- 1Institue of Sport Sciences, University of Lausanne, Lausanne, Switzerland
- 2Applied Signal Processing Group, Ecole Polytechnique Fédérale de Lausanne, Lausanne, Switzerland
- 3Altitude Research Center, Department of Medicine, University of Colorado Anschutz Medical Campus, Aurora, CO, United States
- 4Human Physiology and Nutrition, University of Colorado Colorado Springs, Colorado Springs, CO, United States
- 5Department of Human Physiology, University of Oregon, Eugene, OR, United States
Introduction: Baroreflex sensitivity (BRS) is essential to ensure rapid adjustment to variations in blood pressure (BP). Spontaneous baroreflex function can be assessed using continuous recordings of blood pressure. The goal of this study was to compare four methods for BRS quantification [the sequence, Bernardi’s (BER), frequency and transfer function methods] to identify the most consistent method across an extreme range of conditions: rest and exercise, in normoxia, hypoxia, hypocapnia, and hypercapnia.
Methods: Using intra-radial artery BP in young healthy participants, BRS was calculated and compared using the four methods in normoxia, acute and chronic hypoxia (terrestrial altitude of 5,260 m) in hypocapnia (hyperventilation), hypercapnia (rebreathing) and during ramp exercise to exhaustion.
Results: The sequence and BER methods for BRS estimation showed good agreement during the resting and exercise protocols, whilst the ultra- and very-low frequency bands of the frequency and transfer function methods were more discrepant. Removing respiratory frequency from the blood pressure traces affected primarily the sequence and BER methods and occasionally the frequency and transfer function methods.
Discussion/Conclusion: The sequence and BER methods contained more respiratory related information than the frequency and transfer function methods, indicating that the former two methods predominantly rely on respiratory effects of BRS. BER method is recommended because it is the easiest to compute and even though it tends to overestimate BRS compared to the sequence method, it is consistent with the other methods, whilst its interquartile range is the smallest.
Introduction
Blood pressure (BP) must be tightly regulated to guarantee adequate perfusion of organs, especially the brain. BP homeostasis is challenged in numerous situations such as postural change, exercise, and exposure to environmental stresses like heat and altitude. Long and short-term regulation (few heart beats or minutes to lifetime) of BP is achieved by modifications of the vascular tone whilst short-term regulation (few heart beats or minutes) is achieved by heart rate modification (Messerli et al., 2007; Michelini et al., 2015). Altitude induces hypoxemia, leading to vasodilation and BP reduction (Bourdillon et al., 2018). Conversely, hypocapnia induces vasoconstriction and therefore increases BP. Baroreflex is a vital mechanism that triggers variations in heart rate to attenuate variations in BP. The peripheral chemoreceptor-mediated sympathetic excitation in hypoxia results in an increase in baroreflex set point (Halliwill and Minson, 1982) and a decrease in gain (Bernardi et al., 1998; Cooper et al., 2005). During dynamic exercise (walking, running, cycling), cardiac output increases and systemic vascular resistances change (vasodilation in working muscle, vasoconstriction of renal and gastro-intestinal vascular beds) leading to an increased BP, which triggers the baroreflex (Michelini et al., 2015). Yet, mean BP only increases moderately, because there is a resetting of the baroreflex to increased arterial BP as a function of exercise intensity (Bevegård and Shepherd, 1966; Eckberg et al., 1975; Pawelczyk and Raven, 1989; Joyner, 2006) from rest to 75% of maximum oxygen consumption (Potts et al., 1993; Papelier et al., 1994).
Baroreflex sensitivity (BRS) is a measure of baroreflex function. The faster the response to small changes in BP, the more sensitive the autonomic control of BP and the higher the BRS. Originally, BRS quantification gained acceptance by injecting a pressor drug (alpha-adrenergic phenylephrine) which resulted in baroreflex increased vagal efferent activity, lengthening the beat-to-beat intervals, followed by a reduction in sympathetic vasoconstrictor tone within a few seconds delay (La Rovere et al., 2008). There are several components of the baroreflex (cardiac and peripheral). Our focus here is on the cardiac BRS. It is evaluated as the slope of the regression line fitting the sequence of the increases in beat-to-beat intervals with the increases in systolic arterial pressure elicited by the drug. This is called the sequence method, which allows a direct interpretation of the causal link between blood pressure and heart rate changes (Parati et al., 1988).
Spontaneous BRS assessment relies on the same principle but without the injection of a pressor drug. BRS is quantified using any spontaneous consistent sequences of BP vs. beat-to-beat-interval that occur. In the absence of a gold standard (e.g., comparison to the modified Oxford method), we use the sequence method for comparative purposes because it is the most commonly used method for baroreflex analysis. Whilst this method works well in healthy people, the lack of such sequences to fit may be a problem under certain circumstances such as hypoxia or pathological conditions. Therefore, other methods have been developed, known as Bernardi’s ratio of the standard deviations (BER), the frequency method and the transfer function method (Pinna and Maestri, 2002; Bernardi et al., 2010).
The frequency and the transfer function methods both rely on the frequency domain analysis of the blood pressure trace, which is made of the ultra low-, very low-, low- and high- frequency ranges. The low-frequency range has previously been used to describe cardiac BRS during exercise (Fisher et al., 2009) whilst blood pressure fluctuations in the high-frequency range were attributed to respiratory frequency (Frederiks et al., 2000). Interpretation of the ultra- and very low-frequency ranges regarding BRS remains unclear.
Previous studies explored consistency of the various methods (Bernardi et al., 2010) but to the best of our knowledge, there has been no head-to-head comparison of these methods to identify which one is the most consistent across a range of various stressors. Thus, the intent of this study was to compare these four different methods across an extreme range of conditions, rest and exercise in normoxia, hypoxia and hypercapnia during the acclimatization phase to 5,260 m of the AltitudeOmics project (Subudhi et al., 2014), and to formulate recommendations on their use.
Materials and Methods
The Materials and Methods section has been published previously (Bourdillon et al., 2018), it is reproduced here for readers’ convenience.
Subject Recruitment and Screening
Twenty-one young, healthy, sea-level residents, average age 21, range 19–23 years, were recruited in the region of Eugene, OR, United States (130 m). Physical examinations and the U.S. Army Physical Fitness Test [APFT, push-ups, sit-ups, and a 3.2-km run (Knapik, 1989)] were performed to characterize health and fitness status. Exclusion criteria included being born at > 1,500 m, having traveled to altitudes > 1,000 m in the past 3 months (including air travel), using prescription medications, smoking, being pregnant or lactating, having a history of serious head injury (loss of consciousness), self or familial history of migraine, known hematologic or cardiovascular abnormality (e.g., sickle cell trait, cardiac arrhythmia), pulmonary function or diffusion capacity for carbon monoxide < 90% of predicted, or failure to meet the minimal age/gender standards for the APFT (Knapik, 1989). BRS results using the sequence method have been published previously (Bourdillon et al., 2018). There is no further redundancy between the present data analysis and other publications from the AltitudeOmics project.
Ethics Statement
The study was approved by the Institutional Review Boards of the University of Colorado and the University of Oregon and by the Human Research Protection Office of the US Department of Defense and was performed according to the Declaration of Helsinki. The subjects were informed about the procedures and risks and gave written consent prior to participation.
Experimental Design
Familiarization with the experimental procedures included a graded exercise test up to exhaustion (O2peak test) to assess the aerobic fitness of the subjects and to ensure that the inclusion criteria were met. After familiarization, the subjects underwent experimental trials near sea level (SL, 130 m; barometric pressure 749 mmHg) and on the 1st (ALT1) and 16th day (ALT16) at 5,260 m (barometric pressure 406 mmHg). For each subject, all ALT measurements were carried out around the same time of day to minimize any confounding effects of the circadian rhythm. During ascent (from 1,525 to 5,260 m) the subjects breathed supplemental oxygen (2 L/min, nasal cannula or mask). Administration of O2 was ceased just before ALT1 measurements. This ensured standardized acute exposure at ALT1 and minimized any influence of early acute mountain sickness (AMS) during ALT1. Likewise, no symptoms of AMS were observed at ALT16 because of successful acclimatization. An overview of the entire experimental design of the AltitudeOmics project may be found elsewhere (Subudhi et al., 2014).
Experimental Protocol
Before entering the experimental room, the subjects laid down in a room dedicated to the insertion of an arterial catheter (20–22 gauge) into a radial artery (Arrow International, Reading, PA, United States) under local anesthesia (2% lidocaine). Arterial blood pressure was measured using this catheter and a calibrated pressure transducer (Deltran®, Utah Medical, Midvale, UT, United States) connected to an amplifier (BP amp, ADInstruments, Colorado Springs, CO, United States). After ∼30 min of instrumentation, the subjects underwent the resting protocol, followed by the exercise protocol.
Resting Protocol
Following 10–15 min of quiet rest in a seated position, each experimental testing session consisted of (1) instrumentation; (2) 10 min in room air for baseline; (3) 10 min with end-tidal partial CO2 pressure (PETCO2) clamped at 40 mmHg (cl-40); (4) 3 min of voluntary hyperventilation to lower PETCO2 to ∼20 mmHg (HVE); and (5) a modified rebreathing test (REB, details below). Stages 3 to 5 of the protocol were carried out in a background of hyperoxia (end-tidal partial O2 pressure [PETO2] ∼250 mmHg).
Resting Protocol Experimental Setup
Throughout the protocol, the subjects sat upright and breathed through a mouthpiece attached to a two-way, non-rebreathing valve (Hans-Rudolph 2700, Hans-Rudolph, Shawnee, KS, United States). The breathing circuit allowed switching from room air to either an end-tidal clamping system or a rebreathing system. The end-tidal clamping setup used in the present study was a modified version of the system previously described by Olin et al. (2012). The setup allowed stabilizing PETCO2 at 40 mmHg by constantly adding a varying portion of CO2 into the inspired gas mixture. Throughout the end-tidal PCO2 clamping, we maintained PETO2 at ∼250 mmHg by titrating 50% (balanced with N2) or 100% O2 into the inspiratory reservoir, at SL and ALT, respectively.
Modified Rebreathing Method
The rebreathing bag was filled with gas to achieve inspired PCO2 and PO2 of 0 and 300 mmHg, respectively, at each altitude. Subjects were instructed to hyperventilate for 3 min (part 4) to lower and then maintain PETCO2 at 20 mmHg at both sea level and 5,260 m (in a background PETO2 of ∼250 mmHg). Participants were then switched to the rebreathing bag and following two initial deep breaths to mix the gas from the bag with that in the respiratory system, they were instructed to breathe ad libitum (part 5). The rebreathing tests were terminated when PETCO2 reached 50 mmHg, PETO2 dropped below 200 mmHg, or the subject reached the end of their hypercapnia tolerance.
Exercise Protocol
Participants were seated on an electrically braked cycle ergometer (Velotron Elite, Racermate, Seattle, WA, United States). The protocol began with a three-min resting baseline in pedaling position on the ergometer. The subjects then completed four 3-min stages at 70, 100, 130 and 160 Watts, followed by 15 Watts/min increments until they could no longer maintain pedaling > 50 rpm despite strong verbal encouragement. No specific pedaling frequency was required. Maximal power output (Watts) was calculated as: work rate of last stage completed + [(work rate increment) × (time into final stage/duration of stage, in seconds)] (Subudhi et al., 2008). Participants breathed room air throughout the exercise protocol.
Measurements
Data Acquisition
All analog data were sampled and recorded at 200 Hz on a personal computer for off-line analysis (Powerlab 16/30; ADInstruments, Bella Vista, NSW, Australia).
Sequence Method
Heart beat-to-beat time intervals were extracted directly from BP recordings. Initially, systolic blood pressure (SBP) peaks were extracted from the BP waveform. Time of systolic peak represented occurrence of heartbeat. However, low sampling rates (<250 Hz) may produce jitter in the estimation of peaks (Merri et al., 1990; Task Force, 1996). For instance, at 200 Hz the highest time resolution is within a confidence interval of 5 ms. To refine the location of heartbeats and the SBP values, a second order polynomial was fitted to each extracted peak using four neighbor samples from the BP waveform (two immediately before and two immediately after). Heartbeats were selected as the location of the maximum of the interpolation polynomial. Furthermore, SBP values were updated as the maximum in their corresponding polynomial. Finally, the inter-beat intervals (IBIs) were created as the interval between successive peaks.
The sequence method is based on the identification of at least three consecutive beats in which a strictly defined increase (or decrease) in SBP is followed by a strictly defined increase (or decrease) in the IBI. Fixed minimal changes were considered for SBP and IBI to validate a sequence. Specifically, a minimum change of 1 mmHg between two consecutive SBP values or of 5 ms for IBI was set as the smallest increase (or decrease) in a sequence (Bernardi et al., 2010). Furthermore, the minimum correlation coefficient between changes in SBP and IBI to validate a sequence was set at 0.85. Finally, a minimum number of five sequences was set to validate a BRS estimate. The sensitivity of the baroreflex is obtained by computing the slope of the regression line between changes in SBP and IBI. All computed slopes are finally averaged to obtain the BRS-Seq. The advantage of this method is that the computations are automatic and standardized, which virtually eliminates intra- and inter-subject measurement variability (La Rovere et al., 2008). The baroreflex nature of these spontaneous beat-to-beat interval-systolic pressure sequences was demonstrated by showing that in cats the number of sequences markedly dropped (−89%) after the surgical opening of the baroreflex loop by sinoaortic denervation (Di Rienzo et al., 2001).
Bernardi’s Ratio of the Standard Deviations
In this method, the ratio between the standard deviation of the beat-to-beat intervals and the standard deviation of SBP is calculated (BRS-BER) (Bernardi et al., 2010). All other BRS methods use “selected” variability of the BP trace (i.e., selected relationship between RR intervals and systolic BP or specific frequency bands) whilst BER uses “overall” variability. Yet, the BER method can be used for the determination of BRS. Such a non-specific approach must include non-BRS variability. Heart rate and blood pressure fluctuation are not exclusively determined by the arterial baroreflex. Nevertheless, BER showed good agreement with other standard methods (Maestri et al., 1998; Eckberg, 2009; Bernardi et al., 2010). A possible explanation for this apparent discrepancy between evidence and theory is that in a closed-loop system any cardiovascular change induced by different mechanisms (thus even if not initially originated by the baroreflex) is sensed and influenced by the baroreceptors. Finally, this method is free from the mathematical constraints present in the other methods, and therefore much easier to standardize.
Frequency Method
For the spectral method (BRS-F), the IBI and BP time series were regularly resampled at 4 Hz. Then, the square root of the ratio of the autoregressive spectral powers of beat-to-beat intervals and SBP series in the ultra low- (0–0.0033 Hz BRS-FULF) very low- (0.0033–0.05 BRS-FVLF), low- (0.05 Hz–0.15 Hz BRS-FLF), and high- (0.15–0.4 Hz BRS-FHF) frequency range, were calculated, respectively. This computation was performed when coherence between beat-to-beat intervals and SBP was greater than 0.5 in the corresponding passband.
Transfer Function Method
In the transfer function method (BRS-TF), resampling of time series was identical to BRS-F and BRS was calculated as the average value of SBP-beat-to-beat cross-spectrum divided by the SBP spectrum in the same frequency ranges as BRS-F.
In all cases, BRS was assessed using a 90-s window immediately before the termination of each resting intervention and exercise stage.
Influence of Respiration
Baroreflex sensitivity depends on SBP and IBI fluctuations. However, respiration affects both SBP and IBI via mechanisms that are not necessarily of baroreflex origin. Whether respiratory sinus arrhythmia (RSA) is due to a central mechanism or to the baroreflex mechanism is still debated (Eckberg, 2009; Karemaker, 2009). Previous work attempted to separate the effects of the baroreflex and respiration using metronome-guided respiration and adaptive filtering of the data (Tiinanen et al., 2008), showing that the respiratory rate, but not the pattern (i.e., duration of inspiration and expiration phases) is of primary importance (Paprika et al., 2014). Therefore, to control for a potential effect of hyperventilation on BRS, the respiration frequency was extracted via an autoregressive power spectral density (PSD) estimation of the IBI. The PSD was estimated with an autoregressive order of 50, and the respiration frequency was extracted as that of the largest peak in the range [0.1–0.4] Hz. This IBI frequency band was larger than that used in the conventional heart rate variability (HRV) high frequency band, i.e., [0.15–0.4] Hz, as respiration frequency can migrate to the low frequency band [0.04–0.15] Hz.
Statistical Analysis
Figures 1–3 display Tukey boxplots of the data in which the horizontal line inside the boxes denotes the median, whilst the upper and lower lines of the boxes represent the 75th and 25th percentiles, respectively. The upper and lower whiskers denote the highest and lowest data points within the 1.5 inter quartile range (Frigge et al., 1989). This corresponds to approximately ± 2.7σ and 99.3% coverage of the data (McGill et al., 1978). Outliers were marked using the + sign. Smaller inter quartile range on the figures denote good consistency across the participants when making recommendations on which method should be used. Bias, reproducibility coefficient and coefficient of variations are available in the Supplementary Material.
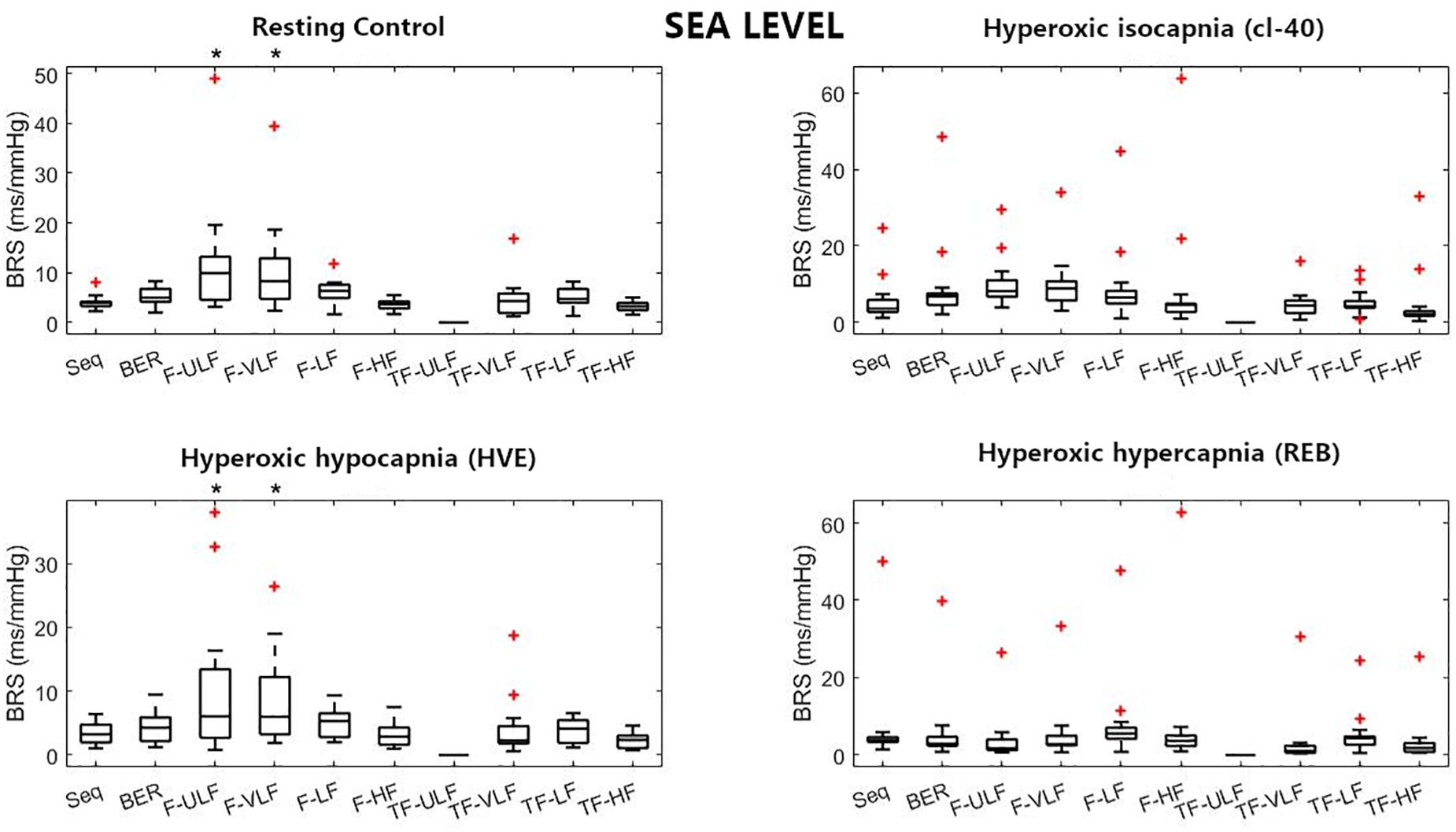
Figure 1. Baroreflex sensitivity (BRS) estimation for the resting protocol at sea level. cl-40, clamp 40 mmHg of inspired CO2; HVE, hyperventilation; REB, rebreathing up to PACO2 of 50 mmHg. +Denotes outliers. ∗Different from Seq (p < 0.05).
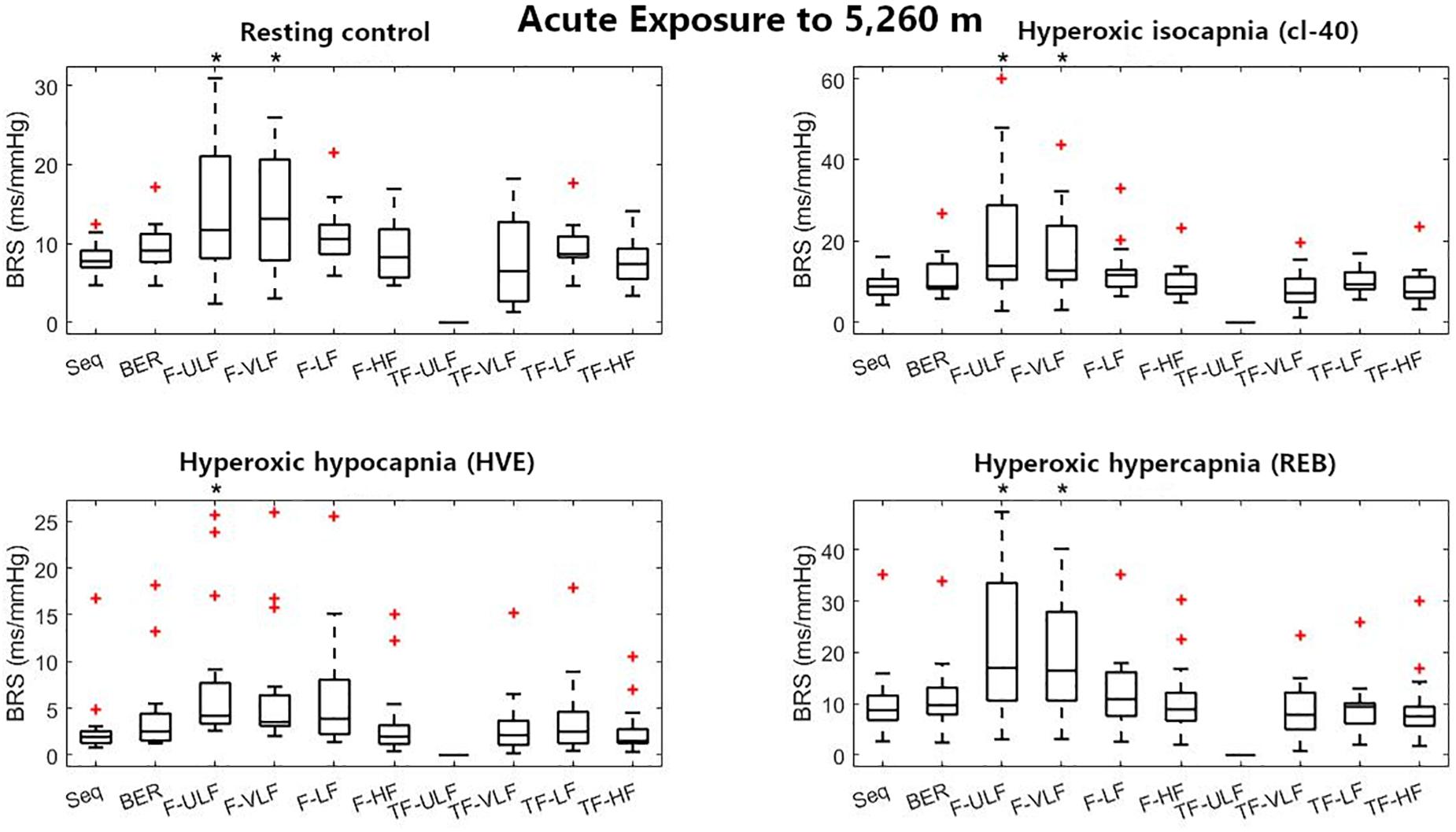
Figure 2. Baroreflex sensitivity estimation for the resting protocol on the first day at 5,260 m. cl-40, clamp 40 mmHg of inspired CO2; HVE, hyperventilation; REB, rebreathing up to PACO2 of 50 mmHg. +Denotes outliers. ∗Different from Seq (p < 0.05).
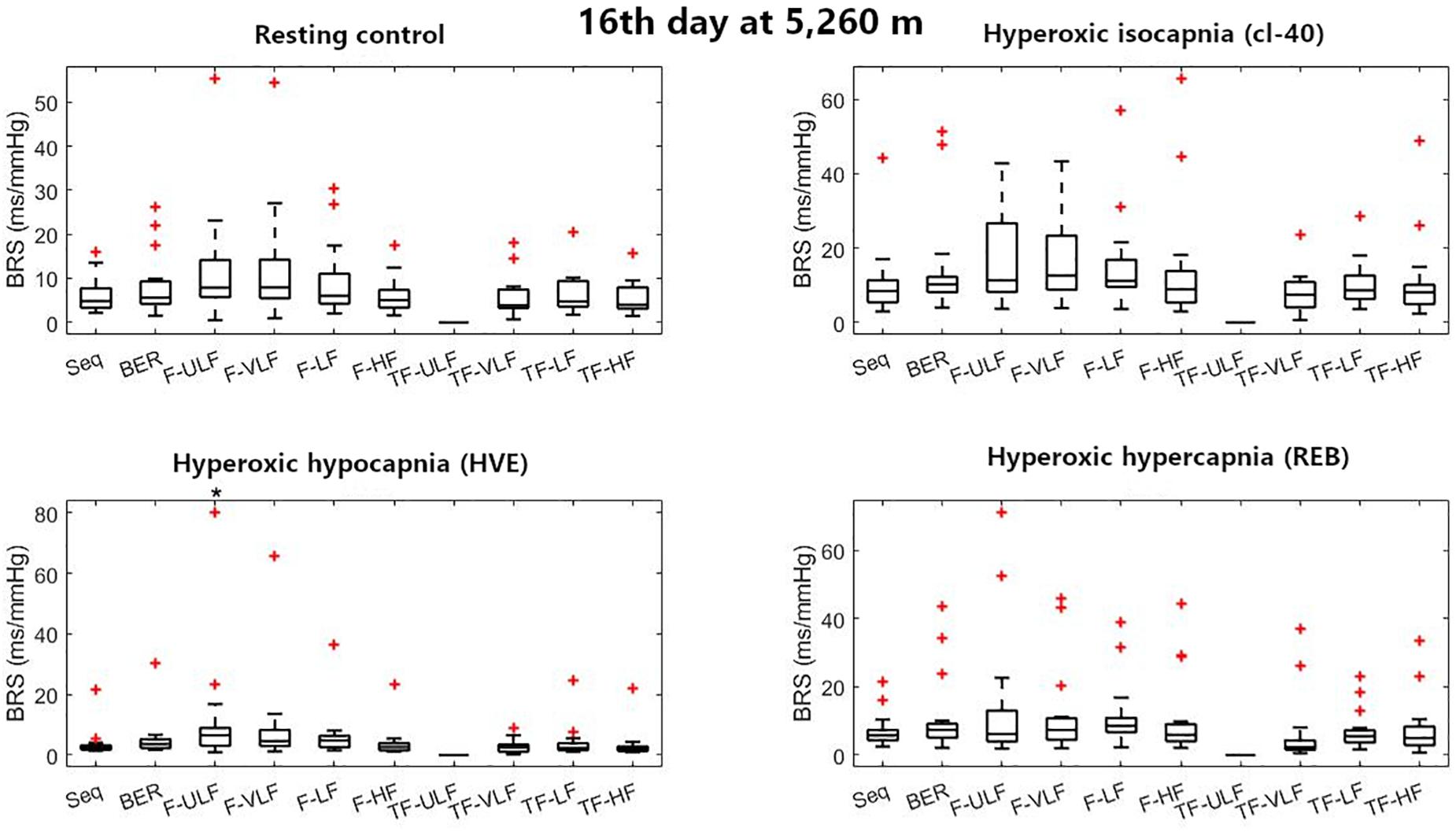
Figure 3. Baroreflex sensitivity estimation for the resting protocol on the 16th day at 5,260 m. cl-40, clamp 40 mmHg of inspired CO2; HVE, hyperventilation; REB, rebreathing up to PACO2 of 50 mmHg. +Denotes outliers. ∗Different from Seq (p < 0.05).
Two-way ANOVAs were performed to compare the various methods in each condition in the rest protocol, and each exercise intensity (PRE, 70W, 100W, 130W, 160W, and MAX) in the exercise protocol across SL, ALT1, and ALT16. The Tukey-Kramer post hoc test was performed when appropriate. The alpha level for significance was set at 0.05 and is reported rounded to three digits after the decimal point. All analyses were completed using MATLAB® (MathWorks, Natick, MA, United States). The Bland & Altman parameters are reported in Supplementary Tables S1, S2 for the rest protocol and Supplementary Table S3 for the exercise protocol. p-Values for the Bland & Altman are reported in these tables and may differ from the ANOVA significant levels indicated on the figures. In our case, ANOVAs are more reliable as they take into account the two ways (time × altitude) and that the same participants were measured in each condition. The Bland & Altman method is used to analyze the agreement between two different assays by determining the agreement between two methods used to measure the same parameter. The graphical analysis plots the difference between the two methods versus the mean (or median) of the two methods (Bland and Altman, 1986). In the present work, the median is used to make the analyses more robust between conditions and less sensitive to the extreme values of some of the participants.
Results
Twenty one participants were included in this study (12M/9F) aged 20.8 ± 1.4 years, height 175.8 ± 7.9 cm, weight 69.7 ± 9.0 kg, and BMI 22.4 ± 1.8 kg/m2.
Variations of BRS along the various experimental conditions and their physiological correlates are detailed in a previous paper (Bourdillon et al., 2018). This report focuses on the comparison between the four methods used to assess BRS. Briefly, resting heart rate increased in hypoxic conditions (77 ± 17; 90 ± 12, p < 0.05; 97 ± 15, p < 0.05, bpm SL, ALT1, and ALT16, respectively, all p-values vs. SL) whilst heart rate at maximal exercise decreased (185 ± 14; 170 ± 12, p < 0.05; 166 ± 13, p < 0.05, bpm, SL, ALT1, and ALT16, respectively, all p-values vs. SL). Mean resting BP was unchanged in hypoxic conditions (95 ± 11; 94 ± 9; 92 ± 9 mmHg, SL, ALT1, and ALT16, respectively), whilst it decreased at maximal exercise (121 ± 8; 109 ± 10, p < 0.05; 110 ± 11, p < 0.05, mmHg, SL, ALT1, and ALT16, respectively, all p-values vs. SL).
Resting Protocol Results
Figure 1 shows BRS estimation during the rest protocol at SL. When compared with the Seq method, BRS was significantly higher when estimated using F-ULF and F-VLF during rest and HVE. TF-ULF estimate was arbitrarily set at 0 because not relevant (actual values were close to zero).
Figure 2 shows BRS estimation during the rest protocol at ALT1. When compared with the Seq method, BRS was significantly higher when estimated using F-ULF and F-VLF in almost all conditions.
Figure 3 shows BRS estimation during the rest protocol at ALT16. When compared with the Seq method, BRS was consistent across all methods in all conditions except F-ULF during HVE.
Bland & Altman parameters for the resting protocol are detailed in Supplementary Tables S1, S2. In the latter, respiration frequency has been removed from the BP signal.
Exercise Protocol Results
Figure 4 shows BRS estimation during the exercise protocol at SL. When compared with the Seq method, BRS was significantly lower when estimated using the TF method from 100 W up. All methods underestimated BRS at 160 W compared to Seq.
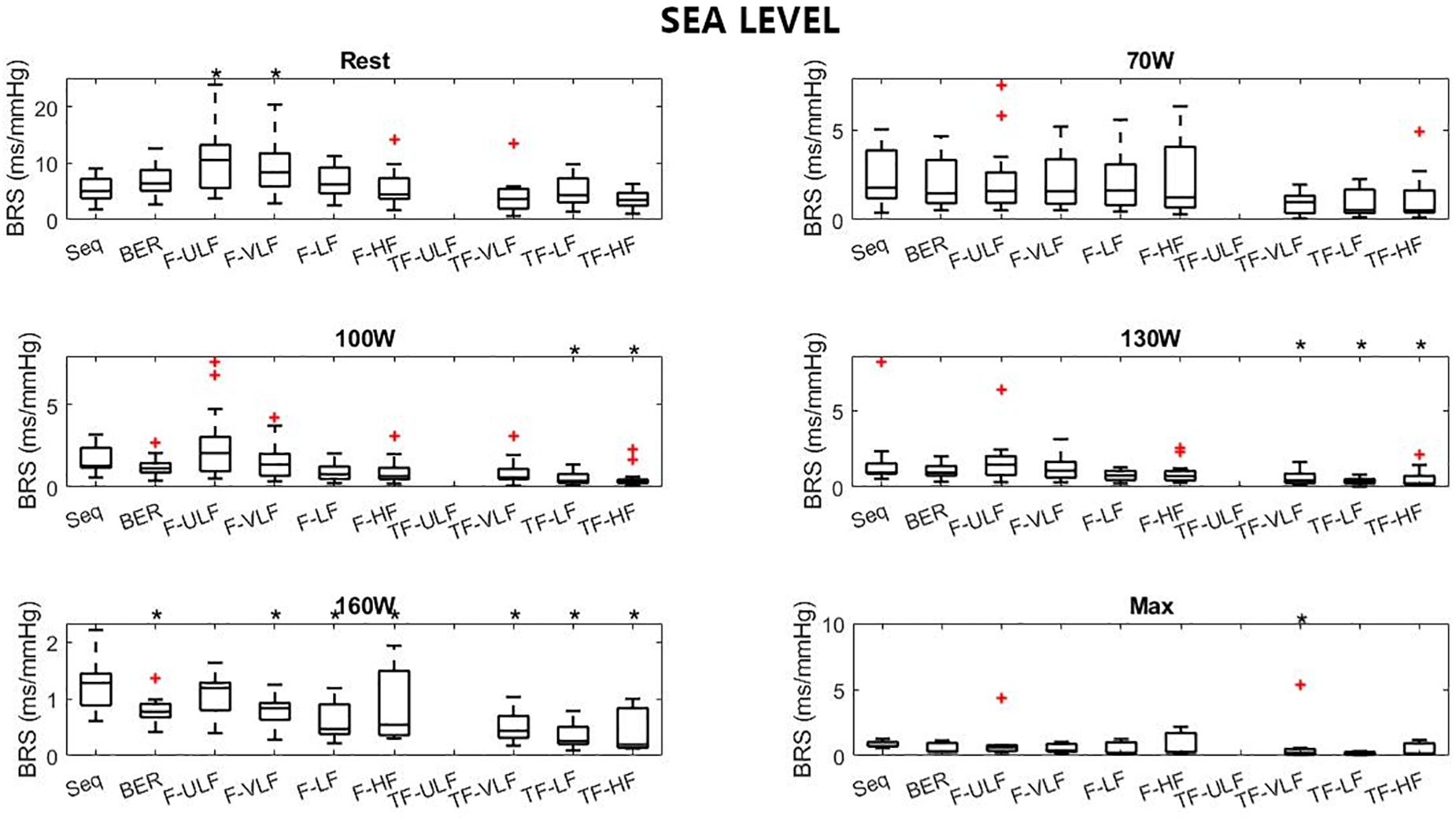
Figure 4. Baroreflex sensitivity estimation for the exercise protocol at sea level. +Denotes outliers. ∗Different from Seq (p < 0.05).
Figure 5 shows BRS estimation during the exercise protocol at ALT1. No differences between methods were observed.
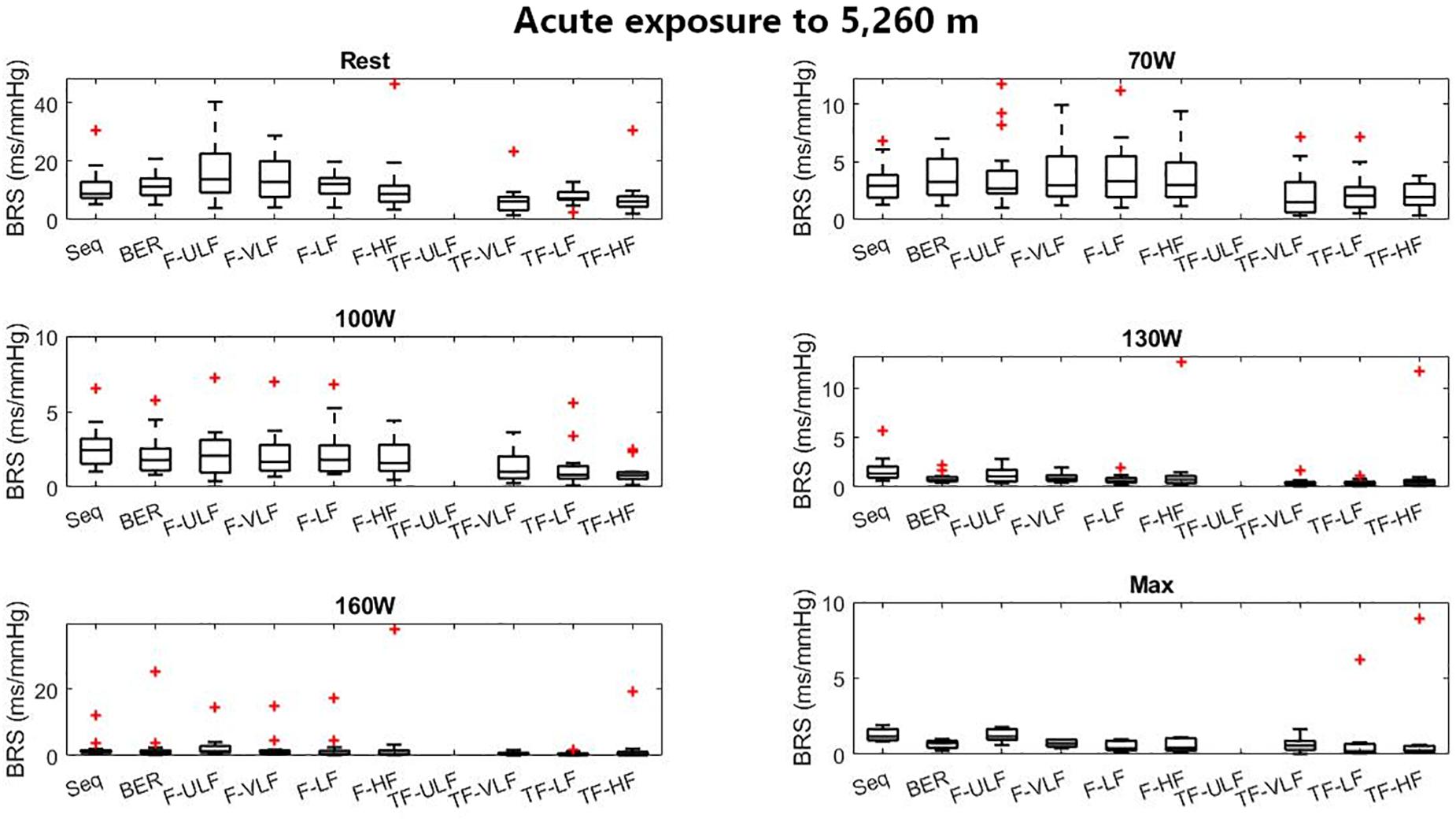
Figure 5. Baroreflex sensitivity estimation for the exercise protocol on the first day at 5,260 m. +Denotes outliers.
Figure 6 shows BRS estimation during the exercise protocol at ALT16. Somewhat comparable to SL, BRS was significantly lower when estimated using the TF method from 70 W up. All methods underestimated BRS at 130 W compared to Seq.
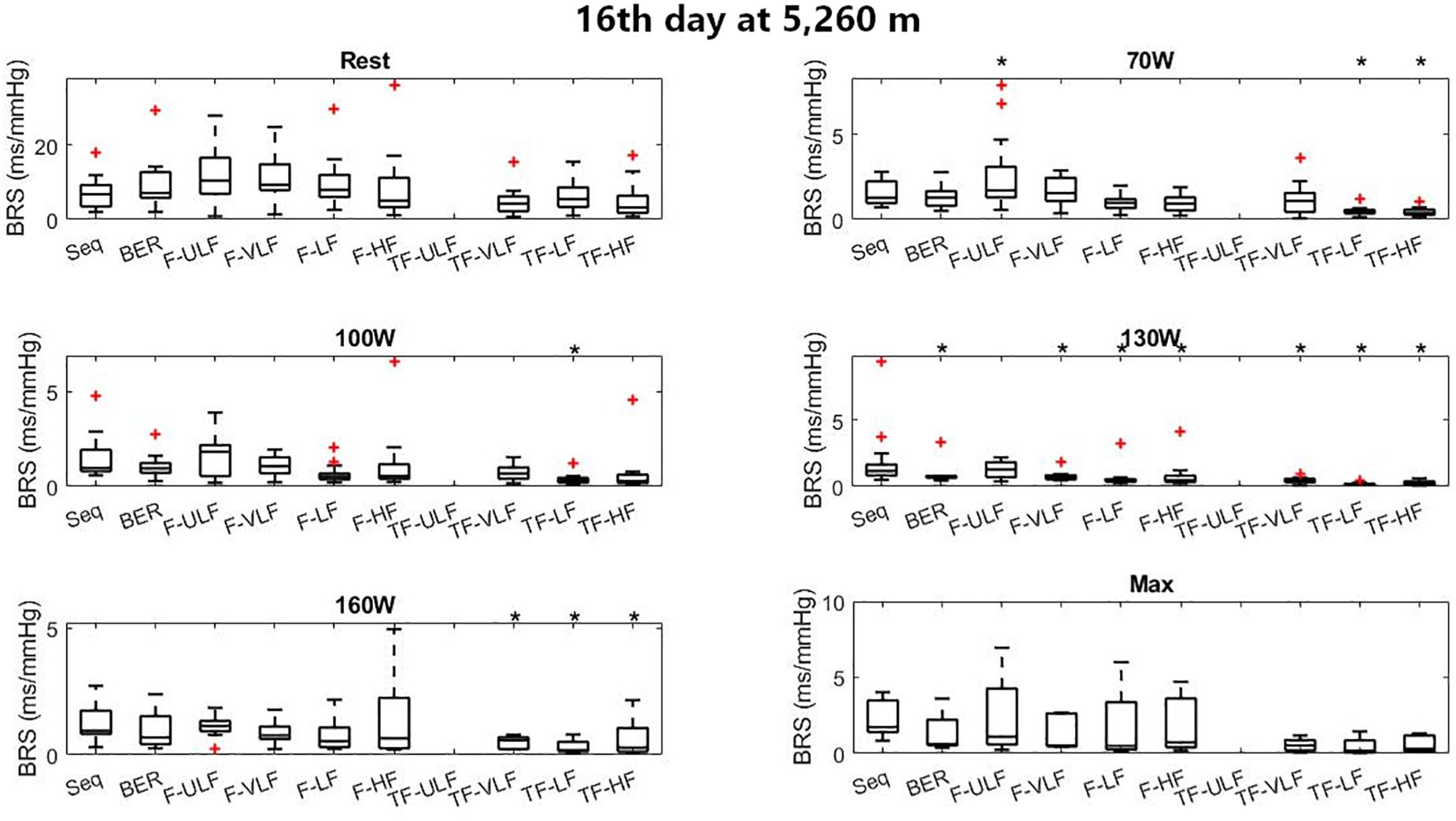
Figure 6. Baroreflex sensitivity estimation for the exercise protocol on the 16th day at 5,260 m. +Denotes outliers. ∗Different from Seq (p < 0.05).
Influence of Respiration on BRS
Supplementary Figures S1–S6 report the same data as Figures 1–6 respectively but with respiration removed. Tables 1, 2 summarize when removing respiration had a significant effect on BRS estimation during rest and exercise.
Table 3 shows which method is recommended depending on the stressor used.
Bland & Altman parameters for the exercise protocol are detailed in Supplementary Table S3. Removing respiration during exercise resulted in doubtful comparison between methods and therefore is not reported (also see below). During exercise, baroreflex activity decreased drastically as previously reported on the same set of participants (Bourdillon et al., 2018) whilst RSA increased. The beat-to-beat-interval time series variability became dependent only on RSA as exercise intensity increased. Therefore, removing respiration frequency led to a flat beat-to-beat interval shape, which made it hard to identify sequences for BRS estimation using the Seq method.
Discussion
This study compared four BRS estimation methods across an extreme range of conditions including rest and exercise, in hypoxia, hypocapnia, and hypercapnia in order to make recommendations on which method is most consistent depending on the stressor used. The main findings were: (1) The sequence and BER methods showed good agreement throughout whilst the frequency and transfer function methods were more discrepant (see Supplementary Material for detailed Bland & Altman parameters1); (2) conversely, when removing respiration from the blood pressure signal, the sequence and BER methods were more affected than the frequency and transfer function methods.
When it comes to choosing a method, BER seems the best candidate because (1) it is the easiest to compute; (2) it is consistent with the other methods, even though it tends to overestimate with respect to the sequence method; and (3) its interquartile range is smaller compared to that of the other methods, indicating it is more consistent across participants. Also, this method is free from the mathematical constraints present in the other methods, and therefore much easier to standardize (Bernardi et al., 2010).
BRS Estimation at Rest
Previous comparative studies showed fair agreement between BRS estimation methods in homogeneous groups of humans (Maestri et al., 1998; Pitzalis et al., 1998; Colombo et al., 1999; Laude et al., 2004). Comparing various populations, high-pass filtering further improved the agreement between methods (Bernardi et al., 2010). In the present study, a homogeneous population was exposed to very challenging situations. There was good agreement between Seq, BER and TF methods and not as good with the frequency method. Overestimation of BRS was quasi-systematic using the ULF and VLF bands. These bands represent long-term variations in the baroreflex function and may be associated to progressive changes in catecholamines and slow drifts in the blood pressure signal. Decreased plasma and increased urinary excretion of catecholamines have been reported during acute hypoxia (for review, Rostrup, 1998), whilst in chronic hypoxia, plasma catecholamine levels may normalize. In our experimental setup changes in plasma catecholamine levels were likely a confounding factor for BRS estimation in those frequency bands. At ALT1 and ALT16, during REB, high doses of CO2 resulted in very strong ventilatory drive (Bourdillon et al., 2018), which potentially masked the plasma catecholamine variations, hence the absence of significant differences compared to Seq.
BRS Estimation During Exercise
Baroreflex sensitivity estimation during exercise was consistent between methods as the decrease in BRS was detectable in all methods when exercise intensity increased and hypoxia exposure shifted from ALT1 to ALT16 (Figures 4–6). However, compared to the other methods TF underestimated BRS at almost all exercise stages. BRS estimates become very small when exercise intensity increases, which may make the Seq, F, and TF methods unstable. The numerous constraints for these methods were established for a resting sea level condition. They make Seq look at periods of parallel changes only, whereas F and TF methods look at fluctuations at specific frequencies only. There is no evidence that the established constraints do not induce a bias when estimating BRS under such a challenging situation as incremental exercise performed until exhaustion at high altitude.
Influence of the Respiratory Rate on BRS Estimation
One of the main physiological factors affecting BRS is the respiratory rate (Horsman et al., 2015). Removing respiration lowered primarily Seq, then BER and occasionally F-LF, F-HF, TF-LF and TF-HF, during the resting protocol. Therefore, Seq and BER contained information on the influence of respiration on blood pressure, whilst the other methods were less sensitive. It remains unclear whether blood pressure and beat-to-beat interval fluctuations coinciding with respiration are of baroreflex origin or arise from another central neural mechanism (Diaz and Taylor, 2006; Tan and Taylor, 2011). Without controlled respiratory rate (with a metronome for example) disentangling the effects of respiratory rate on BRS from the effects of blood pressure oscillation frequency is very difficult if not impossible (Goldstein et al., 1982; Pagani et al., 1988; La Rovere et al., 2008; Bourdillon et al., 2018). However, lowered BRS estimation when removing respiration in Seq and BER indicate that those methods report for the major part the effects of breathing on BRS and for a minor part the effect of blood pressure oscillation frequency. The frequency methods were more balanced and reported in roughly equivalent proportions the two phenomena.
During progressive exercise to exhaustion, there is a resetting of baroreflex to increased arterial pressures (Bevegård and Shepherd, 1966; Eckberg et al., 1975; Pawelczyk and Raven, 1989; Joyner, 2006) which proportionally make the effects of baroreflex on beat-to-beat intervals smaller and the effects of respiratory sinus arrhythmia larger. Also, as beat-to-beat interval decreases drastically, the possibility of variation with regards to blood pressure is much less if not absent. Therefore, the estimate of BRS using Seq is drastically decreased and even more when respiration is removed. The other methods seem to better ‘adapt’ to the exercise condition. The ULF and VLF bands were generally more affected by respiration removal during exercise than during rest. There may be a further confounding factor as during exercise there are important increases in plasma and neural catecholamine levels, associated to increased arterial tone and vasodilation in working muscles, which may flatten the long-term variations in blood pressure.
Limitations
Guideline recommendations are a window duration between 2 and 5 min for frequency analyses (Task Force, 1996). We are slightly under the lower limit, but as stated above this was imposed by the extreme experimental conditions. This may have made the frequency analyses a little less stable and added noise in our dataset.
The effects of respiration on BRS were only estimated from a frequency point of view. However, tidal volume may also interfere with BRS (Taha et al., 1995; St Croix et al., 1999). Greater tidal volumes (typically hypercapnia and high exercise intensities) may increase the influence of respiration on BRS.
Removing respiration lowers BRS estimate. During exercise, BRS seems to reach its lower limit, and removing respiration during exercise resulted in barely significant BRS estimate for most methods and Bland & Altman computations. Therefore, no table reports Bland & Altman parameters for methods comparison with respiration removed during exercise.
Baroreflex sensitivity in premenopausal females varies across the menstrual cycle. Given the design of this field study, it would have been impractical to control for menstrual cycle across the period of acclimatization. Variations due the menstrual cycle may have added noise in the recordings of the 9 females included in this study.
Using the sequence method, positive and negative sequences were isolated and treated separately, which did not significantly change the results compared to pooling. When more than three consecutive points constituting a sequence were found BRS was calculated with and without overlap of those points, which again did not significantly change the results. The sequence method has a number of limits concerning the criteria about what sequences can be used, but we have taken as many precautions as possible to ensure that the reported BRS represent as accurately as possible what is actually ongoing during acclimatization to altitude. Also, a 90-s window for the sequence method is short, usually 5-min window are used (Ogoh et al., 2005) but, in the context of AltitudeOmics 90 s was the longest steady state that could be tolerated by the participants in hypercapnic condition and at several exercise stages, especially in hypoxic conditions. Although this may limit the number of sequences detected and would influence the overall gain, we have the lower limit of 5 sequences to validate a BRS measurement, as usual in studies using the sequence method. A strength of our study is the use of intra-arterial catheterization for the continuous measurement of blood pressure. Therefore, the signal was clean (low noise) and better delineated than in the great majority of other studies, where generally finger cuff coupled to photo-plethysmography originated signals were recorded. Therefore, the technique presently used (interpolation coupled to maximum finding) is deemed of good quality.
This study is part of the AltitudeOmics project which primary goal was not to explore BRS function. Therefore, there was no condition with controlled respiratory frequency using a metronome, which would have allowed better determination of respiratory and blood pressure oscillations on BRS estimation. Within this article all methods are compared to the sequence method, but there was no comparison to a gold standard method such as the modified Oxford method, therefore methods are only compared between them and under or over-estimation of BRS is only considered as such. However, the AltitudeOmics database richness and the quality of the raw intra-arterial (BP) data deserved being reported to the scientific community.
Conclusion
This study is the first to report systematic comparison of four BRS estimation methods using blood pressure data obtained with an arterial catheter, during acute, and chronic exposure to 5,260 m, at rest and during dynamic exercise to exhaustion. The sequence and BER methods for BRS estimation showed good coherence but contained more respiratory related information than the frequency and transfer function methods, indicating that the first two methods indicate for a major part respiratory effect on BRS. BER method is recommended because it is the easiest to compute, it is consistent with other methods, even though it tends to overestimate compared to the sequence method, whilst its interquartile range is the smallest, meaning it is more consistent across participants.
Author’s Note
This paper is part of a series titled “AltitudeOmics” that together represent a group of studies that explore the basic mechanisms controlling human acclimatization to hypoxia and its subsequent retention. Many people and organizations have invested enormous amounts of time and resources to make AltitudeOmics a success.
Data Availability Statement
All datasets generated for this study are included in the article/Supplementary Material.
Ethics Statement
This study was approved by the Institutional Review Boards of the University of Colorado and the University of Oregon and by the Human Research Protection Office of the US Department of Defense and was performed according to the Declaration of Helsinki. The patients/participants provided their written informed consent to participate in this study.
Author Contributions
AL, AS, and RR conceived and designed the experiments, and managed the ethics process. All authors listed in Subudhi et al. (2014) performed the experiments. NB, SY, and J-MV analyzed the data. NB interpreted the data, wrote the first version of the manuscript, and prepared the figures. SY, AS, AL, RR, JM-V, and BK revised the manuscript. All authors approved the final version of the manuscript.
Funding
This study was supported by the Swiss National Science Foundation, the Faculty of Medicine of the University of Geneva and the Institute of Sport Sciences or the University of Lausanne. The overall AltitudeOmics study was funded in part by U.S. Department of Defense Grants W81XWH- 11-2-0040 TATRC to RR and W81XWH-10-2-0114 to AL; the Cardiopulmonary & Respiratory Physiology Laboratory, University of Oregon; and by the Altitude Research Center and the Charles S. Houston Endowed Professorship, Department of Medicine, School of Medicine, University of Colorado Denver.
Conflict of Interest
The authors declare that the research was conducted in the absence of any commercial or financial relationships that could be construed as a potential conflict of interest.
Acknowledgments
Foremost, the study was made possible by the tireless support, generosity, and tenacity of our research subjects. AltitudeOmics principal investigators were A. T. Lovering, A. W. Subudhi, and R. C. Roach. A complete list of other investigators on this multinational, collaborative effort involved in development, subject management and data collection, supporting industry partners, and people and organizations in Bolivia that made AltitudeOmics possible is available in the first paper in this series (Subudhi et al., 2014).
Supplementary Material
The Supplementary Material for this article can be found online at: https://www.frontiersin.org/articles/10.3389/fphys.2019.01505/full#supplementary-material
FIGURE S1 | Baroreflex sensitivity BRS estimation for the resting protocol at sea level, respiration removed. cl-40, clamp 40 mmHg of inspired CO2; HVE, hyperventilation; REB, rebreathing up to PACO2 of 50 mmHg. +Denotes outliers. ∗Different from Seq (p < 0.05).
FIGURE S2 | Baroreflex sensitivity estimation for the resting protocol on the first day at 5,260 m, respiration removed. cl-40, clamp 40 mmHg of inspired CO2; HVE, hyperventilation; REB, rebreathing up to PACO2 of 50 mmHg. +Denotes outliers. ∗Different from Seq (p < 0.05).
FIGURE S3 | Baroreflex sensitivity estimation for the resting protocol on the 16th day at 5,260 m, respiration removed. cl-40, clamp 40 mmHg of inspired CO2; HVE, hyperventilation; REB, rebreathing up to PACO2 of 50 mmHg. +Denotes outliers. ∗Different from Seq (p < 0.05).
FIGURE S4 | Baroreflex sensitivity estimation for the exercise protocol at sea level, respiration removed. +Denotes outliers. ∗Different from Seq (p < 0.05).
FIGURE S5 | Baroreflex sensitivity estimation for the exercise protocol on the first day at 5,260 m, respiration removed. +Denotes outliers. ∗Different from Seq (p < 0.05).
FIGURE S6 | Baroreflex sensitivity estimation for the exercise protocol on the 16th day at 5,260 m, respiration removed. +Denotes outliers. ∗Different from Seq (p < 0.05).
TABLE S1 | Bland & Altman parameters during the rest protocol.
TABLE S2 | Bland & Altman parameters during the rest protocol, without respiration.
TABLE S3 | Bland & Altman parameters during the exercise protocol.
References
Bernardi, L., De Barbieri, G., Rosengård-Bärlund, M., Mäkinen, V.-P., Porta, C., Groop, P.-H., et al. (2010). New method to measure and improve consistency of baroreflex sensitivity values. Clin. Auton. Res. 20, 353–361. doi: 10.1007/s10286-010-0079-1
Bernardi, L., Passino, C., Spadacini, G., Calciati, A., Robergs, R., Greene, R., et al. (1998). Cardiovascular autonomic modulation and activity of carotid baroreceptors at altitude. Clin. Sci. 95, 565–573. doi: 10.1042/cs0950565
Bevegård, B. S., and Shepherd, J. T. (1966). Circulatory effects of stimulating the carotid arterial stretch receptors in man at rest and during exercise. J. Clin. Invest. 45, 132–142. doi: 10.1172/jci105317
Bland, J. M., and Altman, D. G. (1986). Statistical methods for assessing agreement between two methods of clinical measurement. Lancet 1, 307–310. doi: 10.1016/s0140-6736(86)90837-8
Bourdillon, N., Yazdani, S., Subudhi, A. W., Lovering, A. T., Roach, R. C., Vesin, J.-M., et al. (2018). AltitudeOmics: baroreflex sensitivity during acclimatization to 5,260 m. Front. Physiol. 9:767. doi: 10.3389/fphys.2018.00767
Colombo, R., Mazzuero, G., Spinatonda, G., Lanfranchi, P., Giannuzzi, P., Ponikowski, P., et al. (1999). Comparison between spectral analysis and the phenylephrine method for the assessment of baroreflex sensitivity in chronic heart failure. Clin. Sci. 97, 503–513. doi: 10.1042/cs0970503
Cooper, V. L., Pearson, S. B., Bowker, C. M., Elliott, M. W., and Hainsworth, R. (2005). Interaction of chemoreceptor and baroreceptor reflexes by hypoxia and hypercapnia - a mechanism for promoting hypertension in obstructive sleep apnoea. J. Physiol. 568, 677–687. doi: 10.1113/jphysiol.2005.094151
Di Rienzo, M., Parati, G., Castiglioni, P., Tordi, R., Mancia, G., Pedotti, A., et al. (2001). Baroreflex effectiveness index: an additional measure of baroreflex control of heart rate in daily life. Am. J. Physiol. Regul. Integr. Comp. Physiol. 280, R744–R751.
Diaz, T., and Taylor, J. A. (2006). Probing the arterial baroreflex: is there a “spontaneous” baroreflex? Clin. Auton. Res. 16, 256–261. doi: 10.1007/s10286-006-0352-5
Eckberg, D. L. (2009). Point:counterpoint: respiratory sinus arrhythmia is due to a central mechanism vs. respiratory sinus arrhythmia is due to the baroreflex mechanism. J. Appl. Physiol. 106, 1740–1742. doi: 10.1152/japplphysiol.91107.2008
Eckberg, D. L., Cavanaugh, M. S., Mark, A. L., and Abboud, F. M. (1975). A simplified neck suction device for activation of carotid baroreceptors. J. Lab. Clin. Med. 85, 167–173.
Fisher, J. P., Ogoh, S., Junor, C., Khaja, A., Northrup, M., Fadel, P. J., et al. (2009). Spontaneous baroreflex measures are unable to detect age-related impairments in cardiac baroreflex function during dynamic exercise in humans. Exp. Physiol. 94, 447–458. doi: 10.1113/expphysiol.2008.044867
Frederiks, J., Swenne, C. A., TenVoorde, B. J., Honzíková, N., Levert, J. V., Maan, A. C., et al. (2000). The importance of high-frequency paced breathing in spectral baroreflex sensitivity assessment. J. Hypertens. 18, 1635–1644. doi: 10.1097/00004872-200018110-00015
Frigge, M., Hoaglin, D. C., and Iglewicz, B. (1989). Some implementations of the boxplot. Am. Stat. 43, 50–54. doi: 10.1080/00031305.1989.10475612
Goldstein, D. S., Horwitz, D., and Keiser, H. R. (1982). Comparison of techniques for measuring baroreflex sensitivity in man. Circulation 66, 432–439. doi: 10.1161/01.cir.66.2.432
Halliwill, J. R., and Minson, C. T. (1982). Effect of hypoxia on arterial baroreflex control of heart rate and muscle sympathetic nerve activity in humans. J. Appl. Physiol. 93, 857–864. doi: 10.1152/japplphysiol.01103.2001
Horsman, H. M., Peebles, K. C., and Tzeng, Y. C. (2015). Interactions between breathing rate and low-frequency fluctuations in blood pressure and cardiac intervals. J. Appl. Physiol. 119, 793–798. doi: 10.1152/japplphysiol.00525.2015
Joyner, M. J. (2006). Baroreceptor function during exercise: resetting the record. Exp. Physiol. 91, 27–36. doi: 10.1113/expphysiol.2005.032102
Karemaker, J. M. (2009). Counterpoint: respiratory sinus arrhythmia is due to the baroreflex mechanism. J. Appl. Physiol. 106, 1742–1743. doi: 10.1152/japplphysiol.91107.2008a
Knapik, J. (1989). The army physical fitness test (apft): a review of the literature. Mil. Med. 154, 326–329. doi: 10.1093/milmed/154.6.326
La Rovere, M. T., Pinna, G. D., and Raczak, G. (2008). Baroreflex sensitivity: measurement and clinical implications. Ann. Noninvasive Electrocardiol. 13, 191–207. doi: 10.1111/j.1542-474x.2008.00219.x
Laude, D., Elghozi, J.-L., Girard, A., Bellard, E., Bouhaddi, M., Castiglioni, P., et al. (2004). Comparison of various techniques used to estimate spontaneous baroreflex sensitivity (the EuroBaVar study). Am. J. Physiol. Regul. Integr. Comp. Physiol. 286, R226–R231.
Maestri, R., Pinna, G. D., Mortara, A., La Rovere, M. T., and Tavazzi, L. (1998). Assessing baroreflex sensitivity in post-myocardial infarction patients: comparison of spectral and phenylephrine techniques. J. Am. Coll. Cardiol. 31, 344–351. doi: 10.1016/s0735-1097(97)00499-3
Merri, M., Farden, D. C., Mottley, J. G., and Titlebaum, E. L. (1990). Sampling frequency of the electrocardiogram for spectral analysis of the heart rate variability. IEEE Trans. Biomed. Eng. 37, 99–106. doi: 10.1109/10.43621
Messerli, F. H., Williams, B., and Ritz, E. (2007). Essential hypertension. Lancet Lond. Engl. 370, 591–603.
Michelini, L. C., O’Leary, D. S., Raven, P. B., and Nóbrega, A. C. L. (2015). Neural control of circulation and exercise: a translational approach disclosing interactions between central command, arterial baroreflex, and muscle metaboreflex. Am. J. Physiol. Heart Circ. Physiol. 309, H381–H392. doi: 10.1152/ajpheart.00077.2015
Ogoh, S., Fisher, J. P., Dawson, E. A., White, M. J., Secher, N. H., and Raven, P. B. (2005). Autonomic nervous system influence on arterial baroreflex control of heart rate during exercise in humans. J. Physiol. 566, 599–611. doi: 10.1113/jphysiol.2005.084541
Olin, J. T., Dimmen, A. C., Subudhi, A. W., and Roach, R. C. (2012). A simple method to clamp end-tidal carbon dioxide during rest and exercise. Eur. J. Appl. Physiol. 112, 3439–3444. doi: 10.1007/s00421-012-2433-6
Pagani, M., Somers, V., Furlan, R., Dell’Orto, S., Conway, J., Baselli, G., et al. (1988). Changes in autonomic regulation induced by physical training in mild hypertension. Hypertension 12, 600–610. doi: 10.1161/01.hyp.12.6.600
Papelier, Y., Escourrou, P., Gauthier, J. P., and Rowell, L. B. (1994). Carotid baroreflex control of blood pressure and heart rate in men during dynamic exercise. J. Appl. Physiol. 77, 502–506. doi: 10.1152/jappl.1994.77.2.502
Paprika, D., Gingl, Z., Rudas, L., and Zöllei, E. (2014). Hemodynamic effects of slow breathing: does the pattern matter beyond the rate? Acta Physiol. Hung. 101, 273–281. doi: 10.1556/APhysiol.101.2014.3.2
Parati, G., Di Rienzo, M., Bertinieri, G., Pomidossi, G., Casadei, R., Groppelli, A., et al. (1988). Evaluation of the baroreceptor-heart rate reflex by 24-hour intra-arterial blood pressure monitoring in humans. Hypertension 12, 214–222. doi: 10.1161/01.hyp.12.2.214
Pawelczyk, J. A., and Raven, P. B. (1989). Reductions in central venous pressure improve carotid baroreflex responses in conscious men. Am. J. Physiol. 257, H1389–H1395.
Pinna, G. D., and Maestri, R. (2002). New criteria for estimating baroreflex sensitivity using the transfer function method. Med. Biol. Eng. Comput. 40, 79–84. doi: 10.1007/bf02347699
Pitzalis, M. V., Mastropasqua, F., Massari, F., Passantino, A., Totaro, P., Forleo, C., et al. (1998). Beta-blocker effects on respiratory sinus arrhythmia and baroreflex gain in normal subjects. Chest 114, 185–191. doi: 10.1378/chest.114.1.185
Potts, J. T., Shi, X. R., and Raven, P. B. (1993). Carotid baroreflex responsiveness during dynamic exercise in humans. Am. J. Physiol. 265, H1928–H1938.
Rostrup, M. (1998). Catecholamines, hypoxia and high altitude. Acta Physiol. Scand. 162, 389–399. doi: 10.1046/j.1365-201x.1998.00335.x
St Croix, C. M., Satoh, M., Morgan, B. J., Skatrud, J. B., and Dempsey, J. A. (1999). Role of respiratory motor output in within-breath modulation of muscle sympathetic nerve activity in humans. Circ. Res. 85, 457–469. doi: 10.1161/01.res.85.5.457
Subudhi, A. W., Bourdillon, N., Bucher, J., Davis, C., Elliott, J. E., Eutermoster, M., et al. (2014). altitudeomics: the integrative physiology of human acclimatization to hypobaric hypoxia and its retention upon reascent. PLoS One 9:e92191. doi: 10.1371/journal.pone.0092191
Subudhi, A. W., Lorenz, M. C., Fulco, C. S., and Roach, R. C. (2008). Cerebrovascular responses to incremental exercise during hypobaric hypoxia: effect of oxygenation on maximal performance. Am. J. Physiol. Heart Circ. Physiol. 294, H164–H171.
Taha, B. H., Simon, P. M., Dempsey, J. A., Skatrud, J. B., and Iber, C. (1995). Respiratory sinus arrhythmia in humans: an obligatory role for vagal feedback from the lungs. J. Appl. Physiol. 78, 638–645. doi: 10.1152/jappl.1995.78.2.638
Tan, C. O., and Taylor, J. A. (2011). Feedback and feedforward sympathetic haemodynamic control: chicken or egg? J. Physiol. 589, 1867. doi: 10.1113/jphysiol.2011.208199
Task Force (1996). Heart rate variability standards of measurement, physiological interpretation, and clinical use. task force of the european society of cardiology and the north american society of pacing and electrophysiology. Eur. Heart J. 17, 354–381.
Keywords: baroreflex sensitivity, hypoxia, exercise, altitude, hypercapnia
Citation: Bourdillon N, Yazdani S, Vesin J-M, Subudhi AW, Lovering AT, Roach RC and Kayser B (2019) AltitudeOmics: Spontaneous Baroreflex Sensitivity During Acclimatization to 5,260 m: A Comparison of Methods. Front. Physiol. 10:1505. doi: 10.3389/fphys.2019.01505
Received: 04 September 2019; Accepted: 28 November 2019;
Published: 10 December 2019.
Edited by:
Barbara Morgan, University of Wisconsin–Madison, United StatesReviewed by:
Nisha Charkoudian, US Army Research Institute of Environmental Medicine (USARIEM), United StatesStéphane Doutreleau, Centre Hospitalier Universitaire de Grenoble, France
Sarah L. Hissen, Western Sydney University, Australia
Copyright © 2019 Bourdillon, Yazdani, Vesin, Subudhi, Lovering, Roach and Kayser. This is an open-access article distributed under the terms of the Creative Commons Attribution License (CC BY). The use, distribution or reproduction in other forums is permitted, provided the original author(s) and the copyright owner(s) are credited and that the original publication in this journal is cited, in accordance with accepted academic practice. No use, distribution or reproduction is permitted which does not comply with these terms.
*Correspondence: Nicolas Bourdillon, nicolas.bourdillon@unil.ch; nicolas.bourdillon@gmail.com