Fungi: A Neglected Candidate for the Application of Self-Healing Concrete
- 1Department of Mechanical Engineering, Binghamton University, Binghamton, NY, United States
- 2Materials Science and Engineering Program, Binghamton University, Binghamton, NY, United States
- 3State Key Laboratory of Silicate Materials for Architectures, Wuhan University of Technology, Wuhan, China
- 4Zhongshan Institute of Advanced Engineering Technology, Wuhan University of Technology, Zhongshan, China
Self-healing mechanism in concrete has been so far achieved mainly by three approaches: autogenous healing, encapsulation of polymeric material, and biologically induced mineralization of calcium carbonate. The microbial approach prevails over the other two approaches due to the high compatibility between the filler material and the concrete compositions. Although the term “microbe” refers to many different types of organisms, research work on self-healing concrete has been so far restricted to bacteria. In this perspective article, we review the current status of bacteria-mediated self-healing concrete and summarize the prospects for future advances. In particular, we point out that fungi may have distinctive advantages over other microbes to be used as self-healing agents.
Introduction
Sustainability—the ability of being durable, reliable, and economically affordable during the entire lifetime, and resiliency—the capacity of withstanding and rapidly recovering from all hazards, are the two key characteristics of the infrastructural system. However, it's no secret that the infrastructure system in many countries suffers from deterioration and in desperate need of rehabilitation. For example, the United States has more than 600 K bridges: 40% are 50 years or older; 9% are not structurally robust; and on average there are 188 M trips across a structurally deficient bridge every day (American Society of Civil Engineers, 2018).
In particular, concrete structures, as a primary element of our infrastructure system, have serious deterioration issues (Association of State Dam Safety Officials, 2018). Cracking in concrete is very common owing to many different chemical and physical phenomena that occur on daily basis. Durability of concrete is significantly weakened by the presence of cracks, as they provide channels for the harmful ingress of liquids and gasses potentially containing corrosive agents for the steel reinforcement.
Maintenance and repair techniques for concrete infrastructure have thus attracted considerable amount of attention from the research community. However, the implementation of continuous maintenance often requires enormous amounts of labor and investment. Moreover, diagnosis and repair work may become quite challenging to be executed in a timely or cost-effective manner due to the damage conditions, e.g., the location of the damage in the affected concrete structure. Under such conditions, transformative ideas are urgently needed to tackle the serious challenge—long-term and preventive, rather than short-term and corrective.
During the past decade, significant amount of research has been conducted to understand how these harmful cracks could heal themselves without human intervention or interference. Self-healing mechanism in concrete has been so far achieved mainly by three approaches: autogenous healing (Edvardsen, 1999), encapsulation of polymeric material (Dry, 1994), and biologically induced mineralization of calcium carbonate. Autogenous healing is the natural process of concrete crack repair when moisture is present (Edvardsen, 1999). It can only heal narrow cracks whose widths are < 0.3 mm. Polymeric material fills cracks in concrete by converting healing agents to foam when water or humidity is present. Although the chemicals released from hollow fibers embedded in concrete matrix can heal the cracks, these materials are not usually compatible with concrete compositions, e.g., filler and crack have different mechanical properties and thermal expansion coefficients, which could cause the enlargement and propagation of the existing cracks (Dry, 1994).
Due to the above-mentioned weakness, the use of the biological approach using calcium mineral-producing microbes becomes extremely desirable. It has been demonstrated that when a calcium source is present, calcium carbonate, which possesses high compatibility with concrete compositions, can be precipitated by bacteria via various biologically induced mineralization processes (Fortin et al., 1997; Stocks-Fischer et al., 1999; Bang et al., 2001, 2010; Ramachandran et al., 2001; Dick et al., 2006; Ramakrishnan, 2007; De Muynck et al., 2008, 2010; Jonkers and Schlangen, 2009; Jonkers et al., 2010; Van Tittelboom et al., 2010, 2011; Jonkers, 2011; Wiktor and Jonkers, 2011; Wang J. et al., 2012; Wang J. Y et al., 2012; Wang et al., 2014a,b; Ersan et al., 2015a,b; Khaliq and Ehsan, 2016; Seifan et al., 2016; Zhang et al., 2017).
Mechanisms and Limitations of Bacteria-Mediated Self-Healing Concrete
Natural organisms are known to be able to generate crystalline materials at ambient temperature by biomineralization (Tebo et al., 2005; Seifan et al., 2016). Most biomineralization processes are achieved via biologically induced mineralization, during which the organism alters its local micro-environment to create proper physicochemical conditions for mineral precipitation (Rivadeneyra et al., 1994; Seifan et al., 2016). Calcium carbonate is a prevalent biomineralization product and calcite precipitation is considered as one of the most common microbially-mediated phenomena in the biosphere (Kumari et al., 2016).
Bacteria-mediated self-healing concrete based on biologically induced mineralization processes has been extensively studied during the past decade (Fortin et al., 1997; Stocks-Fischer et al., 1999; Bang et al., 2001, 2010; Ramachandran et al., 2001; Dick et al., 2006; Ramakrishnan, 2007; De Muynck et al., 2008, 2010; Jonkers and Schlangen, 2009; Jonkers et al., 2010; Van Tittelboom et al., 2010, 2011; Jonkers, 2011; Wiktor and Jonkers, 2011; Wang J. et al., 2012; Wang J. Y et al., 2012; Wang et al., 2014a,b; Ersan et al., 2015a,b; Khaliq and Ehsan, 2016; Seifan et al., 2016; Zhang et al., 2017). It has been shown that bacteria can precipitate calcium carbonate through three different pathways. (1) Some ureolytic bacteria have been explored based on their ability to indirectly cause the formation of calcium precipitates by increasing the local pH via catalyzing the hydrolysis of urea to ammonium (Stocks-Fischer et al., 1999; Bang et al., 2001; Ramachandran et al., 2001; Dick et al., 2006; Ramakrishnan, 2007). (2) Metabolic conversion of organic compound to calcium carbonate was also extensively investigated (Jonkers and Schlangen, 2009; Jonkers et al., 2010; Jonkers, 2011). During this process, aerobic oxidation of organic acids produces carbon dioxide, which produces carbonates in an alkaline environment. (3) The third pathway is referred to as dissimilatory nitrate reduction (Ersan et al., 2015a). Bacterial denitrification is defined as a respiratory process to reduce nitrate stepwise to nitrogen gas. Minerals are precipitated via oxidation of organic compounds by the reduction of nitrate via denitrifying bacteria (Van Paassen et al., 2010). For the three pathways, an external calcium source usually needs to be provided, as shown in Table 1 in Seifan et al. (2016), and the optimal concentration of the provided calcium ions depends on the type of calcium source, the type of bacterial culture, and the method of application, etc.
To make bacteria-mediated self-healing concrete, the bacterial spores, as well as their nutrients, are added to concrete matrix during mixing process (EPOfilms, 2018). When cracks occur, and water finds its way in, the dormant bacterial spores will germinate, grow, consume the nutrients, and precipitate calcium carbonate to heal the cracks in situ. When the cracks are completely healed with no more water inside, the bacteria will become dormant again. As the environmental conditions become favorable in later stages, the spores could be wakened again. For existing concrete structures with cracks, the bacterial spores and their nutrients are injected or sprayed into the cracks (EPOfilms, 2018).
Although the term “microbe” refers to many different types of microorganisms, studies on self-healing concrete have been so far limited to bacteria. The research on bacteria-mediated self-healing concrete indeed achieved a significant level of success (Fortin et al., 1997; Stocks-Fischer et al., 1999; Bang et al., 2001, 2010; Ramachandran et al., 2001; Dick et al., 2006; Ramakrishnan, 2007; De Muynck et al., 2008, 2010; Jonkers and Schlangen, 2009; Jonkers et al., 2010; Van Tittelboom et al., 2010, 2011; Jonkers, 2011; Wiktor and Jonkers, 2011; Wang J. et al., 2012; Wang J. Y et al., 2012; Wang et al., 2014a,b; Ersan et al., 2015a,b; Khaliq and Ehsan, 2016; Seifan et al., 2016; Zhang et al., 2017; EPOfilms, 2018). However, it still has some limitations as listed below.
Limitation #1: There Has Been Little Success With Respect to Long-Term Self-Healing Efficacy
The bacterial spores can usually remain viable in dry state for more than 50 years (Jonkers, 2011). However, bacteria and their spores could not generally survive the harmful environment of concrete. When the bacterial spores are directly put in concrete matrix, their lifetime is limited to <2 months (Jonkers, 2011). For example, B. cohnii spores were embedded directly in concrete matrix, and the number of viable bacteria cells decreased after 22 and 42 days of curing by 80 and 90%, respectively (Jonkers et al., 2010). This is because the typical bacterial spores are larger than the pores in concrete, and if they are directly added to concrete matrix, most spores are squeezed and crushed during the hydration process when the pores in concrete significantly shrink. Therefore, encapsulation or immobilization of bacterial spores in a protective carrier becomes necessary. However, the protection techniques only increased their lifetime to about 6 months (Jonkers, 2011), which is still far from being practical considering the fact that the lifetime of concrete infrastructure is often more than 50 years or even a century.
Concrete is considered as an extreme environment for bacteria mainly due to its high pH values. The pH-values of concrete matrix are between 11 and 13 owing to the formation of calcium hydroxide, one of the most important hydration products. Even for alkaliphilic bacteria, concrete is still a harsh environment due to severe moisture deficit, varied temperatures, limited nutrient availability, and possible exposure to ultraviolet light, which often dramatically influence the bacterial metabolic activities and make bacteria and their spores vulnerable to death.
Limitation #2: There Has Been Little Success With Respect to Repair of Wide Cracks or Rapid Crack Repair
Although there is no limit for the length of the cracks, the crack width is crucial (EPOfilms, 2018)—bacteria can only heal narrow cracks with crack widths <1.0 mm (Wiktor and Jonkers, 2011; EPOfilms, 2018). The healing process usually takes 21–100 days. Wang J. Y et al. (2012) reported that utilization of immobilized bacteria was able to completely heal cracks of a width of 0.17 mm after 40 days of healing. Wiktor and Jonkers (2011) reported that utilization of encapsulated bacterial spores was able to close cracks with widths ~0.46 mm after 100 days of healing. Wang et al. (2014b) demonstrated that encapsulation in hydrogels allowed bacteria to fill cracks with widths varied from 0.2 to 0.5 mm after 28 days of healing. Zhang et al. (2017) reported that utilization of immobilized bacteria was able to heal cracks with widths ~0.79 mm after 28 days of healing. According to Wang et al. (2014a), encapsulated bacterial spores were able to induce calcium carbonate precipitates that were able to close cracks up to 1.0 mm after 21 days of healing, which is by far the largest crack width and the highest healing rate we could find in existing literature.
This limitation is due to the insufficient ability of the bacteria to promote large amounts of calcium carbonate precipitates within short periods of time. The factors affecting bacterial calcium carbonate production include the concentration of calcium ions, the pH and temperature of the concrete matrix, the growth medium composition, and most importantly, the availability of nucleation sites—usually the bacterial cell walls (Hammes and Verstraete, 2002; Seifan et al., 2016).
Limitation #3: Incorporation of Healing Agents, i.e., Bacterial Spores and Nutrients, Results in Unwanted Loss of Concrete Compressive Strength
In many previous studies, healing agents were put into tubular or spherical capsules to improve the viability of bacteria, since capsules could resist mechanical forces during the mixing process. When cracks occur, the capsules are ruptured and the self-healing process is triggered by release of the healing agent. However, capsules-incorporated concrete often results in much lower mechanical strength due to the empty space that appears after capsules rupture (Wang et al., 2014a; Seifan et al., 2016). According to Wang et al. (2014b), the application of encapsulated B. sphaericus spores in mortar reduces its compressive strength by 15–34%.
Immobilization of bacteria into porous carriers was often used to address the encapsulation drawbacks (Jonkers, 2011; Ersan et al., 2015b). Although no empty space was left by using this approach, replacement of a fraction of sand and gravel with porous carriers could also lead to a dramatic decrease of concrete compressive strength (Jonkers, 2011; Ersan et al., 2015b). Jonkers (2011) reported that utilization of immobilized B. pseudofirmus and B. cohnii spores in concrete decreased compressive strength after 28 days of curing by 50% compared with concrete specimens without replacement of sand and gravel fractions. In the study conducted by Ersan et al. (2015b), the porous carriers were included in addition to the standard aggregate content, but the utilization of immobilized B. sphaericus spores in concrete still reduced compressive strength after 7 and 28 days of curing by 63 and 60%, respectively, mainly owing to the non-encapsulated nutrients.
Besides the three limitations listed above, we want to point out that it is still very difficult to make self-healing concrete economically competitive with currently existing repair techniques. According to current industrial data (Silva et al., 2015), average concrete prices are ~110 dollars/m3. The bacteria-based self-healing concrete with encapsulated or immobilized spores currently results in prices of about 6,876 dollars/m3, making this self-healing technique unlikely to be used in practical applications (Silva et al., 2015). This high price is mainly caused by the need of aseptic conditions to obtain bacterial spores, the use of expensive growth media, and most importantly, the encapsulation or immobilization process to protect bacterial spores (Silva et al., 2015).
Prospects for Fungi-Mediated Self-Healing Concrete
To address the limitations of bacteria-mediated self-healing concrete, the authors have conducted extensive investigation on alternative microorganisms for biogenic crack repair in the past few years. The results demonstrated that fungi could be one of the best candidates to be used as self-healing agent due to the following reasons.
Perspective #1: Fungi-Mediate Self-Healing Concrete May Possess Long-Term Self-Healing Capacity
Long-term self-healing capacity is crucial for sustainable and resilient infrastructure. It is hypothesized that fungi and their spores can better adapt to the deleterious environment of concrete, which includes low humidity, varied temperatures, possible exposure to ultraviolet light, and severe nutrient limitation, and most importantly, high alkalinity.
Fungi are known to possess remarkable ability to utilize different strategies to survive and prosper in harsh environments (Ehrlich, 1981; Staley et al., 1982; Johnstone and Vestal, 1993; Urzi et al., 1995; Panina et al., 1997; Raghukumar and Raghukumar, 1998; Sterflinger, 2000; Kis-Papo et al., 2001; Robinson, 2001; Burford et al., 2003a,b; Sancho et al., 2007; Sterflinger et al., 2012; Chavez et al., 2015). Certain species of fungi have evolved the ability of adaptation to a range of so-called extreme environments, where few other microorganisms could survive (Magan, 2007). Most relevantly, rocks are often considered as an extreme environment for fungi because of limited nutrient availability, exposure to ultraviolet light, and moisture deficit. However, due to their extraordinary ability to grow oligotrophically by scavenging nutrients from air and rainwater, fungi have been reported in a wide range of substrates including limestone, sandstone, granite, and marble, where they constitute an important component of both epilithic and endolithic microbial communities (Ehrlich, 1981; Staley et al., 1982; Johnstone and Vestal, 1993; Urzi et al., 1995; Panina et al., 1997; Sterflinger, 2000; Burford et al., 2003a,b).
According to Magan (2007), many different species of fungi can grow in alkaline environments with pH values above 10. For example, Paecillomyces lilacimus and Chrysosporium spp. are both alkaliphilic and able to grow very well at pH-values of 7.5–11.0. The possession of an osmotic barrier to the external environment can help the fungi maintain their internal cellular pH at close to neutrality for efficient cellular functioning, even when the external pH is extreme. It is this ability that has enabled fungi to become well established in various extremely alkaline environments, such as soda lakes, desert soils, and alkaline springs (Longworthy, 1978).
Besides wild-type strains, genetically engineered fungi are also important candidates for self-healing concrete. Fungi respond to ambient pH levels via a dedicated transcription factor PacC (Lamb et al., 2001; Caracuel et al., 2003a,b; Nobile et al., 2008; Penalva et al., 2008; Hervas-Aguilar et al., 2010). Mutants in the pacC regulatory gene are extremely heterogeneous in phenotype, and a major class of mutations are gain-of-function pacCc created by truncating the C-terminal region (Penalva and Arst, 2002, 2004). The pacCc mutations bypassing the need for the ambient pH signal lead to permanent activation of alkaline genes and superrepression of acidic genes, resulting in alkalinity mimicry (Penalva et al., 2008). Irrespective of ambient pH, alkalinity-mimicking mutants believe that they are always at alkaline pH and trigger a pattern of gene expression similar to that in the wild type grown under alkaline conditions, which is exactly what is needed for the application of self-healing concrete.
To date, the investigation on fungi-mediated self-healing concrete is lacking. The authors believe that a wide screening of different species of fungi for the application of biogenic crack repair is urgently needed. The candidates should be selected from the alkaliphilic spore-forming fungi. Most fungi can form spores that can survive almost any natural environment (Moore-Landecker, 2002; Hamilton, 2006). They should pose little risk to human health and are appropriate to be used in infrastructure. It is preferred if the genomes of the fungi are publicly available so that they can be genetically manipulated to enhance their survivability.
Perspective #2: Fungi-Mediated Self-Healing Concrete May Heal Wider Cracks Within Shorter Periods of Time
If the cracks, both narrow and wide, can be healed in a timely manner, the maintenance costs will be greatly reduced, the service life of the infrastructure will be significantly extended, and the catastrophic consequences caused by vulnerable structures will be avoided or mitigated. It is hypothesized that fungi are more effective than bacteria in biogenic crack repair owing to their exceptional ability to promote calcium carbonate precipitates within short periods of time.
Fungi generally display either of two growth modes, yeast-like or filamentous. The cells of filamentous fungi grow as tubular, elongated, and threadlike structures called hyphae, which contain multiple nuclei and grow apically with new apices emerging from the formation of lateral branches, creating a 3D network called mycelium. It is widely believed that filamentous fungi possess distinctive advantages over other microbial groups to be used in a variety of applications of biomineralization-based technologies due to their superior cell wall-binding capacity and extraordinary metal-uptake capability (Kahle, 1977; Klappa, 1979; Wright, 1986; Gadd, 1990, 1993, 1999, 2007; Verrecchia et al., 1990, 1993, 2006; Arnott, 1995; Volesky and Holan, 1995; Monger and Adams, 1996; Manoli et al., 1997; Sayer et al., 1997; Gharieb et al., 1998; Bruand and Duval, 1999; Verrecchia, 2000; Chander et al., 2001a,b; Dias et al., 2002; Khan and Scullion, 2002; Roncero, 2002; Burford et al., 2006; Burbank et al., 2011; Takey et al., 2013; Li et al., 2014; Phanjom and Ahmed, 2015; Bindschedler et al., 2016).
One of the critical factors that influence the amount of produced mineral precipitates is the availability of the nucleation sites—usually the microbial cell walls (Hammes and Verstraete, 2002). It is obvious that the microbes that exhibit higher surface-to-volume ratios possess a larger fraction of potential organic templates for mineral precipitation (Bindschedler et al., 2016). Compared with the much smaller and simpler bacterial cell, the fungal branching architecture and filamentous growth habit provide more nucleation sites and framework support for the calcium carbonate precipitates, as shown in Figure 1.
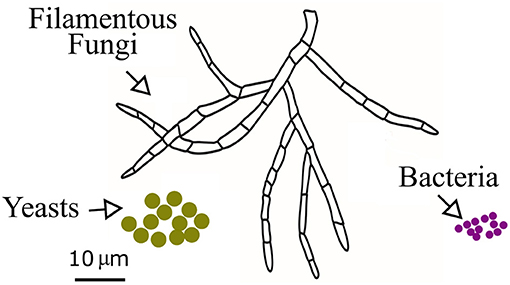
Figure 1. The main morphological and size differences between filamentous fungi, yeast, and bacteria, showing that the fungal branching architecture could provide more nucleation sites and framework support for the calcium carbonate precipitates.
While bacteria promote mineral precipitation only through induced biomineralization processes, fungi are capable of both induced biomineralization and organomineralization processes (Bindschedler et al., 2016).
To precipitate calcium carbonate, two factors are critical, i.e., carbonate alkalinity and calcium ion concentration. Fungal metabolic activities can influence both, leading to induced calcium carbonate biomineralization. Fungal activities that can increase alkalinity typically include water consumption, physicochemical degassing of fungal respired carbon dioxide, organic acid oxidation, nitrate assimilation, and urea degradation (Bindschedler et al., 2016). In addition to their influence on carbonate alkalinity, fungi can also influence calcium ion concentration. Calcium ion concentration within metabolically active fungal cells is under strict control: calcium ions must be concentrated at the apex for proper apical growth and instantly decreased in subapical regions (Bindschedler et al., 2016). To keep this steep gradient, fungi need to efficiently regulate calcium ions by actively pumping it out of the cell or by binding it onto cytoplasmic proteins (Bindschedler et al., 2016).
Besides induced biomineralization, fungi also participate through organomineralization, which involves an organic substrate to initiate or enhance crystal nucleation and growth. In this type of biomineralization, living organisms are not required and only the organic fraction matters as a template for nucleation (Bindschedler et al., 2016). An important characteristic that places fungi in a different kingdom from bacteria is the chitin in their cell walls, which is a substrate that significantly reduces the required activation energy for nucleus formation so that the interfacial energy between the fungi and the mineral crystal becomes much lower than the interfacial energy between the mineral crystal and the solution (Manoli et al., 1997; Roncero, 2002). Therefore, cation binding by fungi can occur by means of metabolism-independent binding of ions onto cell walls, which is an important passive property of both living and dead biomass. Bound calcium ions can interact with soluble carbonate ions, leading to calcium carbonate deposition on the fungal hyphae. Calcite formed in the aqueous phase could also nucleate onto the hyphae.
Fungi could also promote calcium carbonate precipitation out of self-protection. Metals that are essential for fungal growth and metabolism can all exert toxicity when present above certain threshold concentrations. However, many fungi can survive and prosper in apparently metal-polluted locations. Many scientific articles reported a population shift from bacteria to fungi in metal-contaminated soils and waters (Chander et al., 2001a,b; Khan and Scullion, 2002; Gadd, 2007), and precipitation of metal minerals onto hyphae was considered as one of the most important mechanisms to explain the superior toxic metal tolerance in fungi (Gadd, 2007; Bindschedler et al., 2016). Concrete is a calcium-rich environment, which likely represents a stress for fungal cells owing to calcium cytotoxicity and subsequent osmotic pressure. The precipitation of calcium oxalates has been suggested as a strategy to immobilize excessive calcium ions (Gadd, 1999). The formation of calcium carbonate precipitates may represent a similar means to decrease their internal calcium concentration.
Fungi can promote large amount of calcium carbonate precipitates within short periods of time (Rautaray et al., 2003, 2004; Ahmad et al., 2004). Ahmad et al. (2004) demonstrated that calcite crystals were obtained by simple exposure of aqueous calcium ions to the two fungi Trichothecium sp. and Fusarium oxysporum, respectively, at 27°C. Immature calcite crystals were observed after 12 h of reaction. The morphology evolves from structures with plenty of defects to smooth structures after 3 days of reaction. While many plate-shaped crystals were observed in the case of Trichothecium sp., the crystallites assembled into ring-like superstructures in the case of F. oxysporum. In another study, Burford et al. (2006) grew Serpula himantioides in laboratory microcosms consisting of Carboniferous limestone at 25°C. Extensive crystal production of calcium carbonate was observed after 21 days of incubation. Crystal production was observed with both living and dead fungal biomass, indicating that both induced biomineralization and organomineralization processes were involved.
The previous studies on self-healing concrete mainly center upon the macroscopic properties of the system. Due to the microscopic nature of fungal filaments, it is essential to understand the mineral precipitation processes at the microscopic level and directly in the native conditions. With the goal of optimizing the biomineralization process to significantly increase calcium carbonate precipitation within short periods of time, a deep understanding of the fundamental mechanisms by which fungi facilitates crystal nucleation and subsequent growth is urgently needed for achieving a high level of control over the development of the resulting minerals.
Perspective #3: Incorporation of Healing Agents, i.e., Fungal Spores and Nutrients, Could Lead to No Negative Consequences on Concrete Compressive Strength
Compressive strength is a crucial parameter used in the design and evaluation of concrete structures. Building codes and standards for the infrastructure system impose strict requirements for the compressive strength of its concrete components. Self-healing concrete with decreased compressive strength is useful only in a limited range of applications.
Fungal spores are usually larger than bacterial spores (Luo et al., 2018), which could have been a weakness of using fungi, because too many fungal spore-containing capsules or porous carriers would significantly lower compressive strength. However, it is hypothesized that in an optimized system, effective self-healing can be achieved without compromising concrete compressive strength.
Seifan et al. (2016) concluded that the healing agents increased mortar compressive strength when the cell concentration is about 5 × 106 cells/mm3, but the compressive strength was decreased for higher cell concentration. Jonkers (2011) also indicated that the amount of healing agents should be decreased to reduce unwanted loss in concrete compressive strength. It can be seen that while too few healing agents will leave many cracks unhealed, too many will decrease compressive strength, mainly due to the empty spaces that appear after capsules rupture, the replacement of a certain portion of sand and gravel with porous carriers, the distribution of non-encapsulated nutrients, or the poor bonding between the healing agents and the concrete matrix. On the other hand, the positive influence of incorporation of healing agents on compressive strength has been reported by several studies (Ersan et al., 2015b; Seifan et al., 2016). According to Bang et al. (2010), the utilization of immobilized S. pasteurii on porous glass beads can considerably improve the compressive strength of the mortar specimen by 24% due to the self-healed cracks.
Therefore, the amount, size, shape, and distribution of healing agent-containing capsules or porous carriers can be optimized to achieve effective self-healing without compromising compressive strength. Moreover, the material of the capsules or porous carriers needs to be carefully selected so that strong bonding is achieved between the healing agents and the concrete matrix.
The research on self-healing concrete has been mainly focusing on laboratory and experimental work, suffering from a lack of numerical simulation tools, which can help optimize the proposed system and reduce experimental costs and time. A computational framework for fungi-mediated self-healing concrete is urgently needed to help understand the effect of the design parameters on the rate and quality of the crack healing and the conditions under which healing progresses in an optimized way without inflicting negative effect on concrete strength.
Besides the three perspectives listed above, we want to point out that using fungi could potentially lower the costs of self-healing concrete, because fungi are easier to acquire, to handle, and to culture in large quantities (Buckley, 2008; Soccol et al., 2017). Moreover, fungi have simpler nutritional needs. Many species of fungi are oligotrophic, surviving by scavenging minute quantities of volatile organic compounds from the atmosphere (Kavanagh, 2011).
Concluding Remarks
Bacteria-mediated self-healing concrete based on biologically induced mineralization processes has been extensively studied during the past decade, but it still has important limitations. Fungi, as one of the best candidates to be used as self-healing agents due to their superior ability to adjust to the deleterious environment of concrete and extraordinary capability to promote calcium mineralization, should no longer be neglected. The investigation on fungi-mediated self-healing concrete is urgently needed.
Author Contributions
All authors listed have made a substantial, direct and intellectual contribution to the work, and approved it for publication.
Funding
This work is supported by the Foundation of Zhongshan Institute of Advanced Engineering Technology, Wuhan University of Technology (grant no. WUT-2018-04).
Conflict of Interest Statement
The authors declare that the research was conducted in the absence of any commercial or financial relationships that could be construed as a potential conflict of interest.
Acknowledgments
CJ expresses her appreciation for the hospitality of Zhongshan Institute of Advanced Engineering Technology, Wuhan University of Technology, when CJ was a visiting scholar there.
References
Ahmad, A., Rautaray, D., and Sastry, M. (2004). Biogenic calcium carbonate: calcite crystals of variable morphology by the reaction of aqueous Ca2+ ions with fungi. Adv. Funct. Mater. 14, 1075–1080. doi: 10.1002/adfm.200400005
American Society of Civil Engineers (2018). America's Infrastructure Report Card, 2017. Available online at: http://www.infrastructurereportcard.org (accessed May 12, 2018).
Arnott, H. J. (1995). “Calcium oxalate in fungi,” in Calcium Oxalate in Biological Systems, ed S. R. Khan (Boca Raton, FL: CRC Press), 73–111.
Association of State Dam Safety Officials (2018). Failures and Incidents at Dams. Available online at: http://damsafety.org/dam-failures (accessed 2018).
Bang, S. S., Galinat, J. K., and Ramakrishnan, V. (2001). Calcite precipitation induced by polyurethane-immobilized Bacillus pasteurii. Enzyme Microb. Technol. 28, 404–409. doi: 10.1016/S0141-0229(00)00348-3
Bang, S. S., Lippert, J. J., Yerra, U., Mulukutla, S., and Ramakrishnan, V. (2010). Microbial calcite, a bio-based smart nanomaterial in concrete remediation. Int. J. Smart Nano Mater. 1, 28–39. doi: 10.1080/19475411003593451
Bindschedler, S., Cailleau, G., and Verrecchia, E. (2016). Role of fungi in the biomineralization of calcite. Minerals 6, 1–19. doi: 10.3390/min6020041
Bruand, A., and Duval, O. (1999). Calcified fungal filaments in the petrocalcic horizon of Eutrochrepts in Beauce, France. Soil Sci. Soc. Amer. J. 63, 164–169.
Buckley, M. (2008). The Fungal Kingdom: Diverse and Essential Roles in Earth's Ecosystem. A report from the American College of Microbiology, 1–48.
Burbank, M. B., Weaver, T. J., Green, T. L., Williams, B., and Crawford, R. L. (2011). Precipitation of calcite by indigenous microorganisms to strengthen liquefiable soils. Geomicrobiol. J. 28, 301–312. doi: 10.1080/01490451.2010.499929
Burford, E. P., Fomina, M., and Gadd, G. M. (2003a). Fungal involvement in bioweathering and biotransformation of rocks and minerals. Mineral Mag. 67, 1127–1155. doi: 10.1180/0026461036760154
Burford, E. P., Hillier, S., and Gadd, G. M. (2006). Biomineralization of fungal hyphae with calcite (CaCO3) and calcium oxalate mono- and dihydrate in carboniferous limestone microcosms. Geomicrobiol. J. 23, 599–611. doi: 10.1080/01490450600964375
Burford, E. P., Kierans, M., and Gadd, G. M. (2003b). Geomycology: fungal growth in mineral substrata. Mycologist 17, 98–107. doi: 10.1017/S0269-915X(03)00311-2
Caracuel, Z., Casanova, C., Roncero, M. I., Di Pietro, A., and Ramos, J. (2003a). pH response transcription factor PacC controls salt stress tolerance and expression of the P-Type Na+ –ATPase Ena1 in Fusarium oxysporum. Eukaryot. Cell 2, 1246–1252. doi: 10.1128/EC.2.6.1246-1252.2003
Caracuel, Z., Roncero, M. I., Espeso, E. A., Gonzalez-Verdejo, C. I., Garcia-Maceira, F. I., and Di Pietro, A. (2003b). The pH signalling transcription factor PacC controls virulence in the plant pathogen Fusarium oxysporum. Mol. Microbiol. 48, 765–779. doi: 10.1046/j.1365-2958.2003.03465.x
Chander, K., Dyckmans, J., Hoeper, H., Joergensen, R. G., and Raubuch, M. (2001a). Long-term effects on soil microbial properties of heavy metals from industrial exhaust deposition. J. Plant Nutr. Soil Sci. 164, 657–663. doi: 10.1002/1522-2624(200112)164:6<657::AID-JPLN657>3.0.CO;2-J
Chander, K., Dyckmans, J., Joergensen, R. G., Meyer, B., and Raubuch, M. (2001b). Different sources of heavy metals and their long-term effects on soil microbial properties. Biol. Fertil. Soils 34, 241–247. doi: 10.1007/s003740100406
Chavez, R., Fierro, F., Garcia-Rico, R. O., and Vaca, I. (2015). Filamentous fungi from extreme environments as a promising source of novel bioactive secondary metabolites. Front. Microbiol. 6:903. doi: 10.3389/fmicb.2015.00903
De Muynck, W., De Belie, N., and Verstraete, W. (2010). Microbial carbonate precipitation improves the durability of cementitious materials: a review. Ecol. Eng. 36, 118–36. doi: 10.1016/j.ecoleng.2009.02.006
De Muynck, W., Debrouwer, D., De Belie, N., and Verstraete, W. (2008). Bacterial carbonate precipitation improves the durability of cementitious materials. Cem. Concr. Res. 38, 1005–1014. doi: 10.1016/j.cemconres.2008.03.005
Dias, M. A., Lacerda, I. C. A., Pimentel, P. F., De Castro, H. F., and Rosa, C. A. (2002). Removal of heavy metals by an Aspergillus terreus strain immobilized in a polyurethane matrix. Lett. Appl. Microbiol. 34, 46–50. doi: 10.1046/j.1472-765x.2002.01040.x
Dick, J., De Windt, W., De Graef, B., Saveyn, H., Van der Meeren, P., De Belie, N., et al. (2006). Bio-deposition of a calcium carbonate layer on degraded limestone by Bacillus species. Biodegradation 17, 357–367. doi: 10.1007/s10532-005-9006-x
Dry, C. (1994). Matrix cracking repair and filling using active and passive modes for smart timed release of chemicals from fibers into cement matrices. Smart Mater. Struct. 3, 118–123.
Edvardsen, C. (1999). Water permeability and autogenous healing of cracks in concrete. ACI Mater. J. 96, 448–454.
Ehrlich, H. L. (1981). “Microbial formation and decomposition of carbonates,” in Geomicrobiology, ed H. L. Ehrlich (New York, NY: Marcel Dekker Inc.), 101–123.
EPOfilms (2018). Hendrik Marius Jonkers: Self-Healing Concrete Containing Bacteria. Available online at: http://www.youtube.com/watch?v=OXkW1q9HpFA (accessed May 12, 2018).
Ersan, Y. C., Da Silva, F. B., Boon, N., Verstraete, W., and De Belie, N. (2015b). Screening of bacteria and concrete compatible protection materials. Constr. Build. Mater. 88, 196–203. doi: 10.1016/j.conbuildmat.2015.04.027
Ersan, Y. C., De Belie, N., and Boon, N. (2015a). Microbially induced CaCO3 precipitation through denitrification: an optimization study in minimal nutrient environment. Biochem. Eng. J. 101, 108–118. doi: 10.1016/j.bej.2015.05.006
Fortin, D., Ferris, F. G., and Beveridge, T. J. (1997). Surface-mediated mineral development by bacteria. Rev. Mineral. 35, 161–180.
Gadd, G. M. (1990). “Fungi and yeasts for metal accumulation,” in Microbial Mineral Recovery, eds H. L. Ehrilich and C. Brierley (New York, NY: McGraw-Hill), 249–275.
Gadd, G. M. (1999). Fungal production of citric and oxalic acid: importance in metal speciation, physiology and biogeochemical processes. Adv. Microb. Physiol. 41, 47–92. doi: 10.1016/S0065-2911(08)60165-4
Gadd, G. M. (2007). Geomycology: biogeochemical transformations of rocks, minerals, metals and radionuclides by fungi, bioweathering and bioremediation. Mycol. Res. 111, 3–49. doi: 10.1016/j.mycres.2006.12.001
Gharieb, M. M., Sayer, J. A., and Gadd, G. M. (1998). Solubilization of natural gypsum (CaSO4·2H2O) and formation of calcium oxalate by Aspergillus niger and Serpula himantioides. Mycol. Res. 102, 825–830. doi: 10.1017/S0953756297005510
Hammes, F., and Verstraete, W. (2002). Key roles of pH and calcium metabolism in microbial carbonate precipitation. Rev. Environ. Sci. Biotechnol. 1, 3–7. doi: 10.1023/A:1015135629155
Hervas-Aguilar, A., Galindo, A., and Penalva, M. A. (2010). Receptor-independent Ambient pH signaling by ubiquitin attachment to fungal arrestin-like PalF. J. Biol. Chem. 285, 18095–18102. doi: 10.1074/jbc.M110.114371
Johnstone, C. G., and Vestal, J. R. (1993). Biogeochemistry of oxalate in the Antarctic cryptoendolithic lichen-dominated community. Microbiol. Ecol. 25, 305–319.
Jonkers, H. M., and Schlangen, E. (2009). A two component bacteria-based self-healing concrete,” in Concrete Repair, Rehabilitation and Retrofitting II, eds M. G. Alexander, H. D. Beushausen, F. Dehn, and P. Moyo (Boca Raton, FL: CRC Press, Taylor and Francis Group), 119–120.
Jonkers, H. M., Thijssen, A., Muyzer, G., Copuroglu, O., and Schlangen, E. (2010). Application of bacteria as self-healing agent for the development of sustainable concrete. Ecol. Eng. 36, 230–235. doi: 10.1016/j.ecoleng.2008.12.036
Kahle, C. F. (1977). Origin of subaerial Holocene calcareous crusts: role of algae, fungi and sparmicristisation. Sedimentology 24, 413–435. doi: 10.1111/j.1365-3091.1977.tb00130.x
Khaliq, W., and Ehsan, M. B. (2016). Crack healing in concrete using various bio influenced self-healing techniques. Constr. Build. Mater. 102, 349–357. doi: 10.1016/j.conbuildmat.2015.11.006
Khan, M., and Scullion, J. (2002). Effects of metal (Cd, Cu, Ni, Pb or Zn) enrichment of sewage-sludge on soil micro-organisms and their activities. Appl. Soil Ecol. 20, 145–155. doi: 10.1016/S0929-1393(02)00018-5
Kis-Papo, T., Grishkan, I., Oren, A., Wasser, S. P., and Nevo, E. (2001). Spatiotemporal diversity of filamentous fungi in the hypersaline Dead Sea. Mycol. Res. 105, 749–756. doi: 10.1017/S0953756201004129
Klappa, C. F. (1979). Calcified filaments in Quaternary calcretes: organo-mineral interactions in the subaerial vadose environment. J. Sediment Petrol. 49, 955–968. doi: 10.2110/jsr.49.955
Kumari, D., Qian, X.-Y., Pan, X., Achal, V., Li, Q., and Gadd, G. M. (2016). Microbially-induced carbonate precipitation for immobilization of toxic metals. Adv. Appl. Microbiol. 94, 79–108. doi: 10.1016/bs.aambs.2015.12.002
Lamb, T. M., Xu, W., Diamond, A., and Mitchell, A. P. (2001). Alkaline response genes of Saccharomyces cerevisiae and their relationship to the RIM101 pathway. J. Biol. Chem. 276, 1850–1856. doi: 10.1074/jbc.M008381200
Li, Q., Csetenyi, L., and Gadd, G. M. (2014). Biomineralization of metal carbonates by Neurospora crassa. Environ. Sci. Technol. 48, 14409–14416. doi: 10.1021/es5042546
Longworthy, T. A. (1978). “Microbial life in extreme pH values,” in Microbial Life in Extreme Environments, ed D. J. Kushner (London: Academic Press), 279–315.
Luo, J., Chen, X., Crump, J., Davies, D. G., Zhou, G., Zhang, N., et al. (2018). Interactions of fungi with concrete: significant importance for bio-based self-healing concrete. Construct. Build. Mater. 164, 275–285. doi: 10.1016/j.conbuildmat.2017.12.233
Magan, N. (2007). “Fungi in extreme environments,” in Environmental and Microbial Relationships, 2nd Edn., The Mycota IV, eds C. P. Kubicek and I. S. Druzhinina (Berlin: Springer-Verlag), 85–103.
Manoli, F., Koutsopoulos, E., and Dalas, E. (1997). Crystallization of calcite on chitin. J. Cryst. Growth 182, 116–124. doi: 10.1016/S0022-0248(97)00318-7
Monger, C. H., and Adams, H. P. (1996). Micromorphology of calcite-silica deposits, Yucca Mountain, Nevada. Soil Sci. Soc. Amer. J. 60, 519–530. doi: 10.2136/sssaj1996.03615995006000020026x
Moore-Landecker, E. (2002). “Fungal spores,” in Encyclopedia of Life Sciences (Chichester: John Wiley & Sons, Ltd). doi: 10.1002/9780470015902.a0000378.pub2
Nobile, C. J., Solis, N., Myers, C. L., Fay, A. J., Deneault, J. S., Nantel, A., et al. (2008). Candida albicans transcription factor Rim101 mediates pathogenic interactions through cell wall functions. Cell Microbiol. 10, 2180–2196. doi: 10.1111/j.1462-5822.2008.01198.x
Panina, L. K., Badlyan, A. G., Bogomolova, E., Wollenzien, U., Gorbushina, A. A., Krumbein, W. E., et al. (1997). EPR-spectra of dark-coloured micromycetes isolated from marble. Mikol. Fitopatol. 31, 46–51.
Penalva, M. A., and Arst, H. N. Jr. (2002). Regulation of gene expression by ambient pH in filamentous fungi and yeasts. Microbiol. Mol. Biol. Rev. 66, 426–446. doi: 10.1128/MMBR.66.3.426-446.2002
Penalva, M. A., and Arst, H. N. Jr. (2004). Recent advances in the characterization of ambient pH regulation of gene expression in filamentous fungi and yeasts. Annu. Rev. Microbiol. 58, 425–451. doi: 10.1146/annurev.micro.58.030603.123715
Penalva, M. A., Tilburn, J., Bignell, E., and Arst, H. N. Jr. (2008). Ambient pH gene regulation in fungi: making connections. Trends Microbiol. 16, 291–300. doi: 10.1016/j.tim.2008.03.006
Phanjom, P., and Ahmed, G. (2015). Biosynthesis of silver nanoparticles by Aspergillus oryzae (MTCC No. 1846) and its characterizations. Nanosci. Nanotech. 5, 14–21. doi: 10.5923/j.nn.20150501.03
Raghukumar, C., and Raghukumar, S. (1998). Barotolerance of fungi isolated from deep-sea sediments of the Indian Ocean. Aquat. Microbiol. Ecol. 15, 153–163. doi: 10.3354/ame015153
Ramachandran, S. K., Ramakrishnan, V., and Bang, S. S. (2001). Remediation of concrete using micro-organisms. ACI Mater. J. 98, 3–9. doi: 10.14359/10154
Ramakrishnan, V. (2007). “Performance characteristics of bacterial concrete: a smart biomaterial,” in Proceedings of the 1st International Conference on Recent Advances in Concrete Technology (Washington, DC), 67–78.
Rautaray, D., Ahmad, A., and Sastry, M. (2003). Biosynthesis of CaCO3 crystals of complex morphology using a fungus and an actinomycete. J. Am. Chem. Soc. 125, 14656–14657. doi: 10.1021/ja0374877
Rautaray, D., Ahmad, A., and Sastry, M. (2004). Biological synthesis of metal carbonate minerals using fungi and actinomycetes. J. Mater. Chem. 14, 2333–2340. doi: 10.1039/b401431f
Rivadeneyra, M. A., Delgado, R., Del Moral, A., Ferrer, M. R., and Ramos-Cormenzana, A. (1994). Precipitation of calcium carbonate by Vibrio spp. from an inland saltern. FEMS Microbiol. Ecol. 13, 197–204. doi: 10.1111/j.1574-6941.1994.tb00066.x
Robinson, C. H. (2001). Cold adaptation in Arctic and Antarctic fungi. New Phytol. 151, 341–353. doi: 10.1046/j.1469-8137.2001.00177.x
Roncero, C. (2002). The genetic complexity of chitin synthesis in fungi. Curr. Genet. 41, 367–378. doi: 10.1007/s00294-002-0318-7
Sancho, L. G., De La Torre, R., Horneck, G., Ascaso, C., De Los Rios, A., Pintado, A., et al. (2007). Lichens survive in space: results from the 2005 LICHENS experiment. Astrobiology 7, 443–454. doi: 10.1089/ast.2006.0046
Sayer, J. A., Kierans, M., and Gadd, G. M. (1997). Solubilisation of some naturally occurring metal-bearing minerals, limescale and lead phosphate by Aspergillus niger. FEMS Microbiol. Lett. 154, 29–35. doi: 10.1111/j.1574-6968.1997.tb12620.x
Seifan, M., Samani, A., and Berenjian, A. (2016). Bioconcrete: next generation of self-healing concrete. Appl. Microbiol. Biotechnol. 100, 2591–2602. doi: 10.1007/s00253-016-7316-z
Silva, F., Boon, N., De Belie, N., and Verstraete, W. (2015). Industrial application of biological self-healing concrete: challenges and economical feasibility. J. Commer. Biotechnol. 21, 31–38. doi: 10.5912/jcb662
Soccol, R. C., Ferreira da Costa, E. S., Letti, L. A. J., Karp, S. G., Woiciechowski, A. L., and Porto de Souza Vandenberghe, L. (2017). Recent developments and innovations in solid state fermentation. Biotechnol. Res. Innov. 1, 52–71. doi: 10.1016/j.biori.2017.01.002
Staley, J. T., Palmer, F., and Adams, J. B. (1982). Microcolonial fungi: common inhabitants on desert rocks. Science 215, 1093–1095.
Sterflinger, K. (2000). Fungi as geologic agents. Geomicrobiol. J. 17, 97–124. doi: 10.1080/01490450050023791
Sterflinger, K., Tesei, D., and Zakharova, K. (2012). Fungi in hot and cold deserts with particular reference to microcolonial fungi. Fungal Ecol. 5, 453–462. doi: 10.1016/j.funeco.2011.12.007
Stocks-Fischer, S., Galinat, J. K., and Bang, S. S. (1999). Microbiological precipitation of CaCO3. Soil Biol. Biochem. 31, 1563–1571.
Takey, M., Shaikh, T., Mane, N., and Majumder, D. R. (2013). Bioremediation of xenobiotics: use of dead fungal biomass as biosorbent. Int. J. Res. Eng. Technol. 3, 565–570.
Tebo, B. M., Johnson, H. A., McCarthy, J. K., and Templeton, A. S. (2005). Geomicrobiology of manganese(II) oxidation. Trends Microbiol. 13, 421–428. doi: 10.1016/j.tim.2005.07.009
Urzi, C., Wollenzien, U., Crizeo, G., and Krumbein, W. E. (1995). “Biodiversity of the rock inhabiting microbiota with special reference to black fungi and yeasts,” in Microbial Diversity and Ecosystem Function, eds D. Allsopp, R. R. Cowell, and D. L. Hawksworth (Wallingford: CAB International), 289–302.
Van Paassen, L. A., Daza, C. M., Staal, M., Sorokin, D. Y., Van Der Zon, W., and Van Loosdrecht, M. C. M. (2010). Potential soil reinforcement by biological denitrification. Ecol. Eng. 36, 168–175. doi: 10.1016/j.ecoleng.2009.03.026
Van Tittelboom, K., De Belie, N., De Muynck, W., and Verstraete, W. (2010). Use of bacteria to repair cracks in concrete. Cem. Concr. Res. 40, 157–66. doi: 10.1016/j.cemconres.2009.08.025
Van Tittelboom, K., De Belie, N., Van Loo, D., and Jacobs, P. (2011). Self-healing efficiency of cementitious materials containing tubular capsules filled with healing agent. Cem. Concr. Comp. 33, 497–505. doi: 10.1016/j.cemconcomp.2011.01.004
Verrecchia, E. P. (2000). “Fungi and sediments,” in Microbial Sediments, eds R. E. Riding and S. M. Awramik (Berlin: Springer-Verlag), 69–75.
Verrecchia, E. P., Braissant, O., and Cailleau, G. (2006). “The oxalate-carbonate pathway in soil carbon storage: the role of fungi and oxalotrophic bacteria,” in Fungi in Biogeochemical Cycles, ed G. M. Gadd (Cambridge: Cambridge University Press), 289–311.
Verrecchia, E. P., Dumont, J. L., and Rolko, K. E. (1990). Do fungi building limestones exist in semi-arid regions? Naturwissenschaften 77, 584–586.
Verrecchia, E. P., Dumont, J. L., and Verrecchia, K. E. (1993). Role of calcium oxalate biomineralization by fungi in the formation of calcretes: a case study from Nazareth, Israel. J. Sediment Petrol 65, 1000–1006.
Volesky, B., and Holan, Z. R. (1995). Biosorption of heavy metals. Biotechnol. Prog. 11, 235–250. doi: 10.1021/bp00033a001
Wang, J., Van Tittelboom, K., De Belie, N., and Verstraete, W. (2012). Use of silica gel or polyurethane immobilized bacteria for self-healing concrete. Constr. Build. Mater, 26, 532–540. doi: 10.1016/j.conbuildmat.2011.06.054
Wang, J. Y., De Belie, N., and Verstraete, W. (2012). Diatomaceous earth as a protective vehicle for bacteria applied for self-healing concrete. J. Ind. Microbiol. Biotechnol. 39, 567–577. doi: 10.1007/s10295-011-1037-1
Wang, J. Y., Snoeck, D., Van Vlierberghe, S., Verstraete, W., and De Belie, N. (2014b). Application of hydrogel encapsulated carbonate precipitating bacteria for approaching a realistic self-healing in concrete. Constr. Build. Mater. 68, 110–119. doi: 10.1016/j.conbuildmat.2014.06.018
Wang, J. Y., Soens, H., Verstraete, W., and De Belie, N. (2014a). Self-healing concrete by use of microencapsulated bacterial spores. Cem. Concr. Res. 56, 139–152. doi: 10.1016/j.cemconres.2013.11.009
Wiktor, V., and Jonkers, H. M. (2011). Quantification of crack-healing in novel bacteria-based self-healing concrete. Cem. Concr. Comp. 33, 763–770. doi: 10.1016/j.cemconcomp.2011.03.012
Wright, V. P. (1986). The role of fungal biomineralization in the formation of early carboniferous soil fabrics. Sedimentol 33, 831–838. doi: 10.1111/j.1365-3091.1986.tb00985.x
Keywords: concrete, self-healing materials, strength, fungi, bacteria
Citation: Jin C, Yu R and Shui Z (2018) Fungi: A Neglected Candidate for the Application of Self-Healing Concrete. Front. Built Environ. 4:62. doi: 10.3389/fbuil.2018.00062
Received: 25 May 2018; Accepted: 11 October 2018;
Published: 29 October 2018.
Edited by:
Antonis Kanellopoulos, University of Hertfordshire, United KingdomReviewed by:
Bao-Jie He, University of New South Wales, AustraliaSukhdeo Rao Karade, Central Building Research Institute (CSIR), India
Copyright © 2018 Jin, Yu and Shui. This is an open-access article distributed under the terms of the Creative Commons Attribution License (CC BY). The use, distribution or reproduction in other forums is permitted, provided the original author(s) and the copyright owner(s) are credited and that the original publication in this journal is cited, in accordance with accepted academic practice. No use, distribution or reproduction is permitted which does not comply with these terms.
*Correspondence: Congrui Jin, cjin@binghamton.edu