- 1Drexel University, Philadelphia, PA, USA
- 2Rutgers, The State University of New Jersey, New Brunswick, NJ, USA
- 3The University of Texas at El Paso, El Paso, TX, USA
The primary objective of this discussion is to argue that the need for innovation in civil engineering practice is becoming more critical, especially as the goals and expectations associated with infrastructure performance expand to include livability, sustainability, and resilience (LSR). Current approaches to assess and characterize constructed infrastructures rely primarily on visual inspection procedures, which largely serve to guard against blatant safety problems. These efforts generally include a thorough documentation of visual appearance guided by heuristics. Unfortunately, the relationship between key limit states (e.g., structural safety, serviceability, functionality, etc.) and visual condition is nebulous, and thus decision-makers are often forced to rely on conservatism at the expense of accuracy. Furthermore, documenting visual distresses do not always lend to understanding the root causes. As a result, visual assessment procedures make organizations struggle to design proper and effective maintenance and make prudent asset management decisions. In general, completely visual infrastructure evaluation may lead to incomplete diagnoses followed by ineffective prognoses, often leading to highly consequential disruptions impacting LSR. To illustrate this argument further, the paper draws upon the authors’ experiences evaluating bridges over the last several decades. More specifically, this paper aims to present an overview of the current highway bridge engineering and management practices in the U.S. and to postulate how technologies may be incorporated to achieve LSR goals. Wherever possible, the authors have tried to present this discussion in sufficiently general terms as many aspects of it may equally apply to other infrastructure nodes and sectors as well.
Introduction
Leveraging technology (defined in this context as sensing, imaging, data, information, communication, computing, scenario simulation, and decision) to support the adaption, reuse, engineering, and management of the urban built environment is difficult. The livability, sustainability, and resilience (LSR) of dense urban regions is emerging as perhaps the single greatest challenge to the civil engineering profession. By 2030, 60% of the world’s population will be living in cities and especially mega-cities (Bouton et al., 2016) thereby increasing the importance of these large metropolitan regions as political, economic, and social hubs (Khanna, 2016). Not surprisingly, improving urban infrastructures was selected as one of 14 grand challenges for engineering by the National Academy of Engineering (NAE) in the U.S. since 2008. The NAE indicated that infrastructure challenges are “particularly acute in urban areas, where growing populations stress society’s support systems, and natural disasters, accidents, and terrorist attacks threaten infrastructure safety and security.”
Livability, Sustainability, and Resilience
The shift from defining systems as “constructed” or “natural” to more realistic definitions that recognize their social components is critical, and it is a natural byproduct of adopting human-centric (i.e., LSR) goals for infrastructure. LSR are in fact highly related concepts that all depend on proper functioning and performance of infrastructures and infrastructure services. As a result, we posit that planning, engineering, and managing a city for LSR is the fundamental or parent systems problem facing our urban regions and should therefore impact the curricula of all forward-thinking government agency, foundations, education, and research institutions.
Currently, we expect elected officials, policy-makers, and planners to lead the efforts for providing livability, sustainability, and resilience. Although their responsibility in this regard is not challenged, the writers believe such activities cannot be decoupled from the engineers, technicians, infrastructure managers, and program planners who make day-to-day management decisions and are responsible for assessment, design, construction, and operation of the built environment. The implementation of master plans based on forecasts of society’s future growth, economic demands, and expectations cannot ignore the reality of existing infrastructures with all the shortcomings, vulnerabilities, financial liabilities, resources, and opportunities they present. Meeting this challenge will require engineers to embrace technologies that have the ability to quantify and reduce uncertainties and provide a more detailed, accurate, and objective understanding of the reality of our complex built environment.
Our current approaches to assessing and characterizing infrastructures rely primarily on visual inspection procedures (developed before the availability of “modern” sensing, simulation, and information technologies), which have served to guard against blatant safety problems. These efforts, which were legislated following the 1967 collapse of the Silver Bridge over the Ohio River in the US, generally include a thorough documentation of visual appearance (or “condition”) guided by heuristics. Unfortunately, the relationship between key limit states (e.g., structural safety, serviceability, functionality, etc.) and visual condition is nebulous, and thus decision-makers are often forced to rely on conservatism at the expense of accuracy. Today, when these procedures are called upon to play a role in meeting LSR goals for our urban regions, they are found wanting. In addition to their alarmist qualities, these procedures lack the resolution, accuracy, and precision to identify the root cause of performance problems and vulnerabilities, which are prerequisites to creative adaption and reuse options. Meanwhile, even after more than 40 years of biennial highway bridge inspections, there remain many other constructed systems such as buried water and gas lines and storm-water drainage facilities that are not regularly inspected or maintained until they fail, causing significant disruption.
To illustrate this argument further and in greater detail, the writers would like to take advantage of their experiences with bridges as the nodes where highway, rail, transit, and river transportation networks commonly intersect. This paper aims to present the current highway bridge engineering and management practices in the U.S. and to postulate how technologies may be incorporated to allow the more fundamental issues associated with LSR goals to be attained. The primary objective of this discussion is to argue the importance of innovation in civil engineering and the challenges that need to be overcome for proper integrative technology applications.
The U.S. Bridge Ecosystem
The majority of highway bridges in the U.S. were constructed in the mid-20th century by the first few generations of engineers educated through the university-based applied science model. While today’s Professional Engineer Licensure requires 5 years of experience under another professional engineer, this is a substantially diluted version of the former apprenticeship model, which included design and construction over a decade or more. With the proliferation of educational programs, many of which focused more on processes than products, civil engineering has started to become a commodity. Today, various domains of bridge design, construction, inspection, load rating, operations, routine maintenance, and asset management are delegated to many different components within the management organization essentially creating disconnected silos. In addition to the inefficiencies that often accompany fragmentation, such an organizational construct also makes it difficult to preserve institutional knowledge (the primary outcome of the apprenticeship model).
Although there have been numerous focusing events (which generally take the form of tragic collapses) that have altered bridge management practice since 1967, the explosion of technology that has revolutionized numerous domains, has yet to make any substantial impact. Today, visual assessment remains the most critical component of bridge management, and technologies remain relegated to the fringes. As infrastructure policy aims to meet LSR goals and the resulting debates solidify their place within the nation’s political discourse, the authors believe that the untapped potential of technology will emerge as a critical tool for reimagining bridge engineering practice. As a result, the authors believe an open discussion of the value, means, and challenges of integrating various technologies within bridge assessment practice is quite timely, and this may also serve other infrastructure domains.
Overview of U.S. Bridge Assessment Practice
Current bridge design limit states include strength, service, fatigue and fracture, and extreme events. A recent study on bridge performance (Chase et al., 2016) offers lifecycle limit states of: utility and functionality; serviceability and durability; life-safety and reparability (under 50- to 500-year return period extreme events); and resilience (under 1,000-year return period extremely rare events). Meanwhile, the current bridge assessment practice in the U.S. requires the collection/calculation and reporting of four general pieces of information. The first is condition ratings that are based on visual inspection procedures. Although these metrics are the most influential in terms of bridge lifecycle management, their subjectivity and variability are well-documented. This issue was first quantified through a study led by the FHWA NDE Validation Center that compared results from 49 inspectors (selected from 25 states) who inspected the same seven bridges (Phares et al., 2004). The results of this study demonstrated the inherent variability of visual inspection, which reflects the disparate supplemental educational and experience requirements for inspectors across different states.
In addition to condition ratings, during regular inspections, more than 100 inventory items are collected either from past inspection reports, design/as-built plans, or field survey. These items describe both the attributes of a bridge as well some demands. For example, this information includes bridge type and material, overall dimensions, number of spans, average daily traffic, and average daily truck traffic, among others. Inventory items of particular importance are related to the scour and fracture criticality of the bridge, which can influence the type and frequency of required visual inspections.
The third item required is the calculation and reporting of a “load rating” for each bridge that reflects its capacity to carry live loads. A recent NCHRP study investigated current bridge load rating and posting practices within the U.S. (Hearn, 2014) and found that over 80% of bridges are rated using simplified structural analysis procedures, which represents 95% of the ratings reported. Load ratings are noteworthy as they remain the only assessment activity to attempt to quantitatively assess a safety-related (strength) limit state based on the principles of physics.
The final activity associated with assessment is the calculation and reporting of indices and designations that combine information from the other three information sources to aide in decision-making and resource allocation. These historically have taken the form of structurally deficient and functionally obsolete designations as well as the computation of the sufficiency rating. With the increased popularity (and now a requirement for most bridges) of element-level inspections, the development of indices that combine element-level condition ratings is now becoming more relevant. Examples include the California Health Index and the AASHTO Health Index.
Shortcomings of Current U.S. Bridge Assessment
Visual Inspection Practice
In addition to the subjectivity and variability of visual inspection mentioned above, there are two other shortcomings that are rarely discussed but are significant, especially as owners adopt LSR goals. First, while visual inspection can identify visible deterioration, this does not necessarily translate into the identification of its root cause. Without understanding root causes, the design of effective interventions is difficult as attention may focus on symptoms whose treatment has little ability to curb the underlying performance issue. Similarly, we are destined to repeat design inefficiencies that may potentially be the root cause for performance problems if we do not identify that relationship. The authors offer the following structure of the coupled influences that may drive the type and extent of bridge deterioration:
1. Structural characteristics (geometry such as skew, horizontal, and vertical curvature; geology and hydrology of the site; soil, foundations, and substructures; approach slabs and spans; structural materials; dynamic characteristics, stiffness, redundancy, detailing; bearings and joints).
2. Construction quality (material and fabrication quality, tolerances (e.g., cover), intrinsic forces such as due to cambering, transport, lifting, and fit-up during erection, placing, vibration, and curing of concrete, protection of the concrete by membranes or coatings, weather during construction).
3. Environmental inputs (climate and freeze-thaw, weather and temperature fluctuations, air quality and precipitation, site conditions, fill erosion, flow conditions, settlements, etc.).
4. Live load environment (truck types and weights, frequencies, axle configurations, speed and dynamics).
5. Maintenance activities (clearing drainage systems, selection and application of de-icing agents, joint/bearing servicing, bridge washing, deck sealing, etc.).
While many of these factors are fairly well understood, what is less clear is the role that the global structural characteristics of a bridge play in observed deterioration. Fundamentally, this stems from the view that bridge systems are disconnected, individual elements (e.g., NBIS, element-level inspection). Such an approach is efficient, but it neglects system-level behaviors that result more from element interactions than individual element responses. For example, the deterioration of bridge decks is commonly viewed as a “material-level” problem—exclusively resulting from freeze-thaw, de-icing chemicals, rebar corrosion, etc., but this ignores the fact that bridge decks are an integral component of a structural system through which they are also subjected to vibrations, live load actions, thermal variations and gradients, etc. How the structural performance under operational conditions, such as vibrations may exacerbate material-level problems and distresses have not been properly investigated to this date.
In addition to their inability to trace performance deficiencies to root causes, the relevance of visual inspections is largely limited to serviceability/durability limit states and struggles to reliably assess structural safety and resilience limits states. Just as in the case of new design, the evaluation of performance limit states associated with safety (or strength) and resilience (i.e., the ability to deflect and bounce back from extreme events) should become a required component of bridge assessment and inventory recording practice. In contrast to the service-level limit states, performance at the safety and resilience limit states cannot be directly observed. Rather, such an evaluation requires experience and simulation-based estimation of likely future performance given a specific bridge system (inclusive of site, roadway, approaches, superstructure, substructures, foundations, soil), condition states, and applicable hazards.
Given the complexity of issues surrounding safety and resiliency limit states, it is unrealistic to expect visual appearance alone to be used as the basis of such an assessment. The authors believe a systematic approach that includes a more complete understanding of the entire bridge system’s behavior and performance and how these are affected by various hazards is desirable. While this may require additional resources, given that the likely performance at safety and resiliency limit states evolve slowly with time, their evaluation intervals may be much longer than for operation and service limit states.
Common Load Rating Practice
Current load rating practice within the U.S. relies heavily on simplified modeling approaches that may offer conservative estimates of load-carrying capacity but are unable to estimate actual structural characteristics or behavior. As a result, they fall short of being able to facilitate meeting LSR goals in three important ways. First, in cases where a bridge fails to rate, such simplified models cannot be relied upon to accurately identify the governing “critical” member or location (especially important in cases where girder transitions are present). Second, the simplified methods cannot accurately simulate the effects of deterioration on both capacity and demand (since many elements are not explicitly modeled), and thus they are forced to rely on the use of subjective reduction factors. Third, such approaches cannot be relied upon to offer accurate estimates of stresses, stiffness (transverse or longitudinal), or dynamic properties, which hampers their ability to be used to diagnose deterioration caused or exacerbated by structural responses.
In many cases, this approach is also employed to post structures (i.e., to limit the weight of traffic permitted), which may unnecessarily hamper commerce or the mobility of emergency vehicles and school buses. The AASHTO Manual for Bridge Evaluation (MBE) second edition (American Association of State Highway and Transportation Officials, 2008/2010) recognizes this conservatism and allows bridges “that exhibit insufficient load capacity when analyzed with approximate methods” to be “analyzed by refined methods of analysis.” Furthermore, the AAHSTO MBE (2008/2011) allows bridges to be rated by “field testing (load testing)” if the evaluator feels that analytical approaches do not accurately represent the true behavior and load distribution. Although such methods are available to practicing engineers, they are rarely employed. For example, Hearn (2014) reports that less than 0.1% of bridges are rated based on load testing procedures, even though over 10% of bridges in the U.S. are posted for less than legal loads.
Current Load Testing Practice
The AASHTO MBE (2008/2011) with 2013 interim revisions (2013) recognizes two types of non-destructive load testing: diagnostic- and proof-level. These tests are distinguished generally by the level of load, with diagnostic-level loads near service loads and proof-level loads closer to design loads. While proof-level tests are conducted using statically positioned trucks or other special devices to generate the load levels required, diagnostic-level tests can be carried out using static or crawling trucks, operating traffic, or vibration testing techniques.
In its current form, owners do not consider load testing an attractive value proposition. Consider that over 61,000 bridges are currently posted for less than legal loads, but fewer than 600 bridges nationwide have been rated using load testing procedures (Hearn, 2014). This no doubt stems from the cost associated with such tests (which may range from 5 to 10 times the cost of a visual inspection). While technologies may mitigate the cost issue, there is an issue with value as well. Load testing is focused almost exclusively on load rating. Substantial value may be seen by expanding the focus of load testing to characterize and quantify various structural properties, which may be used to both diagnose the root causes of various forms of deterioration and forecast their future propagation.
There are three more specific shortcomings of the current load testing guidance.
1. There is a lack of specific guidance for what constitutes an acceptable load test. This was done by design at a time when technology was not as integrated into most aspects of society’s day-to-day lives. While the skills to execute load tests are not trivial, they are not as rare as they were two decades ago when the current load testing procedures were first codified.
2. Proof testing does not explicitly make use of simulation, which is inherently less safe than utilizing a model to at least verify assumptions on system behavior during testing. This is analogous to Roman engineers standing under the bridge they constructed as it was first loaded to ensure the quality of the work.
3. Diagnostic testing is used to update load ratings based on correcting a non-representative model with data from the actual structure. The discrepancy between model and reality is a fundamental challenge in modeling and also what forces an engineer to better understand the mechanisms and variables governing reality. The issue is there is a disconnect between the physical mechanisms which govern load distribution and the procedures that govern load ratings. Physical mechanisms that perhaps should not be included in rating, like frozen rocker bearings may directly influence measured responses, and should not be used to update ratings.
Technology Policy and Research
As the practice of bridge assessment evolved, research efforts aimed at developing and identifying the role various technologies may serve also ramped up. Although it is difficult to pin-point its origins, the confluence of new federal policies, the rising awareness of aging infrastructures as a key societal challenge, and a clear articulation of needed infrastructure research that occurred in the early 1990s all played a role. The focusing event came in September of 1993 with the tragic derailment of Amtrak’s cross-country Sunset Limited on a bridge over the Big Bayou Canot near Mobile, AL, USA (US NTSB, 1994). This derailment resulted in the death of 47 people (103 injured), and it remains the deadliest train accident in Amtrak history. The cause was traced to a track misalignment resulting from a barge impact 8 min prior to the train crossing the bridge. Fueled by the burgeoning revolution in information technology, the prospect of a monitoring system that may have offered early warning caught the attention of many researchers, practitioners, and government officials, and the FHWA Advanced Research Office (now the Exploratory Advanced Research Program) began to explore the feasibility of bridge monitoring to mitigate similar events.
Around the same time the U.S. National Science Foundation convened a Civil Infrastructure Task Group composed of multi-disciplinary experts with the goal of defining a research agenda for the renewal of the U.S. infrastructure. The resulting publication (NSF, 1993) argues that infrastructures must necessarily be viewed from a systems perspective as opposed to the reductionist, component-level perspective. More specifically, the group recommended a series of strategic research directions, including (a) deterioration science (toward the development of a mechanistic understanding of deterioration and its causes), (b) assessment technologies [inclusive of non-destructive evaluation (NDE), long-term monitoring, and evaluation of remaining service life], and (c) renewal engineering (identification of effective preservation, repair, retrofit, or renewal strategies). In the last two decades, much progress has been made related to individual elements of this agenda, and additional elements such as “organizational effectiveness” and “valuation of infrastructures” were added. While the systems perspective espoused by this task group remains unrealized in practice, it is now almost universally recognized as a key barrier to effective infrastructure management (especially when owners aim to meet LSR goals).
Following these events in the early 1990s, a dramatic increase in research activities related to technology-based infrastructure assessment occurred. A detailed review of the literature is beyond the scope of this paper, but a brief chronology of a few key conferences and professional societies that were developed to disseminate and guide this research may be useful. Key examples include the biennial “Structural Materials Technology: An NDT Conference” held under the auspices of the FHWA since 1994 as well as the international NDT conferences starting from 1995 at Berlin (Schickert, 1995). These recurring conferences, as well as the annual Transportation Research Board meetings since late 1980s served to introduce new technology tools, as well as associated concepts and terms to bridge and transportation engineers within the U.S. The NDE Center was established by the Federal Highway Administration (FHWA) in 1998 in an effort to centralize and better coordinate research related to non-destructive testing. In addition, the International Structural Health Monitoring (IWSHM) Workshop was launched in 1997 and has taken place every 2 years since. Similarly, an International Society for Health Monitoring Intelligent Infrastructures was established in 2003.
During the 1990s, the American Society of Civil Engineers (ASCE) Structural Engineering Institute (SEI) formed a Technical Committee named “Structural Identification (St-Id) of Constructed Systems,” which brought together leading multi-disciplinary experts to focus on integrative approaches to characterizing actual constructed systems. The ASCE published the Committee Report: “Approaches, Methods, and Technologies for Effective Practice of St-Id” as a book (ASCE 2013). The writers have leveraged the St-Id concept for bridge condition assessment and established a robust approach to properly utilize sensing, simulation, information, and decision technologies for this purpose discussed in the following. The St-Id methodology (Figure 1) indicates that a priori modeling, experiment, model calibration, and utilization are the fundamental steps of St-Id requiring a coordinated, integrative multi-disciplinary application together. The practices and multi-disciplinary integration needed for successful implementations of St-Id have been discussed further by Aktan and Brownjohn (2013) and Smith (2016).
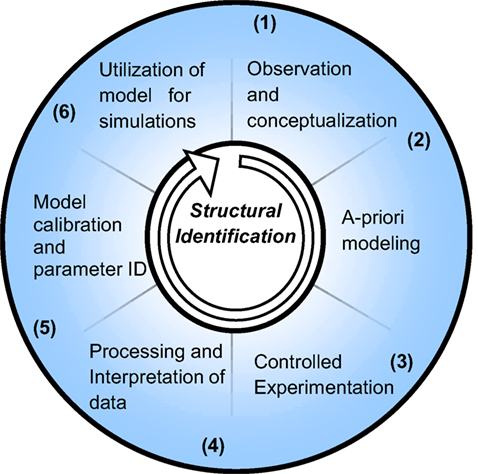
Figure 1. Structural identification (Catbas et al., 2013).
Integration of Technology
Given all of the investment into technology development since the early 1990s, it is interesting to consider why bridge assessments are carried out today in much the same manner as they have been since the 1970s. Perhaps the most obvious reason is that without the adoption of more ambitious goals (like the LSR goals that are now gaining attention worldwide) it was difficult to justify the increased investments. Additional barriers were also present. For example, until recently there has been no concerted effort to implement and integrate the various technologies within a framework that is compatible with current practice. Although it is changing, researchers remain prone to pursue well-defined, highly technical development efforts in their areas of expertise. Such efforts are valuable, and they have led to the impressive sensing, simulation, and data processing/visualization tools now available. However, they are of limited use to the profession in this rather “raw,” unintegrated form. For their part, practitioners have been engaged in the incremental adoption of such technologies, but this generally occurs in a piecemeal, ad hoc manner and without an effective market-pull strategy.
Truly benefiting from the use of technologies requires more than simply adding them into current practice in a few isolated areas based on specific problems. What is required is a top-down and bottom-up systems approach that revisits both the criteria and constraints associated with bridge and infrastructure assessment and risk-based performance management in light of current and developing technologies, the characteristics of the work force, available funding, and our current understanding of bridge performance. The writers submit that the current incremental and opportunistic (market-push) approach to technology adoption has not proven very effective, and thus a top-down restructuring of bridge assessment practice, which is currently missing, is proposed. As a first step toward this larger effort, the authors have developed a potential approach to structuring various assessment technologies within the context of bridge assessment.
The utilization of the proposed framework (for bridge applications) requires an integration of at least four distinct technology domains in addition to domain knowledge (both heuristic and mechanistic) in bridge engineering. This would be similar for innovating other domains outside of bridge engineering. From the authors’ perspective, the key technology domains are as follows:
(a) Experimental arts—civil/structural engineering heuristics, observation and conceptualization of structural performance, societal expectations of performance, design of experiments (both laboratory and field), field logistics, sensing and imaging technologies, etc.
(b) Information technology—electrical and computer engineering, and computer science expertise in communication, signal processing, coding, data and image acquisition, processing, visualization, analysis, search and archival management.
(c) Simulation and scenario analysis—civil engineering heuristics, analytical arts, modeling and simulation of complex systems with multi-physics phenomena.
(d) Decision arts—civil engineering heuristics, uncertainty and risk analysis, lifecycle benefit/cost analysis, asset management and multi-hazards mitigation, and emergency response decisions.
Technology Leveraging Framework
The following technology framework is an organization of potential actions, complemented by or inclusive of, technology applications. The framework requires a business case and organizational commitment for success and adopts six steps, which loosely classify applications that would benefit from technology:
1. Collect and digitally document infrastructure provenance and past condition/performance history.
2. Determine current conditions of infrastructure—geometry, materials, flexibility, levels of intrinsic forces, and selected load-response relationships.
3. Collect objective and quantitative data on current infrastructure performance and organize the data, information, and other relevant materials in an electronic searchable database.
4. Use a system identification approach to correlate quantitative performance data with simulation models to develop/enhance predictive capabilities for scenario analyses.
5. Use the enhanced predictive capabilities to implement a risk-based approach for decision-making.
6. Integrate the prior efforts into a sustainable and sensible infrastructure preservation strategy leveraging asset management principles.
Some steps, like condition assessment are a regular part of the conversation surrounding technology for bridge management, while others, like development of predictive capabilities, are less common. Note that the examples discussed below in the framework are not intended to be comprehensive or apply to all bridges but are offered for illustrative purposes. The authors believe that implementation of this framework would necessarily be different for every structure, and, more generally, for every infrastructure domain.
Technology Integration Framework—Bridge Example
Recognizing that the current bridge population of over 600,000 in the U.S. is quite difficult to classify, we may never-the-less consider this population comprised (a) about 1,000–1,500 unique long-span signature crossings that are suspension, cable-stay, arch, truss, or segmental post-tensioned construction; (b) over 350,000 RC deck-on-girder (either steel or prestressed concrete) bridges; and (c) about 100,000 short-span cast-in-place RC bridges (T-Beam, slab, or jack arch); among others such as adjacent prestressed concrete box-girder bridges, steel trusses, and combinations as well as variants of these.
While the operation and preservation of major signature bridges such as the Golden Gate Bridge and Verrazano Bridge are the responsibility of their dedicated organizations, the vast majority of bridges are owned by states or local governments. Bridges that make up the largest number of the population such as RC deck on steel girders or RC deck on PC girders often carry their loads with common, repeating mechanisms that may be parameterized. Given such population characteristics, we may develop a number of bridge-specific or bridge population-specific technology frameworks. Furthermore, although the following framework is specific to highway bridges, it would also serve for other infrastructure domains:
Step 1:placing the bridge in context: this is primarily focused on collecting and archiving all existing documentation in a format that is not only accessible but also interactive and offering 3D visualization of the bridge as a system. In addition, this step requires bringing together stakeholders and eliciting expert opinions and institutional knowledge about the performance of similar kinds of bridges.
Step 2:capturing bridge geometry and forces: this step consists of non-contact geometry capture by one of high resolution imaging, laser-based survey, installation of a GPS-based system for monitoring absolute displacements, or LiDAR scanning. Additionally, where possible, in situ forces may be determined (e.g., hanger tension) to better understand current structural demands.
Step 3:condition and performance assessment: traditional condition assessment by visual approach and material sampling is augmented with wearable computer technology such as Google Glass; digital image processing that may also be performed by leveraging unmanned aerial systems; local or wide-area non-destructive scans; and short-term operational monitoring of critical structural responses as appropriate.
Step 4:development of predictive capabilities (structural system identification): utilization of targeted St-Id to address specific performance questions, resulting in calibrated FE models that can be used to interpret experimental data and address remaining uncertainties. Systems identification as a concept for field testing has been advocated since the 1960s in earthquake engineering. Many successful applications to constructed systems in conjunction with FE modeling have been published by an ASCE Committee of experts (2013) and many others.
Step 5:risk assessment and mitigation: identification of critical hazards, vulnerabilities, and exposures with expected failure modes. These can then be used (with caution) to conduct scenario analyses and identify potential interventions and corrective actions.
Step 6:performance and health monitoring for asset management: implement either discrete (monthly, bi-annual, annual) or continuous monitoring on a scale appropriate to the bridge(s) in question to directly support management of the asset(s).
Conclusion and Recommendations
The emerging LSR goals for dense urban regions are perhaps the single greatest challenge to the civil engineering profession. These goals demand a significant departure of the often employed “worst, first” prioritization approaches for infrastructure investments that focus narrowly on condition and visual appearance. The authors believe that in order to realize these LSR goals, it is necessary to develop effective and efficient approaches to leverage technology (sensing, imaging, data, information, communication, computing, scenario simulation, and decision) to support creative adaption, reuse, engineering, and management practices. As the internet of things and cyber-physical system technologies advance and information technology and 3D visualization are being adapted by many construction companies, the civil engineering and especially bridge engineering practice have to embrace technology innovation.
To provide a tangible illustration of these broad arguments, the paper delved into the U.S. practice of bridge assessment practices as an archetypal infrastructure challenge. The authors posit that we need to rethink our definitions for, and improve our assessment of, bridge performance. This cannot happen by considering each bridge as different, and judging its performance based on the appearance of its elements while ignoring the fact that the structure is but one component of a complex natural, engineered and social system of systems. This concept is now at the foundation of FHWA’s Long Term Bridge Performance Program, which is expected to define and collect data on the performance of hundreds of bridges for decades.
We need to emphasize the importance of a meaningful policy for selecting and integrating technology tools for field research and applications, especially if the goal in field applications is generic knowledge and not just data. This requires technology applications to be coordinated by engineers with domain knowledge. Unfortunately, far too often, technology applications are characterized by a lack of proper coordination, supervision, and integration by bridge engineers. Thus, it is common for objectives, such as “data collection” to be satisfied while the root causes of fundamental performance problems remain obscure, and owners are left with little, if any, actionable recommendations.
A technology application hierarchy that would help overcome this shortcoming is suggested in this paper, and this may serve as a draft for further discussion by the bridge, engineering, and urban science communities. Technology policy and strategy for leveraging technology to innovate practice cannot work unless we first understand the reality of how a built environment actually performs and interacts with the natural and socio-technical systems. We also have to identify and circumvent the mechanisms of uncertainty and human inexperience as well as errors that may impact any attempt to perform experiments and measurements in the field reliably and to reach meaningful conclusions by properly assuring the data quality, data visualization, and interpretation.
Author Contributions
AA, FM, and JW collaborated equally on the paper.
Conflict of Interest Statement
The authors declare that the research was conducted in the absence of any commercial or financial relationships that could be construed as a potential conflict of interest.
References
Aktan, A., and Brownjohn, J. (2013). Structural identification: opportunities and challenges. Special Issue: real-world applications for structural identification and health monitoring methodologies. J. Struct. Eng. 139, 1639–1647. doi: 10.1061/(ASCE)ST.1943-541X.0000723
American Association of State Highway and Transportation Officials. (2008/2010). The Manual for Bridge Evaluation, First edition. Edn. Washington, DC: American Association of State Highway and Transportation Officials.
Bouton, S., Cis, D., Mendonca, L., Pohl, H., Remes, J., Ritchie, H., et al. (2016). How to make a city great. McKinsey & Company. Available at: http://www.mckinsey.com/global-themes/urbanization/how-to-make-a-city-great
Catbas, F. N., Kijewski-Correa, T. L., Aktan, A. E., Structural Engineering Institute, and Committee on Structural Identification of Constructed Systems. (2013). Structural Identification of Constructed Systems: Approaches, Methods, and Technologies for Effective Practice of St-Id. Reston, VA: American Society of Civil Engineers.
Chase, S. B., Adu-Gyamfi, Y., Aktan, A. E., and Minaie, E. (2016). Synthesis of National and International Methodologies Used for Bridge Health Indices. Washington, DC. Report No: FHWA-HRT-15-081.
Hearn, G. (2014). State Bridge Load Posting Processes and Practices. Washington, DC: Transportation Research Board of the National Academies.
Khanna, P. (2016). ‘Connectography,’ Random House. NDE. Available at: https://fhwaapps.fhwa.dot.gov/ndep/
NSF. (1993). Civil Infrastructure Systems Research – Strategic Issues: A Report. Washington, DC: National Science Foundation.
Phares, B., Washer, G., Rolander, D., Graybeal, B., and Moore, M. (2004). Routine highway bridge inspection condition documentation accuracy and reliability. J. Bridge Eng. 9, 403–413. doi:10.1061/(ASCE)1084-0702(2004)9:4(403)
Schickert, G. (1995). NDT-CE Symposium Berlin 1995: A Concluding Review, International Symposium on Nondestructive Testing in Civil Engineering. Available at: http://www.ndt.net/article/civil497/gschic/gschic_1.htm
Smith, I. F. C. (2016). Studies of sensor data interpretation for asset management of the built environment. Front. Built. Environ. 2:8. doi:10.3389/fbuil.2016.00008
Keywords: structural sensing, infrastructure management, asset management, structural identification, non-destructive testing
Citation: Aktan AE, Moon FL and Weidner JS (2016) Leveraging Technology for Infrastructure Condition and Performance Assessment. Front. Built Environ. 2:36. doi: 10.3389/fbuil.2016.00036
Received: 19 September 2016; Accepted: 14 December 2016;
Published: 27 December 2016
Edited by:
James Mark William Brownjohn, University of Exeter, UKReviewed by:
Eliz-Mari Lourens, Delft University of Technology, NetherlandsErin Santini Bell, University of New Hampshire, USA
Copyright: © 2016 Aktan, Moon and Weidner. This is an open-access article distributed under the terms of the Creative Commons Attribution License (CC BY). The use, distribution or reproduction in other forums is permitted, provided the original author(s) or licensor are credited and that the original publication in this journal is cited, in accordance with accepted academic practice. No use, distribution or reproduction is permitted which does not comply with these terms.
*Correspondence: Ahmet Emin Aktan, YWFrdGFuQGRyZXhlbC5lZHU=