- 1Department of Agricultural and Biosystems Engineering, Iowa State University, Ames, IA, United States
- 2Department of Biosystems and Agricultural Engineering, University of Kentucky, Lexington, KY, United States
- 3Department of Biological Systems Engineering, University of Nebraska-Lincoln, Lincoln, NE, United States
- 4United State Department of Agriculture (USDA) Agricultural Research Service (ARS), U.S. Meat Animal Research Center, Clay Center, NE, United States
Swine heat production (HP) data are an essential element of numerous aspects affecting swine production sustainability, such as, housing environmental control design, energetics and thermoregulation modeling, as well as understanding of feed energy partitioning. Accurate HP values that reflect the continuous advances in growth, nutrition, health, and reproduction are needed to update outdated models and data; hence, this review of swine HP values is a critical contribution. This review updates the last previous review conducted in 2004, by reviewing literature from growing and breeding pigs from 2003 to 2020. In total, 33 references were identified that provided relevant HP data and from these references, 192 records were identified for pigs ranging in weight from 12.5 to 283 kg and exposed to temperatures between 12.0°C and 35.5°C. For growing pigs at thermoneutral conditions, a 4.7% average increase in HP was observed compared to HP data summarized from 1988 to 2004. Only five records were identified for gestating sows and the 43 records for lactating sows plus litter. This sow data shows high variability and inconsistent trends with temperature, most likely attributed to variation in experimental protocols, management, and limited reported information. There is still a lack of data on growing pigs greater than 105 kg, gilts and gestating sows housed in different systems (stall, pen, mixed, etc.), and latent HP values that reflect different housing systems. Further, there is a need to standardize reporting of HP values (with an example provided) across different disciplines to drive documentation of increased swine production efficiency, environmental control design, and energetics modeling.
1 Introduction
The global swine industry is constantly evolving as genetic potential, management, nutrition, health, consumer demand, and numerous other factors change over time. For example, animal growth in U.S. production systems continues to get faster and more efficient as indicated by an average 6.2 days less on feed, 0.045 kg (0.1 lb) greater average daily gain, and 0.07 lower feed conversion efficiency for wean-finish systems from 2011 to 2019 (Stalder, 2018; MetaFarms, 2021). Selection of efficient sows has led to an 80 g increase in birth weight per market piglet from 2013 to 2018 (PIC, 2018) as well as larger litter sizes. (MetaFarms, 2021). A consequence of these substantial productivity improvements is increased metabolic heat production (HP; Mayorga et al., 2019).
Pigs are homeothermic animals that use physiological and behavioral mechanisms to keep a near-constant body temperature (Mount, 1968). The thermal energy balance of the pig is maintained such that the total metabolic heat produced must be dissipated to the environment, or a change in body temperature occurs. Metabolizable energy in feed is used for maintenance, growth, and production, which generates metabolic heat as a by-product. This internally generated heat is conducted and circulated through the animal to its outer surface where it is dissipated to the environment (Close and Mount, 1978). Pigs uniquely thermoregulate (i.e., adjust the flow of metabolic generated heat to the environment) compared to other mammals with body weight (BW) and maturity having an important impact on thermoregulation capabilities (Curtis, 1983). Young pigs have minimal metabolic heat production, lack vasomotor control to regulate conductance of heat from their core body to surface, are hairless, and lack subcutaneous muscle and fat, as well as have a high surface area to volume ratio (Heath, 1983; Herpin et al., 2002). This combination results in high heat loss compared to body weight and metabolic HP leading to increased susceptibility of chilling (Ramirez et al., 2022). Pigs also have no functional sweat glands, limited lung capacity (small tidal volumes for respiration), and a thick subcutaneous adipose tissue layer (Curtis, 1983). This combination results in limited ability to thermoregulate (dissipate heat) for heavier, more mature animals which have a greater metabolic activity due to high feeding level, relative to maintenance requirements (Gourdine et al., 2021). This issue is further exacerbated in sows which are heavy, a low surface area to body weight ratio, higher energy intake relative to maintenance requirement (in lactating sows), and higher subcutaneous fat tissues (Renaudeau et al., 2013). Continual changes in swine genetics and nutrition impact these numerous thermoregulation abilities and subsequently heat production and ability to dissipate heat.
Typically, environmental conditions are colder than the body temperature of the animal; hence, heat is lost to the environment and the rate of heat loss is affected by environmental heat demand (DeShazer et al., 2009). Energy exchange from the outer surface of the animal to the environment occurs via sensible (temperature gradient dependent) and latent (water vapor pressure gradient dependent) modes (Curtis, 1983). Sensible heat loss is described by convection, conduction, and radiation and latent heat loss is characterized by the evaporation of water, primarily through respiratory exchange (DeShazer et al., 2009). From an environmental control perspective, the sensible and latent heat losses from the animals are considered production (i.e., generation) terms since they add energy (in the form of heat) to the environment (e.g., air, floor, room, chamber, etc.) that needs to be controlled (Albright, 1990). This may create confusion when the animal is considered the control volume, since metabolically generated heat is entirely in the sensible form. The following sections use the terminology of total HP (equivalent to metabolic heat production) as well as sensible and latent HP to describe the partitions of heat lost to the environment from the pigs.
Brown-Brandl et al. (2004) showed HP (total) to have increased by 16% compared to studies from the 1950s to 1970s. This was mainly attributed to increased feed conversion and average daily gain. In addition, changes in the chemical composition of body weight gain (greater lean deposition and lower fat deposition) and thermic effect of feed have led to an elevation of fasting HP. For early and late gestation sows, Stinn and Xin (2014) showed increases of 35% and 12% in HP, 72% and 34% in latent heat production (LHP), and 19% and 3% in sensible heat production (SHP), respectively, when also compared to studies from the 1950s and 1970s. As HP increases, and subsequently, the thermoneutral zone shifts colder (subsequently increasing susceptibility to heat stress), design and management of environment control systems for intensive swine housing or transportation trailers must be updated to adequately provide ventilation and an environment to meet the needs of modern swine genetics.
Pigs raised in intensive housing systems or transported in climatized trailers require controlled environments that balance energy usage, productivity, animal well-being, and environmental impact (Bracke et al., 2020). The basis for environmental control is adequately supplying the proper fresh-air air exchange rate (or ventilation) to remove heat, moisture, noxious gases, and other airborne contaminants produced inside the housing (Albright, 1990). The SHP is a major contribution to calculating the ventilation rate for temperature control; hence, increases in pig SHP will increase the required maximum ventilation in hot weather. Conversely, design ventilation rates from moisture control based on outdated moisture production (derived from LHP) data have been shown to be between 30% to 69% lower than newly calculated ventilation rates (Lu et al., 2017).
Due to the constant evolution in swine industry and need for improved environmental control for intensive swine housing and transport, the goal of this review article is to discuss changes in growing and breeding pigs’ heat production from 2003 to 2020. This is an update of a similar review performed by Brown-Brandl et al. (2004) which documented swine HP changes from the 1950s to 2003. Further, this review expands the scope of Brown-Brandl et al. (2004), which was limited to growing pigs, to include lactating sow heat production, which has not been previously summarized in literature. The consistent changes in the swine industry (Davis et al., 2022) have uniquely warranted this review to help provide a summary of recent HP data to guide future design of environmental control systems for different applications of swine housing.
2 Article search, screening, and selection
Peer-reviewed articles were identified by systematic search in electronic literature databases including Web of Science (all databases) and Google Scholar on February 1, 2021. Search terms included the following within the title or abstract fields: (swine OR porcine* OR sow* OR pig* OR hog* OR boar) AND (“heat production”) NOT (waste*). These terms address themes related to energetics, nutrition, climate physiology, and environmental management. Articles identified by the searches were in Google Scholar reference manager and duplicates were automatically detected and removed followed by manual removal of any additional duplicates (i.e., publications published in more than one format, or indexed in more than one database).
Literature was screened according to the schematic framework following the Preferred Reporting Items for Systematic reviews and Meta-Analysis (PRISMA) guidelines (Page et al., 2021). Peer-reviewed articles were excluded if other animal species, except swine, were the focus, and publication was in a language other than English. Each title and abstract were evaluated using the following criteria:
a. Date of publication: articles published from 2003 and 2020 (inclusive)
b. Geographic focus: worldwide
c. Population: applied to individual pigs or groups
d. Results: included total heat production
e. Facility style: measurements made at house-level or calorimeter
For each selected article, a record was made in a Microsoft Excel spreadsheet documenting: (1) days of exposure, (2) feeding program, (3) calorimeter type, (4) number of pigs, (5) body weight (divided into lower, upper, and average), (6) sex, (7), dry-bulb temperature, (8) dew-point temperature, (9) relative humidity, (10) airspeed, (11) feed energy, (12) feed intake, (13) manure handling, (14) fasting heat production, and (15) total heat production. Reported unit of measure for heat production data varied considerably throughout the literature. All heat production data were converted to MJ d-1 kg-0.75, W kg-1, and W to accommodate the convention of different disciplines.
The Web of Science search yielded 24 results while the first 15 pages (150 results) of Google Scholar were parsed on pig-related studies focusing on heat production. In total, 33 references were identified that provided updated heat production data relevant to this study. From these references, 192 records were identified for pigs ranging in weight from 12.5 to 283 kg and exposed to temperatures between 12.0°C and 35.5°C. Of these 192 records, 71 were barrow-only studies, 38 were gilt-only studies, 35 were mixed (barrow and gilts) studies, 5 were sow-only studies, and 43 were sow plus litter-only studies. A limited amount of latent heat production (LHP) data (66 of the 192 records included LHP) was found that also included total heat production (THP).
3 Growing pigs
Growing pig data included 145 records from 19 references (Fialho et al., 2004; Galassi et al., 2004; de Lange et al., 2006; Huynh et al., 2007; Barea et al., 2010; Labussière et al., 2011; Labussière et al., 2013; Brown-Brand et al., 2014; Campos et al., 2014a; Campos et al., 2014b; Zhang et al., 2014; Kiarie et al., 2015; Liu et al., 2015; Galassi et al., 2015; Batorek-Lukacˇ et al., 2016; Shaffer et al., 2017; Li et al., 2018; Liu et al., 2019; Lyu et al., 2018) with a BW range of 12.5 to 142.5 kg and a temperature range of 12°C to 35.5°C. Data were initially separated by thermoneutral (TN) conditions. Then, temperature effects on HP were analyzed, followed by reporting on LHP data.
3.1 Heat production at thermoneutral conditions
The thermoneutral (TN) zone can be classically described as the range of environmental conditions (i.e., inclusive of variable environmental factors of dry-bulb temperature, airspeed, vapor pressure, and surrounding surface temperatures; Curtis, 1983) in which an animal can maintain a constant body temperature where metabolic heat production is reasonably minimal and constant (Mount, 1974; Yousef, 1985). The TN zone of the animal will vary depending on numerous metabolic and environmental factors (Hillman, 2009). The lower limit of the TN zone is the lower critical temperature, in which pigs use metabolic energy and other physiological and behavioral adaptations to offset heat lost to the environment to maintain core body temperature (Black et al., 1986). The upper limit lacks a clear definition, but is often regarded as high latent heat loss and the onset of reduced metabolic HP (Curtis, 1983; CIGR, 2002; Hillman, 2009; Renaudeau et al., 2011). In response to a warming environment, pigs show increased respiration rates and a decreased voluntary feed intake as well as pigs increase body exposure to increased airspeeds and/or cool and wet surfaces to increase heat loss (Curtis, 1983). Due to this reliance on evaporative heat loss at warm environmental temperatures, heat stress effects on pigs are more definitive at high relative humidity levels (Huynh et al., 2005).
Data were separated by thermoneutral (TN) conditions as defined by the lower critical temperature (LCT; equation 1) and critical temperature (CT; equation 2; Renaudeau et al., 2011) to describe the impact of environmental temperature on HP. These threshold temperatures were previously developed from meta-analyses to model the environmental temperature at which average daily gain deteriorates and feed intake changes in responses to a cooling or warming environment (Renaudeau et al., 2011). The upper range of the TN zone was selected due to the primarily application of this work in environmental control design for housing of growing pigs where high feeding levels are used and the primary goal of the environmental control system design is to limit the negative effects of heat stress (Ames, 1980). Data were further refined to include only ab libitum feeding, resulting in 62 records from 11 references with a temperature range of 14°C to 28°C and weight range of 12.5 to 106 kg.
LCT = Lower Critical Temperature (°C)
CT = Critical Temperature (°C)
BW = Body Weight (kg)
Data were log transformed and a Standard Least Squares regression (equation 3) was completed in (JMP Pro 16.1, SAS Inc, Cary, NC) to predict coefficients (± SE) and predict HP (P=<0.01).
Growing pigs (12.5 to 106 kg; R2 = 0.707, RMSE = 0.62 W kg-1):
HP = Heat Production (W kg-1)
This power law model was compared to Brown-Brandl et al. (2004) and CIGR (1999) in Table 1 and graphically depicted in Figure 1. The CIGR Handbook (CIGR, 1999) documents HP prediction equations based on the biological principles of heat loss rather than solely based on literature data. The current regression (equation 3) showed an average 4.7% increase in HP compared to HP data summarized from 1988 to 2004 (Brown-Brandl et al., 2004) over the studied BW range. Percent increase was greater for lower BW (average of 6.1% for 10 to 40 kg) and lower for higher BW (average of 3.9% for 45 to 105 kg) as shown in Figure 1. The CIGR model using the constants given in Pedersen (2002) seems to over-predict HP in the lighter pigs, as noted by Brown-Brandl et al. (2004); however, this equation for pigs heavier than 50 kg, underpredicted HP by an average of 6.0%. The heaviest BW found in the literature was 106 kg which is below the current U.S.-finishing weight of 127 to 145 kg (MetaFarms, 2021). Limited information on heavier BW finishing pigs may be attributed to the challenges working with these pigs in a research setting and potentially due to marketing agreements that limit the finishing BW.
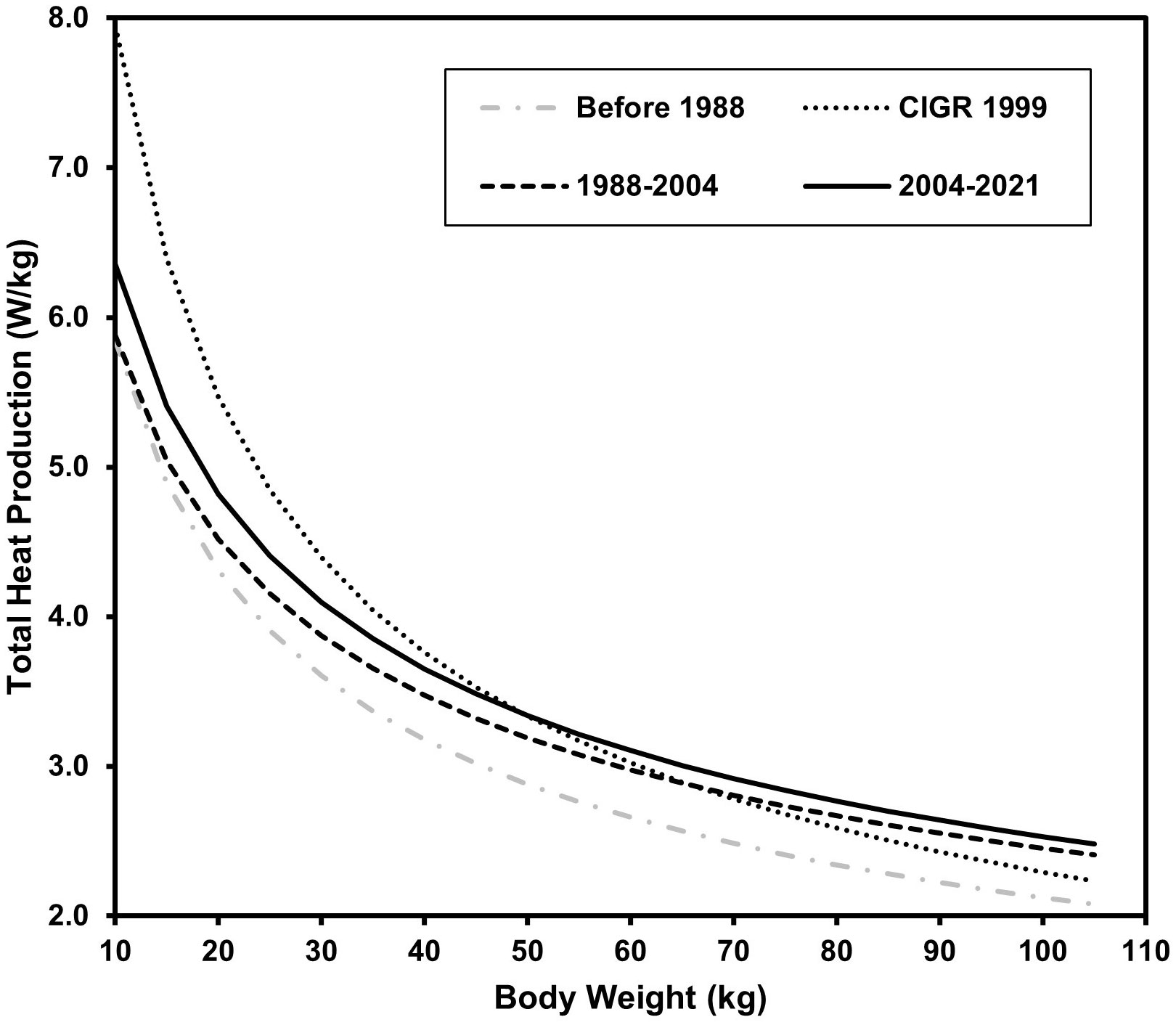
Figure 1 Swine heat production data for a BW range between 12.5 and 106 kg from 19 independent studies from 2004 through 2020. Parameters estimates of the regression equation were significantly different from 0 (P< 0.01).
3.2 Temperature effects on heat production
Homeothermic animals, such as swine, must balance heat loss and metabolic HP to maintain a constant core body temperature (Mount, 1968). The rate of heat loss is governed by the exchange of heat from the pig to its surrounding environment via conduction, convection, radiation, and evaporation (Curtis, 1983). Through a combination of behavioral and physiological responses pigs can modify both heat loss and metabolic HP (Mount, 1968). In responses to a warming environment, HP is decreased through reduced feed intake (Quiniou and Noblet, 1999) and for lactating sows, reduced milk production (Black et al., 1993; Hawe et al., 2020). The extent a warming environment has on decreased HP is dependent on several factors, such as, age, health status, diet, feed intake, prior thermal conditioning, duration of exposure to elevated temperature, housing conditions (individually or groups housed), and floor type (DeShazer et al., 2009). Many of these factors are difficult to ascertain from the literature as well as their impact on the production/experimental settings.
The effect of temperature and BW on HP was analyzed using a Mixed model (JMP Pro 16.1, SAS Inc, Cary, NC) with exposed air temperature (T, °C), body weight (BW, kg), BW2, and BW × T as fixed effects and study as a random effect with a residual covariance structure. Parameters such as, acclimatization or sex were not considered due to the lack of balanced data. The random effect of study was found to be significant (P = 0.036). The fixed effects were all significant except BW × T (P = 0.09) was trending for significance.
For comparison purposes, a Standard Least Squares model (fixed effects of temperature and BW) was used to compare with Brown-Brandl et al. (2004). The resulting equation (R2 = 0.61; RMSE = 0.08 W/kg; P<0.001) is depicted in equation 4 and depicted in Figure 2. Regardless of temperature, the prediction formula showed greater estimates of HP for pigs ≤30 kg BW and lower estimates of HP for pigs ≥75 kg BW compared to Brown-Brandl et al. (2004). The difference in HP was rather insensitive to temperature, that is, if BW was constant and temperature ranged from 16°C to 30°C, difference in HP between Brown-Brandl et al. (2004) and this study was relatively similar; however, a slight decreasing trend in the difference in HP was noted for increasing temperatures.
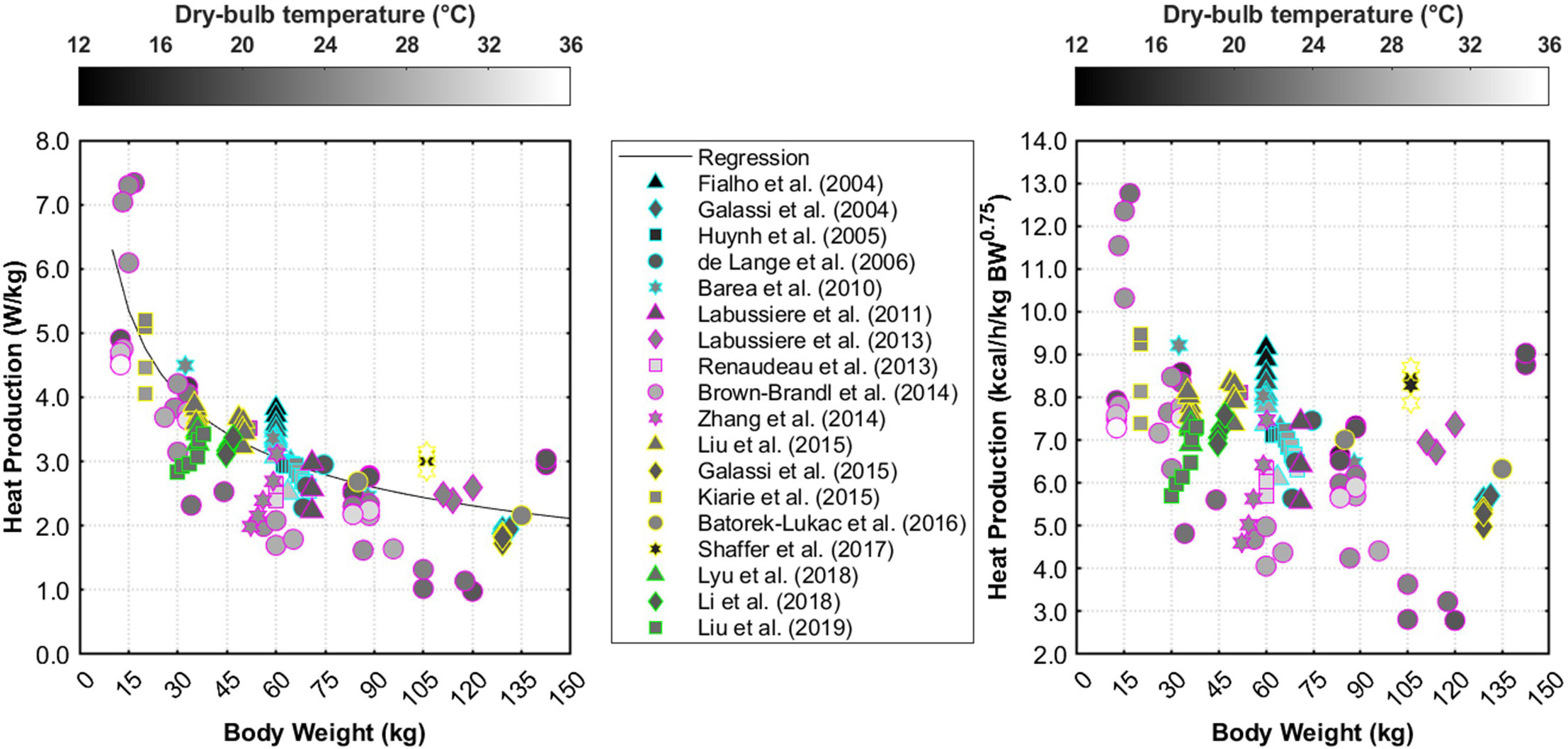
Figure 2 All growing pig total heat production as a function of reported or mean body weight identified by reference (marker shape and outline color) and reported study temperature (marker color).
HP = Heat Production (W/kg)
T = Air Temperature (°C)
BW = Body Weight (kg)
3.3 Latent heat production
Only 2 (i.e., Renaudeau et al., 2013 and Brown-Brandl et al., 2014) studies of 33 references reported LHP. The rate of LHP depends on the thermal environment (i.e., temperature) the pigs are exposed to and the experiment setting (i.e., housing), such that, the LHP of the animal is different than the LHP of the facility (i.e., due to manure handling, drinker type, and other moisture sources; DeShazer et al., 2009). Moisture sources contributing to the latent heat load are important for building design and are poorly documented in livestock and poultry housing (Albright, 1990). Conversely, the LHP of the pigs is important to understanding the onset and response to heat stress conditions (Huynh et al., 2005). Classical thermoregulation shows the ratio between SHP and LHP decreases as the environment warms (Curtis, 1983). As the difference between air temperature (or surrounding temperatures) and pig skin temperature decreases, pigs must lose heat via evaporation resulting in increased LHP (DeShazer et al., 2009). Understanding the thermal conditions that cause the increased rate of change in the SHP to LHP ratio is important for assessing thermoregulatory responses (Curtis, 1983). More data were present in this study, compared to Brown-Brandl et al. (2004); however, creation of correction factors for facility contributions to reported LHP could not be calculated due to lack of information and variety of experimental settings.
Production facility contributions to latent heat load are important for building design (Albright, 1990). Unfortunately, it is difficult to determine a global LHP estimate that works for the multitude of different animal facilities. A statistical analysis of the LHP was not performed because of lack of sufficient data and variable experimental design; however, Table 2 presents the summary statistics.
4 Breeding pigs
Breeding pig data were separated into gestating sows and lactating sows plus litter. For gestating sows, data included 5 records from 2 references (Brown-Brandl et al., 2014; Stinn and Xin, 2014); therefore, gestating sows were excluded from statistical analysis and summary statistics are presented in Table 3. The data represented in Table 3 were collected from sows housed in gestation stalls. However, Brown-Brandl et al. (2014) collected data on pen gestation sows, but they were in small pens and floor fed. As transitions to pen gestation occur (Schulz and Tonsor, 2015), updated heat and moisture production values will be needed because increased activity in pen housing is expected to increase HP (Lucy and Safranski, 2017).
For lactating sows plus litter, data included 43 records from 5 references (Jakobsen et al., 2005; Stinn and Xin, 2014; Brown-Brandl et al., 2014; Pedersen et al., 2019; Cabezón et al., 2017) representing a mass range of 184 to 283 kg and a temperature range of 18°C to 29°C. A visual representation of the data (Figure 3) shows high variability and no apparent trends related to the influence of sow plus litter mass or temperature. This may be attributed to feeding level and regime during HP data collection. If sows were potentially fed ad-libitum, but simply did not consume feed during the measurements, which is expected, or if they were moved to conduct the measurements – this may result in less or greater levels of HP during data collection. As a result, no statistical analysis was performed using these data. Summary statistics for lactating sows plus litter are presented in Table 3. More information is needed on breeding stock pigs, especially as housing systems continue to change.
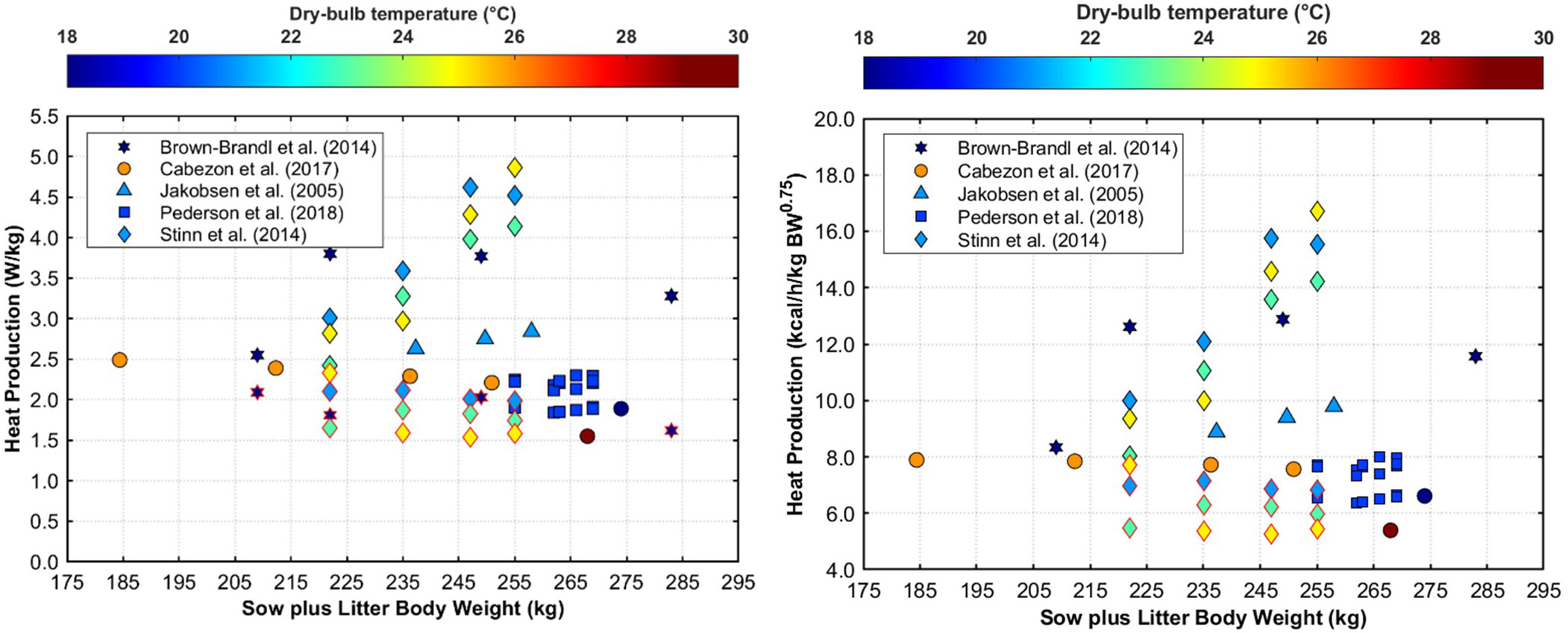
Figure 3 Lactating sow plus litter total heat production (black marker outline) and latent heat production (red marker outline) as a function of sow plus litter mass and identified by reference (marker shape) and reported study temperature (marker color).
5 Future considerations
Measurement of metabolic HP provides information to address a variety of different research goals, such as estimating ventilation rate to remove heat and moisture from facilities (Albright, 1990), understanding the effects of different feed ingredients energy partitioning (DeShazer and Yen, 2009), and impact of heat stress on lactation output (Cabezón et al., 2017). However, there are numerous factors that impact heat production (e.g., feed energy, environment, genetics, housing, measurement; Curtis, 1983), scholarly sources, and disciplines involved in the research (Brown-Brandl et al., 2004; Renaudeau et al., 2011), thereby leading to inconsistent and incomplete reporting to create robust datasets of HP. We encourage the creation of a standardized reporting procedure that could accompany studies that report metabolic heat production. Such a procedure would involve the usage of standard units of measure and completion of a table that could accompany the research as a supplementary table in the appendix or supplemental material section. This table would include key information for reliable and accurate comparison across studies. Examples are provided in Tables 4 and 5.
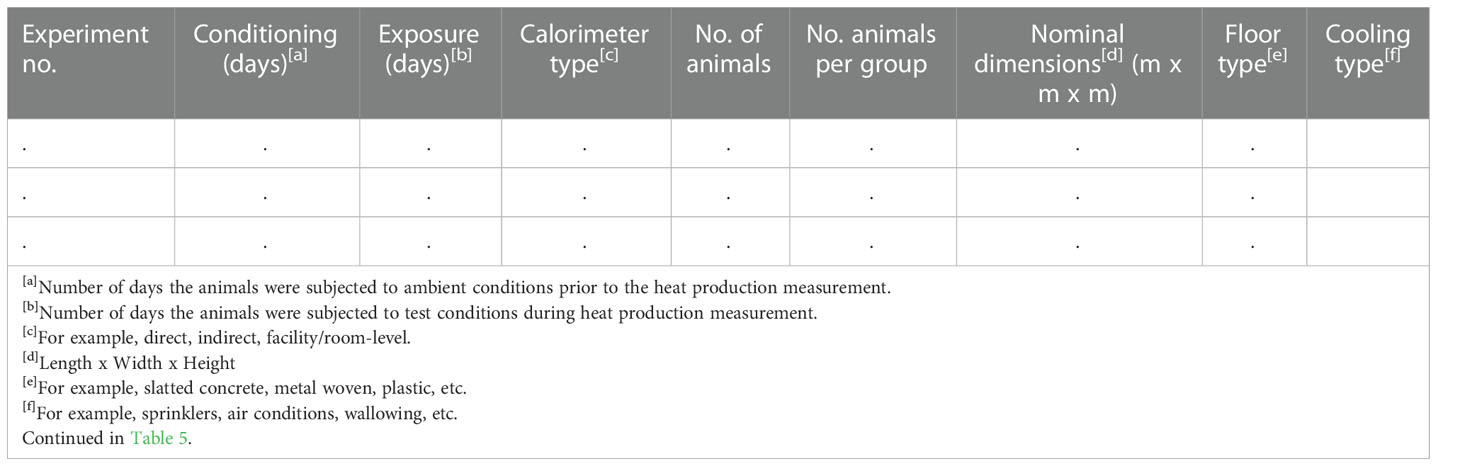
Table 4 Proposed standard reporting of heat production data with respect to housing and environment data.
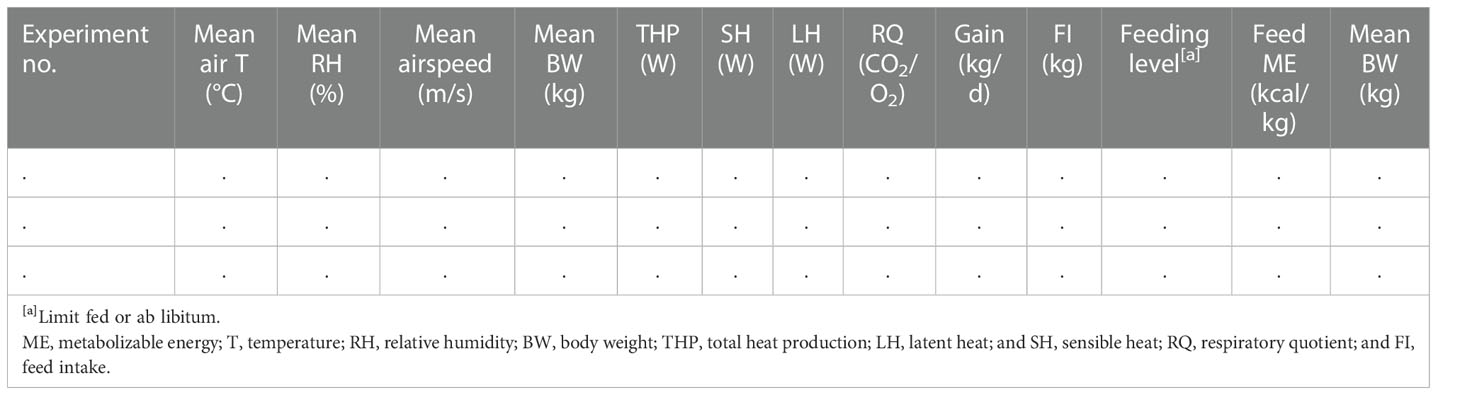
Table 5 Continuation of Table 4 of proposed standard reporting of heat production data.
More information on the range of environmental conditions affecting heat loss and the subsequent impact of the effective environment on HP are needed. Studies need to report the effective environment, that is, air temperature, relative humidity, air velocity, and mean radiant temperature to ensure sufficient detail is present to drive the context of results for greater comparison and to support the development of more robust heat transfers models. An imbalance in metabolic HP and heat lost to the environment via convection, conduction, radiation, or evaporation, directly impacts core body temperature (Mount, 1968). Hence, the basis for mechanistic thermo-physiological models is metabolic HP (DeShazer and Yen, 2009). For example, Huang et al. (2021) used the CIGR (2002) model for growing pigs and for sows, six days of CO2 production collected in 2016, where they found HP to be 14% greater than using CIGR (2002).
While calorimetry methods for individual or small groups of animals can be used to measure HP and LHP data (Brown-Brandl et al., 2004); however, field data collected at the facility or room level are necessary to supplement LHP data and subsequently determine moisture production (Albright, 1990; Brown-Brandl et al., 2004). Facilities that characterize different manure management systems, ventilation system styles, and production environments are needed to create more robust information in SHP and moisture production for use in ventilation design.
Heat stress negatively affects the overall sustainability of swine production by negatively impacting environmental footprint, profitability, and animal welfare. Climate forecasts and improved genetic performance suggest potentially increasingly negative impacts of heat stress (Mayorga et al., 2019). Significant increases in heat stress intensity and duration, as well as warming temperatures will have an impact on current and future heat stress abatement strategies for growing and breeding pigs (Schauberger et al., 2020). Heat stress abatement strategies include improved mechanical ventilation/cooling systems, stocking density, slower growing pigs, more heat tolerant lines/strains (genetic selection/genomic strategies), and nutritional measures (Skuce et al., 2013; Schauberger et al., 2019). Timely and accurate heat production data directly informs the calculation of thermal loads for ventilation/cooling systems and assesses the impacts of different stocking density, growth rate, genetic lines, and nutrition. Therefore, heat production data are required to properly design and evaluate heat stress abatement strategies to lessen negative impacts. Engineering of future controlled swine environments will need improved energetics modeling at thermoneutral and heat stress conditions, heat exchange modeling of building structures with updated energetics data, advanced approaches to environmental control, and development technology that improves sensing, controlling, and modifying the environment (Ramirez, 2022). Established standards and models that describe key analytical methods for design and include foundational data to enable design computations, and criteria/goals for different environments are paramount to the engineering process. Consistent and accurate updates to heat and moisture production of growing and breeding pigs are essential to ensure modern intensive housing is able to provide the proper housing and ventilation for prolific pigs in a changing climate.
Data availability statement
The raw data supporting the conclusions of this article will be made available by the authors, without undue reservation.
Author contributions
BR: Conceptualization, Methodology, Investigation, Writing – Original Draft, Supervision, Project administration. SH: Conceptualization, Methodology, Formal Analysis, Investigation, Writing – Original Draft. MH: Conceptualization, Writing – Review and Editing. TB-B: Conceptualization, Writing – Review and Editing. JH: Conceptualization, Writing – Review and Editing. GR: Conceptualization, Writing – Review and Editing. All: Funding Acquisition. All authors have read and agreed to the published version of the manuscript.
Funding
This research was funded in part by Critical Agricultural Research and Extension (CARE) grant no. 2020-68008-31558/project accession no. 1022743 from the USDA National Institute of Food and Agriculture (NIFA) as well as internal funding from USDA Agricultural Research Service (ARS). BR and JH: This work is also a product of the Iowa Agriculture and Home Economics Experiment Station, Ames, Iowa. Project Number IOW04100 is sponsored by Hatch Act and State of Iowa funds. The open access publication fees for this article were covered by the Iowa State University Library.
Conflict of interest
The authors declare that the research was conducted in the absence of any commercial or financial relationships that could be constructed as a potential conflict of interest.
This study received funding from USDA NIFA. The funder was not involved in the study design, collection, analysis, interpretation of data, the writing of this article, or the decision to submit it for publication.
Publisher’s note
All claims expressed in this article are solely those of the authors and do not necessarily represent those of their affiliated organizations, or those of the publisher, the editors and the reviewers. Any product that may be evaluated in this article, or claim that may be made by its manufacturer, is not guaranteed or endorsed by the publisher.
Author disclaimer
The content of this article was however solely the responsibility of the authors and does not represent the official views of the USDA. USDA is an equal opportunity employer.
References
Ames D. (1980). Thermal environment affects production efficiency of livestock. Bioscience 30 (7), 457–460. doi: 10.2307/1307947
Barea R., Dubois S., Gilbert H., Sellier P., van Milgen J., Noblet J. (2010). Energy utilization in pigs selected for high and low residual feed intake. J. Anim. Sci. 88, 2062–2072. doi: 10.2527/jas.2009-2395
Batorek-Lukacˇ N., Dubois S., Noblet J., Čandek-Potokar M., Labussière E. (2016). Effect of high dietary fat content on heat production and lipid and protein deposition in growing immunocastrated male pigs. Animal 10 (12), 1941–1948. doi: 10.1017/S1751731116000719
Black J. L., Campbell R. G., Williams I. H., James K. J., Davies G. T. (1986). Simulation of energy and amino acid utilization in the pig. Res. Dev. Agric. 3 (3), 121–145.
Black J. L., Mullan B. P., Lorschy M. L., Giles L. R. (1993). Lactation in the sow during heat stress. Livest. Prod. Sci. 35, 153–170. doi: 10.1016/0301-6226(93)90188-N
Bracke M. B. M., Herskin M. S., Marahren M., Gerritzen M. A., Spoolder H. A. M. (2020) Review of climate control and spaceallowance during transport of pigs (European Reference Centre for Animal Welfare: Pigs (EURCAW-Pigs). Available at: https://library.wur.nl/WebQuery/wurpubs/fulltext/515292 (Accessed 15 January2022). version 1.0.
Brown-Brandl T. M., Nienaber J. A., Xin H., Gates R. S. (2004). A literature review of swine heat production. Transactions of the ASAE 47(1), 259.
Brown-Brandl T. M., Hayes M. D., Xin H., Nienaber J. A., Li H., Eigenberg R. A., et al. (2014). Heat and moisture production of modern swine. ASHRAE Trans. 120, 469.
Cabezón F. A., Schinckel A. P., Richert B. T., Peralta W. A., Gandarillas M. (2017). Technical note: Application of models to estimate daily heat production of lactating sows. Prof. Anim. Sci. 33, 357–362. doi: 10.15232/pas.2016-01583
Campos P. H. R. F., Labussière E., Hernández-García J., Dubois S., Renaudeau D., Noblet J. (2014a). Effects of ambient temperature on energy and nitrogen utilization in lipopolysaccharide-challenged growing pigs. J. Anim. Sci. 92(11), 4909–4920. doi: 10.2527/jas2014-8108
Campos P. H. R. F., Noblet J., Jaguelin-Peyraud Y., Gilbert H., Mormède P., de Oliveira Donzel R. F. M., et al. (2014b). Thermoregulatory responses during thermal acclimation in pigs divergently selected for residual feed intake. Int. J. Biometeorol. 58, 1545–1557. doi: 10.1007/s00484-013-0759-3
CIGR (2002). “Heat and moisture production at animal and house levels,” in CIGR section II, 4th report of working group on climatization of animal houses. (Horsens, Denmark) Eds. Pedersen S., Sällvik K. Available at: www.agrsci.dk/jbt/spe/CIGRreport.
Close W. H., Mount L. E. (1978). The effects of plane of nutrition and environmental temperature on the energy metabolism of the growing pig: 1. heat loss and critical temperature. Brit. J. Nutr. 40, 413–421. doi: 10.1079/BJN19780142
Curtis S. E. (1983). Environmental management in animal agriculture (Ames, IA: The Iowa State University Press).
Davis C. G., Dimitri C., Nehring R., Collins L. A., Haley M., Ha K., et al. (2022). U.S. hog production: Rising output and changing trends in productivity growth (U.S. Department of Agriculture, Economic Research Service). ERR-308.
de Lange K., van Milgen J., Noblet J., Dubois S., Birkett S. (2006). Previous feeding level influences plateau heat production following a 24 h fast in growing pigs. Br. J. Nutr. 95, 1082–1087. doi: 10.1079/BJN20061748
DeShazer J. A., Hahn G. L., Xin H. (2009). “Chapter 1: Basic principles of the thermal environment and livestock energetics,” in Livestock energetics and thermal environmental management. Ed. DeShazer J. A. (St. Joseph, Mich: ASABE), 1–22.
DeShazer J. A., Yen J.-T. (2009). “Chapter 3: Energetics of biological processes,” in Livestock energetics and thermal environmental management. Ed. DeShazer J. A. (St. Joseph, Mich: ASABE), 49–71.
Fialho F. B., van Milgen J., Noblet J., Quiniou N. (2004). Modelling the effect of heat stress on food intake, heat production and growth in pigs. Anim. Sci. 79, 135–148. doi: 10.1017/S1357729800054606
Galassi G., Crovetto G. M., Rapetti L., Tamburini A. (2004). Energy and nitrogen balance in heavy pigs fed different fibre sources. 2004. Livestock Prod. Sci. 85, 253–262. doi: 10.1016/S0301-6226(03)00124-6
Galassi G., Malagutti L., Colombini S., Rapetti L., Gallo L., Schiavon S., et al. (2015). Nitrogen and energy partitioning in two genetic groups of pigs fed low-protein diets at 130 kg body weight. Ital. J. Anim. Sci. 14, 3. doi: 10.4081/ijas.2015.4012
Gourdine J. L., Rauw W. M., Gilbert H., Poullet N. (2021). The genetics of thermoregulation in pigs: A review. Front. Veterinary Sci. 8. doi: 10.3389/fvets.2021.770480
Hawe S. J., Scollan N., Gordon A., Magowan E. (2020). Impact of sow lactation feed intake on the growth and suckling behavior of low and average birthweight pigs to 10 weeks of age. Trans. Anim. Sci. 4 (2), 655–665. doi: 10.1093/tas/txaa057
Heath M. E. (1983). The effects of rearing temperature on body-composition in young pigs. Comp. Biochem. Physiol. A. 76 (2), 363–366. doi: 10.1016/0300-9629(83)90338-9
Herpin P., Damon M., Le Dividich J. (2002). Development of thermoregulation and neonatal survival in pigs. Livestock Prod. Sci. 78 (1), 25–45. doi: 10.1016/S0301-6226(02)00183-5
Hillman P. E. (2009). “Chapter 2: Thermoregulatory physiology,” in Livestock energetics and thermal environmental management. Ed. DeShazer J. A. (St. Joseph, Mich: ASABE), 23–48.
Huang T., Rong L., Zhang G., Brandt P., Bjerg B., Pedersen P., et al. (2021). A two-node mechanistic thermophysiological model for pigs reared in hot climates–part 1: Physiological responses and model development. Biosys. Eng. 212, 302–317. doi: 10.1016/j.biosystemseng.2021.08.024
Huynh T. T. T., Aarnink A. J. A., Heetkampb M. J. W., Verstegenb M. W. A., Kemp B. (2007). Evaporative heat loss from group-housed growing pigs at high ambient temperatures. J. Thermal Biol. 32, 293–299. doi: 10.1016/j.jtherbio.2007.03.001
Huynh T. T. T., Aarnink A. J. A., Verstegen M. W. A., Gerrits W. J. J., Heetkamp M. J. W., Kemp B. (2005). Effects of increasing temperatures on physiological changes in pigs at different relative humidities. Can. J. Anim. Sci. 83, 1385–1396. doi: 10.2527/2005.8361385x
Jakobsen K., Theil P. K., Jørgensen H. (2005). Methodological considerations as to quantify nutrient and energy metabolism in lactating sows. J. Anim. Feed Sci. 14, Suppl. 1, 31–47. doi: 10.22358/jafs/70353/2005
Kiarie E., Kim I. H., Nyachoti C. M. (2015). Effect of genotype on heat production and net energy value of a corn-soybean meal-based diet fed to growing pigs. Veterinarni Med. 60 (9), 489–498. doi: 10.17221/8440-VETMED
Labussière E., Dubois S., van Milgen J., Noblet J. (2013). Partitioning of heat production in growing pigs as a tool to improve the determination of efficiency of energy utilization. Front. Physiol. 4. doi: 10.3389/fphys.2013.00146
Labussière E., van Milgen J., de Lange C. F. M., Noblet J. (2011). Maintenance energy requirements of growing pigs and calves are influenced by feeding level. J. Nutr 141 (10). doi: 10.3945/jn.111.141291
Li Z., Liu H., Li Y., Lv Z., Liu L., Lai C., et al. (2018). Methodologies on estimating the energy requirements for maintenance and determining the net energy contents of feed ingredients in swine: A review of recent work. J. Anim. Sci. Biotechnol. 9, 39. doi: 10.1186/s40104-018-0254-0
Liu H., Chen Y., Li Z., Li Y., Lai C., Piao X., et al. (2019). Metabolizable energy requirement for maintenance estimated by regression analysis of body weight gain or metabolizable energy intake in growing pigs. Asian-Australas J. Anim. Sci. 32 (9), 1397–1406. doi: 10.5713/ajas.17.0898
Liu D. W., Liu L., Li D. F., Wang F. L. (2015). Determination and prediction of the net energy content of seven feed ingredients fed to growing pigs based on chemical composition. Anim. Prod. Sci. 55, 1152–1163. doi: 10.1071/AN14091
Lucy M. C., Safranski T. J. (2017). Heat stress in pregnant sows: thermal responses and subsequent performance of sows and their offspring. Mol. Reprod. Dev. 84 (9), 946–956. doi: 10.1002/mrd.22844
Lu Y., Hayes M., Stinn J. P., Brown-Brandl T., Xin H. (2017). Evaluating ventilation rates based on new heat and moisture production data for swine production. Trans. ASABE 60 (1), 237–245. doi: 10.13031/trans.11888
Lyu Z. Q., Huang C. F., Li Y. K., Li P. L., Liu H., Chen Y. F., et al. (2018). Adaptation duration for net energy determination of high fiber diets in growing pigs. Anim. Feed Sci. Technol. 241, 15–26. doi: 10.1016/j.anifeedsci.2018.04.008
Mayorga E. J., Renaudeau D., Ramirez B. C., Ross J. W., Baumgard L. H. (2019). Heat stress adaptations in pigs. Anim. Front. 9 (1), 54–61. doi: 10.1093/af/vfy035
MetaFarms (2021)Production analysis summary for U.S. pork industry: 2017-2020. In: National pork board research grant report (Des Moines, IA: National Pork Board). Available at: https://porkcheckoff.org/wp-content/uploads/2021/12/MetaFarms-2021-Production-Analysis-for-NPB-1-1.pdf (Accessed January 15, 2022).
Mount L. E. (1974). “The concept of thermal neutrality,” in Heat loss from animals and man: Assessment and control (London, UK: Butterworths), 425–439.
Page M. J., McKenzie J. E., Bossuyt P. M., Boutron I., Hoffmann T. C., Mulrow C. D., et al. (2021). The PRISMA 2020 statement: An updated guideline for reporting systematic reviews. Systematic Rev. 10 (1), 1–11. doi: 10.1186/s13643-021-01626-4
Pedersen S. (2002). Heat and moisture production for pigs on animal and house level. ASAE Paper No. 024178. St. Joseph, Mich.: ASAE.
Pedersen T. F., Chang C. Y., Trottier N. J. L., Bruun T. S., Theil P. K. (2019). Effect of dietary protein intake on energy utilization and feed efficiency of lactating sows. J. Anim. Sci. 97 (2), 779–793. doi: 10.1093/jas/sky462
PIC (2018) Sow herd productivity. Available at: https://pichermitage.com/sow-herd-productivity/ (Accessed January 21, 2022).
Quiniou N., Noblet J. (1999). Influence of high ambient temperaturees on performance of multiparous lactating sows. J. Anim. Sci. 77, 2124–2134. doi: 10.2527/1999.7782124x
Ramirez B. C. (2022). Engineering strategies and solutions to improve swine resilience and alleviate physiological stress in a changing climate 100, 90. doi: 10.1093/jas/skac064.147. Abstract presented at Midwest Section American Society of Animal Science Meeting, Omaha, NE.
Ramirez B. C., Hayes M. D., Condotta I. C., Leonard S. M. (2022). Impact of housing environment and management on pre-/post-weaning piglet productivity. J. Anim. Sci. 100 (6), skac142. doi: 10.1093/jas/skac142
Renaudeau D., Frances G., Dubois S., Gilbert H., Noblet J. (2013). Effect of thermal heat stress on energy utilization in two lines of pigs divergently selected for residual feed intake. J. Anim. Sci. 91, 1162–1175. doi: 10.2527/jas2012-5689
Renaudeau D., Gourdine J. L., St-Pierre N. R. (2011). A meta-analysis of the effects of high ambient temperature on growth performance of growing-finishing pigs. J. Anim. Sci. 89 (7), 2220–2230. doi: 10.2527/jas.2010-3329
Schauberger G., Hennig-Pauka I., Zollitsch W., Hörtenhuber S. J., Baumgartner J., Niebuhr K., et al. (2020). Efficacy of adaptation measures to alleviate heat stress in confined livestock buildings in temperate climate zones. Biosyst. Eng. 200, 157–175. doi: 10.1016/j.biosystemseng.2020.09.010
Schauberger G., Mikovits C., Zollitsch W., Hörtenhuber S. J., Baumgartner J., Niebuhr K., et al. (2019). Global warming impact on confined livestock in buildings: efficacy of adaptation measures to reduce heat stress for growing-fattening pigs. Climatic Change 156 (4), 567–587. doi: 10.1007/s10584-019-02525-3
Shaffer C. S., Riskowski G. L., Harrison P. C. (2017). Effect of conductive cooling pads on heat and moisture production of gilts in hot and thermoneutral environments. Agric. Eng. Int.: CIGR J. 19 (4), 13–19.
Skuce P. J., Morgan E. R., Van Dijk J., Mitchell M. (2013). Animal health aspects of adaptation to climate change: Beating the heat and parasites in a warming Europe. Animal 7 (s2), 333–345. doi: 10.1017/S175173111300075X
Stalder K. J. (2018). 2017 pork industry productivity analysis. In: National pork board research grant report. (Des Moines, IA: National Pork Board). Available at: https://jygatech.com/wp-content/uploads/2021/05/PigmortalityStalderIowa.pdf
Stinn J. P., Xin H. (2014). Heat and moisture production rates of a modern U.S. swine breeding, gestation, and farrowing facility. Trans. ASABE 57 (5), 1517–1528. doi: 10.13031/trans.57.10711
Yousef M. K. (1985). Stress physiology in livestock. volume i. basic principles (Boca Raton, Florida: CRC press).
Keywords: calorimetry, genetics, growth, moisture production, nutrition, temperature
Citation: Ramirez BC, Hoff SJ, Hayes MD, Brown-Brandl T, Harmon JD and Rohrer GA (2022) A review of swine heat production: 2003 to 2020. Front. Anim. Sci. 3:908434. doi: 10.3389/fanim.2022.908434
Received: 30 March 2022; Accepted: 05 December 2022;
Published: 21 December 2022.
Edited by:
Pasquale De Palo, University of Bari Aldo Moro, ItalyReviewed by:
Takashi Bungo, Okayama University of Science, JapanFrançois-Xavier Philippe, Université libre de Bruxelles, Belgium
Copyright © 2022 Ramirez, Hoff, Hayes, Brown-Brandl, Harmon and Rohrer. This is an open-access article distributed under the terms of the Creative Commons Attribution License (CC BY). The use, distribution or reproduction in other forums is permitted, provided the original author(s) and the copyright owner(s) are credited and that the original publication in this journal is cited, in accordance with accepted academic practice. No use, distribution or reproduction is permitted which does not comply with these terms.
*Correspondence: Brett C. Ramirez, bramirez@iastate.edu