- 1Department of Anatomy, Physiology and Pharmacology, College of Medicine, University of Saskatchewan, Saskatoon, SK, Canada
- 2College of Dentistry, University of Saskatchewan, Saskatoon, SK, Canada
- 3Department of Surgery, College of Medicine, University of Saskatchewan, Saskatoon, SK, Canada
Background: Stromal interaction molecule 1 (STIM1) is one of the main components of the store operated Ca2+ entry (SOCE) signaling pathway. Individuals with mutated STIM1 present severely hypomineralized enamel characterized as amelogenesis imperfecta (AI) but the downstream molecular mechanisms involved remain unclear. Circadian clock signaling plays a key role in regulating the enamel thickness and mineralization, but the effects of STIM1-mediated AI on circadian clock are unknown.
Objectives: The aim of this study is to examine the potential links between SOCE and the circadian clock during amelogenesis.
Methods: We have generated mice with ameloblast-specific deletion of Stim1 (Stim1fl/fl/Amelx-iCre+/+, Stim1 cKO) and analyzed circadian gene expression profile in Stim1 cKO compared to control (Stim1fl/fl/Amelx-iCre–/–) using ameloblast micro-dissection and RNA micro-array of 84 circadian genes. Expression level changes were validated by qRT-PCR and immunohistochemistry.
Results: Stim1 deletion has resulted in significant upregulation of the core circadian activator gene Brain and Muscle Aryl Hydrocarbon Receptor Nuclear Translocation 1 (Bmal1) and downregulation of the circadian inhibitor Period 2 (Per2). Our analyses also revealed that SOCE disruption results in dysregulation of two additional circadian regulators; p38α mitogen-activated protein kinase (MAPK14) and transforming growth factor-beta1 (TGF-β1). Both MAPK14 and TGF-β1 pathways are known to play major roles in enamel secretion and their dysregulation has been previously implicated in the development of AI phenotype.
Conclusion: These data indicate that disruption of SOCE significantly affects the ameloblasts molecular circadian clock, suggesting that alteration of the circadian clock may be partly involved in the development of STIM1-mediated AI.
Introduction
Calcium plays a pivotal role in enamel mineralization, yet the exact mechanisms involved in Ca2+ transport and the roles of Ca2+ signaling in regulating ameloblast functions during amelogenesis remain unclear (Berdal et al., 1995; Bailleul-Forestier et al., 1996; Nurbaeva et al., 2017). Initially, Ca2+ influx into ameloblasts was thought to occur passively via paracellular diffusion (Bawden, 1989). However, several subsequent reports have shown that Ca2+ transport occurs principally transcellularly and mainly through high capacity intracellular stores in endoplasmic reticulum (ER) (Hubbard, 2000; Lacruz et al., 2012b; Nurbaeva et al., 2015a, b, 2017). This mode of transport is termed Store-operated Ca2+ entry (SOCE) and is mainly mediated by the ER transmembrane proteins Stromal interaction molecule 1 and 2 (STIM1 and STIM2) and the highly selective plasma membrane (PM) calcium release activated channels (CRAC) (Prakriya and Lewis, 2015; Lacruz, 2017). STIM1 and STIM2 serve as intracellular (i) [Ca2+] sensors, while CRAC protein 1, 2 and 3 (ORAI1, 2, and 3) are transmembrane proteins that form the pores of the CRAC channels and serve as a highly selective filter during Ca2+ entry from the circulation to the ameloblasts (Lacruz, 2017; Fahrner et al., 2018). Upon Ca2+ depletion from the ER, STIM1 binds to ORAI to facilitate Ca2+ entry and activate Ca2+ -dependent signal transduction. It must be noted that STIM2 is usually present at lower levels than STIM1, and that ORAI2 and ORAI3 activation results in a lower Ca2+ intake compared with ORAI1 (Hoth and Niemeyer, 2013). The importance of SOCE in regulating calcium transport during enamel mineralization is clearly reflected in the fact that patients with mutations in either STIM1 or ORAI1 have severe dental enamel defects, characterized as hypocalcified Amelogenesis Imperfecta (AI) (Eckstein and Lacruz, 2018; Feske, 2019). Moreover, several in vivo and in vitro studies have shown that SOCE components are robustly expressed by ameloblasts and served as key modulators of ameloblast’s differentiation and function (Nurbaeva et al., 2015a, b; Zheng et al., 2015; Eckstein et al., 2017; Furukawa et al., 2017; Said et al., 2019).
It is well established that Ca2+ may act as a second messenger with a broad role in regulating gene expression via orchestrating several major signaling pathways (Tonelli et al., 2012). One of the main molecular systems essential for amelogenesis is the circadian clock (Athanassiou-Papaefthymiou et al., 2011). Circadian clock is an intracellular mechanism that regulates gene and protein expression levels oscillations over a 24-h period (Adeola et al., 2019). These circadian genetic rhythms are directly controlled by a complex system of body clocks, which entails a central or master clock located in the hypothalamic suprachiasmatic nucleus (SCN) and several subordinate peripheral clocks located in multiple tissues including in ameloblasts (Adeola et al., 2019). At the molecular level, circadian rhythms are maintained via the differential expression of several transcription factors called clock genes (Partch et al., 2014; Takahashi, 2017). The main mammalian clock genes include Circadian Locomotor Output Cycles Kaput (Clock), Brain and Muscle Aryl Hydrocarbon Receptor Nuclear Translocation (Arntl or Bmal1), Period 1 (Per1), Period 2 (Per2), Period 3 (Per3), and Cryptochromes (Cry1) and Cry2 (Partch et al., 2014). Clock genes interact with each other in an intricate manner and form perpetual autoregulatory transcription translation feedback loops (TTFLs) that control the rhythmic expression of several clock-controlled genes (CCG) to create 24-h repetitive expression patterns necessary for normal functions in several physiological processes (Takahashi, 2017). TTFLs are affected directly by extracellular events whose actions are transduced intracellularly by second messengers such as calcium and cAMP (Honma and Honma, 2003; Hastings et al., 2018). In mammalian tissues, several studies showed that calcium can act directly on TTFLs via the calcium/cAMP-dependent transcription factor (CREB) (O’Neill and Reddy, 2012; Herzog et al., 2017). In the mouse SCN, it was shown that blocking calcium influx and lowering i[Ca2+] had abolished the rhythmic expression of Per1 (Lundkvist et al., 2005). In addition, the amplitude of Per1 and Per2 expression was significantly decreased by voltage-gated Ca2+ channel antagonists (Lundkvist et al., 2005). On the other hand, it must be noted that i[Ca2+] and cAMP levels oscillate in a circadian manner in the SCN neurons (O’Neill and Reddy, 2012). Thus, it has been suggested that cAMP and Ca2+ signaling may not only contribute in regulating timekeeping, but also that they are regulated by the cellular clock (i.e., Ca2+ and cAMP signaling is both output from, as well as input into the core clock pathway) (Palacios-Muñoz and Ewer, 2018).
The periodical nature of enamel secretion and the synchronized sequential pattern of ameloblast differentiation and mineralization strongly suggest that the process of amelogenesis is under circadian regulation (Papagerakis et al., 2014). Moreover, several publications from our group and others showed that this time-related control of ameloblasts activities may be orchestrated by the differential expression of circadian clock proteins during the distinct stages of enamel formation (Simmer et al., 2010; Athanassiou-Papaefthymiou et al., 2011; Lacruz et al., 2012a; Zheng et al., 2013, 2014). However, the molecular mechanisms involved in regulating the circadian clock during amelogenesis remain largely unexplored. In this study, we aimed to investigate the effects of calcium disruption on the ameloblasts molecular clock in vivo using a unique Stim1fl/fl/Amelx-iCre+/+ (Stim1 cKO) model (Said et al., 2019). The significant impact of Ca2+ and circadian clock in ameloblasts makes amelogenesis an excellent model system for deciphering the mechanistic links that may exist between intracellular calcium dynamics and molecular circadian clock. Indeed, our analysis showed that Stim1 ablation in ameloblasts results in significant dysregulation of the ameloblasts’ circadian clock. More specifically, we found that loss of Stim1 results in significant upregulation of the master circadian clock gene Bmal1 and down regulation of its antagonist, Per2. We also found that targeting Stim1 leads to significant changes of several other circadian regulators, including the p38α mitogen-activated protein kinase (MAPK14) and transforming growth factor-beta1 (TGF-β1). Both MAPK14 and TGF-β1 pathways are known to play a key role in tooth morphogenesis and enamel secretion, and their dysregulation has been previously implicated in the development of AI phenotype. Our data strongly suggest that SOCE affects the molecular circadian clock in ameloblast, which could be one of the downstream pathways involved in the development of Stim1-mediated AI.
Materials and Methods
Animals
Stim1 cKO generation and characterization were previously described (Said et al., 2019). Animals were housed in a pathogen-free facility in the Lab Animal Service Unit (LASU) at the University of Saskatchewan and all procedures for this study were authorized by the University Animal Care Committee (UACC) under an approved protocol (#20170014). All mice were housed under standard laboratory conditions in light-dark (LD) 12:12 conditions, normal room temperature, with ad libitum access to food and water. Genotypes were determined by tail biopsy and conventional polymerase chain reaction (PCR). The mice were humanely culled at postnatal day 14 (P14).
PCR Arrays
Enamel organ and secretory ameloblasts were micro-dissected from 2-week-old incisors in the left hemimandibles as previously described (Houari et al., 2018) and used for RNA extraction. RNA from Stim1 cKO and control animals was extracted using the RNeasy MiniKit (Qiagen). cDNA was made using RT2 First Strand Kit (Qiagen). The cDNA was used on the real-time RT2 Profiler PCR Array (QIAGEN, Cat. no. PAMM-153Z) in combination with RT2 SYBR Green qPCR Mastermix (Cat. no. 330529). CT values were exported to an Excel file to create a table of CT values. This table was then uploaded onto the data analysis web portal at http://www.qiagen.com/genelobe. Samples were assigned to controls and test groups. CT values were normalized based on a manual selection of two reference genes (HKG), B-actin (Actb) and Glyceraldehyde 3-phosphate dehydrogenase (Gapdh). The CT cut-off was set to 35. The data analysis web portal calculated fold change/regulation using ΔΔCT method, in which ΔCT is calculated between gene of interest (GOI) and an average of reference genes (HKG), followed by delta-delta CT (ΔΔCT) calculations [ΔCT (Test Group)– ΔCT (Control Group)]. Fold Change was then calculated using 2^ (−ΔΔCT) formula. The data analysis web portal also plots scatter plot, volcano plot, clustergram, and heat map. DAVID software tool was used to analyze the examined genes (Huang et al., 2009).
qRT-PCR
RNA was extracted with the RNeasy MiniKit (Qiagen) from Stim1 cKO and control mice (Stim1fl/fl/Amelx-iCre–/–) (n = 5 per genotype). After cDNA synthesis, the gene expression of Bmal1, Clock, Per1 & 2, Cry 1&2, Mapk14, Tgf-β1, Signal Transducer, and Activator of Transcription 5A (Stat5a), F-Box and Leucine Rich Repeat Protein (Fbxl) 3, RAR-related orphan receptor alpha (Rora), Nuclear Receptor Subfamily 1 Group D Member 1 (NR1D1), was assessed by quantitative PCR (qRT-PCR) with the 2^(−ΔΔCt) method. Gapdh was used as the reference gene. All primer sequences are included (Supplementary Table 1).
Statistics
All data are presented as mean ± standard deviation (SD). Student’s t-test was used to compare the gene expression. For the PCR profiler assays, fold-change values greater than 2 or less than 0.5 were considered differentially expressed. The P-values were calculated based on a Student’s t-test of the replicate 2^(−ΔΔCt) values for each gene in the control and Stim1 cKO groups; P-value < 0.05 was considered significant.
Immunohistochemistry
The right hemimandibles were sectioned in a sagittal plane and immunostained to assess BMAL1, PER2, and STIM1 protein cellular and tissue localization with a rabbit anti-PER2 antibody (1:200, LS-C2836; LifeSpan Biosciences), a rabbit anti-BMAL1 (1:250, NB100-2288; Novus Biologicals) and a rabbit anti-STIM1 (1:200, LSC34692; LSBio).
Results
The Circadian Profile of Ameloblasts Changes Significantly After Stim1 Deletion
We first assessed the effect of Stim1 ablation on ameloblasts’ circadian clock signaling by performing a validated circadian pathway PCR RNA microarray analysis. This analysis enabled us to evaluate the mRNA levels of 84 different genes in the circadian network. Gene Ontology and KEGG pathway analysis in the DAVID software (Huang et al., 2009) showed that the examined genes function in several essential signaling pathways including many calcium dependent ones (Figure 1A). Compared to control, we found that fourteen circadian genes were significantly differentially expressed (P < 0.05) in Stim1 cKO ameloblasts with at least a twofold differential expression compared to control (Figures 1B,C, Supplementary Table 2, and Supplementary Datasheet 1). Specifically, we found that Stim1 disruption had led to significant changes in the expression levels of several key clock genes that form the circadian loops, including Bmal1, Per2, Fbxl3, Ror-a, and Ror-c, which strongly indicates that SOCE may influence the ameloblast circadian clock system. The most significant changes were observed in Bmal1 and Per2 expression, as Bmal1 expression was greatly upregulated while Per2 was greatly downregulated in Stim1 cKO ameloblasts. We also found that Stim1 knock out resulted in significant changes in the expression levels of circadian regulators that are also known to be involved in regulating amelogenesis throughout its different stages whether it is during early craniofacial development (Transcription Factor AP-2 Alpha, Tfap2a), tooth morphogenesis and early enamel secretion (Mapk14), or enamel maturation and mineralization (Tgf- β1). Additionally, the RNA profile revealed that SOCE disruption altered the expression of other circadian transcription factors including, the nuclear receptor subfamily 2 group F member 6 (NR2F6) and early growth response proteins 1 & 3 (EGR1, EGR3).
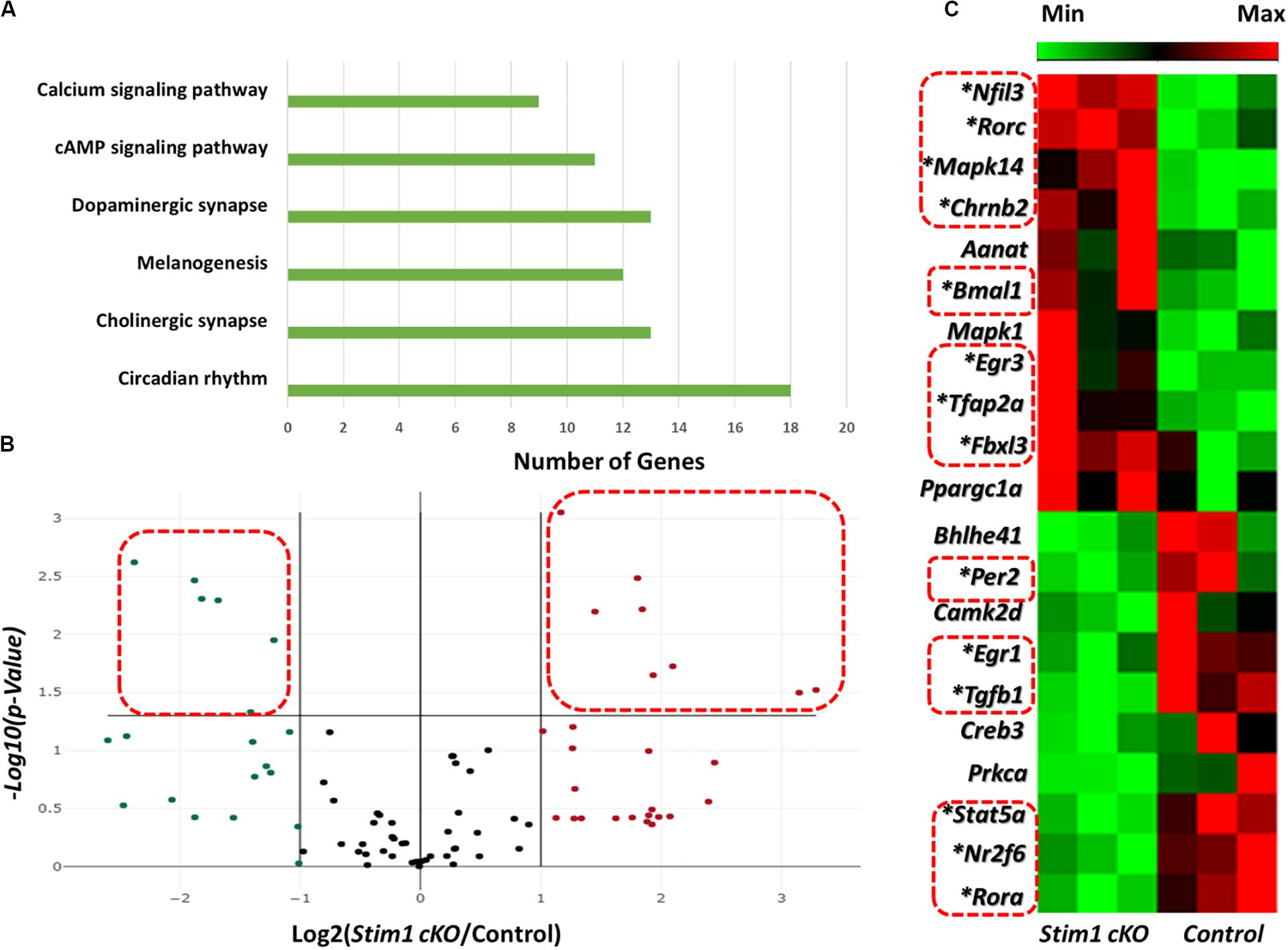
Figure 1. Effects of Stim1 KO on the gene expression profiles in ameloblasts. The expression change of 84 genes in ameloblasts from Stim1 cKO and control mice was performed by real-time PCR based array analysis. (A) A bar diagram showing the functional clustering of the examined genes in the 6 most enriched signal transduction pathways according to the Gene Ontology and KEGG pathway analysis performed by the DAVID software (B) A volcano plot for the examined genes in total, black dots indicates genes with unchanged gene expression in Stim 1 cKO versus control; the genes with at least two fold change [(i.e., Log2 (Stim1 cKO/Control) > 1 or < −1] were represented as red dots for the upregulated genes and in green dots for the downregulated genes; the genes were deemed to be significantly differentially expressed if presented with at least two fold change and a P-value < 0.05 [i.e., −Log10(P-Value) > 1.305, represented by the dots within the dashed red rectangles]. (C) A cluster heatmap showing the expression changes in some of the examined genes in Stim1 cKO ameloblasts against the control group. A total of 14 genes were found to be significantly differentially expressed in Stim1 cKO ameloblasts compared to the control group (P < 0.05, labeled by asterisks and within dashed red rectangles).
Validation of Stim1 Deletion Effects on the Ameloblasts Circadian Clock
We further validated these substantial changes in the circadian clock gene expression by qRT-PCR analysis in which we first assessed the differences in the mRNA levels of 8 core clock genes (Figures 2A,B). Similar to profiler RNA micro-array analysis, the qRT-PCR analysis showed that Bmal1 was greatly upregulated in Stim1 cKO mice while both Per2 and Ror-a were significantly downregulated. In addition to examining the core circadian genes we further assessed the changes of four additional circadian clock related genes of interest (Figure 2C). Similar to the profiler RNA micro-array pathway analysis, the qRT-PCR analysis showed that loss of Stim1 function in ameloblasts significantly alters the expression pattern of Tgf-β1 (downregulated) and Mapk14 (upregulated) and Fbxl3 (upregulated). It must be noted however, that contrary to the profiler assay analysis results, no significant changes were detected in the Stat5a gene.
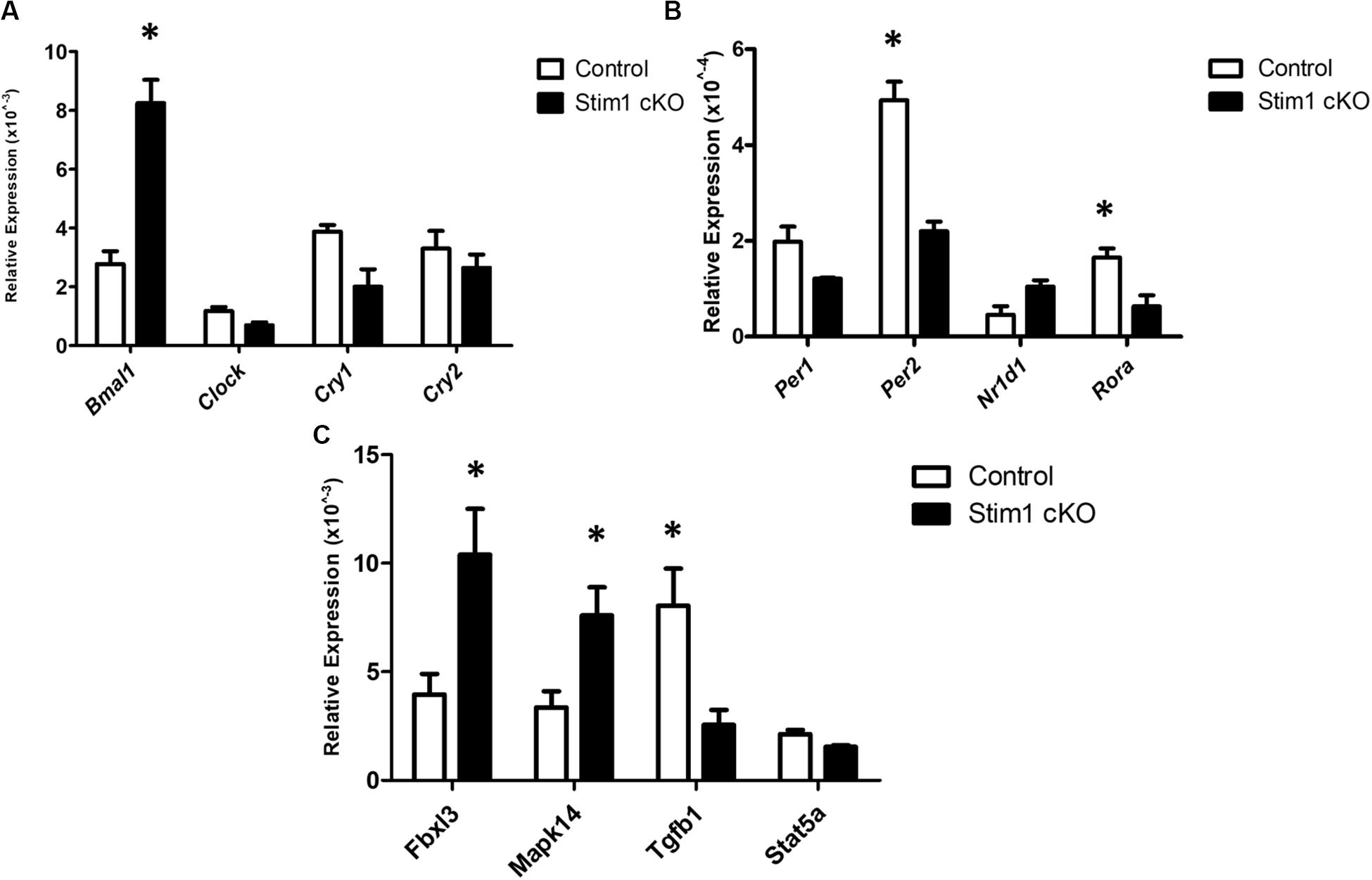
Figure 2. The validation for selected candidate genes by qRT-PCR (A–C). Data showed the relative mRNA fold changes of candidate genes in Stim1 cKO groups versus the control groups (n = 5 per genotype). All experiments were performed in triplicates (∗P < 0.05).
Examining the Clock Proteins in Stim1 cKO Ameloblasts
Immunohistochemical analysis using specific antibodies against BMAL1, PER2, and STIM1 was performed in order to further validate the RNA data reported above and to confirm Stim1 deletion in ameloblasts. Our data clearly showed that in Stim1 cKO ameloblasts BMAL1 proteins levels were much higher compared to control (Figure 3A). The PER2 expression levels were clearly downregulated overall, although PER2 expression pattern was quite peculiar as it appears to be expressed strongly in some ameloblasts nuclei and very weakly in others (Figure 3B, stars). This heterogeneity of PER2 localization in Stim1 cKO ameloblasts may be attributed to posttranslational modifications including abnormal protein sequestering and degradation. Finally, our data here clearly showed that PER2 protein is mainly located in the nucleus (Figure 3B arrows) while BMAL1 is concentrated in the apical cytoplasm (Figure 3A, arrows). We previously reported the alternating pattern of these BMAL1 and PER2 localizations, where BMAL1 is cytoplasmic in the morning (8:00 AM) and translocates into the nucleus at night (8:00 PM) (Zheng et al., 2014). All mice for the current study were euthanized at 1:00 PM which may explain the differential localization between PER2 and BMAL1 in our data. Finally, only a very weak STIM1 signal can be detected in Stim1 cKO ameloblasts, which confirms the validity of our experimental model (Figure 3C).
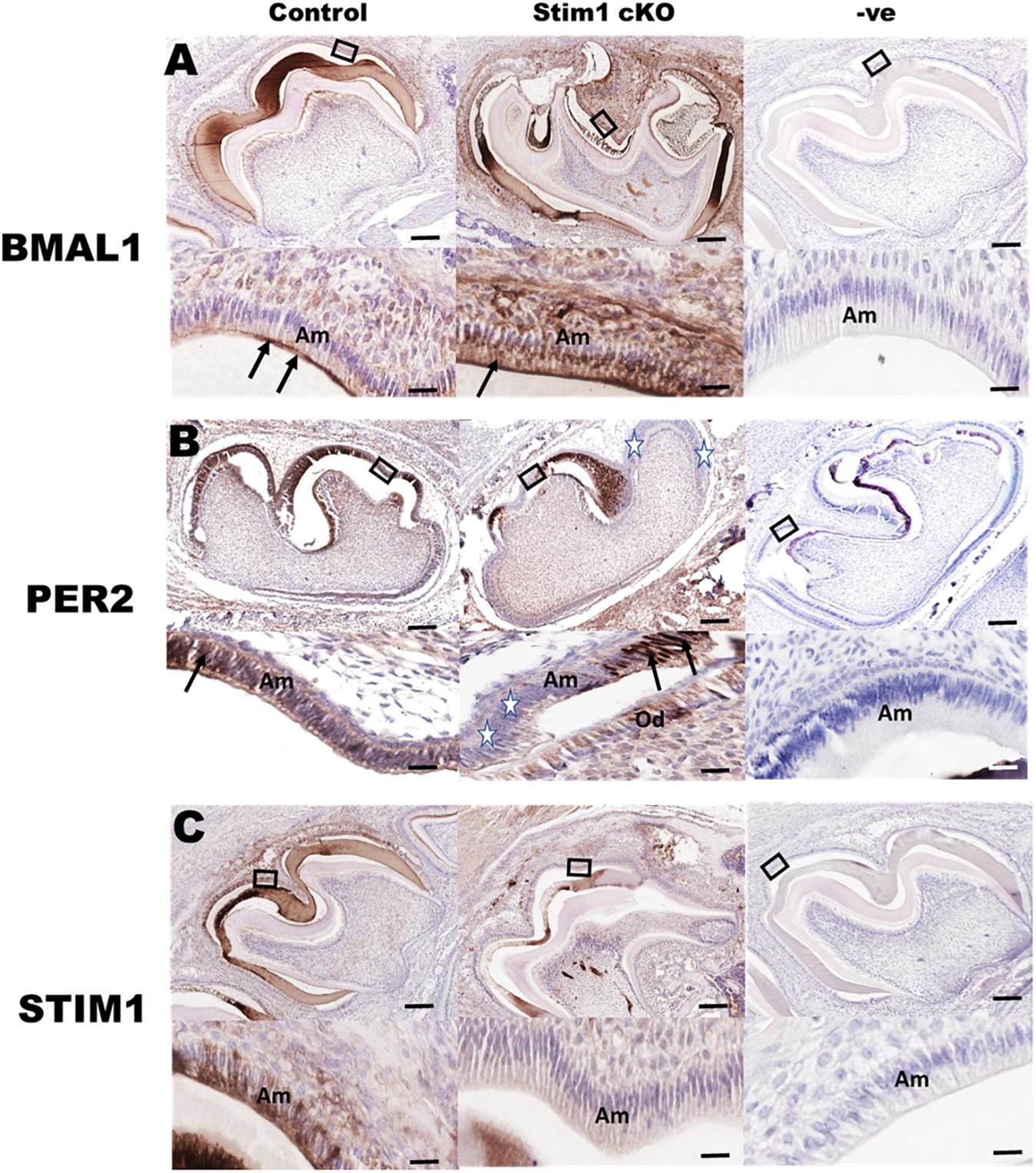
Figure 3. Immunohistochemical validation of gene expression data. Representative images of mouse molar sections stained using specific antibodies against BMAL1 (A), PER2 (B), and STIM1 (C). BMAL1 proteins levels are higher in the Stim1 cKO group compared to control and is mainly localized in the apical cytoplasm (arrows) of the ameloblasts. PER2 expression is weaker in the Stim1 cKO compared to control and is localized in the nucleus (arrows). Very weak STIM1 signal can be detected in Stim1 cKO ameloblasts which confirms the validity of our experimental model. The rectangular areas are enlarged in the lower panel of each image. White stars label the cells expressing very low levels of Per2. Am, ameloblasts; Od, odontoblasts. Scale bars, 250 μm in upper panels, 50 μm in lower panels.
Discussion
In this study we investigated the possible links between two important molecular regulators of amelogenesis, SOCE and the circadian clock. Using the Stim1 cKO model, we demonstrated that enamel specific disruption of SOCE had profound effects on the ameloblasts’ circadian gene profile as Stim1-deficient ameloblasts showed an altered expression of several key clock genes including Bmal1, Per2, Ror-a, and Fbxl3. Furthermore, our analyses demonstrated that SOCE disruption affected the expression levels of other circadian regulators that are known to be involved in regulating tooth morphogenesis and ameloblasts differentiation, such as p38 MAPK and TGF- β1. Collectively, these data strongly implicate SOCE as a vital modulator of the ameloblasts’ circadian clock. To the best of our knowledge, this is the first study to show evidence of a direct interplay between circadian clock and calcium signaling in ameloblasts.
Our PCR RNA array, qRT-PCR, and IHC analyses of core clock genes revealed that Stim1 knockdown had significantly affected the expression of the core circadian genes Bmal1 (upregulated) and Per2 (downregulated). Both genes serve as essential components of the autoregulatory mammalian clock gene network. BMAL1 protein heterodimerizes in the cytoplasm with the other circadian activator CLOCK (or NPAS2 in neurons) and then translocate into the nucleus where they enhance the expression of their repressors PER 1&2 and CRY 1&2 via binding to an E-box sequence in their promoter region (Ye et al., 2014). Subsequently, PER and CRY proteins multimerize with another core circadian regulator, casein kinase I δ in the cytoplasm and enter the nucleus to inhibit the activity of BMAL1/CLOCK complexes (St. John et al., 2014). Both genes are involved in the circadian regulation of several physiological (e.g., bone metabolism) and pathological processes (e.g., development of cancer) (Adeola et al., 2019; Janjiæ and Agis, 2019). In enamel, we have previously demonstrated that both Bmal1 and Per2 are robustly expressed by pre-ameloblast and differentiating ameloblasts in both human and mice embryonic teeth in addition to mice postnatal teeth (Zheng et al., 2011, 2014). We and others have also shown that the expression levels and localization of their protein products in ameloblasts regularly alternates in a circadian manner (Lacruz et al., 2012a; Zheng et al., 2013). Preliminary in vivo and vitro analysis from our lab revealed that both genes may play a major role in regulating the expression of key enamel matrix proteins such as amelogenin, and proteases such as Kallikrein 4 (Klk4). Moreover, our preliminary in vivo analysis of Per2 KO mice teeth showed abnormal enamel matrix formation associated with defective hypomineralized AI phenotype similar to AI observed in our Stim1 cKO (unpublished data). All of the above-mentioned observations indicate that STIM1-caused dysregulation of the circadian clock network may lead to impaired enamel formation. Thus, the SOCE-mediated circadian disruption may be critical in the development of AI observed in patients with STIM1/ORAI1 loss of function mutations.
Calcium signaling is particularly important in regulating the circadian expression of Per1&2 genes as their promoters contain functional CREs (cAMP/Ca2+-response elements) (Shim et al., 2007). In vivo and in vitro studies of the SCN showed that calcium influx induces the activity of Ca2+/calmodulin-dependent protein kinase II (CaMKII), resulting in the activation of protein kinase A (PKA), and protein kinase C (PKC). These kinases then phosphorylate CREB in a circadian manner (Agostino et al., 2004; Hegazi et al., 2019). The phosphorylated CREB translocates into the nucleus where it recognizes the CRE of the Per genes promoters and induces their expression (Lee et al., 2010). Furthermore, it has been shown that SOCE is essential for activating CREB in multiple tissues including smooth muscles and immune cells (Avila-Medina et al., 2018; Martin-Romero et al., 2018). The fact that SOCE-deficient ameloblast showed significantly lowered levels of the Per2 genes suggests that control of CREB activity may represent one of the main mechanisms by which SOCE can directly affect the circadian TTFLs in ameloblasts, similar to the SCN and other cells (Figure 4). Indeed, other researchers have also postulated that SOCE may be one of the intracellular modulators that links calcium to the circadian clock both centrally and peripherally (Noguchi et al., 2012, 2017; O’Neill and Reddy, 2012). Additional studies are needed in ameloblasts to evaluate why SOCE disruption affected the Per2 gene much more than Per1. Nevertheless, we postulate that Per2 downregulation, which results in Bmal1 upregulation, is one of the main molecular mechanisms involved in the Stim1 cKO-caused AI.
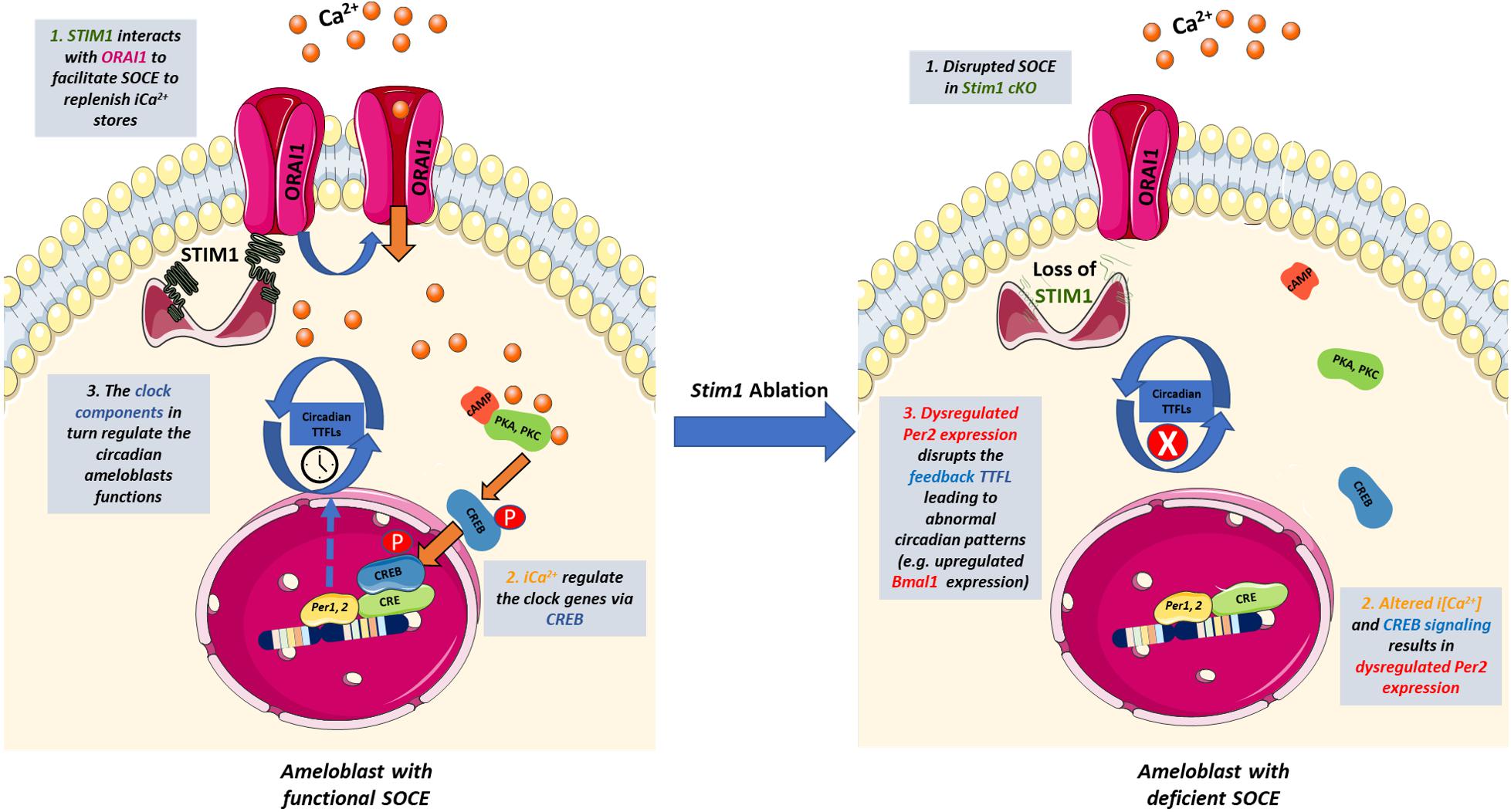
Figure 4. The potential cross-talk between SOCE and the circadian clock in ameloblasts. Schematic representation summarizing how SOCE can potentially influence the ameloblasts’ circadian clock based on our findings. Upon Ca2+ depletion in the ER, STIM1 binds to ORAI to facilitate Ca2+ entry and activate Ca2+-dependent signal transduction. Calcium induces the activity of Ca2+/calmodulin-dependent protein kinases which activate PKA and PKC that phosphorylate CREB in a circadian manner. The phosphorylated CREB translocate to the nucleus where it recognizes the cAMP Response Element in the promoter region of Per1 & 2 and serves as a transcription factor. PER1 & 2 are core regulators of the mammalian circadian clock and participate in forming the autoregulatory TTFL. Disruption of SOCE in Stim1 cKO ameloblasts resulted in dysregulated expression of Per2 that disrupts the TTFLs leading to abnormal circadian patterns including an upregulated Bmal1 expression that maybe involved in the development of SOCE mediated AI. iCa2+, intracellular calcium; TTFL, transcription translation feedback loop; PKA, protein Kinase A; PKC, protein Kinase C; CREB, cAMP response element-binding protein; STIM1, Stromal interaction molecule 1; P, Phosphate group. This figure was created with images adapted from Servier Medical Art by Servier. Original images are licensed under a Creative Commons Attribution 3.0 Unported License.
Another key finding from our work is the observation that Stim1 cKO ameloblasts showed higher level of Fbxl3 expression, a key component of the circadian clock. FBXL3 protein plays an important role in the negative feedback loop of the mammalian molecular circadian rhythm. In the nucleus, the FBXL3 protein targets the CRY2 proteins by ubiquitination to prevent them from inactivating BMAL1 (Huber et al., 2016). Moreover, FBXL3 plays a vital role in promoting Bmal1 transcription by inactivating the Bmal1 suppressor, REV-ERB alpha (Shi et al., 2013). Thus, upregulated levels of Fbxl3 expression might also be one of the ways that lead to the over-expression of Bmal1 in Stim1 cKO ameloblast.
The use of PCR arrays to investigate the genes that are differentially expressed in SOCE-deficient ameloblasts has brought forward genes that are also known to play a role in ameloblast differentiation and tooth morphogenesis, including Tgf-β1 and Mapk14. TGF-β1 is a multi-functional growth factor with important roles in several biological processes, such as cellular proliferation and embryonic development, in addition to its role in the circadian clock signaling (Xu et al., 2018). Our RNA array and qRT-PCR analyses revealed that Tgf-β1 is significantly downregulated in SOCE-deficient ameloblasts. Several previous studies had showed that SOCE plays an important role in regulating the TGF pathway activity in healthy and cancerous tissues (Chaudhari et al., 2017; Bhattacharya et al., 2018). In the context of amelogenesis, TGF-β1 is known to play a key role in the normal enamel development, and similar to STIM1 it appears to play an important role in regulating ameloblast function during enamel maturation through its interactions with Klk4 and matrix metalloprotease 20 (Kobayashi-Kinoshita et al., 2016). In fact, Cho et al. (2013) has shown that ameloblast specific deletion of its receptors has resulted in hypomature AI enamel phenotype with decreased mineral content concomitant with increased attrition and thinner enamel crystallites. This phenotype is not unsimilar to the enamel phenotype observed in other Stim1 cKO mice (Eckstein et al., 2017; Furukawa et al., 2017; Said et al., 2019), suggesting that TGF-β1 dysregulation might be partly involved in the development of Stim1 deletion-caused AI. On the other hand, Mapk14 expression was significantly upregulated in Stim1 deficient ameloblasts. MAPK14 has been shown to regulate SOCE both indirectly (through TGF-β1 and NF-kappa) or directly by phosphorylating Stim1 (Martin-Romero et al., 2018). In ameloblasts, MAPK14 has been shown to play a role in regulating early tooth morphogenesis and its ectodermal tissue deletion results in abnormally shaped dental cusps and a profoundly hypoplastic enamel layer (Greenblatt et al., 2015).
Additionally, the present RNA profile studies revealed that SOCE disruption altered the expression of other circadian transcription factors with currently unknown functions in amelogenesis. These include: transcription Factor AP-2 Alpha (TFA2PA), nuclear receptor subfamily 2 group F member 6 (NR2F6), and early growth response proteins 1&3 (EGR1, EGR3). TFA2PA is expressed in ectoderm and migrating neural crest cell lineages, and plays an important role in early craniofacial morphogenesis (Mitchell et al., 1991). Liu et al. (2015) showed that TFA2PA is highly expressed during the presecretory stage of amelogenesis, and suggested that it participates in the differentiation of pre-ameloblasts to secretory ameloblast. Similar to STIM1, both EGR1, and NR2F6 were shown to be highly expressed during the maturation stage, yet their exact roles in amelogenesis are poorly understood (Tsuchiya et al., 2009; Lacruz et al., 2011). Moreover, EGR1 is a major regulator of STIM1 and its expression is induced by TGF-β1 in the maturational ameloblasts which may explain why it is downregulated in Stim1 cKO (Tsuchiya et al., 2009; Samakai et al., 2016). These preliminary findings and related hypotheses need further confirmation and will be part of future studies. Nevertheless, we can conclude that the apparent differential stage-specific regulation of circadian clock related genes (downregulation of Tgf-β1, Egr1, and Nr2f6 which are expressed in maturation stage, and upregulation of Mapk14 and Tfa2pa which are expressed in pre-secretory and secretory stage) in Stim1 cKO teeth supports the idea of complex downstream effects of altered SOCE signaling in ameloblasts. Our data suggests that SOCE phenotypic and genotypic effects throughout amelogenesis may be produced by directly modulating downstream molecular signaling pathways and by contributing to the circadian clock system dysregulation observed in this model.
The essential role of calcium signaling in regulating the circadian clock has been demonstrated in several studies which have reported that changes in the intracellular [Ca2+] not only contribute directly to the timekeeping mechanism of the central and peripheral clocks, but also that the intracellular [Ca2+] is regulated by the circadian clock suggesting complex regulatory feedback mechanisms between the two systems (Honma and Honma, 2003; Lundkvist et al., 2005; Enoki et al., 2017; Martí Ruiz et al., 2018). Consistently, two studies by Noguchi et al. (2012, 2017) demonstrated how autonomous circadian oscillations in i[Ca2+] correlated with the autonomous rhythmicity of PER2 expression in murine primary fibroblasts and the SCN neurons, and that both PER2 and intracellular[Ca2+] rhythms were abolished in SCN cells deficient in Bmal1. In addition, Chen et al. (2016) reported that CLOCK-BMAL1 heterodimers regulate the expressions and functions of the cardiac L-type calcium channel which play an important role in the cardiac electrogenesis and arrhythmogenesis. Moreover, it was found that Ubiquitin-Specific Protease 2 (Usp2), which is a clock output effector, is involved in regulating bodily Ca2+ homeostasis via controlling the expression of the intestinal Ca2+ channels (Pouly et al., 2016). All the above suggests that an inverse regulation (i.e., the circadian clock regulates calcium homeostasis and rhythmicity) may also exist. However, most of the studies examining this correlation have been conducted in the SCN. The crosstalk between calcium signaling and circadian pathways in the peripheral clocks remain greatly understudied. Our report further contributes in understanding the chronobiology of circadian peripheral clocks and the links to intracellular [Ca2+] signaling. More studies are needed to examine the role of circadian clock in directing Ca2+ rhythmicity and signaling in ameloblasts.
In conclusion, in this work we analyzed the circadian gene profiles in the ameloblasts collected from a SOCE-deficient teeth. We uncovered how SOCE disruption resulted in the dysregulation of multiple circadian rhythm genes which may have a direct impact on certain aspects of STIM1 AI. Our study provides further evidence that ameloblast activity is tightly controlled by several molecular and circadian pathways, and highlights the need to investigate their interactions more thoroughly to achieve a better understanding of amelogenesis. Future studies including additional functional assays are needed to further examine the potential molecular crosslinks between calcium dynamics and the circadian pathways in ameloblasts.
Data Availability Statement
All datasets presented in this study are included in the article/Supplementary Material.
Ethics Statement
The animal study was reviewed and approved by University Animal Care Committee (UACC) of the University of Saskatchewan, Saskatoon, SK, Canada.
Author Contributions
RS, SP, and PP conceived the study. RS performed the experimental procedures, data analysis, experimental designing, and manuscript writing. LL helped with the experimental procedures and data analysis, and provided scientific support. SP and PP provided scientific support and contributed to the experimental design, data analysis, and manuscript writing. All authors read and approved the submitted version of the manuscript.
Funding
Funding is provided by NSERC Development Grant (RGPIN-2020-06025), University of Saskatchewan, College of Dentistry start-up funds and SHRF Establishment Grant to PP. University of Saskatchewan College of Medicine start-up funds to SP.
Conflict of Interest
The authors declare that the research was conducted in the absence of any commercial or financial relationships that could be construed as a potential conflict of interest.
Supplementary Material
The Supplementary Material for this article can be found online at: https://www.frontiersin.org/articles/10.3389/fphys.2020.00920/full#supplementary-material
References
Adeola, H. A., Papagerakis, S., and Papagerakis, P. (2019). Systems biology approaches and precision oral health: a circadian clock perspective. Front. Physiol. 10:399. doi: 10.3389/fphys.2019.00399
Agostino, P. V., Ferreyra, G. A., Murad, A. D., Watanabe, Y., and Golombek, D. A. (2004). Diurnal, circadian and photic regulation of calcium/calmodulin-dependent kinase II and neuronal nitric oxide synthase in the hamster suprachiasmatic nuclei. Neurochem. Int. 44, 617–625. doi: 10.1016/j.neuint.2003.09.005
Athanassiou-Papaefthymiou, M., Kim, D., Harbron, L., Papagerakis, S., Schnell, S., Harada, H., et al. (2011). Molecular and circadian controls of ameloblasts: regulation of ameloblast differentiation. Eur. J. Oral Sci. 119, 35–40. doi: 10.1111/j.1600-0722.2011.00918.x
Avila-Medina, J., Mayoral-Gonzalez, I., Dominguez-Rodriguez, A., Gallardo-Castillo, I., Ribas, J., Ordoñez, A., et al. (2018). The complex role of store operated calcium entry pathways and related proteins in the function of cardiac, skeletal and vascular smooth muscle cells. Front. Physiol. 9:257. doi: 10.3389/fphys.2018.00257
Bailleul-Forestier, I., Davideau, J. L., Papagerakis, P., Noble, I., Nessmann, C., Peuchmaur, M., et al. (1996). Immunolocalization of vitamin D receptor and calbindin-D28k in human tooth germ. Pediatr. Res. 39, 636–642. doi: 10.1203/00006450-199604000-00013
Bawden, J. W. (1989). Calcium transport during mineralization. Anat. Rec. 224, 226–233. doi: 10.1002/ar.1092240212
Berdal, A., Papagerakis, P., Hotton, D., Bailleul-Forestier, I., and Davideau, J. L. (1995). Ameloblasts and odontoblasts, target-cells for 1,25-dihydroxyvitamin D3: a review. Int. J. Dev. Biol. 39, 257–262.
Bhattacharya, A., Kumar, J., Hermanson, K., Sun, Y., Qureshi, H., Perley, D., et al. (2018). The calcium channel proteins ORAI3 and STIM1 mediate TGF-β induced Snai1 expression. Oncotarget 9, 29468–29483. doi: 10.18632/oncotarget.25672
Chaudhari, S., Li, W., Wang, Y., Jiang, H., Ma, Y., Davis, M. E., et al. (2017). Store-operated calcium entry suppressed the TGF-β1/Smad3 signaling pathway in glomerular mesangial cells. Am. J. Physiol. Renal Physiol. 313, F729–F739. doi: 10.1152/ajprenal.00483.2016
Chen, Y., Zhu, D., Yuan, J., Han, Z., Wang, Y., Qian, Z., et al. (2016). CLOCK-BMAL1 regulate the cardiac L-type calcium channel subunit CACNA1C through PI3K-Akt signaling pathway. Can. J. Physiol. Pharmacol. 94, 1023–1032. doi: 10.1139/cjpp-2015-0398
Cho, A., Haruyama, N., Hall, B., Danton, M. J. S., Zhang, L., Arany, P., et al. (2013). TGF-ß regulates enamel mineralization and maturation through KLK4 expression. PLoS One 8:e82267. doi: 10.1371/journal.pone.0082267
Eckstein, M., and Lacruz, R. S. (2018). CRAC channels in dental enamel cells. Cell Calcium 75, 14–20. doi: 10.1016/j.ceca.2018.07.012
Eckstein, M., Vaeth, M., Fornai, C., Vinu, M., Bromage, T. G., Nurbaeva, M. K., et al. (2017). Store-operated Ca2+ entry controls ameloblast cell function and enamel development. JCI Insight 2:e91166. doi: 10.1172/jci.insight.91166
Enoki, R., Ono, D., Kuroda, S., Honma, S., and Honma, K. (2017). Dual origins of the intracellular circadian calcium rhythm in the suprachiasmatic nucleus. Sci. Rep. 7, 1–8. doi: 10.1038/srep41733
Fahrner, M., Schindl, R., and Romanin, C. (2018). “Studies of structure-function and subunit composition of Orai/STIM channel,” in Calcium Entry Channels in Non-Excitable Cells, eds J. A. Kozak and J. W. Putney (Boca Raton, FL: CRC Press).
Feske, S. (2019). CRAC channels and disease – from human CRAC channelopathies and animal models to novel drugs. Cell Calcium 80, 112–116. doi: 10.1016/j.ceca.2019.03.004
Furukawa, Y., Haruyama, N., Nikaido, M., Nakanishi, M., Ryu, N., Oh-Hora, M., et al. (2017). Stim1 regulates enamel mineralization and ameloblast modulation. J. Dent. Res. 96, 1422–1429. doi: 10.1177/0022034517719872
Greenblatt, M. B., Kim, J.-M., Oh, H., Park, K. H., Choo, M.-K., Sano, Y., et al. (2015). p38α MAPK Is required for tooth morphogenesis and enamel secretion. J. Biol. Chem. 290, 284–295. doi: 10.1074/jbc.M114.599274
Hastings, M. H., Maywood, E. S., and Brancaccio, M. (2018). Generation of circadian rhythms in the suprachiasmatic nucleus. Nat. Rev. Neurosci. 19, 453–469. doi: 10.1038/s41583-018-0026-z
Hegazi, S., Lowden, C., Rios Garcia, J., Cheng, A. H., Obrietan, K., Levine, J. D., et al. (2019). A symphony of signals: intercellular and intracellular signaling mechanisms underlying circadian timekeeping in mice and flies. IJMS 20:2363. doi: 10.3390/ijms20092363
Herzog, E. D., Hermanstyne, T., Smyllie, N. J., and Hastings, M. H. (2017). Regulating the suprachiasmatic nucleus (SCN) circadian clockwork: interplay between cell-autonomous and circuit-level mechanisms. Cold Spring Harb. Perspect. Biol. 9:a027706. doi: 10.1101/cshperspect.a027706
Honma, S., and Honma, K. (2003). The biological clock: Ca2+ links the pendulum to the hands. Trends Neurosci. 26, 650–653. doi: 10.1016/j.tins.2003.09.012
Hoth, M., and Niemeyer, B. A. (2013). The neglected CRAC proteins: Orai2, Orai3, and STIM2. Curr. Top. Membr. 71, 237–271. doi: 10.1016/B978-0-12-407870-3.00010-X
Houari, S., Babajko, S., Loiodice, S., Berdal, A., and Jedeon, K. (2018). Micro-dissection of enamel organ from mandibular incisor of rats exposed to environmental toxicants. J. Vis. Exp. 133:57081. doi: 10.3791/57081
Huang, D. W., Sherman, B. T., and Lempicki, R. A. (2009). Systematic and integrative analysis of large gene lists using DAVID bioinformatics resources. Nat. Protoc. 4, 44–57. doi: 10.1038/nprot.2008.211
Hubbard, M. J. (2000). Calcium transport across the dental enamel epithelium. Crit. Rev. Oral Biol. Med. 11, 437–466. doi: 10.1177/10454411000110040401
Huber, A.-L., Papp, S. J., Chan, A. B., Henriksson, E., Jordan, S. D., Kriebs, A., et al. (2016). CRY2 and FBXL3 cooperatively degrade c-MYC. Mol. Cell 64, 774–789. doi: 10.1016/j.molcel.2016.10.012
Janjiæ, K., and Agis, H. (2019). Chronodentistry: the role and potential of molecular clocks in oral medicine. BMC Oral Health 19:32. doi: 10.1186/s12903-019-0720-x
Kobayashi-Kinoshita, S., Yamakoshi, Y., Onuma, K., Yamamoto, R., and Asada, Y. (2016). TGF-β1 autocrine signalling and enamel matrix components. Sci. Rep. 6, 1–14. doi: 10.1038/srep33644
Lacruz, R. S. (2017). Enamel: molecular identity of its transepithelial ion transport system. Cell Calcium 65, 1–7. doi: 10.1016/j.ceca.2017.03.006
Lacruz, R. S., Hacia, J. G., Bromage, T. G., Boyde, A., Lei, Y., Xu, Y., et al. (2012a). The circadian clock modulates enamel development. J. Biol. Rhythms 27, 237–245. doi: 10.1177/0748730412442830
Lacruz, R. S., Smith, C. E., Bringas, P., Chen, Y.-B., Smith, S. M., Snead, M. L., et al. (2012b). Identification of novel candidate genes involved in mineralization of dental enamel by genome-wide transcript profiling. J. Cell. Physiol. 227, 2264–2275. doi: 10.1002/jcp.22965
Lacruz, R. S., Smith, C. E., Chen, Y.-B., Hubbard, M. J., Hacia, J. G., and Paine, M. L. (2011). Gene expression analysis of early and late maturation stage rat enamel organ. Eur. J. Oral Sci. 119, 149–157. doi: 10.1111/j.1600-0722.2011.00881.x
Lee, Y., Lee, J., Kwon, I., Nakajima, Y., Ohmiya, Y., Son, G. H., et al. (2010). Coactivation of the CLOCK–BMAL1 complex by CBP mediates resetting of the circadian clock. J. Cell Sci. 123, 3547–3557. doi: 10.1242/jcs.070300
Liu, C., Niu, Y., Zhou, X., Xu, X., Yang, Y., Zhang, Y., et al. (2015). Cell cycle control, DNA damage repair, and apoptosis-related pathways control pre-ameloblasts differentiation during tooth development. BMC Genomics 16:592. doi: 10.1186/s12864-015-1783-y
Lundkvist, G. B., Kwak, Y., Davis, E. K., Tei, H., and Block, G. D. (2005). A calcium flux is required for circadian rhythm generation in mammalian pacemaker neurons. J. Neurosci. 25, 7682–7686. doi: 10.1523/JNEUROSCI.2211-05.2005
Martí Ruiz, M. C., Hubbard, K. E., Gardner, M. J., Jung, H. J., Aubry, S., Hotta, C. T., et al. (2018). Circadian oscillations of cytosolic free calcium regulate the Arabidopsis circadian clock. Nat. Plants 4, 690–698. doi: 10.1038/s41477-018-0224-8
Martin-Romero, F. J., Pascual-Caro, C., Lopez-Guerrero, A., Espinosa-Bermejo, N., and Pozo-Guisado, E. (2018). “Regulation of calcium signaling by STIM1 and ORAI1,” in Calcium and Signal Transduction, ed. J. N. Buchholz (London: Intech Open), doi: 10.5772/intechopen.78587
Mitchell, P. J., Timmons, P. M., Hébert, J. M., Rigby, P. W., and Tjian, R. (1991). Transcription factor AP-2 is expressed in neural crest cell lineages during mouse embryogenesis. Genes Dev. 5, 105–119. doi: 10.1101/gad.5.1.105
Noguchi, T., Leise, T. L., Kingsbury, N. J., Diemer, T., Wang, L. L., Henson, M. A., et al. (2017). Calcium circadian rhythmicity in the suprachiasmatic nucleus: cell autonomy and network modulation. eNeuro 4:ENEURO.0160-17.2017. doi: 10.1523/ENEURO.0160-17.2017
Noguchi, T., Wang, C. W., Pan, H., and Welsh, D. K. (2012). Fibroblast circadian rhythms of PER2 expression depend on membrane potential and intracellular calcium. Chronobiol. Int. 29, 653–664. doi: 10.3109/07420528.2012.679330
Nurbaeva, M. K., Eckstein, M., Concepcion, A. R., Smith, C. E., Srikanth, S., Paine, M. L., et al. (2015a). Dental enamel cells express functional SOCE channels. Sci. Rep. 5:15803. doi: 10.1038/srep15803
Nurbaeva, M. K., Eckstein, M., Feske, S., and Lacruz, R. S. (2017). Ca 2+ transport and signalling in enamel cells: calcium in enamel cells. J. Physiol. 595, 3015–3039. doi: 10.1113/JP272775
Nurbaeva, M. K., Eckstein, M., Snead, M. L., Feske, S., and Lacruz, R. S. (2015b). Store-operated Ca 2+ entry modulates the expression of enamel genes. J. Dent. Res. 94, 1471–1477. doi: 10.1177/0022034515598144
O’Neill, J. S., and Reddy, A. B. (2012). The essential role of cAMP/Ca2+ signalling in mammalian circadian timekeeping. Biochem. Soc. Trans. 40, 44–50. doi: 10.1042/BST20110691
Palacios-Muñoz, A., and Ewer, J. (2018). Calcium and cAMP directly modulate the speed of the Drosophila circadian clock. PLoS Genet. 14:e1007433. doi: 10.1371/journal.pgen.1007433
Papagerakis, S., Zheng, L., Schnell, S., Sartor, M. A., Somers, E., Marder, W., et al. (2014). The circadian clock in oral health and diseases. J. Dent. Res. 93, 27–35. doi: 10.1177/0022034513505768
Partch, C. L., Green, C. B., and Takahashi, J. S. (2014). Molecular architecture of the mammalian circadian clock. Trends Cell Biol. 24, 90–99. doi: 10.1016/j.tcb.2013.07.002
Pouly, D., Chenaux, S., Martin, V., Babis, M., Koch, R., Nagoshi, E., et al. (2016). USP2-45 is a circadian clock output effector regulating calcium absorption at the post-translational level. PLoS One 11:e0145155. doi: 10.1371/journal.pone.0145155
Prakriya, M., and Lewis, R. S. (2015). Store-operated calcium channels. Physiol. Rev. 95, 1383–1436. doi: 10.1152/physrev.00020.2014
Said, R., Zheng, L., Saunders, T., Zeidler, M., Papagerakis, S., and Papagerakis, P. (2019). Generation of amelx-iCre mice supports ameloblast-specific role for Stim1. J. Dent. Res. 98, 1002–1010. doi: 10.1177/0022034519858976
Samakai, E., Hooper, R., Martin, K. A., Shmurak, M., Zhang, Y., Kappes, D. J., et al. (2016). Novel STIM1−dependent control of Ca 2+ clearance regulates NFAT activity during T−cell activation. FASEB J. 30, 3878–3886. doi: 10.1096/fj.201600532R
Shi, G., Xing, L., Liu, Z., Qu, Z., Wu, X., Dong, Z., et al. (2013). Dual roles of FBXL3 in the mammalian circadian feedback loops are important for period determination and robustness of the clock. Proc. Natl. Acad. Sci. U.S.A. 110, 4750–4755. doi: 10.1073/pnas.1302560110
Shim, H. S., Kim, H., Lee, J., Son, G. H., Cho, S., Oh, T. H., et al. (2007). Rapid activation of CLOCK by Ca2+-dependent protein kinase C mediates resetting of the mammalian circadian clock. EMBO Rep. 8, 366–371. doi: 10.1038/sj.embor.7400920
Simmer, J. P., Papagerakis, P., Smith, C. E., Fisher, D. C., Rountrey, A. N., Zheng, L., et al. (2010). Regulation of dental enamel shape and hardness. J. Dent. Res. 89, 1024–1038. doi: 10.1177/0022034510375829
St. John, P. C., Hirota, T., Kay, S. A., and Doyle, F. J. (2014). Spatiotemporal separation of PER and CRY posttranslational regulation in the mammalian circadian clock. Proc. Natl. Acad. Sci. U.S.A. 111, 2040–2045. doi: 10.1073/pnas.1323618111
Takahashi, J. S. (2017). Transcriptional architecture of the mammalian circadian clock. Nat. Rev. Genet. 18, 164–179. doi: 10.1038/nrg.2016.150
Tonelli, F. M. P., Santos, A. K., Gomes, D. A., da Silva, S. L., Gomes, K. N., Ladeira, L. O., et al. (2012). Stem cells and calcium signaling. Adv. Exp. Med. Biol. 740, 891–916. doi: 10.1007/978-94-007-2888-2_40
Tsuchiya, M., Sharma, R., Tye, C. E., Sugiyama, T., and Bartlett, J. D. (2009). TGF-β1 expression is up-regulated in maturation stage enamel organ and may induce ameloblast apoptosis. Eur. J. Oral Sci. 117, 105–112. doi: 10.1111/j.1600-0722.2009.00612.x
Xu, X., Zheng, L., Yuan, Q., Zhen, G., Crane, J. L., Zhou, X., et al. (2018). Transforming growth factor-β in stem cells and tissue homeostasis. Bone Res. 6:2. doi: 10.1038/s41413-017-0005-4
Ye, R., Selby, C. P., Chiou, Y.-Y., Ozkan-Dagliyan, I., Gaddameedhi, S., and Sancar, A. (2014). Dual modes of CLOCK:BMAL1 inhibition mediated by cryptochrome and period proteins in the mammalian circadian clock. Genes Dev. 28, 1989–1998. doi: 10.1101/gad.249417.114
Zheng, L., Ehardt, L., McAlpin, B., About, I., Kim, D., Papagerakis, S., et al. (2014). The tick tock of odontogenesis. Exp. Cell Res. 325, 83–89. doi: 10.1016/j.yexcr.2014.02.007
Zheng, L., Papagerakis, S., Schnell, S. D., Hoogerwerf, W. A., and Papagerakis, P. (2011). Expression of clock proteins in developing tooth. Gene Expr. Patterns 11, 202–206. doi: 10.1016/j.gep.2010.12.002
Zheng, L., Seon, Y. J., Mourão, M. A., Schnell, S., Kim, D., Harada, H., et al. (2013). Circadian rhythms regulate amelogenesis. Bone 55, 158–165. doi: 10.1016/j.bone.2013.02.011
Keywords: ameloblast, enamel, STIM1, calcium, store operated Ca2+ channels, circadian clock, amelogenesis, amelogenesis imperfecta
Citation: Said R, Lobanova L, Papagerakis S and Papagerakis P (2020) Calcium Sets the Clock in Ameloblasts. Front. Physiol. 11:920. doi: 10.3389/fphys.2020.00920
Received: 14 April 2020; Accepted: 09 July 2020;
Published: 31 July 2020.
Edited by:
Maisa Hanna-Maija Seppala, King’s College London, United KingdomReviewed by:
Marianna Bei, Harvard Medical School, United StatesJavier Catón, Universidad Complutense de Madrid, Spain
Copyright © 2020 Said, Lobanova, Papagerakis and Papagerakis. This is an open-access article distributed under the terms of the Creative Commons Attribution License (CC BY). The use, distribution or reproduction in other forums is permitted, provided the original author(s) and the copyright owner(s) are credited and that the original publication in this journal is cited, in accordance with accepted academic practice. No use, distribution or reproduction is permitted which does not comply with these terms.
*Correspondence: Petros Papagerakis, petros.papagerakis@usask.ca