- 1Department of Applied Physiology and Kinesiology, University of Florida, Gainesville, FL, United States
- 2Colegiado de Educação Física, Federal University of Vale do São Francisco, Petrolina, Brazil
Hyperthermia and dehydration can occur during exercise in hot environments. Nevertheless, whether elevations in extracellular osmolality contributes to the increased skeletal muscle tension, sarcolemmal injury, and oxidative stress reported in warm climates remains unknown. We simulated osmotic and heat stress, in vitro, in mouse limb muscles with different fiber compositions. Extensor digitorum longus (EDL) and soleus (SOL) were dissected from 36 male C57BL6J and mounted at optimal length in tissue baths containing oxygenated buffer. Muscles were stimulated with non-fatiguing twitches for 30 min. Four experimental conditions were tested: isotonic-normothermia (285 mOsm•kg-1 and 35∘C), hypertonic-normothermia (300 mOsm•kg-1 and 35∘C), isotonic-hyperthermia (285 mOsm•kg-1 and 41∘C), and hypertonic-hyperthermia (300 mOsm•kg-1 and 41∘C). Passive tension was recorded continuously. The integrity of the sarcolemma was determined using a cell-impermeable fluorescent dye and immunoblots were used for detection of protein carbonyls. In EDL muscles, isotonic and hypertonic-hyperthermia increased resting tension (P < 0.001). Whereas isotonic-hyperthermia increased sarcolemmal injury in EDL (P < 0.001), this effect was absent in hypertonic-hyperthermia. Similarly, isotonic-hyperthermia elevated protein carbonyls (P = 0.018), a response not observed with hypertonic-hyperthermia. In SOL muscles, isotonic-hyperthermia also increases resting tension (P < 0.001); however, these effects were eliminated in hypertonic-hyperthermia. Unlike EDL, there were no effects of hyperthermia and/or hyperosmolality on sarcolemmal injury or protein carbonyls. Osmolality selectively modifies skeletal muscle response to hyperthermia in this model. Fast-glycolytic muscle appears particularly vulnerable to isotonic-hyperthermia, resulting in elevated muscle tension, sarcolemmal injury and protein oxidation; whereas slow-oxidative muscle exhibits increased tension but no injury or protein oxidation under the conditions and duration tested.
Introduction
Hyperthermia is a common feature of exercise in hot environments and is accompanied by sweat-induced dehydration when fluid losses are not adequately matched by fluid replacement. As sweat is normally hypotonic in relation to plasma, sweat losses are associated with a hypertonic plasma (Wendt et al., 2007). Several secondary factors related to both hyperthermia and dehydration may contribute to muscle injury in the heat. For instance, hyperthermia per se can induce increases in passive tension (Oliver et al., 2008), a response often attributed to elevations in intracellular calcium (Ca2+) release from the sarcoplasmic reticulum (SR) (Hill, 1972; van der Poel and Stephenson, 2002, 2007). Increases in extracellular osmolality associated with dehydration can also induce increases in muscle tension (Clausen et al., 1979; Farhat et al., 2018) by affecting muscle lattice structure and Ca2+ release (Clausen et al., 1979; Millman, 1998; Cleary et al., 2005). Calcium can influence cross bridge alignment, optimum myosin-actin interactions, and presumably susceptibility to stretch-induced damage leading to sarcolemmal injury. Extensive sarcolemmal injury is an underlying feature of exertional rhabdomyolysis, which is of concern in athletes as well as in active duty military personnel (Clarkson, 2006; Cleary et al., 2011; Rawson et al., 2017). The incidence of rhabdomyolysis is 5 to 7 times greater during summer months (Armed Forces Health Surveillance Bureau, 2017), suggesting that elevated temperatures and disorders of fluid balance may be contributing factors. However, the extent of osmotic and heat stress involvement and the mechanisms behind their influences are not well understood.
Hyperthermia stimulates the production of skeletal muscle reactive oxygen species (ROS) (Zuo et al., 2000). In humans, exercise-induced ROS are attenuated when elevations in body temperature are suppressed (Laitano et al., 2010; Hillman et al., 2011; Quindry et al., 2013; King et al., 2016). The production of ROS could contribute to injury of the sarcolemmal membrane, cytoskeleton and DNA (Powers and Jackson, 2008; Reid, 2016). ROS have been reported to contribute to SR-mediated Ca2+ release in this setting (van der Poel and Stephenson, 2007; Oliver et al., 2008), which can result in secondary mechanisms of injury through activation of proteases and phospholipases. The molecular sources of ROS produced during hyperthermia remain poorly understood, but ROS may be a result of skeletal muscle requirements for adapting to osmotic challenges (King et al., 2016), direct effects of hyperthermia (Zuo et al., 2000), or dehydration (Eisner et al., 2006; Laitano et al., 2012; King et al., 2016), or the heat-induced activation of eicosanoid pathways (Calderwood et al., 1989; Zuo et al., 2004). Carbonyl groups are produced on protein side chains when they are oxidized by ROS. Due to their high chemical stability, protein carbonyls have been used as biomarkers of oxidative stress (Dalle-Donne et al., 2003), including in exercise-hyperthermia settings (Souza-Silva et al., 2016), which makes them an attractive biomarker of protein oxidation in skeletal muscle ex vivo preparations.
In this study we tested the hypothesis that elevations in extracellular osmolality and temperature, within physiological ranges experienced by skeletal muscle during exercise in the heat (González-Alonso et al., 1997; Parkin et al., 1999; Laitano et al., 2012), contribute to changes in skeletal muscle resting tension. Secondary objectives were to evaluate differences in susceptibility to sarcolemmal injury between fast and slow-fiber dominant muscles and to determine whether protein oxidation was affected by these stressors.
Materials and Methods
Mice Care and Handling
The study protocol was approved by the University of Florida Institutional Animal Care and Use Committee. Thirty-six male adult C57BL/6J mice (22–26 g) were obtained from Jackson Laboratories (Bar Harbor, ME, United States) and housed at the University of Florida on a 12/12 h light-dark cycle with the dark cycle between 07:00 PM and 07:00 AM. The temperature and humidity of the room was maintained at 19–23°C and approximately 40–60%, respectively. Animals were brought from the animal care facility to the laboratory one-day prior to the experiment in their own cages, remaining on a 12/12 light cycle, in order to adapt to the laboratory and to recover from the stress of transport.
Skeletal Muscle Excision and Resting Tension
Prior to amputation of both legs, mice were anesthetized with 3% isoflurane and skin was removed with forceps. Mice were euthanized immediately after the amputation. Legs were immediately transferred to a dish containing freshly prepared, modified isotonic Krebs-Henseleit (KH) buffer described in detail in Table 1. The buffer was continuously bubbled with 95%O2/5%CO2. Soleus (SOL) of the right leg and extensor digitorum longus (EDL) of the left leg were quickly removed under a magnifying microscope and mounted in water-jacketed tissue baths containing 4 ml of either isotonic (285 mOsm•kg-1) or hypertonic (300 mOsm•kg-1) KH buffer with 95%O2 and 5%CO2. We used 4-0 silk suture to tie the proximal tendon to the force transducer (GrassFT03). The distal tendon was attached to a glass rod using a loop of a suture. Initially, muscles were kept resting in the baths at optimal length for 10 min period under isotonic buffer and 35°C temperature. Thereafter, we switched the connectors of the water circulation unit to 41°C for hyperthermia conditions or simply maintained at 35°C for control conditions and we switched the buffer to fresh hypertonic or isotonic depending on the condition studied. For hyperthermia experiments, the buffer added to the bath at the time of temperature increase (after 10 min of resting equilibration) was pre heated to 42°C to avoid delays required to achieve the desired experimental temperature. Buffer osmolality was manipulated by increasing sodium chloride (NaCl) content of the buffer as described in Table 1. Buffer osmolality was confirmed using a vapor pressure micro osmometer (Vapro 5520, Wescor Biomedical Systems). EDL and SOL muscles were chosen because they are known to express a more pronounced glycolytic and oxidative phenotypes, respectively (Augusto et al., 2004).
Muscles were electrically stimulated to perform 30 min of non-fatiguing twitch contractions at a frequency of 5 contractions/min (Grass S88 Square Pulse Stimulator), while maintained at initial length. Resting tension was recorded (DasyLab Software) throughout the twitch contraction protocol by having the muscles attached to a strain gauge force transducer. After the stimulation protocol, muscles were exposed to 0.15% wt/v of procion orange MX2R (Sigma Aldrich, Ref. 311391) in isotonic KH buffer with 95% O2/5% CO2 at 35°C for 45 min, followed by a 15-min rinse with fresh buffer. Muscles were immediately embedded in a medium for frozen tissue specimens to ensure optimal cutting temperature (OCT, Sakura Tissue Tek) and frozen with liquid nitrogen in cold 2-methylbutane.
Skeletal Muscle Histology and Microscopy Assessments
Soleus (SOL) and EDL samples were cut in sections ranging from 10–30 μm by using a cryostat (Leica Biosystems, model CM3050 S) set at -20°C. Slices were placed on glass slides and were washed with phosphate saline buffer to remove OCT. We used an anti-fade mounting medium (Vectashield – H-1000) to prevent photobleaching. Slides were kept at 4°C until microscopy analysis. Multiple images of each section were recorded with an epifluorescence microscope (Olympus DP71) using standard filter settings for fluorescein. The numbers of cells containing procion orange MX2R were delineated as the percentage of total number of cells in the whole field and were analyzed by two independent, trained raters who were blinded to the experimental conditions.
SDS-PAGE and Western Blot
Skeletal muscle samples were washed in phosphate saline buffer and subsequently homogenized using a Kontes Duall Homogenizer in cell lysis buffer (No. 9803; Cell Signaling Technology) containing 20 mM Tris–HCl (pH 7.5), 150 mM NaCl, 1 mM Na2EDTA, 1 mM EGTA, 1% Triton-X, 2.5 mM sodium pyrophosphate, 1 mM-glycerophosphate 1 mM Na3PO4, 1 μg/ml leupeptin, and protease and phosphatase inhibitor cocktail (no. 5872; Cell Signaling Technology). We performed a detergent compatible assay (DC Assay, Bio-Rad Laboratories) to determine the total protein content of the lysates. The lysates were mixed 1:1 with 2X Laemmli sample buffer (Bio-Rad Laboratories). We loaded 20 μg/lane into 4–20% stain-free TGX gels (Bio-Rad Laboratories) and performed electrophoresis at 200 V for 50 min with the chamber buried in ice. Proteins were transferred to a nitrocellulose membrane at 100 mA for 18 h at 4°C. The membranes were scanned with Revert Total Protein Stain (Li-COR, Lincoln, NE, United States) at the 700 nm channel to determine the total amount of protein transferred. We then washed the membranes to remove the revert staining, according to manufacturer instructions, and blocked the membrane immediately after by using Li-COR PBS Blocking Buffer (Li-COR, Lincoln, NE, United States) for 1 h at room temperature.
To determine protein carbonyls, we used the Oxi-Select Protein Carbonyl Immunoblot kit (STa-308, Cell Biolabs) following the manufacturer recommendations. In brief, to promote protein derivatization, we incubated the membranes with TBS + 20% methanol for 5 min and then washed in 2N HCL for 5 min. We then incubated the membranes in 2,4-Dinitrophenylhydrazine (DNPH) for 5 min in the dark. Thereafter, the membranes were washed 3X in 2N HCL and 5× in 50% methanol, each wash was for 5 min. Membranes were further incubated with anti-DNPH primary antibody diluted 1:100 in Li-COR PBS Blocking Buffer for 1 h at room temperature. After 3 washes with TBS-T, the membranes were finally incubated with anti-rabbit secondary antibody diluted 1:20000 in Li-COR PBS Blocking Buffer for another hour. We scanned the membranes using an Odyssey Infrared Imaging system (LI-COR CLX) at 800 nm channel. The immunoblot signal was normalized to the total protein signal measured in the corresponding membrane lanes. These procedures are consistent with recent recommendations for data analysis of Western Blots using fluorescence methods (Eaton et al., 2013; Murphy and Lamb, 2013; Bass et al., 2017).
Statistical Analysis
Data are reported as mean ± standard deviation, unless otherwise stated. Data distribution was determined by using the Shapiro–Wilk test. We used repeated measures two-way ANOVA to compare data over time and independent two-way ANOVA to compare means among treatments. Tukey’s post hoc was used to confirm where the differences occurred over time and among groups. To test the hypothesis, we predetermined the 95% level of confidence as the minimum criterion to denote a statistical difference (p ≤ 0.05). All analyses were made using SAS JMP Pro v.12.0 and GraphPad Prism v. 7.0 software.
Results
Isotonic hyperthermia (41°C) increased resting tension in EDL [F(6,192) = 22.24, P < 0.001] over time. There was an increase in tension from baseline starting at 20 min (0 min vs. 20 min, P = 0.007) after the onset of hyperthermia. The tension remained elevated at 25 min (0 min vs. 25 min, P < 0.001) and 30 min (0 vs. 30 min, P < 0.001). The highest tension achieved by EDL muscles at 30 min with isotonic hyperthermia was higher than the tension achieved with isotonic (P = 0.008) and hypertonic normothermia (P = 0.023) as shown in Figure 1A. Similarly, hypertonic hyperthermia also increased tension over time in EDL. The tension increased at 20 min (P = 0.001) and remained elevated at 25 min (P < 0.001) and 30 min (P < 0.001). The highest tension achieved at 30 min with hypertonic hyperthermia was higher than the tension recorded for muscles under isotonic (P < 0.01) and hypertonic normothermia (P = 0.01) at the same time point.
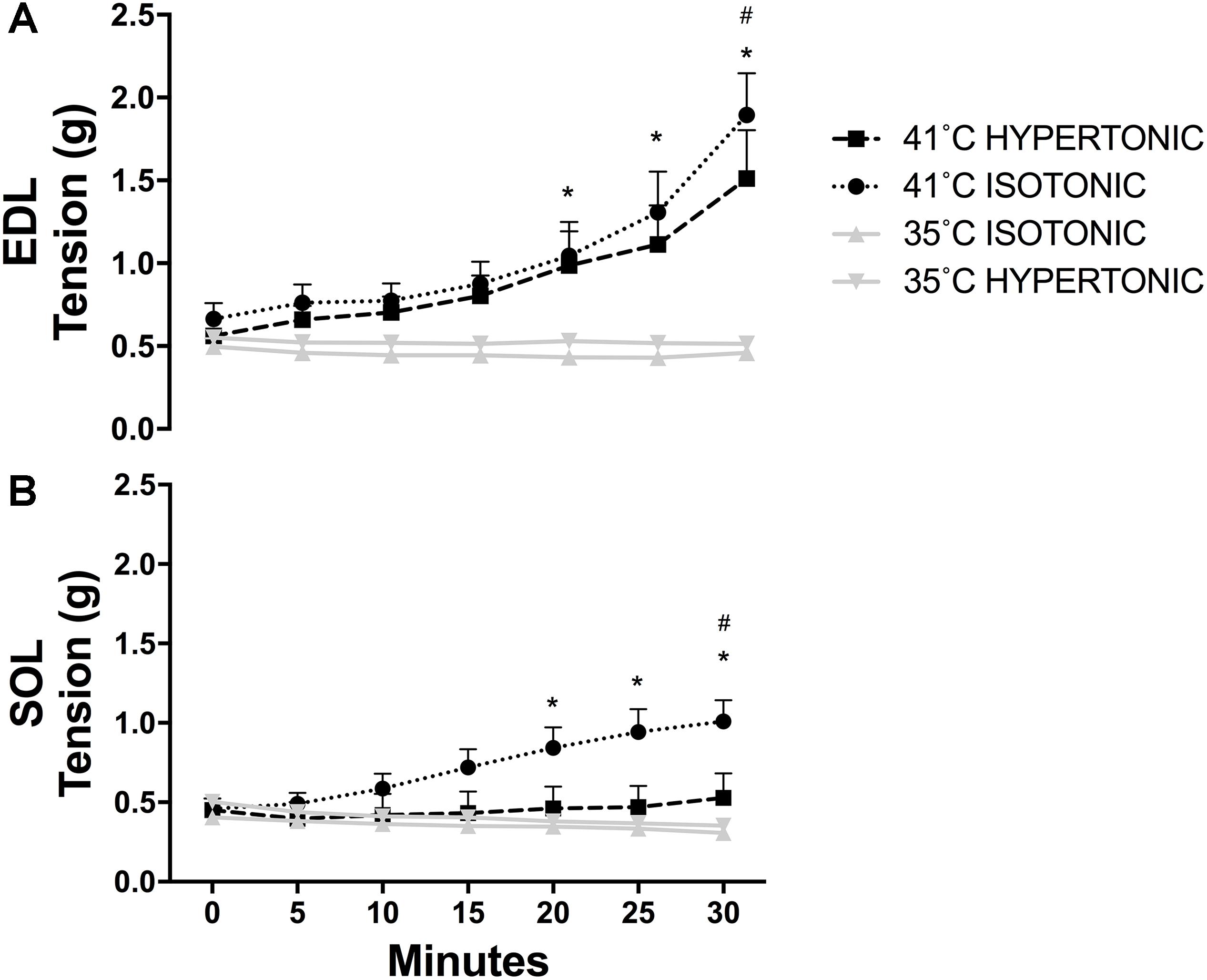
FIGURE 1. Skeletal muscle resting tension recorded throughout twitch contractions for EDL (A) ∗P < 0.05 over time, #P < 0.05 in comparison to 35°C isotonic and hypertonic; and SOL (B) ∗P < 0.05 over time, #P < 0.05 in comparison to 35°C isotonic, hypertonic, and 41°C hypertonic. N = 8 for 35°C and N = 10 for 41°C conditions per group. Repeated measures two-way ANOVA was used over time and independent two-way ANOVA was used to compare different experimental conditions.
In SOL muscles, only isotonic hyperthermia caused an increase in tension over time [F(6,186) = 3.73, P < 0.001]. Resting tension began to increase at 20 min (0 min vs. 20 min, P < 0.001) and remained elevated at 25 min (0 min vs. 25 min, P < 0.001) and 30 min (0 min vs. 30 min, P < 0.001). Interestingly, there was no increase in resting tension with hypertonic hyperthermia. The tension observed for SOL at 30 min with isotonic hyperthermia was higher than the tension recorded with both isotonic (P = 0.001) and hypertonic normothermia (P = 0.003) and higher than the tension recorded at 30 min with hypertonic hyperthermia (P = 0.022) as can be seen in Figure 1B.
Isotonic hyperthermia caused sarcolemmal injury in EDL muscles as assessed by the presence of cell-impermeable dye in individual muscle fibers [F(3,18) = 10.42, P < 0.001]. There was an increase in the percentage of fluorescent dye-positive fibers with isotonic hyperthermia in comparison to both isotonic (P < 0.001) and hypertonic normothermia (P = 0.002). Interestingly, isotonic hyperthermia showed a higher percentage of dye-positive fibers than hypertonic hyperthermia (P = 0.013), as seen in Figure 2A. There was no effect of any experimental treatment on SOL muscle permeability, which suggests a greater tolerance of SOL muscle to sarcolemma injury induced by hyperthermia and changes in osmolality, Figure 2B.
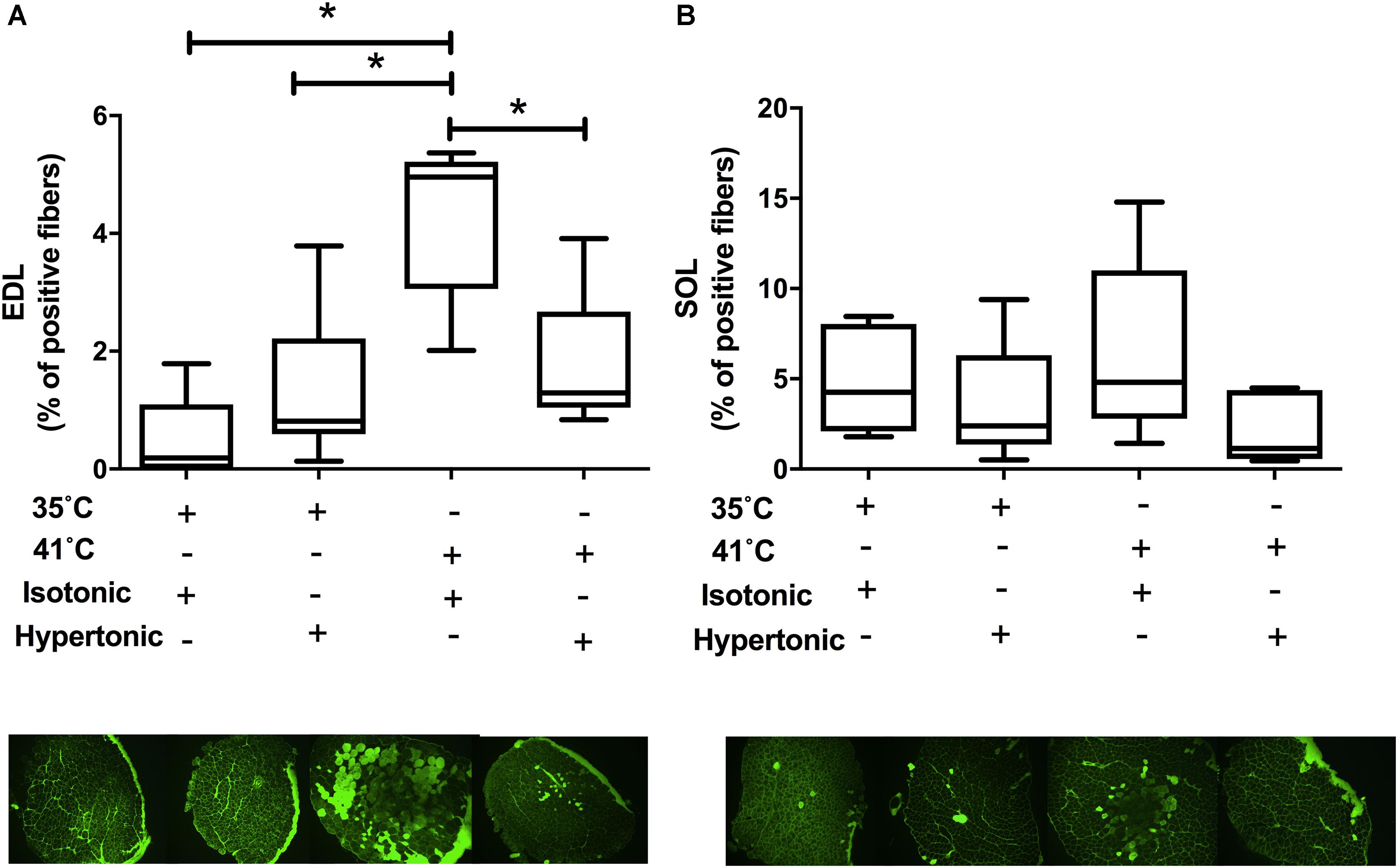
FIGURE 2. Procion orange leakage into skeletal muscle fibers under isotonic and hypertonic conditions with and without heat stress (N = 8 for 35°C and N = 10 for 41°C conditions). Pictures are from representative muscles at respective experimental condition. EDL muscle results are displayed in panel A and SOL data in panel B. Independent two-way ANOVA was used to compare different experimental conditions. Data are median of percentage with interquartile range. ∗P < 0.05.
Isotonic hyperthermia increased protein carbonyls in EDL muscles F(3,18) = 3.87, P = 0.018. While there was an increase in protein carbonyls with isotonic hyperthermia in comparison to isotonic normothermia (p = 0.009), no differences were observed with hypertonic normothermia or hyperthermia as seen in Figure 3, panel A. In SOL muscles, hyperthermia had no effects on protein carbonyls regardless of the osmolality employed.
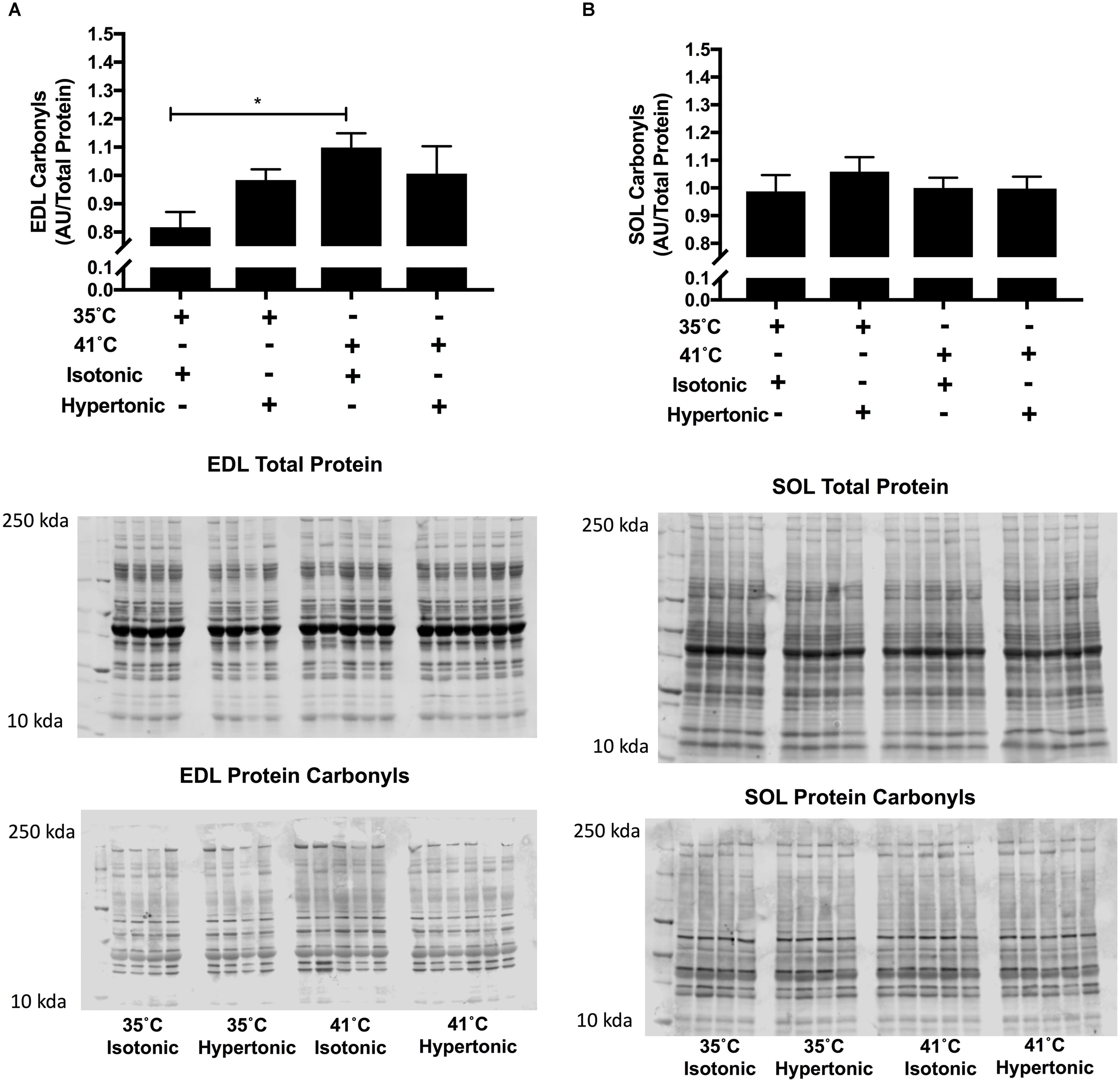
FIGURE 3. Protein carbonyls for EDL (A) and SOL (B) immunoblot relative to total protein (N = 8 for 35°C and N = 10 for 41°C conditions, ∗P < 0.05). Independent two-way ANOVA was used to compare different experimental conditions. Data are arbitrary units from fluorescent signal intensity divided by total protein.
Discussion
The present study demonstrates that elevations in temperature, typical of skeletal muscle temperatures during exercise in the heat (Parkin et al., 1999), resulted in significant elevations in passive tension in both fast glycolytic and slow oxidative skeletal muscles. Our main finding was that the elevation in passive force was prevented in slow oxidative muscles when hyperthermia was accompanied by increased extracellular osmolality. In fast glycolytic muscle, there was evidence for both sarcolemmal injury and increased protein carbonyls that were not seen in slow oxidative muscle with hyperthermia. Additionally, hypertonic hyperthermia attenuated sarcolemmal injury and elevations in protein carbonyls in fast glycolytic muscle. In neither skeletal muscle type, did extracellular osmolality alone cause increase in tension, sarcolemmal injury or increases in protein carbonyls.
Our findings of increased skeletal muscle resting tension with hyperthermia are consistent with previous studies exploring the impact of temperature on skeletal muscle physiology (Hill, 1972; Oliver et al., 2008). There is consensus that the changes in skeletal muscle resting tension with hyperthermia involve altered Ca2+ homeostasis (van der Poel and Stephenson, 2002, 2007). These changes in Ca2+ homeostasis with hyperthermia are triggered, at least in part, by changes in fiber volume as hyperthermia is thought to participate in skeletal muscle fiber swelling responses (Usher-Smith et al., 2009; King et al., 2016). Swelling can increase cation permeability through the sarcolemmal membrane, which leads to extracellular Ca2+ influx into skeletal muscle fibers (King et al., 2016). Hyperthermia has also been shown to increase intracellular Ca2+ leakage from the SR in rodents (van der Poel and Stephenson, 2007). Whether the increased resting muscle tension caused by hyperthermia arises from the impact of elevated Ca2+ on the contractile (i.e., actin-myosin cross bridges) or elastic (i.e., titin) elements of the skeletal muscle remains unknown and warrants further experimentation. Titin is a potential candidate due to its known sensitivity to Ca2+ (Labeit et al., 2003) and its participation in force transmission at the Z line and resting tension in the I band region of striated muscles (Wang et al., 1991; Itoh-Satoh et al., 2002).
This is the first study to demonstrate that elevations in extracellular osmolality offset the impact of hyperthermia, even though the specific responses appear to be unique to fiber phenotype (i.e., slow oxidative vs. fast glycolytic). The increase in extracellular osmolality could counteract the impact in hyperthermia-induced skeletal muscle fiber swelling by activation of regulatory volume response pathways in slow oxidative skeletal muscle fibers (Usher-Smith et al., 2009; King et al., 2016), whereas fast glycolytic fibers may have less sensitivity or lower abundance of proteins involved in this regulatory volume response. This resonates with many previous investigations that have reported greater sensitivity to most forms of injury in fast twitch muscle, as reviewed in (Tiidus, 2008). Nevertheless, follow-up studies employing a wider range of osmolality and temperature are required to demonstrate when this interaction starts to display protective or dysfunctional features in fast-glycolytic skeletal muscle fibers.
Isotonic hyperthermia induced sarcolemmal injury in fast glycolytic muscles and this response was attenuated by hypertonic hyperthermia. Our data for sarcolemmal injury, using a cell-impermeable dye, suggests that fast-twitch muscles are more susceptible to heat induced-injury, but the degree of injury is attenuated when osmolality is elevated. Slow-twitch muscle fibers did not display significant injury with hyperthermia, which might indicate a more resistant phenotype to this stressor (Tiidus, 2008). In alignment with our present findings, a recent study in rodents also demonstrated greater sensitivity to osmolality changes induced by severe water deprivation in fast glycolytic than in slow oxidative muscles (Farhat et al., 2018).
We observed an increase in hyperthermia-induced skeletal muscle protein carbonyls in fast-twitch muscles. This is consistent with previous findings demonstrating that heat stress increases ROS formation in animal and human models. For example, rat diaphragm generates elevated intracellular and extracellular superoxide within a few minutes of exposure to 42°C (Zuo et al., 2000). Mouse EDL fibers exposed to 40°C demonstrate a leaky SR Ca2+ pump attributed to the effects of ROS formation (van der Poel and Stephenson, 2007). Besides causing an increase in tension, elevations in intracellular Ca2+ concentration can activate proteases and phospholipases that are known to increase ROS production and induce further injury (Powers and Jackson, 2008). Hyperthermia, with and without dehydration, exacerbates markers of ROS production in the circulation of resting and exercising humans (Laitano et al., 2010, 2012). In the present study, we observed that the increase in protein carbonyls was halted when hyperthermia was superimposed with extracellular hyperosmolality. These results suggest that physiological elevations in extracellular osmolality might be necessary to counteract the potentially detrimental effects of hyperthermia (e.g., augmented protein oxidation) in skeletal muscles of predominantly glycolytic fiber type compositions.
The source of hyperthermia-induced ROS that cause protein oxidation in skeletal muscle remains a matter of debate. A potential source of ROS in skeletal muscle during heat/osmotic disturbances are the NAD(P)H oxidases enzyme family (Ferreira and Laitano, 2016). Although osmotic disturbances have been associated with increased NAD(P)H oxidase activity in skeletal muscle (Martins et al., 2008), a causal role of hyperthermia upon this enzyme family remains to be established. A possible explanation for the different protein carbonyls response observed between fast and slow muscles in the present study could be that slow oxidative muscles contain a much greater abundance of antioxidant defense mechanisms compared to fast glycolytic muscles (Lawler et al., 1993) and even though the same underlying cell volume homeostatic pathways may be active, they may not result in significant protein oxidation or membrane injury, but this statement requires further investigation.
Our in vitro preparation was designed to mimic physiological alterations observed during an exercise session in the heat with moderate sweat-induced dehydration (e.g., within 4% reductions in body mass). Studies have reported increases in skeletal muscle temperature from 36°C to 41°C (Parkin et al., 1999) and in blood osmolality from ∼280 to 300 mOsm•kg-1 in humans exercising in the heat and eliciting 4% dehydration (González-Alonso et al., 1997; Laitano et al., 2012). Therefore, our in vitro preparation was consistent with hyperthermia and hyperosmolality observed in moderately dehydrated humans exercising in the heat. Severe heat injury is generally associated with accompanying effects of intense muscle contractions, particularly eccentric contractions. We did not incorporate maximal contractions into this experiment in order to isolate the effects of heat and osmolality. We conjecture that the subtler changes we observe here, set muscles up for much more intense injury during eccentric contractions associated with exercise. Recent experiments in humans combining mild heat exposure preceding eccentric contractions have shown no influence of pre-heating to 40°C on the extent of injury (Castellani et al., 2016). However, limitations inherent to the penetration, duration, and intensity of the heat stimulus make it still an open question and warrants further investigation.
Among the disadvantages of skeletal muscles in vitro preparations is the excessive PO2 exposure to peripheral fibers and the diminished O2 perfusion in the fibers located at the core. In this model, O2 diffusion is the transport method of O2 delivery and while more peripheral fibers are adequately, or even excessively, exposed to O2, fibers located in the core of the muscle bundle can experience lower PO2, presumably leading to hypoxia. This is a concern in preparations using hyperthermia since skeletal muscle oxygen consumption is increased by heat and because the PO2 in the bath is constant, PO2 is decreased in the core of the muscle. This has been debated before and while preparations using rat muscles demonstrate a hypoxic core in sections, mice skeletal muscles are small enough to allow adequate O2 diffusion from the bath to the fibers, particularly when in a near resting-state. To address this concern we have employed the equation proposed by Barclay (2005) where the distance from the surface of the muscle to the radial location where PO2 is 0 for different skeletal muscle types. In both EDL and SOL muscles this distance is negligible within the temperature range used in the present study.
Since the responses of hyperosmolality on hyperthermia-induced skeletal muscle alterations were largely unknown, the set of experiments performed were not designed to define the conclusive mechanisms behind the apparent attenuation caused by hyperosmolality on heat-induced increases in resting tension in slow-twitch muscles nor to explain the apparent protective feature of hyperosmolality against oxidative stress and sarcolemmal damage in fast glycolytic fibers. On the other hand, the present results set the ground for future studies to explore the mechanisms behind the responses herein described as well as to establish whether these findings can be extended to human skeletal muscle physiology. For instance, at a more molecular level, whether Ca2+ homeostasis is indeed the reason why hyperosmolality attenuates the rise in tension seen in skeletal muscle warrants further investigation. Likewise, the source of oxidants associated with heat-induced protein oxidation and fiber type specificity, whether it comes primarily from mitochondrial sources or changes in NADPH oxidase activity remains to be explored.
Can our results have implications for human performance and susceptibility to muscle injury during exercise in hot environments? It is difficult to translate directly to in vivo human conditions because it is challenging to predict precisely what the osmolality would be in the interstitial space surrounding an actively contracting muscle, due to the continual flux of K+ and other solutes. Also, we manipulated osmolality by increasing NaCl content in the extracellular buffer while all other solutes were kept under the same concentration as in isotonic buffer, this differs from increases in extracellular osmolality seen in hypohydration states where usually all solutes increase somewhat evenly with fluid losses. Our results highlight and support further precautions against the consequences of overhydration during exercise in the heat, beyond the usual discussion of electrolyte imbalances. We have proposed that muscle cell swelling during intense exercise in the heat may be a significant source of ROS formation and injury (King et al., 2016) and therefore, in addition to the suggestions of deleterious effects of hyponatremia on muscle injury (Cairns and Hew-Butler, 2016), there may be additional risks of muscle injury associated with cell swelling.
Conclusion
In summary, we demonstrate for the first time that osmolality selectively modifies mouse skeletal muscle response to hyperthermia in this model. Specifically, superimposing hyperosmolality to hyperthermia attenuates resting tension in slow twitch skeletal muscle and prevents sarcolemma injury and protein oxidation in fast twitch muscles. Our results are consistent with slow twitch muscles being more resistant to hyperthermia than fast twitch ones regarding protein oxidation and sarcolemma injury.
Author Contributions
OL, LF, and TC conceived the study. OL, LS, AM, and KM performed muscle dissections and ex vivo experiments. OL wrote the manuscript. All authors edited and approved the final version of the manuscript.
Funding
OL was supported by a postdoctoral fellowship from the Brazilian Council for the Development of Science and Technology (CNPq # 249094/2013-4). This study was supported by the BK and Betty Stevens Endowed Professorship to TC.
Conflict of Interest Statement
The authors declare that the research was conducted in the absence of any commercial or financial relationships that could be construed as a potential conflict of interest.
References
Armed Forces Health Surveillance Bureau. (2017). Update: exertional rhabdomyolysis, active component, U.S. Armed Forces, 2012-2016. MSMR 24, 14–18.
Augusto, V., Padovani, C. R., and Campos, G. E. R. (2004). Skeletal muscule fiber types in C57BL6J mice. Braz. J. Morphol. Sci. 21, 89–94.
Barclay, C. J. (2005). Modelling diffusive O2 supply to isolated preparations of mammalian skeletal and cardiac muscle. J. Muscle Res. Cell Motil. 26, 225–235. doi: 10.1007/s10974-005-9013-x
Bass, J. J., Wilkinson, D. J., Rankin, D., Phillips, B. E., Szewczyk, N. J., Smith, K., et al. (2017). An overview of technical considerations for Western blotting applications to physiological research. Scand. J. Med. Sci. Sports 27, 4–25. doi: 10.1111/sms.12702
Cairns, R. S., and Hew-Butler, T. (2016). Proof of concept: hypovolemic hyponatremia may precede and augment creatine kinase elevations during an ultramarathon. Eur. J. Appl. Physiol. 116, 647–655. doi: 10.1007/s00421-015-3324-4
Calderwood, S. K., Bornstein, B., Farnum, E. K., and Stevenson, M. A. (1989). Heat shock stimulates the release of arachidonic acid and the synthesis of prostaglandins and leukotriene B4 in mammalian cells. J. Cell. Physiol. 141, 325–333. doi: 10.1002/jcp.1041410214
Castellani, J. W., Zambraski, E. J., Sawka, M. N., and Urso, M. L. (2016). Does high muscle temperature accentuate skeletal muscle injury from eccentric exercise? Physiol. Rep. 4:e12777. doi: 10.14814/phy2.12777
Clarkson, P. M. (2006). Case report of exertional rhabdomyolysis in a 12-year-old boy. Med. Sci. Sports Exerc. 38, 197–200. doi: 10.1249/01.mss.0000183478.12106.04
Clausen, T., Dahl-Hansen, A. B., and Elbrink, J. (1979). The effect of hyperosmolarity and insulin on resting tension and calcium fluxes in rat soleus muscle. J. Physiol. 292, 505–526.
Cleary, M. A., Sadowski, K. A., Lee, S. Y.-C., Miller, G. L., and Nichols, A. W. (2011). Exertional rhabdomyolysis in an adolescent athlete during preseason conditioning: a perfect storm. J. Strength Cond. Res. 25, 3506–3513. doi: 10.1519/JSC.0b013e318216302f
Cleary, M. A., Sweeney, L. A., Kendrick, Z. V., and Sitler, M. R. (2005). Dehydration and symptoms of delayed-onset muscle soreness in hyperthermic males. J. Athl. Train. 40, 288–297.
Dalle-Donne, I., Rossi, R., Giustarini, D., Milzani, A., and Colombo, R. (2003). Protein carbonyl groups as biomarkers of oxidative stress. Clin. Chim. Acta 329, 23–38. doi: 10.1016/S0009-8981(03)00003-2
Eaton, S. L., Roche, S. L., Hurtado, M. L., Oldknow, K. J., Farquharson, C., Gillingwater, T. H., et al. (2013). Total protein analysis as a reliable loading control for quantitative fluorescent western blotting. PLoS One 8:e72457. doi: 10.1371/journal.pone.0072457
Eisner, V., Criollo, A., Quiroga, C., Olea-Azar, C., Santibañez, J. F., Troncoso, R., et al. (2006). Hyperosmotic stress-dependent NFkappaB activation is regulated by reactive oxygen species and IGF-1 in cultured cardiomyocytes. FEBS Lett. 580, 4495–4500. doi: 10.1016/j.febslet.2006.07.029
Farhat, F., Grosset, J. F., and Canon, F. (2018). Water deprivation decreases strength in fast twitch muscle in contrast to slow twitch muscle in rat. Acta Physiol. 224:e13072. doi: 10.1111/apha.13072
Ferreira, L. F., and Laitano, O. (2016). Regulation of NADPH oxidases in skeletal muscle. Free Radic. Biol. Med. 98, 18–28. doi: 10.1016/j.freeradbiomed.2016.05.011
González-Alonso, J., Mora-Rodríguez, R., Below, P. R., and Coyle, E. F. (1997). Dehydration markedly impairs cardiovascular function in hyperthermic endurance athletes during exercise. J. Appl. Physiol. 82, 1229–1236. doi: 10.1152/jappl.1997.82.4.1229
Hill, D. K. (1972). Resting tension and the form of the twitch of rat skeletal muscle at low temperature. J. Physiol. 221, 161–171. doi: 10.1113/jphysiol.1972.sp009746
Hillman, A. R., Vince, R. V., Taylor, L., McNaughton, L., Mitchell, N., and Siegler, J. (2011). Exercise-induced dehydration with and without environmental heat stress results in increased oxidative stress. Appl. Physiol. Nutr. Metab. 36, 698–706. doi: 10.1139/h11-080
Itoh-Satoh, M., Hayashi, T., Nishi, H., Koga, Y., Arimura, T., Koyanagi, T., et al. (2002). Titin mutations as the molecular basis for dilated cardiomyopathy. Biochem. Biophys. Res. Commun. 291, 385–393. doi: 10.1006/bbrc.2002.6448
King, M. A., Clanton, T. L., and Laitano, O. (2016). Hyperthermia, dehydration, and osmotic stress: unconventional sources of exercise-induced reactive oxygen species. Am. J. Physiol. Regul. Integr. Comp. Physiol. 310, R105–R114. doi: 10.1152/ajpregu.00395.2015
Labeit, D., Watanabe, K., Witt, C., Fujita, H., Wu, Y., Lahmers, S., et al. (2003). Calcium-dependent molecular spring elements in the giant protein titin. Proc. Natl. Acad. Sci. 100, 13716–13721. doi: 10.1073/pnas.2235652100
Laitano, O., Kalsi, K. K., Pearson, J., Lotlikar, M., Reischak-Oliveira, A., and González-Alonso, J. (2012). Effects of graded exercise-induced dehydration and rehydration on circulatory markers of oxidative stress across the resting and exercising human leg. Eur. J. Appl. Physiol. 112, 1937–1944. doi: 10.1007/s00421-011-2170-2
Laitano, O., Kalsi, K. K., Pook, M., Oliveira, A. R., and González-Alonso, J. (2010). Separate and combined effects of heat stress and exercise on circulatory markers of oxidative stress in euhydrated humans. Eur. J. Appl. Physiol. 110, 953–960. doi: 10.1007/s00421-010-1577-5
Lawler, J. M., Powers, S. K., Visser, T., Van Dijk, H., Kordus, M. J., and Ji, L. L. (1993). Acute exercise and skeletal muscle antioxidant and metabolic enzymes: effects of fiber type and age. Am. J. Physiol. 265, R1344–R1350. doi: 10.1152/ajpregu.1993.265.6.R1344
Martins, A. S., Shkryl, V. M., Nowycky, M. C., and Shirokova, N. (2008). Reactive oxygen species contribute to Ca2 + signals produced by osmotic stress in mouse skeletal muscle fibres. J. Physiol. 586, 197–210. doi: 10.1113/jphysiol.2007.146571
Millman, B. M. (1998). The filament lattice of striated muscle. Physiol. Rev. 78, 359–391. doi: 10.1152/physrev.1998.78.2.359
Murphy, R. M., and Lamb, G. D. (2013). Important considerations for protein analyses using antibody based techniques: down-sizing Western blotting up-sizes outcomes. J. Physiol. 591, 5823–5831. doi: 10.1113/jphysiol.2013.263251
Oliver, S. R., Wright, V. P., Parinandi, N., and Clanton, T. L. (2008). Thermal tolerance of contractile function in oxidative skeletal muscle: no protection by antioxidants and reduced tolerance with eicosanoid enzyme inhibition. Am. J. Physiol. Regul. Integr. Comp. Physiol. 295, R1695–R1705. doi: 10.1152/ajpregu.90429.2008
Parkin, J. M., Carey, M. F., Zhao, S., and Febbraio, M. A. (1999). Effect of ambient temperature on human skeletal muscle metabolism during fatiguing submaximal exercise. J. Appl. Physiol. 86, 902–908. doi: 10.1152/jappl.1999.86.3.902
Powers, S. K., and Jackson, M. J. (2008). Exercise-induced oxidative stress: cellular mechanisms and impact on muscle force production. Physiol. Rev. 88, 1243–1276. doi: 10.1152/physrev.00031.2007
Quindry, J., Miller, L., McGinnis, G., Kliszczewiscz, B., Slivka, D., Dumke, C., et al. (2013). Environmental temperature and exercise-induced blood oxidative stress. Int. J. Sport Nutr. Exerc. Metab. 23, 128–136.
Rawson, E. S., Clarkson, P. M., and Tarnopolsky, M. A. (2017). Perspectives on exertional rhabdomyolysis. Sports Med. 47, 33–49. doi: 10.1007/s40279-017-0689-z
Reid, M. B. (2016). Reactive oxygen species as agents of fatigue. Med. Sci. Sports Exerc. 48, 2239–2246. doi: 10.1249/MSS.0000000000001006
Souza-Silva, A. A., Moreira, E., de Melo-Marins, D., Schöler, C. M., de Bittencourt, P. I. H., and Laitano, O. (2016). High intensity interval training in the heat enhances exercise-induced lipid peroxidation, but prevents protein oxidation in physically active men. Temperature 3, 167–175. doi: 10.1080/23328940.2015.1132101
Usher-Smith, J. A., Huang, C. L.-H., and Fraser, J. A. (2009). Control of cell volume in skeletal muscle. Biol. Rev. Camb. Philos. Soc. 84, 143–159. doi: 10.1111/j.1469-185X.2008.00066.x
van der Poel, C., and Stephenson, D. G. (2002). Reversible changes in Ca2+ - activation properties of rat skeletal muscle exposed to elevated physiological temperatures. J. Physiol. 544, 765–776.
van der Poel, C., and Stephenson, D. G. (2007). Effects of elevated physiological temperatures on sarcoplasmic reticulum function in mechanically skinned muscle fibers of the rat. Am. J. Physiol. Cell Physiol. 293, C133–C141. doi: 10.1152/ajpcell.00052.2007
Wang, K., McCarter, R., Wright, J., Beverly, J., and Ramirez-Mitchell, R. (1991). Regulation of skeletal muscle stiffness and elasticity by titin isoforms: a test of the segmental extension model of resting tension. Proc. Natl. Acad. Sci. 88, 7101–7105. doi: 10.1073/pnas.88.16.7101
Wendt, D., van Loon, L. J. C., Lichtenbelt, W. D., and van, M. (2007). Thermoregulation during exercise in the heat: strategies for maintaining health and performance. Sports Med. 37, 669–682.
Zuo, L., Christofi, F. L., Wright, V. P., Bao, S., and Clanton, T. L. (2004). Lipoxygenase-dependent superoxide release in skeletal muscle. J. Appl. Physiol. 97, 661–668. doi: 10.1152/japplphysiol.00096.2004
Keywords: heat stress, dehydration, oxidative stress, osmotic stress, rhabdomyolysis
Citation: Laitano O, Sheikh LH, Mattingly AJ, Murray KO, Ferreira LF and Clanton TL (2018) Osmolality Selectively Offsets the Impact of Hyperthermia on Mouse Skeletal Muscle in vitro. Front. Physiol. 9:1496. doi: 10.3389/fphys.2018.01496
Received: 10 July 2018; Accepted: 03 October 2018;
Published: 31 October 2018.
Edited by:
Stefano Biressi, University of Trento, ItalyReviewed by:
Han-Zhong Feng, Wayne State University School of Medicine, United StatesLars R. McNaughton, Edge Hill University, United Kingdom
Copyright © 2018 Laitano, Sheikh, Mattingly, Murray, Ferreira and Clanton. This is an open-access article distributed under the terms of the Creative Commons Attribution License (CC BY). The use, distribution or reproduction in other forums is permitted, provided the original author(s) and the copyright owner(s) are credited and that the original publication in this journal is cited, in accordance with accepted academic practice. No use, distribution or reproduction is permitted which does not comply with these terms.
*Correspondence: Orlando Laitano, b3JsYW5kby5sYWl0YW5vQHVmbC5lZHU=