-catenin (Ctnnb1), which is the core mediator of canonical Wnt/β-catenin signaling. β-catenin localizes to various subcellular sites dynamically, including adherens junctions, the cytoplasm, and the nucleus, contributing to cell–cell contacts, transcriptional regulation, and chromatin modifications (Fagotto, 2013; Miller et al., 2013). Wnts are the key extracellular regulators of β-catenin stabilization; however, there are several factors that influence β-catenin dynamics, including hypoxia, hepatocyte growth factor, prostaglandins, E-cadherin, and protein kinase A (Brembeck et al., 2006; Kawabata, 2011; van Veelen et al., 2011). Wnts combine with low-density lipoprotein receptor-related protein 5 (LRP5) or LRP6 and Frizzled (FZD) seven-transmembrane domain receptors as Wnt-FZD-LRP5/6 complex, the cytoplasmic part of FZD interacts with Disheveled (DVL) (Chen et al., 2003), tightly regulates the activity of β-catenin cytoplasmic pool by phosphorylation through inhibiting the “destruction complex”, consisting of casein kinase 1α (CK1α), glycogen synthase kinase 3β (GSK3β), tumor suppressor adenomatous polyposis coli (APC), the scaffold protein AXIN, and others (Gumbiner, 1997). Then β-catenin enters the nucleus, turns on transcription via binding to members of the lymphoid enhancer factor (TCF/LEF) family of transcription factors (Figure 1). In addition, β-catenin can bind to other transcription factors, thereby modulating many downstream biological processes including epithelial to mesenchymal transition, pluripotency, and melanocyte development (Le and Fodde, 2008). In the absence of Wnts, phosphorylation plays the dominant role in cytoplasmic β-catenin for its ubiquitination and proteasomal degradation (Rubinfeld et al., 1996). It is now clear there exists non-canonical Wnt signaling crosstalk with the canonical Wnt/β-catenin signaling to modulate nuclear β-catenin accumulation (Coluccia et al., 2007; Rasola et al., 2007); this review does not go into great detail about this.
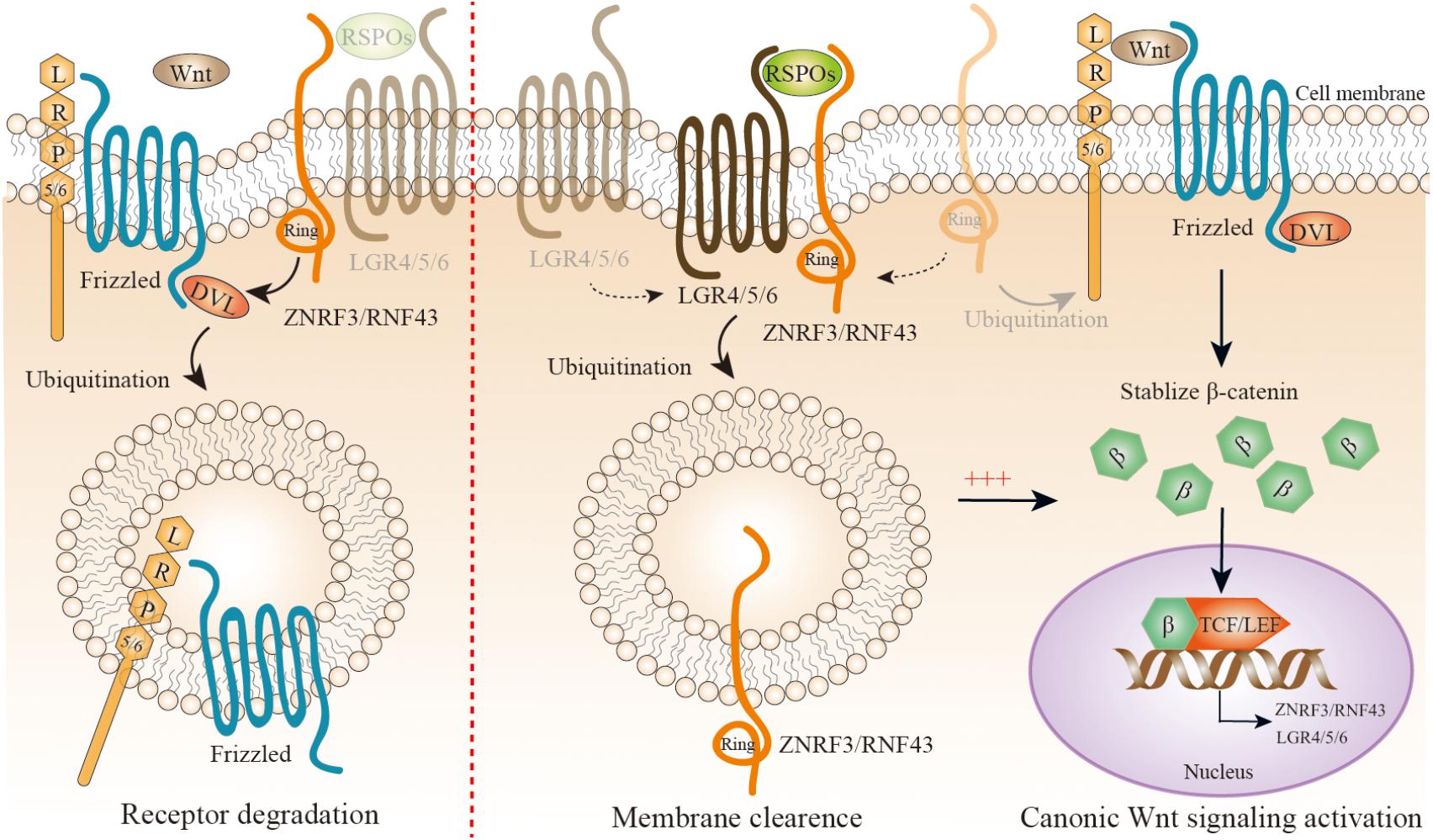
FIGURE 1. A simplified representation of precise modulation of canonic Wnt/β-catenin signaling. Wnt proteins combine with low-density lipoprotein receptor-related protein (LRP) 5 or 6 coreceptor and Frizzled (FZD) seven transmembrane domain receptors as Wnt-FZD-LRP5/6 complex; the cytoplasmic part of FZD interacts with Disheveled (DVL), and tightly regulates the activity of the β-catenin cytoplasmic pool by inhibiting its phosphorylation. Then β-catenin enters the nucleus, and turns on transcription via binding to members of the lymphoid enhancer factor (TCF/LEF) family of transcription factors. ZNRF3/RNF43 is a negative feedback regulator of Wnt/β-catenin signaling. With a lack of R-spondin proteins (RSPOs), ZNRF3/RNF43 is recruited by DVL to Wnt-FZD-LRP5/6 complex to mediate their degradation (left panel). When RSPOs are present, they simultaneously combine with the extracellular regions of both ZNRF3/RNF43 and LGR4/5/6, leading to autoubiquitination, endocytosis, and membrane clearance of ZNRF3/RNF43. Therefore, RSPOs sensitizes cells to Wnt/β-catenin signaling by inhibiting ZNRF3/RNF43, resulting in increased Wnt-FZD-LRP5/6 complex availability at the cell surface (right panel). Interestingly, both ZNRF3/RNF43 and LGR4/5/6 could be the downstream targets of Wnt/β-catenin signaling.
Regulation of Wnt-FZD-LRP5/6 Complex by RSPOs–LGR4/5/6–ZNRF3/RNF43 Complex
R-spondin proteins (RSPOs), including RSPO1-4 in mammals, are secreted proteins that amplify canonical Wnt/β-catenin signaling, but not by themselves – they only sensitize cells to Wnt/β-catenin signaling. RSPOs have a similar structure consisting of one C-terminal TSR-1 domain and two N-terminal furin repeats. The two furin repeats are pivotal for potentiating Wnt/β-catenin signaling (Kazanskaya et al., 2004; Kim et al., 2008; Li et al., 2009; Glinka et al., 2011). The TSR-1 domain might keep RSPOs close to the cell surface. LGR4/5/6 is a newly identified receptor for the RSPOs with high affinity (Carmon et al., 2011; de Lau et al., 2011; Glinka et al., 2011; Gong et al., 2012; Leushacke and Barker, 2012; Ruffner et al., 2012), and belongs to the rhodopsin G protein-coupled receptor (GPCR) family. LGRs are composed of a large 17 leucine-rich repeats extracellular domain, a hinge region, a seven-transmembrane field, and a short intracellular tail. RSPOs physically interact with LGR4/5/6, they do not activate canonical GPCR signaling (Carmon et al., 2011; de Lau et al., 2011; Ruffner et al., 2012). RSPOs–LGR4/5/6 complex is essential for amplifying Wnt/β-catenin signaling, but the way to activate the complex was unclear until the recent identification of two highly functional homologous proteins, Zinc and ring finger 3 (ZNRF3) and ring finger protein 43 (RNF43), which are the negative feedback regulators of Wnt/β-catenin signaling (Figure 2) (Hao et al., 2012; Koo et al., 2012). ZNRF3 and RNF43 are E3 ubiquitin ligases located on the cell surface. Their basic structure and sequence are related to the Goliath family of RING domain E3 ligases, with an extracellular protease-associated domain, a single-pass transmembrane domain, and an intracellular RING domain. ZNRF3/RNF43 inhibits Wnt/β-catenin signaling by specifically mediating the ubiquitination of the Wnt receptor FZD and coreceptor LRP6, which results in the endocytosis of Wnt receptors and their subsequent degradation. How ZNRF3/RNF43 recognizes FZD is still unknown now, and it seems that the intracellular domain of ZNRF3/RNF43 is involved in the action. Recent reports suggest that DVL is mediated as an adaptor protein combining ZNRF3/RNF43 with FZD (Jiang et al., 2015). ZNRF3/RNF43 physically interacting with DVL is required for Wnt/β-catenin signaling pathway inhibition by two proteins. Interestingly, ZNRF3/RNF43 as well as LGR4/5/6 are the downstream targets of Wnt/β-catenin, which forms an automatically negative and positive feedback loop (Jiang and Cong, 2016).
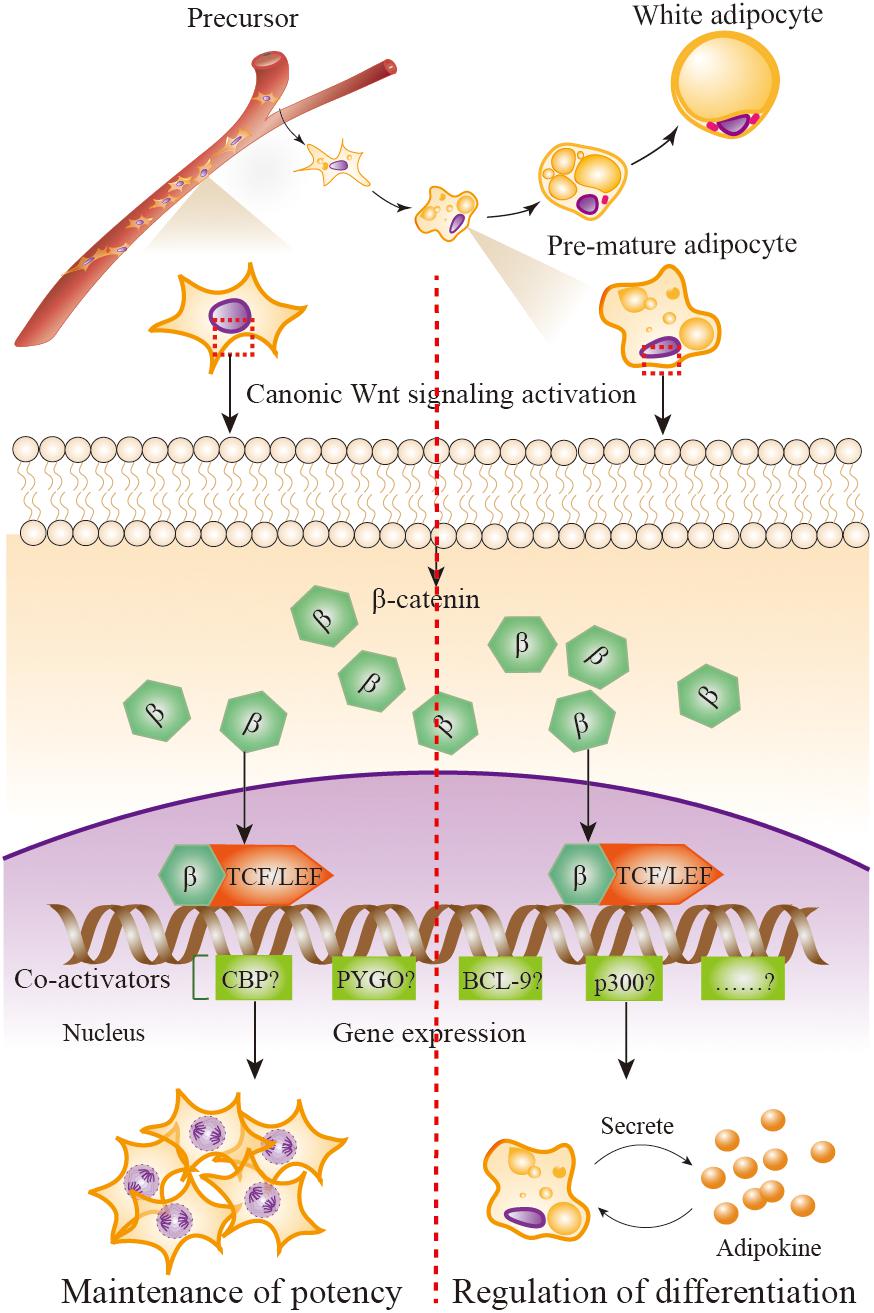
FIGURE 2. Wnt/β-catenin signaling in adipose precursors and pre-mature/mature adipocytes. When Wnt/β-catenin signaling is activated, β-catenin enters the nucleus and it turns on transcription via binding to TCF/LEF family of transcription factors. β-catenin–TCF/LEF complex is needed to recruit transcriptional co-activator to be functional, including the CREB-binding protein (CBP) or its closely associated homolog E1A associated protein p300, B cell lymphoma 9 (BCL-9), the co-activator Pygopus (PYGO), etc. Wnt/β-catenin signaling activation in adipose precursor cells leads β-catenin to combine with co-activators CBP, resulting in precursor proliferation and potency maintenance; in pre-mature/mature adipocytes, β-catenin combines with co-activator p300, resulting in the initiation of differentiation.
The discovery of ZNRF3/RNF43 gives a potential explanation to the molecular mechanisms of RSPOs–LGR4/5/6 complex-induced Wnt/β-catenin signaling (Figure 1). An initial clue to the role ZNRF3/RNF43 plays in RSPOs–LGR4/5/6 complex is that the upregulation of FZDs in cell-surface, a typical RSPOs-induced event, can be represented when ZNRF3/RNF43 lost function. This hypothesis is further supported by cell biology and biochemical experiments, in which RSPOs simultaneously combine with the extracellular regions of ZNRF3/RNF43 and/or LGR4/5/6, leading to a self-ubiquitination and endocytosis, sensitizing cells to Wnt/β-catenin signaling by increasing Wnt-FZD-LRP5/6 complex availability at the cell surface (Hao et al., 2012; Szenker-Ravi et al., 2018). This conclusion is consistent with extensive researches focusing on the RSPOs–LGR4/5/6–ZNRF3/RNF43 complex (Chen et al., 2013; Peng et al., 2013; Wang D. et al., 2013; Xie et al., 2013; de Lau et al., 2014; Zebisch and Jones, 2015). Importantly, single amino acids replacement in RSPOs completely halts the Wnts stimulatory activity of RSPOs by destructing either the RSPOs–LGR4/5/6 or RSPOs–ZNRF3/RNF43 interaction, suggesting that RSPOs need to function through interacting with both LGR4/5/6 and ZNRF3/RNF43 (Hao et al., 2012). In the complex, LGR4/5/6 works as the engagement receptor for RSPOs and ZNRF3/RNF43 works as the effector receptor. Why LGR4/5/6 are importantly required for ZNRF3/RNF43 interaction with RSPOs is not completely identified currently. It is possible that LGR4/5/6 is required for transferring RSPOs to ZNRF3/RNF43 to enhance the affinity between them (Jiang and Cong, 2016).
Target Wnt/β-Catenin Signaling in Obesity
Wnt/β-Catenin Signals Associate With Metabolic Disorders
During the past decade, the field involving the link between Wnt/β-catenin signaling and metabolic dysfunction is rapidly developing. The crucial role of Wnt/β-catenin signaling factors has been gradually identified by genetic and biological studies in several metabolic disorders. The first association between the Wnt/β-catenin pathway and metabolic disease came about in 2004, in which some specific SNPs in β-catenin-independent WNT5B increased susceptibility for type 2 diabetes (Kanazawa et al., 2004), initiating the discoveries of WNT10B (Christodoulides et al., 2006) and TCF7L2 (Grant et al., 2006; Tong et al., 2009; Savic et al., 2011; Takamoto et al., 2014). More than 80 signals have been found to be associated with the risk of type 2 diabetes by genome-wide association studies (Voight et al., 2010; Cho et al., 2011; Kooner et al., 2011; Morris et al., 2012; Ma et al., 2013; Mahajan et al., 2014; Steinthorsdottir et al., 2014). Among these risk loci, TCF7L2 seems to be one of the strongest risk loci [P = 2.1 × 10-9] for type 2 diabetes (Grant et al., 2006). Grant and his group have observed that heterozygous and homozygous carriers of DG10S478, within intron 3 of TCF7L2, have a higher risk with odds ratio (OR) of 1.45 and 2.41, respectively, compared with non-carriers. A recent study using genetically engineered mice reveals that TCF7L2 might be involved in glucose metabolism through regulating insulin secretion of the pancreatic beta cell mass (Takamoto et al., 2014). The direct association of TCF7L2 with obesity is unclear; however, it is well-established that TCF7L2 is expressed in adipose tissue (Cauchi et al., 2006, 2008; Kovacs et al., 2008) and involved in Wnt/β-catenin signaling dependent regulation of adipogenesis (Ross et al., 2000, 2002; Cawthorn et al., 2007). Recent research observes that TCF7L2 gene variation is associated with less weight loss during lifestyle intervention (Haupt et al., 2010) whereas Josiemer and colleagues support that TCF7L2 genetic variants may reduce body adiposity and further induce better glycemic control (Mattei et al., 2012). The specific mechanism between TCF7L2 and obesity development remains a mystery (Chen et al., 2018).
LRP5/6, is FZD coreceptor in Wnt/β-catenin signaling, the patients carrying mutations of LRP6 showed increasing risk for coronary disease and metabolic disorders (Mani et al., 2007). Previous reports suggest that patients with gain-of-function (GoF) LRP5 mutations show high bone mass (Boyden et al., 2002; Little et al., 2002a), and further study demonstrates that subjects carrying GoF LRP5 mutations and high bone mass are associated with increased lower body fat accumulation (Loh et al., 2015).
The strongest evidence for Wnt/β-catenin pathway module involved in obesity is first from the patients carrying mutations of LGR4, which is a Wnt-FZD-LRP5/6 complex coreceptor. Data from whole-genome sequencing of Icelandic individuals indicates that LGR4 heterozygous mutant subjects showed osteoporosis, electrolyte disturbance, and lowered body weight (Styrkarsdottir et al., 2013). Almost at the same time, our group discovered in humans a novel low-frequency functional missense in LGR4 (A750T), that has been linked with increased obesity risk and metabolic disorders in a study of Chinese obese patients versus controls (Wang J. et al., 2013). Alanine in 750 AAs, located between the third intracellular loop and the sixth transmembrane domain, is conserved among different species and LGRs. In vitro studies showed that this non-synonymous variant has gained higher biological function than wild-type LGR4, which indicates increased LGR4 activity could promote human obesity. Interestingly, constitutively activated point mutations of the corresponding site in LGR1/LHR, LGR2/TSHR, and LGR3/FSHR have been reported (Yano et al., 1995; Gozu et al., 2006; Zhang et al., 2007; Achrekar et al., 2010). In the following studies, we found that the A750T obese carriers have more severe adiposity than those obese non-carriers. Young obese carriers show increased waist circumference, waist-to-height ratio, and a remarkable increase of abdominal visceral fat area. Moreover, the carriers show increased 2-h plasma insulin and the Matsuda index, an indicator of postprandial insulin sensitivity, after an oral glucose tolerance test. These results together suggest that LGR4 might promote central obesity and its metabolic complications. Our mouse model data (Wang J. et al., 2013) has shown that compared with wild-type littermates, Lgr4 homozygous mutant (Lgr4m/m) mice have many more beige adipocytes in vWAT, and further investigation reveals the significant increase of thermogenic genes including UCP1, PGC-1α, and Cidea. These phenomena are amplified by cold stimulation or isoprenaline treatment. Our results demonstrate that ablation of Lgr4 in mice resists dietary and leptin mutant-induced obesity and its metabolic complications, and further prove the crucial role of LGR4 in obesity. Interestingly, recent data show that LGR2/TSHR (Draman et al., 2017) and LGR3/FSHR (Liu et al., 2017) promote browning program. It remains unknown whether the other LGRs could regulate the switch of WAT to BAT and whether they function by Wnt/β-catenin signaling. SNPs in RSPO1/RSPO3/ZNRF43 are also associated with body fat distribution. A genome-wide association studies meta-analysis identified 13 loci are in or near genes, which explains 1.03% of the variance in BMI-adjusted waist-hip ratio. Two of these, RSPO3 and ZNRF3, contribute 0.02 and 0.14%, respectively, of the variance (Heid et al., 2010). It could be concluded here that most of the factors in RSPOs–LGR4/5/6–ZNRF3/RNF43—FZDs-LRPs complex seemed to be important to body fat distribution, and their pivotal role together with Wnt/β-catenin signaling in sWAT and vWAT requires additional study.
The Distinct Role of Wnt/β-Catenin Signaling in APs and Pre-mature/Mature Adipocytes
Zeve and his colleagues observed that altering/enhancing canonical Wnt/β-catenin signaling by transgenic and conditional GoF allele of β-catenin into APs disrupted adipose tissue development in mice. These mutant mice had a lipodystrophic phenotype, a marked lack of fat tissues, and with sequent hypertriglyceridemia. Of note, sWAT of these mice was dense with a complete loss of adipocytes, ensuring fibroblastic lineage cells derived from stem cells, whereas few remaining vWAT adipocytes were hypertrophied. In particular, the secretome of these mutant mice was also altered with increasing levels of glucodyne, a glucose-lowering hormone, which functioned similarly to insulin but through different mechanisms (Zeve et al., 2012). However, the specific origin of the postulated glucose dynamics factor is still unidentified. There are several possibilities that should be investigated, from fibroblastic lineage cells of sWAT, from hypertrophied adipocytes of vWAT, from adipose lineage cells existing in other locations, or from peripheral tissues (e.g., liver and brain), as secondary changes of the adipocyte lineage replaced by fibroblastic cells. These studies highlight the essential role of Wnt signaling in regulating depot-specific adipose precursor cells.
However, there is no systematic researches reported the role of Wnt/β-catenin signaling in the maintenance of potency of adipocyte progenitors. One study has generated NH2-terminally truncated β-catenin mice model, in which stabilized β-catenin could keep activating downstream signals, and observed an expansion of neural precursors (Chenn and Walsh, 2002). Using GSK3 pharmacological inhibitor, it has been suggested that the activation of Wnt/β-catenin signaling was involved in the maintenance of undifferentiated states of both human and mouse embryonic stem cells, along with the persisting expression of Rex-1, Oct-3 and Nanog, which are the specific transcription factors of stemness (Sato et al., 2004). Recently, more and more evidences support the notion that Wnt/β-catenin signaling plays a crucial role in pluripotency (Miyabayashi et al., 2007; Wagner et al., 2010; ten Berge et al., 2011; Clevers et al., 2014), it is not farfetched that we hypothesize this signaling also involves in the adipocyte precursor cells.
It has been proved that mature adipocytes can secrete some factors regulating adipogenesis (Janke et al., 2002). Based on our previous work and other research demonstrating an emerging role of Wnt/β-catenin signaling involved in obesity (Wang J. et al., 2013; Loh et al., 2015), it is hypothesized that Wnt/β-catenin signaling also regulates the secretion of mature adipocytes to affect metabolic function of other cells or tissues.
Why the distinct function of Wnt/β-catenin signaling in adipose precursor cells and pre-mature/mature adipocytes is uncovered till now. As mentioned previously, when β-catenin enters the nucleus, it turns on transcription via binding to members of the TCF/LEF family of transcription factors. The critical step in the activation of Wnt/β-catenin signaling is the formation of a complex comprising β-catenin and members of the TCF/LEF family (Brunner et al., 1997; van de Wetering et al., 1997). β-catenin-TCF/LEF complex needs to recruit transcriptional co-activator to be functional, including the CREB-binding protein (CBP) or its closely associated homolog E1A associated protein p300, B cell lymphoma 9 (BCL-9), the co-activator Pygopus (PYGO) (Hecht et al., 2000; Takemaru and Moon, 2000). Although the co-activators CBP and p300 are up to 93% identical and have extremely high amino acid level homology (McMillan and Kahn, 2005), a report uses the model of differential co-activator and proves the distinct functions of CBP and p300 in the Wnt/β-catenin signaling (Teo et al., 2005). That is, the utilization of CBP leads the proliferation and the maintenance of a potency transcriptional program, whereas utilization of p300 promotes a transcriptional program initiating differentiation. This outcome provides us significant clues to answer the question of whether Wnt/β-catenin signaling activation in adipose precursor cells leads β-catenin to combine with co-activators CBP, resulting in precursor proliferation and potency maintenance; whereas in pre-mature/mature adipocyte, β-catenin combines with co-activators p300, resulting in the initiation of differentiation (Figure 2). Of course, other possible mechanisms should not be excluded.
Depot-Specific Adipocyte Hyperplasia and Hypertrophy in High-Fat Diet (HFD)-Induced Obesity
Although the mechanisms involved in the maintenance of proper adipocyte number and size throughout life are not known, this balance can be disrupted in obesity, leading to adipocyte hyperplasia and hypertrophy in obese models. The relative contributions of hyperplasia and hypertrophy depend on genetic factors, sex, diet risk, exposure time and duration, and the specific depot. Because differentiated adipocytes are postmitotic, adipocyte hyperplasia means an increase in de novo adipogenesis. Adipocyte hypertrophy is a major component of the metabolic syndrome in obese individuals (Sun et al., 2011) and commonly obeserved in obesity development, whereas individual adipocytes have a striking capacity to expand with a twofold to threefold volume (Salans et al., 1973; Hirsch and Batchelor, 1976).
Recent studies suggest that adipocyte hyperplasia plays a crucial role in the development of human obesity (Spalding et al., 2008; Arner et al., 2013). Adipocyte hyperplasia increases the risk of complications that accompany visceral obesity, including diabetes and cardiovascular disease (Wang et al., 2005; Phillips and Prins, 2008). An HFD/high-sugar diet contributes much to human obesity (Ravussin and Tataranni, 1997), and therefore, HFD-induced obesity of rodents is commonly used in the study of obesity development and its metabolic complications (Hariri and Thibault, 2010). A study using immunohistochemical analysis with the thymidine analog bromodeoxyuridine (BrdU) shows that sWAT experiences more hyperplastic growth than vWAT with HFD challenge (Joe et al., 2009). Several studies suggest that new adipocytes are formed several weeks after the initiation of HFD feeding, as the existing mature adipocytes begin to reach their maximal size (Faust et al., 1978; Joe et al., 2009). It was recently shown that the HFD greatly increases WAT mass, and the increasing size of adipocytes indicates that hypertrophy takes a large part of WAT expansion (Lee et al., 2012). In contrast to this conclusion, another study finds that, in response to prolonged HFD, the expansion of gonadal WAT (gWAT, a type of vWAT) in male mice combines both adipocyte hyperplasia and hypertrophy, whereas inguinal WAT (iWAT, a kind of sWAT) in mice does not exhibit significant adipocyte hyperplasia and mass increases mainly through hypertrophy (Wang Q.A. et al., 2013). Of note, both studies suppose that both vWAT and sWAT are first to initiate hypertrophy at the beginning of HFD. By using an adipocyte-specific, tamoxifen-inducible Adiponectin-Cre Estrogen Receptor (Adiponectin-creER) mouse model (Jeffery et al., 2014), combined with a dual fluorescent reporter, Jeffery and her colleagues performed an adipocyte pulse-chase experiment suggesting that HFD in mice rapidly and transiently increased proliferation of APs within vWAT during the first week. Yet, the newly formed APs are not immediately utilized to become mature adipocytes; they are held as a potential reserve and used after seven weeks of HFD stimulation to differentiate into new white adipocytes (Jeffery et al., 2015). These excellent advances prompt us to reflect on the mechanisms that control depot-specific adipogenesis in HFD-induced obesity.
Wnt/β-Catenin Signaling Potential Regulation in Depot-Specific WAT Expansion of HFD-Induced Obesity
Considering the possibility that the activation of Wnt/β-catenin signaling in APs promotes their proliferation whereas in pre-mature/mature adipocytes it regulates differentiation, we hypothesize that Wnt/β-catenin signaling could play an important role in depot-specific WAT expansion (including hyperplasia and hypertrophy) of HFD-induced obesity (Figure 3).
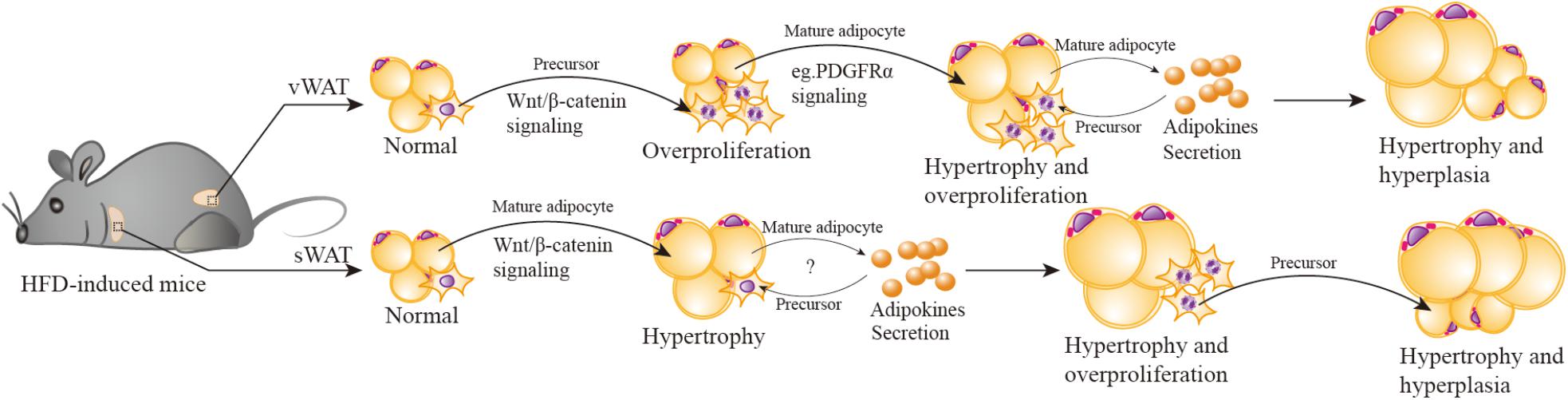
FIGURE 3. Wnt/β-catenin signaling participates in HFD-induced depot-specific hypertrophy and hyperplasia. In response to HFD stimulation, vWAT (including eWAT) adipocyte precursors are first activated by Wnt/β-catenin signaling, leading to their rapid and transient burst of proliferation “overproliferation.” Then adipocyte precursors stop “overproliferation”, remain quiescent, and wait for another signal to reactivate. Meanwhile, the PDGFRα pathway is activated, inhibits “overproliferated” adipocyte precursor adipogenesis (possibly) via suppressing Wnt/β-catenin signaling, then triggers hypertrophy of existing mature adipocytes. Hypertrophied adipocytes may secrete some adipokines to promote the subsequent lipid filling of these activated APs. However, in sWAT (including iWAT), HFD stimulation activates Wnt/β-catenin signaling to promote SWAT mature adipocytes hypertrophy. As hypertrophied adipocytes reach their maximal size and secrete adipokines or emit other signals, adipocyte precursors are then induced to “overproliferation” and new adipocytes will form to meet the demand of increasing lipid storage.
Both vWAT and sWAT are composed of mature adipocytes (the fat-storing cells), adipocyte precursors (that give rise to new adipocytes), and other mesenchymal cells, which include mural, endothelial, inflammatory, and neuronal cells. In response to HFD stimulation, V-APs are first activated via Wnt/β-catenin signaling, leading to their rapid and transient burst of proliferation (“overproliferation”). Based on a previous study (Jeffery et al., 2015), the proliferation of V-APs in HFD mice is significantly decreased after one week and has no statistical difference in comparison with chow-diet mice; the proliferation phase induced by Wnt/β-catenin signaling stands a good chance during the first week. Then APs stop the “overproliferation”, remain quiescent, and wait for another signal to reactivate. Although their transformation happens at least seven weeks after HFD, the fat mass weight continues to increase during this period, indicating the contribution of hypertrophy, in which Wnt/β-catenin signaling maybe deactivated, reactivated, or linked with other mechanisms to regulate the hypertrophy. Notably, a very recent study (Marcelin et al., 2017) reports that in HFD-induced obese mice, platelet-derived growth factor receptor-α-positive (PDGFRα+) adipocyte progenitors can generate a fibrogenic phenotype, which is apt to vWAT fibrosis. More importantly, the activation of PDGFRα pathway promotes PDGFRα+ CD9low cells (committed to adipogenesis) in a phenotypic shift to CD9high fibrogenic cells (originate from profibrotic cells). These observations remind us of the relationship of the PDGFRα pathway and vWAT adipocyte hypertrophy. It is possible that PDGFRα pathway activation inhibits “overproliferation” of APs by suppressing Wnt/β-catenin signaling, then triggers the hypertrophy of existing mature adipocytes. It has been hypothesized that an adipogenic signal is generated to stimulate the production of new adipocytes when mature adipocytes reach their maximal size (Faust et al., 1978; Cleary et al., 1979). Wang also mentioned that because of the in vivo system, the maturation of activated APs takes several weeks, it is likely that the subsequent lipid filling of these APs is promoted by signal(s) from hypertrophied adipocytes (Wang Q.A. et al., 2013). The specific signal(s) is unclear; some unidentified adipokines (secreted by adipocytes) maybe present in parallel. The mechanism that controls the signal(s) activation is also unknown.
It is important to note that the manner of expansion in sWAT depot is significantly distinct from that of vWAT. The activation of Wnt/β-catenin signaling by HFD stimulation mainly promotes sWAT mature adipocytes hypertrophy, and this process is maintained for up to 12 weeks (Wang Q.A. et al., 2013). It is plausible that with a prolonged HFD stimulation exceeding 12 weeks, and hypertrophied adipocytes reach their maximal size and secrete adipokines or emit other signals, APs will be induced to “overproliferation” and new adipocytes will form to meet the increasing demand of lipid storage. To verify this hypothesis, we have ablated Ctnnb1/β-catenin specifically in mature adipocytes using Adiponectin-cre. We observed that the mutant mice resisted to long-term HFD-induced obesity and their adipocyte size was comparable to control mice, but unexpectedly, the number of mature adipocytes and APs were decreased. Further study discovered that β-catenin regulated an adipokine secretion from mature adipocytes, which could promote APs proliferation. This would provide new clues for the roles of Wnt/β-catenin in cross-talk between mature adipocytes and APs under energy enrichment environment.
During the WAT expansion process, there is crosstalk among many other possible regulators with Wnt/β-catenin signaling that control the fate of sWAT and vWAT, and the mechanism deserves more study.
Targeting Wnt/β-Catenin Signaling in Depot-Specific “Browning”
Beige adipocytes (Ishibashi and Seale, 2010) [or brite adipocytes (Petrovic et al., 2010)], which have a multilocular morphology and express uncoupling protein 1 (UCP1) comparable to classical brown adipocytes (Wu et al., 2012; Shabalina et al., 2013), which expend energy via uncoupled mitochondrial respiration (Harms and Seale, 2013), sporadically reside within WAT (Young et al., 1984; Collins et al., 1997; Guerra et al., 1998; Cinti, 1999; Himms-Hagen et al., 2000; Vitali et al., 2012). It has been thought that beige adipocytes have no morphological difference between sWAT and vWAT depots. However, it is hypothesized that beige adipocytes of vWAT depots may have a lower content of mitochondria per cell according to a recent study (Szabo and Zoratti, 2017). Beige adipocytes can protect against the development of obesity in rodents (Seale et al., 2011), and adult humans have functional BAT mainly with beige-like adipocyte features (Sharp et al., 2012; Wu et al., 2012; Lee et al., 2014; Shinoda et al., 2015), so it becomes a novel idea to promote the WAT browning program for the treatment of obesity.
Beige adipocytes are induced by certain external cues, such as cold stimulation, β3 adrenergic receptor agonists, and exercise. This phenomenon is often called “browning” of WAT, which often occurs in the postnatal stages (Harms and Seale, 2013; Wu et al., 2013; Kajimura and Saito, 2014; Wang and Seale, 2016). The browning process involves cell fate switch and differentiation by internal hormonal, neural, and even immunological triggers and following transcription factors such as PRDM16, PGC-1α, ZNF243, Rb1, FOXC2, and EBF2 (Wang and Seale, 2016). However, the mechanisms of depot-specific beige adipocyte formation have not been completely elucidated. The two proposed mechanisms are de novo formation from adipocyte precursor cells and trans-differentiation of mature white adipocytes (Lee and Cowan, 2013). For example, a study has observed that adipocytes that contained “paucilocular” lipid droplets after the cold stimulation may reveal an intermediate morphological state between white and beige adipocytes (Barbatelli et al., 2010). Moreover, a recent study uncovered a fundamental phenomenon that after withdrawing external stimuli, beige adipocytes progressively lose their morphological and molecular characteristics, directly acquiring white-like characteristics bypassing an intermediate precursor stage (Altshuler-Keylin et al., 2016). This is consistent with a previous study (Rosenwald et al., 2013) using a genetic UCP1 lineage-tracing method, showing that after five weeks of warm adaptation, activated beige adipocytes of sWAT would convert into mature white adipocytes; importantly, these white-type adipocytes had the capacity to reconvert into beige adipocytes when re-exposed to cold stimulation. This study also proposed that there may be dormant beige adipocytes (unilocular and UCP1-) that can be active to multilocular and UCP1+ beige adipocytes. Interestingly, a new study found that beige adipocytes of vWAT could clearly be divided into two distinct subpopulations, UCP1+ and UCP1- (Bertholet et al., 2017). However, the work of Wang observed that most of the newly emerging beige adipocytes were derived from de novo differentiation of precursors in sWAT by performing pulse-chase fate-mapping experiments of mature adipocytes (Wang Q.A. et al., 2013). Hence, the specific origin of beige adipocytes still needs to be critically investigated by monitoring the life cycle dynamics of beige adipocytes at a single-cell resolution, and this review does not go into great detail about this.
Previous studies have suggested that the appearance of beige cells is observed mainly in sWAT, and newly emerging beige cells are hardly detected in the vWAT depot (Seale et al., 2008). The most significant functional difference between S-APs and V-APs is that the former has a higher proliferation rate and better differentiation than the latter in response to adipogenic stimuli in vitro (Tchkonia et al., 2005; Macotela et al., 2012). However, several works (Himms-Hagen et al., 2000; Lee et al., 2012; Frontini et al., 2013) found that an increase of the mitotic index of V-APs was far greater than S-APs in response to the treatment of CL316,243, a β3-adrenergic receptor agonist. Most newly emerging beige adipocytes in vWAT were positive for BrdU or Ki67; however, only a small percentage of indicated beige adipocytes in sWAT were BrdU+ or Ki67+.
It is clear that Wnt/β-catenin signaling has a crucial role in the commitment of maintenance and induction of differentiation. The study by Michael (Kahn, 2014) has depicted the model of stem cell division, including symmetric and asymmetric division, and described how the outcome of the division is regulated by mitotic spindle. This model leads us to consider the possibility that Wnt/β-catenin signaling activates the depot-specific APs asymmetric division (Figure 4).
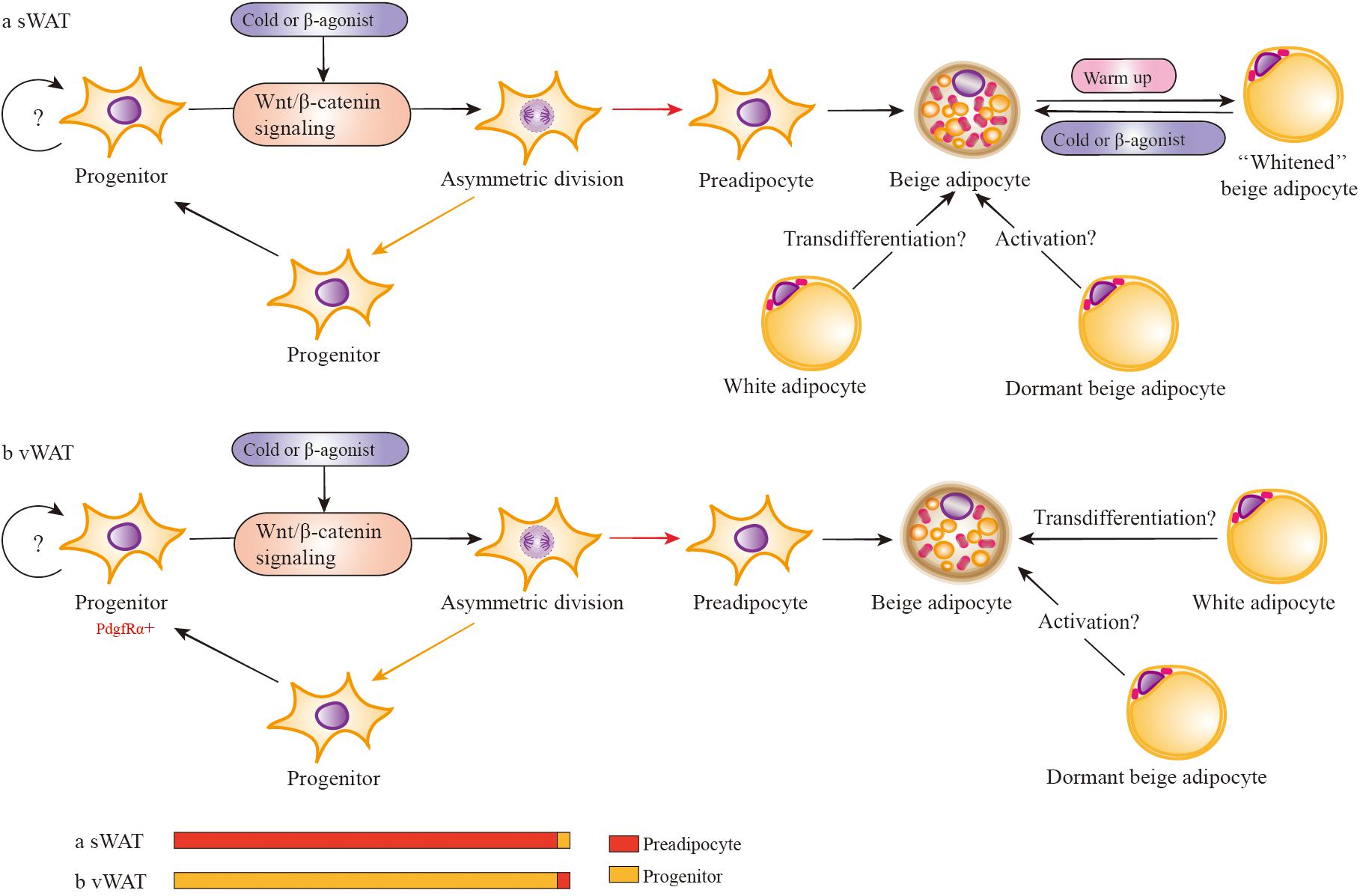
FIGURE 4. Wnt/β-catenin signaling regulates the development of depot-specific beige adipocytes. In response to cold or β3 adrenergic receptor agonists, both sWAT and vWAT adipocyte progenitors (in adipose cells, APs consist of adipocyte progenitors and preadipocytes) are induced to asymmetric division possibly via the activation of Wnt/β-catenin signaling. However, the division percentage between preadipocytes and adipocyte progenitors is different in sWAT and vWAT. Most likely, the percentage of vWAT adipocyte progenitors dividing into progenitors is far more than that of preadipocytes. There is the possibility that mature white adipocytes may directly transform into beige adipocytes via transdifferentiation in vivo. In addition, there are dormant beige adipocytes (unilocular and UCP1-) that can be activated to multilocular and UCP1+ beige adipocytes. Activated beige adipocytes of sWAT can be converted into white-type adipocytes when warmed up, and these white-type adipocytes have the capacity to reconvert into beige adipocytes when re-exposed to cold stimulation.
In response to cold or β3-adrenergic receptor agonists or other beige adipogenic stimulations, both sWAT and vWAT adipocyte progenitors are induced to asymmetric division via some crucial signals, such as the activation of Wnt/β-catenin signaling. However, the division percentage into adipocyte progenitors and preadipocytes is different in sWAT and vWAT. Most likely, the percentage of vWAT adipocyte progenitors dividing into progenitors is far more than preadipocytes, resulting in the majority of newly emerging beige adipocytes being positive for BrdU (Lee et al., 2012). However, the percentage is lower in sWAT adipocyte progenitors, which divide into progenitors much less often than preadipocytes, and the lack of BrdU+ cells (Lee et al., 2012) indicates the absence of the cell-proliferative step in sWAT. If beige adipocytes are derived from differentiated preadipocytes with very limited proliferation of progenitors, the newly emerging cells will also be BrdU-. In addition, it is consistent with previous observations that induced beige cells are mainly found in sWAT and are seldom detected in vWAT (Seale et al., 2008). Regarding previous studies about Wnt/β-catenin signaling with adiposity, it is important to demonstrate the role of RSPOs-LGRs-ZNRF3/RNF43-FZDs-LRPs complex in asymmetric division in depot-specific adipocyte progenitors during browning.
Conclusion
What is the precise role of Wnt/β-catenin signaling in depot-specific browning and white fat expansion? Which factors interact with Wnt/β-catenin signaling underlying the different features of sWAT and vWAT? What is the mechanism by which Wnt/β-catenin signaling maintains the potency of APs and regulates the differentiation of mature adipocytes under external stimuli? If beige cells are derived from preadipocytes, the question is how Wnt/β-catenin signaling regulates the preadipocytes differentiation into beige cells. And if there are other sources for beige cells, such as trans-differentiation from mature adipocytes and activation of dormant beige adipocytes, how does Wnt/β-catenin signaling be involved in these processes, and how does it participate in and are there different roles and mechanisms in different WAT depots? Although we cannot yet definitively answer these questions, according to more and more evidences and our recent interesting discovery about the role of β-catenin in mature adipocytes, it is highly hypothesized that Wnt/β-catenin signaling is crucial for obesity development, including adipocyte hyperplasia, hypertrophy, browning, and even apoptosis, in a depot-specific manner.
With the application of new methodologies and experimental models to the field of adipose tissue biology, we have the opportunity to definitively explore the WAT intrinsic mechanisms that regulate adipocyte expansion and browning in vivo. As the pathogenesis, clinical features, and intervention outcomes of obesity are greatly affected by fat distribution and cellular characteristics, the study of Wnt/β-catenin signaling in WAT expansion and browning in vivo has the potential to discover novel therapeutic targets for precise clinical stratification and treatment for obesity.
Author Contributions
JW and NC conceived the project. NC drafted the paper. JW contributed comments and advice on the manuscript. All authors were involved in editing the manuscript.
Funding
This work was supported by the National Nature Science Foundation of China (Grant No. 81522011).
Conflict of Interest Statement
The authors declare that the research was conducted in the absence of any commercial or financial relationships that could be construed as a potential conflict of interest.
The reviewer DV and handling Editor declared their shared affiliation.
Acknowledgments
The authors thank Dr. Ruixin Liu for her contribution to manuscript editing.
References
Achrekar, S. K., Modi, D. N., Meherji, P. K., Patel, Z. M., and Mahale, S. D. (2010). Follicle stimulating hormone receptor gene variants in women with primary and secondary amenorrhea. J. Assist. Reprod. Genet. 27, 317–326. doi: 10.1007/s10815-010-9404-9
Alberici, P., and Fodde, R. (2006). The role of the APC tumor suppressor in chromosomal instability. Genome Dyn. 1, 149–170. doi: 10.1159/000092506
Alonso, L., and Fuchs, E. (2003). Stem cells in the skin: waste not, Wnt not. Genes Dev. 17, 1189–1200. doi: 10.1101/gad.1086903
Altshuler-Keylin, S., Shinoda, K., Hasegawa, Y., Ikeda, K., Hong, H., Kang, Q., et al. (2016). Beige adipocyte maintenance is regulated by autophagy-induced mitochondrial clearance. Cell Metab. 24, 402–419. doi: 10.1016/j.cmet.2016.08.002
Anastas, J. N., and Moon, R. T. (2013). WNT signalling pathways as therapeutic targets in cancer. Nat. Rev. Cancer 13, 11–26. doi: 10.1038/nrc3419
Arner, P., Andersson, D. P., Thorne, A., Wiren, M., Hoffstedt, J., Naslund, E., et al. (2013). Variations in the size of the major omentum are primarily determined by fat cell number. J. Clin. Endocrinol. Metab. 98, E897–E901. doi: 10.1210/jc.2012-4106
Baker, N. E. (1987). Molecular cloning of sequences from wingless, a segment polarity gene in Drosophila: the spatial distribution of a transcript in embryos. EMBO J. 6, 1765–1773.
Barbatelli, G., Murano, I., Madsen, L., Hao, Q., Jimenez, M., Kristiansen, K., et al. (2010). The emergence of cold-induced brown adipocytes in mouse white fat depots is determined predominantly by white to brown adipocyte transdifferentiation. Am. J. Physiol. Endocrinol. Metab. 298, E1244–E1253. doi: 10.1152/ajpendo.00600.2009
Behrens, J., von Kries, J. P., Kuhl, M., Bruhn, L., Wedlich, D., Grosschedl, R., et al. (1996). Functional interaction of beta-catenin with the transcription factor LEF-1. Nature 382, 638–642. doi: 10.1038/382638a0
Berry, R., Jeffery, E., and Rodeheffer, M. S. (2014). Weighing in on adipocyte precursors. Cell Metab. 19, 8–20. doi: 10.1016/j.cmet.2013.10.003
Berry, R., and Rodeheffer, M. S. (2013). Characterization of the adipocyte cellular lineage in vivo. Nat. Cell Biol. 15, 302–308. doi: 10.1038/ncb2696
Bertholet, A. M., Kazak, L., Chouchani, E. T., Bogaczynska, M. G., Paranjpe, I., Wainwright, G. L., et al. (2017). Mitochondrial patch clamp of beige adipocytes reveals UCP1-positive and UCP1-negative cells both exhibiting futile creatine cycling. Cell Metab. 25, 811.e4–822.e4. doi: 10.1016/j.cmet.2017.03.002
Berwick, D. C., and Harvey, K. (2012). The importance of Wnt signalling for neurodegeneration in Parkinson’s disease. Biochem. Soc. Trans. 40, 1123–1128. doi: 10.1042/BST20120122
Bhanot, P., Brink, M., Samos, C. H., Hsieh, J. C., Wang, Y., Macke, J. P., et al. (1996). A new member of the frizzled family from Drosophila functions as a Wingless receptor. Nature 382, 225–230. doi: 10.1038/382225a0
Birsoy, K., Berry, R., Wang, T., Ceyhan, O., Tavazoie, S., Friedman, J. M., et al. (2011). Analysis of gene networks in white adipose tissue development reveals a role for ETS2 in adipogenesis. Development 138, 4709–4719. doi: 10.1242/dev.067710
Boyden, L. M., Mao, J., Belsky, J., Mitzner, L., Farhi, A., Mitnick, M. A., et al. (2002). High bone density due to a mutation in LDL-receptor-related protein 5. N. Engl. J. Med. 346, 1513–1521. doi: 10.1056/NEJMoa013444
Brembeck, F. H., Rosario, M., and Birchmeier, W. (2006). Balancing cell adhesion and Wnt signaling, the key role of beta-catenin. Curr. Opin. Genet. Dev. 16, 51–59. doi: 10.1016/j.gde.2005.12.007
Brunner, E., Peter, O., Schweizer, L., and Basler, K. (1997). pangolin encodes a Lef-1 homologue that acts downstream of Armadillo to transduce the Wingless signal in Drosophila. Nature 385, 829–833. doi: 10.1038/385829a0
Carmon, K. S., Gong, X., Lin, Q., Thomas, A., and Liu, Q. (2011). R-spondins function as ligands of the orphan receptors LGR4 and LGR5 to regulate Wnt/beta-catenin signaling. Proc. Natl. Acad. Sci. U.S.A. 108, 11452–11457. doi: 10.1073/pnas.1106083108
Cauchi, S., Choquet, H., Gutierrez-Aguilar, R., Capel, F., Grau, K., Proenca, C., et al. (2008). Effects of TCF7L2 polymorphisms on obesity in European populations. Obesity 16, 476–482. doi: 10.1038/oby.2007.77
Cauchi, S., Meyre, D., Dina, C., Choquet, H., Samson, C., Gallina, S., et al. (2006). Transcription factor TCF7L2 genetic study in the French population: expression in human beta-cells and adipose tissue and strong association with type 2 diabetes. Diabetes Metab. Res. Rev. 55, 2903–2908. doi: 10.2337/db06-0474
Cawthorn, W. P., Heyd, F., Hegyi, K., and Sethi, J. K. (2007). Tumour necrosis factor-alpha inhibits adipogenesis via a beta-catenin/TCF4(TCF7L2)-dependent pathway. Cell Death Differ. 14, 1361–1373. doi: 10.1038/sj.cdd.4402127
Chau, Y. Y., Bandiera, R., Serrels, A., Martinez-Estrada, O. M., Qing, W., Lee, M., et al. (2014). Visceral and subcutaneous fat have different origins and evidence supports a mesothelial source. Nat. Cell Biol. 16, 367–375. doi: 10.1038/ncb2922
Chen, P. H., Chen, X., Lin, Z., Fang, D., and He, X. (2013). The structural basis of R-spondin recognition by LGR5 and RNF43. Genes Dev. 27, 1345–1350. doi: 10.1101/gad.219915.113
Chen, W., ten Berge, D., Brown, J., Ahn, S., Hu, L. A., Miller, W. E., et al. (2003). Dishevelled 2 recruits beta-arrestin 2 to mediate Wnt5A-stimulated endocytosis of Frizzled 4. Science 301, 1391–1394. doi: 10.1126/science.1082808
Chen, X., Ayala, I., Shannon, C., Fourcaudot, M., Acharya, N. K., Jenkinson, C. P., et al. (2018). The diabetes gene and Wnt pathway effector TCF7L2 regulates adipocyte development and function. Diabetes 67, 554–568. doi: 10.2337/db17-0318
Chenn, A., and Walsh, C. A. (2002). Regulation of cerebral cortical size by control of cell cycle exit in neural precursors. Science 297, 365–369. doi: 10.1126/science.1074192
Cho, Y. S., Chen, C. H., Hu, C., Long, J., Ong, R. T., Sim, X., et al. (2011). Meta-analysis of genome-wide association studies identifies eight new loci for type 2 diabetes in east Asians. Nat. Genet. 44, 67–72. doi: 10.1038/ng.1019
Choi, M. S., Kim, Y. J., Kwon, E. Y., Ryoo, J. Y., Kim, S. R., and Jung, U. J. (2015). High-fat diet decreases energy expenditure and expression of genes controlling lipid metabolism, mitochondrial function and skeletal system development in the adipose tissue, along with increased expression of extracellular matrix remodelling- and inflammation-related genes. Br. J. Nutr. 113, 867–877. doi: 10.1017/S0007114515000100
Chondronikola, M., Volpi, E., Borsheim, E., Porter, C., Annamalai, P., Enerback, S., et al. (2014). Brown adipose tissue improves whole-body glucose homeostasis and insulin sensitivity in humans. Diabetes Metab. Res. Rev. 63, 4089–4099. doi: 10.2337/db14-0746
Christodoulides, C., Scarda, A., Granzotto, M., Milan, G., Dalla Nora, E., Keogh, J., et al. (2006). WNT10B mutations in human obesity. Diabetologia 49, 678–684. doi: 10.1007/s00125-006-0144-4
Cinti, S. (2005). The adipose organ. Prostaglandins Leukot. Essent. Fatty Acids 73, 9–15. doi: 10.1016/j.plefa.2005.04.010
Cleary, M. P., Brasel, J. A., and Greenwood, M. R. (1979). Developmental changes in thymidine kinase, DNA, and fat cellularity in Zucker rats. Am. J. Physiol. 236, E508–E513. doi: 10.1152/ajpendo.1979.236.5.E508
Clevers, H., Loh, K. M., and Nusse, R. (2014). Stem cell signaling. An integral program for tissue renewal and regeneration: Wnt signaling and stem cell control. Science 346:1248012. doi: 10.1126/science.1248012
Clevers, H., and Nusse, R. (2012). Wnt/beta-catenin signaling and disease. Cell 149, 1192–1205. doi: 10.1016/j.cell.2012.05.012
Cohen, P., Levy, J. D., Zhang, Y., Frontini, A., Kolodin, D. P., Svensson, K. J., et al. (2014). Ablation of PRDM16 and beige adipose causes metabolic dysfunction and a subcutaneous to visceral fat switch. Cell 156, 304–316. doi: 10.1016/j.cell.2013.12.021
Collins, S., Daniel, K. W., Petro, A. E., and Surwit, R. S. (1997). Strain-specific response to beta 3-adrenergic receptor agonist treatment of diet-induced obesity in mice. Endocrinology 138, 405–413. doi: 10.1210/endo.138.1.4829
Coluccia, A. M., Vacca, A., Dunach, M., Mologni, L., Redaelli, S., Bustos, V. H., et al. (2007). Bcr-Abl stabilizes beta-catenin in chronic myeloid leukemia through its tyrosine phosphorylation. EMBO J. 26, 1456–1466. doi: 10.1038/sj.emboj.7601485
Cypess, A. M., Lehman, S., Williams, G., Tal, I., Rodman, D., Goldfine, A. B., et al. (2009). Identification and importance of brown adipose tissue in adult humans. N. Engl. J. Med. 360, 1509–1517. doi: 10.1056/NEJMoa0810780
Cypess, A. M., White, A. P., Vernochet, C., Schulz, T. J., Xue, R., Sass, C. A., et al. (2013). Anatomical localization, gene expression profiling and functional characterization of adult human neck brown fat. Nat. Med. 19, 635–639. doi: 10.1038/nm.3112
de La Coste, A., Romagnolo, B., Billuart, P., Renard, C. A., Buendia, M. A., Soubrane, O., et al. (1998). Somatic mutations of the beta-catenin gene are frequent in mouse and human hepatocellular carcinomas. Proc. Natl. Acad. Sci. U.S.A. 95, 8847–8851. doi: 10.1073/pnas.95.15.8847
de Lau, W., Barker, N., Low, T. Y., Koo, B. K., Li, V. S., Teunissen, H., et al. (2011). Lgr5 homologues associate with Wnt receptors and mediate R-spondin signalling. Nature 476, 293–297. doi: 10.1038/nature10337
de Lau, W., Peng, W. C., Gros, P., and Clevers, H. (2014). The R-spondin/Lgr5/Rnf43 module: regulator of Wnt signal strength. Genes Dev. 28, 305–316. doi: 10.1101/gad.235473.113
Draman, M. S., Stechman, M., Scott-Coombes, D., Dayan, C. M., Rees, D. A., Ludgate, M., et al. (2017). The role of thyrotropin receptor activation in adipogenesis and modulation of fat phenotype. Front. Endocrinol. 8:83. doi: 10.3389/fendo.2017.00083
Droyvold, W. B., Nilsen, T. I., Kruger, O., Holmen, T. L., Krokstad, S., Midthjell, K., et al. (2006). Change in height, weight and body mass index: longitudinal data from the HUNT Study in Norway. Int. J. Obes. 30, 935–939. doi: 10.1038/sj.ijo.0803178
Emdin, C. A., Khera, A. V., Natarajan, P., Klarin, D., Zekavat, S. M., Hsiao, A. J., et al. (2017). Genetic association of waist-to-hip ratio with cardiometabolic traits, type 2 diabetes, and coronary heart disease. JAMA 317, 626–634. doi: 10.1001/jama.2016.21042
Fagotto, F. (2013). Looking beyond the Wnt pathway for the deep nature of beta-catenin. EMBO Rep. 14, 422–433. doi: 10.1038/embor.2013.45
Faust, I. M., Johnson, P. R., Stern, J. S., and Hirsch, J. (1978). Diet-induced adipocyte number increase in adult rats: a new model of obesity. Am. J. Physiol. 235, E279–E286. doi: 10.1152/ajpendo.1978.235.3.E279
Foster, M. T., Shi, H. F., Seeley, R. J., and Woods, S. C. (2010). Transplantation or removal of intra-abdominal adipose tissue prevents age-induced glucose insensitivity. Physiol. Behav. 101, 282–288. doi: 10.1016/j.physbeh.2010.05.014
Frontini, A., Vitali, A., Perugini, J., Murano, I., Romiti, C., Ricquier, D., et al. (2013). White-to-brown transdifferentiation of omental adipocytes in patients affected by pheochromocytoma. Biochim. Biophys. Acta 1831, 950–959. doi: 10.1016/j.bbalip.2013.02.005
Glinka, A., Dolde, C., Kirsch, N., Huang, Y. L., Kazanskaya, O., Ingelfinger, D., et al. (2011). LGR4 and LGR5 are R-spondin receptors mediating Wnt/beta-catenin and Wnt/PCP signalling. EMBO Rep. 12, 1055–1061. doi: 10.1038/embor.2011.175
Gong, X., Carmon, K. S., Lin, Q., Thomas, A., Yi, J., and Liu, Q. (2012). LGR6 is a high affinity receptor of R-spondins and potentially functions as a tumor suppressor. PLoS One 7:e37137. doi: 10.1371/journal.pone.0037137
Gong, Y., Slee, R. B., Fukai, N., Rawadi, G., Roman-Roman, S., Reginato, A. M., et al. (2001). LDL receptor-related protein 5 (LRP5) affects bone accrual and eye development. Cell 107, 513–523. doi: 10.1016/S0092-8674(01)00571-2
Gozu, H. I., Bircan, R., Krohn, K., Muller, S., Vural, S., Gezen, C., et al. (2006). Similar prevalence of somatic TSH receptor and Gsalpha mutations in toxic thyroid nodules in geographical regions with different iodine supply in Turkey. Eur. J. Endocrinol. 155, 535–545. doi: 10.1530/eje.1.02253
Grant, S. F., Thorleifsson, G., Reynisdottir, I., Benediktsson, R., Manolescu, A., Sainz, J., et al. (2006). Variant of transcription factor 7-like 2 (TCF7L2) gene confers risk of type 2 diabetes. Nat. Genet. 38, 320–323. doi: 10.1038/ng1732
Grove, K. L., Fried, S. K., Greenberg, A. S., Xiao, X. Q., and Clegg, D. J. (2010). A microarray analysis of sexual dimorphism of adipose tissues in high-fat-diet-induced obese mice. Int. J. Obes. 34, 989–1000. doi: 10.1038/ijo.2010.12
Guerra, C., Koza, R. A., Yamashita, H., Walsh, K., and Kozak, L. P. (1998). Emergence of brown adipocytes in white fat in mice is under genetic control. Effects on body weight and adiposity. J. Clin. Invest. 102, 412–420. doi: 10.1172/JCI3155
Gumbiner, B. M. (1997). Carcinogenesis: a balance between beta-catenin and APC. Curr. Biol. 7, R443–R446. doi: 10.1016/S0960-9822(06)00214-4
Guo, S. S., Zeller, C., Chumlea, W. C., and Siervogel, R. M. (1999). Aging, body composition, and lifestyle: the Fels Longitudinal Study. Am. J. Clin. Nutr. 70, 405–411. doi: 10.1093/ajcn/70.3.405
Han, J., Lee, J. E., Jin, J., Lim, J. S., Oh, N., Kim, K., et al. (2011). The spatiotemporal development of adipose tissue. Development 138, 5027–5037. doi: 10.1242/dev.067686
Hao, H. X., Xie, Y., Zhang, Y., Charlat, O., Oster, E., Avello, M., et al. (2012). ZNRF3 promotes Wnt receptor turnover in an R-spondin-sensitive manner. Nature 485, 195–200. doi: 10.1038/nature11019
Hariri, N., and Thibault, L. (2010). High-fat diet-induced obesity in animal models. Nutr. Res. Rev. 23, 270–299. doi: 10.1017/S0954422410000168
Harms, M., and Seale, P. (2013). Brown and beige fat: development, function and therapeutic potential. Nat. Med. 19, 1252–1263. doi: 10.1038/nm.3361
Haupt, A., Thamer, C., Heni, M., Ketterer, C., Machann, J., Schick, F., et al. (2010). Gene variants of TCF7L2 influence weight loss and body composition during lifestyle intervention in a population at risk for type 2 diabetes. Diabetes Metab. Res. Rev. 59, 747–750. doi: 10.2337/db09-1050
Hecht, A., Vleminckx, K., Stemmler, M. P., van Roy, F., and Kemler, R. (2000). The p300/CBP acetyltransferases function as transcriptional coactivators of beta-catenin in vertebrates. EMBO J. 19, 1839–1850. doi: 10.1093/emboj/19.8.1839
Heid, I. M., Jackson, A. U., Randall, J. C., Winkler, T. W., Qi, L., Steinthorsdottir, V., et al. (2010). Meta-analysis identifies 13 new loci associated with waist-hip ratio and reveals sexual dimorphism in the genetic basis of fat distribution. Nat. Genet. 42, 949–960. doi: 10.1038/ng.685
Heymsfield, S. B., Longo, D. L., and Wadden, T. A. (2017). Mechanisms, pathophysiology, and management of obesity. N. Engl. J. Med. 376, 254–266. doi: 10.1056/NEJMra1514009
Himms-Hagen, J., Melnyk, A., Zingaretti, M. C., Ceresi, E., Barbatelli, G., and Cinti, S. (2000). Multilocular fat cells in WAT of CL-316243-treated rats derive directly from white adipocytes. Am. J. Physiol. Cell Physiol. 279, C670–C681. doi: 10.1152/ajpcell.2000.279.3.C670
Hirsch, J., and Batchelor, B. (1976). Adipose tissue cellularity in human obesity. Clin. Endocrinol. Metab. 5, 299–311. doi: 10.1016/S0300-595X(76)80023-0
Hoffmeyer, K., Raggioli, A., Rudloff, S., Anton, R., Hierholzer, A., Del Valle, I., et al. (2012). Wnt/beta-catenin signaling regulates telomerase in stem cells and cancer cells. Science 336, 1549–1554. doi: 10.1126/science.1218370
Hu, B., Wang, Q., Wang, Y. A., Hua, S., Sauve, C. G., Ong, D., et al. (2016). Epigenetic activation of WNT5A drives glioblastoma stem cell differentiation and invasive growth. Cell 167, 1281.e18–1295.e18. doi: 10.1016/j.cell.2016.10.039
Inestrosa, N. C., Montecinos-Oliva, C., and Fuenzalida, M. (2012). Wnt signaling: role in Alzheimer disease and schizophrenia. J. Neuroimmune Pharmacol. 7, 788–807. doi: 10.1007/s11481-012-9417-5
Ishibashi, J., and Seale, P. (2010). Medicine. Beige can be slimming. Science 328, 1113–1114. doi: 10.1126/science.1190816
Janke, J., Engeli, S., Gorzelniak, K., Luft, F. C., and Sharma, A. M. (2002). Mature adipocytes inhibit in vitro differentiation of human preadipocytes via angiotensin type 1 receptors. Diabetes Metab. Res. Rev. 51, 1699–1707. doi: 10.2337/diabetes.51.6.1699
Jeffery, E., Berry, R., Church, C. D., Yu, S., Shook, B. A., Horsley, V., et al. (2014). Characterization of Cre recombinase models for the study of adipose tissue. Adipocyte 3, 206–211. doi: 10.4161/adip.29674
Jeffery, E., Church, C. D., Holtrup, B., Colman, L., and Rodeheffer, M. S. (2015). Rapid depot-specific activation of adipocyte precursor cells at the onset of obesity. Nat. Cell Biol. 17, 376–385. doi: 10.1038/ncb3122
Jeffery, E., Wing, A., Holtrup, B., Sebo, Z., Kaplan, J. L., Saavedra-Pena, R., et al. (2016). The adipose tissue microenvironment regulates depot-specific adipogenesis in obesity. Cell Metab. 24, 142–150. doi: 10.1016/j.cmet.2016.05.012
Jiang, X., Charlat, O., Zamponi, R., Yang, Y., and Cong, F. (2015). Dishevelled promotes Wnt receptor degradation through recruitment of ZNRF3/RNF43 E3 ubiquitin ligases. Mol. Cell 58, 522–533. doi: 10.1016/j.molcel.2015.03.015
Jiang, X., and Cong, F. (2016). Novel regulation of wnt signaling at the proximal membrane level. Trends Biochem. Sci. 41, 773–783. doi: 10.1016/j.tibs.2016.06.003
Jiang, Y., Berry, D. C., Tang, W., and Graff, J. M. (2014). Independent stem cell lineages regulate adipose organogenesis and adipose homeostasis. Cell Rep. 9, 1007–1022. doi: 10.1016/j.celrep.2014.09.049
Joe, A. W., Yi, L., Even, Y., Vogl, A. W., and Rossi, F. M. (2009). Depot-specific differences in adipogenic progenitor abundance and proliferative response to high-fat diet. Stem Cells 27, 2563–2570. doi: 10.1002/stem.190
Kahn, M. (2014). Can we safely target the WNT pathway? Nat. Rev. Drug Discov. 13, 513–532. doi: 10.1038/nrd4233
Kajimura, S., and Saito, M. (2014). A new era in brown adipose tissue biology: molecular control of brown fat development and energy homeostasis. Annu. Rev. Physiol. 76, 225–249. doi: 10.1146/annurev-physiol-021113-170252
Kanazawa, A., Tsukada, S., Sekine, A., Tsunoda, T., Takahashi, A., Kashiwagi, A., et al. (2004). Association of the gene encoding wingless-type mammary tumor virus integration-site family member 5B (WNT5B) with type 2 diabetes. Am. J. Hum. Genet. 75, 832–843. doi: 10.1086/425340
Karastergiou, K., and Fried, S. K. (2013). Multiple adipose depots increase cardiovascular risk via local and systemic effects. Curr. Atheroscler. Rep. 15:361. doi: 10.1007/s11883-013-0361-5
Kawabata, A. (2011). Prostaglandin E-2 and pain-an update. Biol. Pharm. Bull. 34, 1170–1173. doi: 10.1248/bpb.34.1170
Kazanskaya, O., Glinka, A., del Barco Barrantes, I., Stannek, P., Niehrs, C., and Wu, W. (2004). R-Spondin2 is a secreted activator of Wnt/beta-catenin signaling and is required for Xenopus myogenesis. Dev. Cell 7, 525–534. doi: 10.1016/j.devcel.2004.07.019
Kelley, D. E., Thaete, F. L., Troost, F., Huwe, T., and Goodpaster, B. H. (2000). Subdivisions of subcutaneous abdominal adipose tissue and insulin resistance. Am. J. Physiol. Endocrinol. Metab. 278, E941–E948. doi: 10.1152/ajpendo.2000.278.5.E941
Kim, K. A., Wagle, M., Tran, K., Zhan, X., Dixon, M. A., Liu, S., et al. (2008). R-Spondin family members regulate the Wnt pathway by a common mechanism. Mol. Biol. Cell 19, 2588–2596. doi: 10.1091/mbc.E08-02-0187
Koo, B. K., Spit, M., Jordens, I., Low, T. Y., Stange, D. E., van de Wetering, M., et al. (2012). Tumour suppressor RNF43 is a stem-cell E3 ligase that induces endocytosis of Wnt receptors. Nature 488, 665–669. doi: 10.1038/nature11308
Kooner, J. S., Saleheen, D., Sim, X., Sehmi, J., Zhang, W., Frossard, P., et al. (2011). Genome-wide association study in individuals of South Asian ancestry identifies six new type 2 diabetes susceptibility loci. Nat. Genet. 43, 984–989. doi: 10.1038/ng.921
Kovacs, P., Berndt, J., Ruschke, K., Kloting, N., Schon, M. R., Korner, A., et al. (2008). TCF7L2 gene expression in human visceral and subcutaneous adipose tissue is differentially regulated but not associated with type 2 diabetes mellitus. Metabolism 57, 1227–1231. doi: 10.1016/j.metabol.2008.04.016
Krueger, K. C., Costa, M. J., Du, H. Q., and Feldman, B. J. (2014). Characterization of cre recombinase activity for in vivo targeting of adipocyte precursor cells. Stem Cell Rep. 3, 1147–1158. doi: 10.1016/j.stemcr.2014.10.009
Le, N. H., and Fodde, R. (2008). Tumour-stroma interactions in colorectal cancer: converging on beta-catenin activation and cancer stemness. Br. J. Cancer 98, 1886–1893. doi: 10.1038/sj.bjc.6604401
Lee, P., Werner, C. D., Kebebew, E., and Celi, F. S. (2014). Functional thermogenic beige adipogenesis is inducible in human neck fat. Int. J. Obes. 38, 170–176. doi: 10.1038/ijo.2013.82
Lee, Y. H., Petkova, A. P., Mottillo, E. P., and Granneman, J. G. (2012). In vivo identification of bipotential adipocyte progenitors recruited by beta3-adrenoceptor activation and high-fat feeding. Cell Metab. 15, 480–491. doi: 10.1016/j.cmet.2012.03.009
Lee, Y. K., and Cowan, C. A. (2013). White to brite adipocyte transition and back again. Nat. Cell Biol. 15, 568–569. doi: 10.1038/ncb2776
Leushacke, M., and Barker, N. (2012). Lgr5 and Lgr6 as markers to study adult stem cell roles in self-renewal and cancer. Oncogene 31, 3009–3022. doi: 10.1038/onc.2011.479
Li, S. J., Yen, T. Y., Endo, Y., Klauzinska, M., Baljinnyam, B., Macher, B., et al. (2009). Loss-of-function point mutations and two-furin domain derivatives provide insights about R-spondin2 structure and function. Cell. Signal. 21, 916–925. doi: 10.1016/j.cellsig.2009.02.001
Lidell, M. E., Betz, M. J., Dahlqvist Leinhard, O., Heglind, M., Elander, L., Slawik, M., et al. (2013). Evidence for two types of brown adipose tissue in humans. Nat. Med. 19, 631–634. doi: 10.1038/nm.3017
Little, R. D., Carulli, J. P., Del Mastro, R. G., Dupuis, J., Osborne, M., Folz, C., et al. (2002a). A mutation in the LDL receptor-related protein 5 gene results in the autosomal dominant high-bone-mass trait. Am. J. Hum. Genet. 70, 11–19. doi: 10.1086/338450
Little, R. D., Recker, R. R., and Johnson, M. L. (2002b). High bone density due to a mutation in LDL-receptor-related protein 5. N. Engl. J. Med. 347, 943–944; author reply 943–944. doi: 10.1056/NEJM200209193471216
Liu, P., Ji, Y., Yuen, T., Rendina-Ruedy, E., DeMambro, V. E., Dhawan, S., et al. (2017). Blocking FSH induces thermogenic adipose tissue and reduces body fat. Nature 546, 107–112. doi: 10.1038/nature22342
Loh, N. Y., Neville, M. J., Marinou, K., Hardcastle, S. A., Fielding, B. A., Duncan, E. L., et al. (2015). LRP5 regulates human body fat distribution by modulating adipose progenitor biology in a dose- and depot-specific fashion. Cell Metab. 21, 262–272. doi: 10.1016/j.cmet.2015.01.009
Longo, K. A., Wright, W. S., Kang, S., Gerin, I., Chiang, S. H., Lucas, P. C., et al. (2004). Wnt10b inhibits development of white and brown adipose tissues. J. Biol. Chem. 279, 35503–35509. doi: 10.1074/jbc.M402937200
Ma, R. C., Hu, C., Tam, C. H., Zhang, R., Kwan, P., Leung, T. F., et al. (2013). Genome-wide association study in a Chinese population identifies a susceptibility locus for type 2 diabetes at 7q32 near PAX4. Diabetologia 56, 1291–1305. doi: 10.1007/s00125-013-2874-4
Macotela, Y., Emanuelli, B., Mori, M. A., Gesta, S., Schulz, T. J., Tseng, Y. H., et al. (2012). Intrinsic differences in adipocyte precursor cells from different white fat depots. Diabetes Metab. Res. Rev. 61, 1691–1699. doi: 10.2337/db11-1753
Mahajan, A., Go, M. J., Zhang, W., Below, J. E., Gaulton, K. J., Ferreira, T., et al. (2014). Genome-wide trans-ancestry meta-analysis provides insight into the genetic architecture of type 2 diabetes susceptibility. Nat. Genet. 46, 234–244. doi: 10.1038/ng.2897
Mani, A., Radhakrishnan, J., Wang, H., Mani, A., Mani, M. A., Nelson-Williams, C., et al. (2007). LRP6 mutation in a family with early coronary disease and metabolic risk factors. Science 315, 1278–1282. doi: 10.1126/science.1136370
Manolopoulos, K. N., Karpe, F., and Frayn, K. N. (2010). Gluteofemoral body fat as a determinant of metabolic health. Int. J. Obes. 34, 949–959. doi: 10.1038/ijo.2009.286
Marcelin, G., Ferreira, A., Liu, Y., Atlan, M., Aron-Wisnewsky, J., Pelloux, V., et al. (2017). A PDGFRα-mediated switch toward CD9high adipocyte progenitors controls obesity-induced adipose tissue fibrosis. Cell Metab. 25, 673–685. doi: 10.1016/j.cmet.2017.01.010
Mattei, J., Qi, Q., Hu, F. B., Sacks, F. M., and Qi, L. (2012). TCF7L2 genetic variants modulate the effect of dietary fat intake on changes in body composition during a weight-loss intervention. Am. J. Clin. Nutr. 96, 1129–1136. doi: 10.3945/ajcn.112.038125
McMahon, A. P., and Moon, R. T. (1989). Ectopic expression of the proto-oncogene int-1 in Xenopus embryos leads to duplication of the embryonic axis. Cell 58, 1075–1084. doi: 10.1016/0092-8674(89)90506-0
McMillan, M., and Kahn, M. (2005). Investigating Wnt signaling: a chemogenomic safari. Drug Discov. Today 10, 1467–1474. doi: 10.1016/S1359-6446(05)03613-5
Miller, R. K., Hong, J. Y., Munoz, W. A., and McCrea, P. D. (2013). Beta-catenin versus the other armadillo catenins: assessing our current view of canonical Wnt signaling. Prog. Mol. Biol. Transl. Sci. 116, 387–407. doi: 10.1016/B978-0-12-394311-8.00017-0
Miyabayashi, T., Teo, J. L., Yamamoto, M., McMillan, M., Nguyen, C., and Kahn, M. (2007). Wnt/beta-catenin/CBP signaling maintains long-term murine embryonic stem cell pluripotency. Proc. Natl. Acad. Sci. U.S.A. 104, 5668–5673. doi: 10.1073/pnas.0701331104
Molenaar, M., van de Wetering, M., Oosterwegel, M., Peterson-Maduro, J., Godsave, S., Korinek, V., et al. (1996). XTcf-3 transcription factor mediates beta-catenin-induced axis formation in Xenopus embryos. Cell 86, 391–399. doi: 10.1016/S0092-8674(00)80112-9
Morris, A. P., Voight, B. F., Teslovich, T. M., Ferreira, T., Segre, A. V., Steinthorsdottir, V., et al. (2012). Large-scale association analysis provides insights into the genetic architecture and pathophysiology of type 2 diabetes. Nat. Genet. 44, 981–990. doi: 10.1038/ng.2383
Nadler, S. T., Stoehr, J. P., Schueler, K. L., Tanimoto, G., Yandell, B. S., and Attie, A. D. (2000). The expression of adipogenic genes is decreased in obesity and diabetes mellitus. Proc. Natl. Acad. Sci. U.S.A. 97, 11371–11376. doi: 10.1073/pnas.97.21.11371
Nicklas, B. J., Cesari, M., Penninx, B. W. J. H., Kritchevsky, S. B., Ding, J. Z., Newman, A., et al. (2006). Abdominal obesity is an independent risk factor for chronic heart failure in older people. J. Am. Geriatr. Soc. 54, 413–420. doi: 10.1111/j.1532-5415.2005.00624.x
Niehrs, C. (2012). The complex world of WNT receptor signalling. Nat. Rev. Mol. Cell Biol. 13, 767–779. doi: 10.1038/nrm3470
Noordermeer, J., Klingensmith, J., Perrimon, N., and Nusse, R. (1994). dishevelled and armadillo act in the wingless signalling pathway in Drosophila. Nature 367, 80–83. doi: 10.1038/367080a0
Nusse, R., and Varmus, H. (2012). Three decades of Wnts: a personal perspective on how a scientific field developed. EMBO J. 31, 2670–2684. doi: 10.1038/emboj.2012.146
Nusse, R., and Varmus, H. E. (1982). Many tumors induced by the mouse mammary tumor virus contain a provirus integrated in the same region of the host genome. Cell 31, 99–109. doi: 10.1016/0092-8674(82)90409-3
Okerlund, N. D., and Cheyette, B. N. (2011). Synaptic Wnt signaling-a contributor to major psychiatric disorders? J. Neurodev. Disord. 3, 162–174. doi: 10.1007/s11689-011-9083-6
Orava, J., Nuutila, P., Noponen, T., Parkkola, R., Viljanen, T., Enerback, S., et al. (2013). Blunted metabolic responses to cold and insulin stimulation in brown adipose tissue of obese humans. Obesity 21, 2279–2287. doi: 10.1002/oby.20456
Palmer, B. F., and Clegg, D. J. (2015). The sexual dimorphism of obesity. Mol. Cell. Endocrinol. 402, 113–119. doi: 10.1016/j.mce.2014.11.029
Peifer, M., Sweeton, D., Casey, M., and Wieschaus, E. (1994). wingless signal and Zeste-white 3 kinase trigger opposing changes in the intracellular distribution of Armadillo. Development 120, 369–380.
Peng, W. C., de Lau, W., Forneris, F., Granneman, J. C., Huch, M., Clevers, H., et al. (2013). Structure of stem cell growth factor R-spondin 1 in complex with the ectodomain of its receptor LGR5. Cell Rep. 3, 1885–1892. doi: 10.1016/j.celrep.2013.06.009
Petrovic, N., Walden, T. B., Shabalina, I. G., Timmons, J. A., Cannon, B., and Nedergaard, J. (2010). Chronic peroxisome proliferator-activated receptor gamma (PPARgamma) activation of epididymally derived white adipocyte cultures reveals a population of thermogenically competent, UCP1-containing adipocytes molecularly distinct from classic brown adipocytes. J. Biol. Chem. 285, 7153–7164. doi: 10.1074/jbc.M109.053942
Phillips, L. K., and Prins, J. B. (2008). The link between abdominal obesity and the metabolic syndrome. Curr. Hypertens. Rep. 10, 156–164. doi: 10.1007/s11906-008-0029-7
Porfiri, E., Rubinfeld, B., Albert, I., Hovanes, K., Waterman, M., and Polakis, P. (1997). Induction of a beta-catenin-LEF-1 complex by wnt-1 and transforming mutants of beta-catenin. Oncogene 15, 2833–2839. doi: 10.1038/sj.onc.1201462
Rasola, A., Fassetta, M., De Bacco, F., D’Alessandro, L., Gramaglia, D., Di Renzo, M. F., et al. (2007). A positive feedback loop between hepatocyte growth factor receptor and beta-catenin sustains colorectal cancer cell invasive growth. Oncogene 26, 1078–1087. doi: 10.1038/sj.onc.1209859
Ravussin, E., and Tataranni, P. A. (1997). Dietary fat and human obesity. J. Am. Diet. Assoc. 97(Suppl. 7), S42–S46. doi: 10.1016/S0002-8223(97)00728-1
Rawson, J. B., Manno, M., Mrkonjic, M., Daftary, D., Dicks, E., Buchanan, D. D., et al. (2011). Promoter methylation of Wnt antagonists DKK1 and SFRP1 is associated with opposing tumor subtypes in two large populations of colorectal cancer patients. Carcinogenesis 32, 741–747. doi: 10.1093/carcin/bgr020
Rodeheffer, M. S., Birsoy, K., and Friedman, J. M. (2008). Identification of white adipocyte progenitor cells in vivo. Cell 135, 240–249. doi: 10.1016/j.cell.2008.09.036
Rosenwald, M., Perdikari, A., Rulicke, T., and Wolfrum, C. (2013). Bi-directional interconversion of brite and white adipocytes. Nat. Cell Biol. 15, 659–667. doi: 10.1038/ncb2740
Ross, S. E., Erickson, R. L., Gerin, I., DeRose, P. M., Bajnok, L., Longo, K. A., et al. (2002). Microarray analyses during adipogenesis: understanding the effects of Wnt signaling on adipogenesis and the roles of liver X receptor alpha in adipocyte metabolism. Mol. Cell. Biol. 22, 5989–5999. doi: 10.1128/MCB.22.16.5989-5999.2002
Ross, S. E., Hemati, N., Longo, K. A., Bennett, C. N., Lucas, P. C., Erickson, R. L., et al. (2000). Inhibition of adipogenesis by Wnt signaling. Science 289, 950–953. doi: 10.1126/science.289.5481.950
Rubinfeld, B., Albert, I., Porfiri, E., Fiol, C., Munemitsu, S., and Polakis, P. (1996). Binding of GSK3beta to the APC-beta-catenin complex and regulation of complex assembly. Science 272, 1023–1026. doi: 10.1126/science.272.5264.1023
Ruffner, H., Sprunger, J., Charlat, O., Leighton-Davies, J., Grosshans, B., Salathe, A., et al. (2012). R-Spondin potentiates Wnt/beta-catenin signaling through orphan receptors LGR4 and LGR5. PLoS One 7:e40976. doi: 10.1371/journal.pone.0040976
Saito, M., Okamatsu-Ogura, Y., Matsushita, M., Watanabe, K., Yoneshiro, T., Nio-Kobayashi, J., et al. (2009). High incidence of metabolically active brown adipose tissue in healthy adult humans: effects of cold exposure and adiposity. Diabetes Metab. Res. Rev. 58, 1526–1531. doi: 10.2337/db09-0530
Salans, L. B., Cushman, S. W., and Weismann, R. E. (1973). Studies of human adipose tissue. Adipose cell size and number in nonobese and obese patients. J. Clin. Invest. 52, 929–941. doi: 10.1172/JCI107258
Sanchez-Gurmaches, J., and Guertin, D. A. (2014). Adipocytes arise from multiple lineages that are heterogeneously and dynamically distributed. Nat. Commun. 5:4099. doi: 10.1038/ncomms5099
Sato, N., Meijer, L., Skaltsounis, L., Greengard, P., and Brivanlou, A. H. (2004). Maintenance of pluripotency in human and mouse embryonic stem cells through activation of Wnt signaling by a pharmacological GSK-3-specific inhibitor. Nat. Med. 10, 55–63. doi: 10.1038/nm979
Sato, T., van Es, J. H., Snippert, H. J., Stange, D. E., Vries, R. G., van den Born, M., et al. (2011). Paneth cells constitute the niche for Lgr5 stem cells in intestinal crypts. Nature 469, 415–418. doi: 10.1038/nature09637
Sato, T., Vries, R. G., Snippert, H. J., van de Wetering, M., Barker, N., Stange, D. E., et al. (2009). Single Lgr5 stem cells build crypt-villus structures in vitro without a mesenchymal niche. Nature 459, 262–265. doi: 10.1038/nature07935
Savic, D., Ye, H., Aneas, I., Park, S. Y., Bell, G. I., and Nobrega, M. A. (2011). Alterations in TCF7L2 expression define its role as a key regulator of glucose metabolism. Genome Res. 21, 1417–1425. doi: 10.1101/gr.123745.111
Schinner, S. (2009). Wnt-signalling and the metabolic syndrome. Horm. Metab. Res. 41, 159–163. doi: 10.1055/s-0028-1119408
Seale, P., Bjork, B., Yang, W., Kajimura, S., Chin, S., Kuang, S., et al. (2008). PRDM16 controls a brown fat/skeletal muscle switch. Nature 454, 961–967. doi: 10.1038/nature07182
Seale, P., Conroe, H. M., Estall, J., Kajimura, S., Frontini, A., Ishibashi, J., et al. (2011). Prdm16 determines the thermogenic program of subcutaneous white adipose tissue in mice. J. Clin. Invest. 121, 96–105. doi: 10.1172/JCI44271
Shabalina, I. G., Petrovic, N., de Jong, J. M., Kalinovich, A. V., Cannon, B., and Nedergaard, J. (2013). UCP1 in brite/beige adipose tissue mitochondria is functionally thermogenic. Cell Rep. 5, 1196–1203. doi: 10.1016/j.celrep.2013.10.044
Sharp, L. Z., Shinoda, K., Ohno, H., Scheel, D. W., Tomoda, E., Ruiz, L., et al. (2012). Human BAT possesses molecular signatures that resemble beige/brite cells. PLoS One 7:e49452. doi: 10.1371/journal.pone.0049452
Shi, H. F., Seeley, R. J., and Clegg, D. J. (2009). Sexual differences in the control of energy homeostasis. Front. Neuroendocrinol. 30, 396–404. doi: 10.1016/j.yfrne.2009.03.004
Shinoda, K., Luijten, I. H., Hasegawa, Y., Hong, H., Sonne, S. B., Kim, M., et al. (2015). Genetic and functional characterization of clonally derived adult human brown adipocytes. Nat. Med. 21, 389–394. doi: 10.1038/nm.3819
Siegfried, E., Chou, T. B., and Perrimon, N. (1992). wingless signaling acts through zeste-white 3, the Drosophila homolog of glycogen synthase kinase-3, to regulate engrailed and establish cell fate. Cell 71, 1167–1179. doi: 10.1016/S0092-8674(05)80065-0
Snijder, M. B., Dekker, J. M., Visser, M., Yudkin, J. S., Stehouwer, C. D. A., Bouter, L. M., et al. (2003). Larger thigh and hip circumferences are associated with better glucose tolerance: the Hoorn Study. Obes. Res. 11, 104–111. doi: 10.1038/oby.2003.18
Spalding, K. L., Arner, E., Westermark, P. O., Bernard, S., Buchholz, B. A., Bergmann, O., et al. (2008). Dynamics of fat cell turnover in humans. Nature 453, 783–787. doi: 10.1038/nature06902
Steinthorsdottir, V., Thorleifsson, G., Sulem, P., Helgason, H., Grarup, N., Sigurdsson, A., et al. (2014). Identification of low-frequency and rare sequence variants associated with elevated or reduced risk of type 2 diabetes. Nat. Genet. 46, 294–298. doi: 10.1038/ng.2882
Strand, M., and Micchelli, C. A. (2011). Quiescent gastric stem cells maintain the adult Drosophila stomach. Proc. Natl. Acad. Sci. U.S.A. 108, 17696–17701. doi: 10.1073/pnas.1109794108
Styrkarsdottir, U., Thorleifsson, G., Sulem, P., Gudbjartsson, D. F., Sigurdsson, A., Jonasdottir, A., et al. (2013). Nonsense mutation in the LGR4 gene is associated with several human diseases and other traits. Nature 497, 517–520. doi: 10.1038/nature12124
Sun, K., Kusminski, C. M., and Scherer, P. E. (2011). Adipose tissue remodeling and obesity. J. Clin. Invest. 121, 2094–2101. doi: 10.1172/JCI45887
Szabo, I., and Zoratti, M. (2017). Now UCP(rotein), now you don’t: UCP1 is not mandatory for thermogenesis. Cell Metab. 25, 761–762. doi: 10.1016/j.cmet.2017.03.013
Szenker-Ravi, E., Altunoglu, U., Leushacke, M., Bosso-Lefevre, C., Khatoo, M., Thi Tran, H., et al. (2018). RSPO2 inhibition of RNF43 and ZNRF3 governs limb development independently of LGR4/5/6. Nature 557, 564–569. doi: 10.1038/s41586-018-0118-y
Takamoto, I., Kubota, N., Nakaya, K., Kumagai, K., Hashimoto, S., Kubota, T., et al. (2014). TCF7L2 in mouse pancreatic beta cells plays a crucial role in glucose homeostasis by regulating beta cell mass. Diabetologia 57, 542–553. doi: 10.1007/s00125-013-3131-6
Takemaru, K. I., and Moon, R. T. (2000). The transcriptional coactivator CBP interacts with beta-catenin to activate gene expression. J. Cell Biol. 149, 249–254. doi: 10.1083/jcb.149.2.249
Tang, W., Zeve, D., Suh, J. M., Bosnakovski, D., Kyba, M., Hammer, R. E., et al. (2008). White fat progenitor cells reside in the adipose vasculature. Science 322, 583–586. doi: 10.1126/science.1156232
Tanko, L. B., Bagger, Y. Z., Alexandersen, P., Larsen, P. J., and Christiansen, C. (2003). Peripheral adiposity exhibits an independent dominant antiatherogenic effect in elderly women. Circulation 107, 1626–1631. doi: 10.1161/01.CIR.0000057974.74060.68
Tchkonia, T., Giorgadze, N., Pirtskhalava, T., Thomou, T., DePonte, M., Koo, A., et al. (2006). Fat depot-specific characteristics are retained in strains derived from single human preadipocytes. Diabetes Metab. Res. Rev. 55, 2571–2578. doi: 10.2337/db06-0540
Tchkonia, T., Lenburg, M., Thomou, T., Giorgadze, N., Frampton, G., Pirtskhalava, T., et al. (2007). Identification of depot-specific human fat cell progenitors through distinct expression profiles and developmental gene patterns. Am. J. Physiol. Endocrinol. Metab. 292, E298–E307. doi: 10.1152/ajpendo.00202.2006
Tchkonia, T., Tchoukalova, Y. D., Giorgadze, N., Pirtskhalava, T., Karagiannides, I., Forse, R. A., et al. (2005). Abundance of two human preadipocyte subtypes with distinct capacities for replication, adipogenesis, and apoptosis varies among fat depots. Am. J. Physiol. Endocrinol. Metab. 288, E267–E277. doi: 10.1152/ajpendo.00265.2004
Tchkonia, T., Thomou, T., Zhu, Y., Karagiannides, I., Pothoulakis, C., Jensen, M. D., et al. (2013). Mechanisms and metabolic implications of regional differences among fat depots. Cell Metab. 17, 644–656. doi: 10.1016/j.cmet.2013.03.008
ten Berge, D., Kurek, D., Blauwkamp, T., Koole, W., Maas, A., Eroglu, E., et al. (2011). Embryonic stem cells require Wnt proteins to prevent differentiation to epiblast stem cells. Nat. Cell Biol. 13, 1070–1075. doi: 10.1038/ncb2314
Teo, J. L., Ma, H., Nguyen, C., Lam, C., and Kahn, M. (2005). Specific inhibition of CBP/beta-catenin interaction rescues defects in neuronal differentiation caused by a presenilin-1 mutation. Proc. Natl. Acad. Sci. U.S.A. 102, 12171–12176. doi: 10.1073/pnas.0504600102
Tong, Y., Lin, Y., Zhang, Y., Yang, J., Zhang, Y., Liu, H., et al. (2009). Association between TCF7L2 gene polymorphisms and susceptibility to type 2 diabetes mellitus: a large Human Genome Epidemiology (HuGE) review and meta-analysis. BMC Med. Genet. 10:15. doi: 10.1186/1471-2350-10-15
Tran, T. T., Yamamoto, Y., Gesta, S., and Kahn, C. R. (2008). Beneficial effects of subcutaneous fat transplantation on metabolism. Cell Metab. 7, 410–420. doi: 10.1016/j.cmet.2008.04.004
van de Wetering, M., Cavallo, R., Dooijes, D., van Beest, M., van Es, J., Loureiro, J., et al. (1997). Armadillo coactivates transcription driven by the product of the Drosophila segment polarity gene dTCF. Cell 88, 789–799. doi: 10.1016/S0092-8674(00)81925-X
van Marken Lichtenbelt, W. D., Vanhommerig, J. W., Smulders, N. M., Drossaerts, J. M., Kemerink, G. J., Bouvy, N. D., et al. (2009). Cold-activated brown adipose tissue in healthy men. N. Engl. J. Med. 360, 1500–1508. doi: 10.1056/NEJMoa0808718
van Veelen, W., Le, N. H., Helvensteijn, W., Blonden, L., Theeuwes, M., Bakker, E. R. M., et al. (2011). beta-catenin tyrosine 654 phosphorylation increases Wnt signalling and intestinal tumorigenesis. Gut 60, 1204–1212. doi: 10.1136/gut.2010.233460
Vishvanath, L., MacPherson, K. A., Hepler, C., Wang, Q. A., Shao, M., Spurgin, S. B., et al. (2016). Pdgfrbeta+ mural preadipocytes contribute to adipocyte hyperplasia induced by high-fat-diet feeding and prolonged cold exposure in adult mice. Cell Metab. 23, 350–359. doi: 10.1016/j.cmet.2015.10.018
Vitali, A., Murano, I., Zingaretti, M. C., Frontini, A., Ricquier, D., and Cinti, S. (2012). The adipose organ of obesity-prone C57BL/6J mice is composed of mixed white and brown adipocytes. J. Lipid Res. 53, 619–629. doi: 10.1194/jlr.M018846
Voight, B. F., Scott, L. J., Steinthorsdottir, V., Morris, A. P., Dina, C., Welch, R. P., et al. (2010). Twelve type 2 diabetes susceptibility loci identified through large-scale association analysis. Nat. Genet. 42, 579–589. doi: 10.1038/ng.609
Voigt, A., Agnew, K., van Schothorst, E. M., Keijer, J., and Klaus, S. (2013). Short-term, high fat feeding-induced changes in white adipose tissue gene expression are highly predictive for long-term changes. Mol. Nutr. Food Res. 57, 1423–1434. doi: 10.1002/mnfr.201200671
Wagner, R. T., Xu, X., Yi, F., Merrill, B. J., and Cooney, A. J. (2010). Canonical Wnt/beta-catenin regulation of liver receptor homolog-1 mediates pluripotency gene expression. Stem Cells 28, 1794–1804. doi: 10.1002/stem.502
Wang, D., Huang, B., Zhang, S., Yu, X., Wu, W., and Wang, X. (2013). Structural basis for R-spondin recognition by LGR4/5/6 receptors. Genes Dev. 27, 1339–1344. doi: 10.1101/gad.219360.113
Wang, J., Liu, R., Wang, F., Hong, J., Li, X., Chen, M., et al. (2013). Ablation of LGR4 promotes energy expenditure by driving white-to-brown fat switch. Nat. Cell Biol. 15, 1455–1463. doi: 10.1038/ncb2867
Wang, Q. A., Tao, C., Gupta, R. K., and Scherer, P. E. (2013). Tracking adipogenesis during white adipose tissue development, expansion and regeneration. Nat. Med. 19, 1338–1344. doi: 10.1038/nm.3324
Wang, W., and Seale, P. (2016). Control of brown and beige fat development. Nat. Rev. Mol. Cell Biol. 17, 691–702. doi: 10.1038/nrm.2016.96
Wang, Y. F., Rimm, E. B., Stampfer, M. J., Willett, W. C., and Hu, F. B. (2005). Comparison of abdominal adiposity and overall obesity in predicting risk of type 2 diabetes among men. Am. J. Clin. Nutr. 81, 555–563. doi: 10.1093/ajcn/81.3.555
Wehrli, M., Dougan, S. T., Caldwell, K., O’Keefe, L., Schwartz, S., Vaizel-Ohayon, D., et al. (2000). arrow encodes an LDL-receptor-related protein essential for Wingless signalling. Nature 407, 527–530. doi: 10.1038/35035110
Weisberg, S. P., McCann, D., Desai, M., Rosenbaum, M., Leibel, R. L., and Ferrante, A. W. (2003). Obesity is associated with macrophage accumulation in adipose tissue. J. Clin. Invest. 112, 1796–1808. doi: 10.1172/Jci200319246
World Health Organisation [WHO] (2017). Obesity and Overweight. Available at: http://www.who.int/mediacentre/factsheets/fs311/en/
Wu, J., Bostrom, P., Sparks, L. M., Ye, L., Choi, J. H., Giang, A. H., et al. (2012). Beige adipocytes are a distinct type of thermogenic fat cell in mouse and human. Cell 150, 366–376. doi: 10.1016/j.cell.2012.05.016
Wu, J., Cohen, P., and Spiegelman, B. M. (2013). Adaptive thermogenesis in adipocytes: is beige the new brown? Genes Dev. 27, 234–250. doi: 10.1101/gad.211649.112
Xie, Y., Zamponi, R., Charlat, O., Ramones, M., Swalley, S., Jiang, X., et al. (2013). Interaction with both ZNRF3 and LGR4 is required for the signalling activity of R-spondin. EMBO Rep. 14, 1120–1126. doi: 10.1038/embor.2013.167
Yano, K., Saji, M., Hidaka, A., Moriya, N., Okuno, A., Kohn, L. D., et al. (1995). A new constitutively activating point mutation in the luteinizing hormone/choriogonadotropin receptor gene in cases of male-limited precocious puberty. J. Clin. Endocrinol. Metab. 80, 1162–1168. doi: 10.1210/jcem.80.4.7714085
Young, P., Arch, J. R., and Ashwell, M. (1984). Brown adipose tissue in the parametrial fat pad of the mouse. FEBS Lett. 167, 10–14. doi: 10.1016/0014-5793(84)80822-4
Zebisch, M., and Jones, E. Y. (2015). ZNRF3/RNF43–A direct linkage of extracellular recognition and E3 ligase activity to modulate cell surface signalling. Prog. Biophys. Mol. Biol. 118, 112–118. doi: 10.1016/j.pbiomolbio.2015.04.006
Zeve, D., Seo, J., Suh, J. M., Stenesen, D., Tang, W., Berglund, E. D., et al. (2012). Wnt signaling activation in adipose progenitors promotes insulin-independent muscle glucose uptake. Cell Metab. 15, 492–504. doi: 10.1016/j.cmet.2012.03.010
Keywords: Wnt/β-catenin signaling, hypertrophy, hyperplasia, beige adipocytes, depot-specific
Citation: Chen N and Wang J (2018) Wnt/β-Catenin Signaling and Obesity. Front. Physiol. 9:792. doi: 10.3389/fphys.2018.00792
Received: 19 April 2018; Accepted: 06 June 2018;
Published: 17 July 2018.
Edited by:
Anna Maria Giudetti, University of Salento, ItalyReviewed by:
Daniele Vergara, University of Salento, ItalyFiorella Biasi, Università degli Studi di Torino, Italy
Copyright © 2018 Chen and Wang. This is an open-access article distributed under the terms of the Creative Commons Attribution License (CC BY). The use, distribution or reproduction in other forums is permitted, provided the original author(s) and the copyright owner(s) are credited and that the original publication in this journal is cited, in accordance with accepted academic practice. No use, distribution or reproduction is permitted which does not comply with these terms.
*Correspondence: Jiqiu Wang, d2FuZ2pxQHNoc211LmVkdS5jbg==