Seismic and Fire Assessment and Upgrading Process for Historical Buildings: The Case Study of Palazzo Colonna in Caggiano
- 1Department of Structures for Engineering and Architecture, University of Naples Federico II, Naples, Italy
- 2Independent Researcher, Salerno, Italy
The assessment and retrofit of existing masonry structures with historical and cultural value in highly seismic zones are challenging issues in earthquake engineering. In fact, the historic and recent earthquakes have shown the problem of the seismic vulnerability of existing masonry constructions. A historical masonry palace located in Caggiano (Salerno, Italy) is used herein as a case study, showing the vulnerability assessment and the seismic upgrading process. The case study building has a masonry structural type at the first two floors while there is a third floor realized in reinforced concrete and a fourth floor realized with a wood structure. The building was characterized by a remarkable seismic vulnerability and needed seismic upgrading operations. After the vulnerability assessment process, some design suggestions are proposed for the seismic upgrading of the building. The structure before and after the upgrading operations has been checked through nonlinear static and dynamic analyses. Then, coherently with the “Sismabonus” approach, the attribution of the seismic risk class, performed through numerical analyses, is founded on two parameters, namely, the expected annual mean losses (PAM), related to economic factors, and the Life Safety Index (IS-V), related to the structure seismic safety. Finally, the overcoming of the different classes of risk is shown and compared with the amount of the retrofit operations, their costs, and the impact on the existing space. Moreover, fire assessment has been investigated. In fact, in many cases, the buildings such as the case study structure are intended for public activities such as museums, so specific fire requirements, like fire resistance, are necessary. This topic became relevant especially if the structure is equipped with particular structural retrofit interventions which can be altered and modified in case of a fire. The paper presents the results of advanced thermomechanical analyses on the historical masonry palace under investigation. Since the case study building has a masonry structural type at the first two floors while there is a third floor realized in reinforced concrete, the fire analyses were conducted on the third and fourth floors, which may be more vulnerable to fire.
Introduction
The presented project involves the recovery of Palazzo Colonna and the surrounding areas, of which the Palazzo is the central fulcrum. The project involves a real urban redevelopment aimed at both the recomposition of this piece of the ancient center with the adjacent areas already previously requalified and the creation of safe spaces with a view to recovering the historical image of the site and its safety in case of calamitous events. Within the urban redevelopment project, the Palazzo Colonna is a true dynamic fulcrum, becoming a sort of incubator for cultural activities, strictly connected to the socioeconomic relaunch of the entire community. The surveys showed situations of extreme degradation of some vertical structures, whose roofs and internal horizontals are largely collapsed. The opportunity to make upgrading interventions allows a better use of the entire building sector in conditions of maximum safety. Therefore, the project includes the structural and functional upgrading of the building aimed at creating exhibition spaces, conference rooms, rooms for coworking, and services for the community in general. This work presents the structural assessment and upgrading design for the case study building, considered together with the goal of the functional upgrading of the whole structure. The assessment and upgrading of historical existing buildings in seismic regions are fundamental to reduce the associated risk. The vulnerability assessment of historical masonry structures has been investigated in the literature through different methods and focusing on different modeling issues, issues such as presented in Giordano et al. (2019), Chieffo et al. (2019a), Clementi et al. (2020), Ferrante et al. (2019), and de Silva (2020), to name a few.
Then, after the understanding of the failure mechanics, the best upgrading strategy can be chosen for the structure as a compromise among different needs (structural, architectural, economical, etc.). The criteria for the choice of the retrofit methods have been highly investigated in the literature (Moehle, 2000; Thermou and Elnashai, 2006; Formisano et al., 2008, 2016a, 2017a; Calvi, 2013; Formisano and Sahoo, 2015; Terracciano et al., 2015; Miano et al., 2017b; Chieffo et al., 2019b). These techniques are usually implemented to evaluate the improving of the structural performance and also to evaluate and minimize the costs and the losses (Aslani and Miranda, 2005; Jalayer et al., 2012, 2015; Liel and Deierlein, 2013; Jalayer and Ebrahimian, 2017; Miano et al., 2018a, 2019a,b). Herein, the Pushover Analysis has been used to assess the performance of the building with respect to vertical and seismic loads and to provide an estimation of the vulnerability index (Frascadore et al., 2015; NTC, 2018). The nonlinear dynamic analysis procedures have also been implemented to implement the seismic assessment. Cloud Analysis is selected herein. It is based on a simple regression in the logarithmic space of structural response vs. seismic intensity using a non-scaled ground motions set. The mathematical formulation is very simple, and the application is very quick and efficient for fragility assessment (Jalayer et al., 2007, 2015; Celik and Ellingwood, 2010; Jalayer and Ebrahimian, 2017; Miano et al., 2017a). The seismic assessment of the case structure has shown deficiencies in seismic and vertical loads conditions. The upgrading techniques have been thought to reduce the criticisms by solving the vertical loads deficiencies and reaching a higher level of the vulnerability index. The technique used to improve the structural performance of the masonry is the “Cuciture attive per la muratura” (CAM) technique. Moreover, in the third floor, RC jacketing is used for the columns, while steel jacketing is used for the beams. The vulnerability index values for the limit state of Life Safety (LS) before and after upgrading are estimated. The fragility curve for the limit state of LS is calculated before and after upgrading. Then, a comparison between the results from pushover and Cloud analyses is proposed. The seismic risk class is calculated before and after the upgrading (Guidelines, 2017; Cosenza et al., 2018). The assigned class is a function of two parameters, e.g., the expected annual mean losses (Perdite Annue medie, PAM) and the Life Safety Index (Indice di sicurezza della vita, IS-V).
Moreover, fire assessment has been investigated. In fact, in many cases, the buildings such as the case study structure are intended for public activities such as museums, so specific fire requirements, like fire resistance, are necessary. This topic became relevant especially if the structure is equipped with particular structural retrofit interventions which can be altered and modified in case of a fire. The paper presents the results of advanced thermomechanical analyses of the historical masonry palace, located in Caggiano (Salerno, Italy), hosting a museum. Since the case study building has a masonry structural type at the first two floors while there is a third floor realized in reinforced concrete and a fourth floor realized with a wood structure, the fire analyses were conducted on the third and fourth floors, which may be more vulnerable to fire.
Building Structural Assessment and Upgrading
Building Description
As previously highlighted, the project involves the recovery of Palazzo Colonna and the surrounding areas. The structure is composed of two masonry floors and a reinforced concrete third floor. The building is composed of two blocks placed around a central courtyard characterized by the presence of a central monumental staircase which leads to the main floor. Figure 1 presents the building south view.
The Palace is today morphologically altered by the restoration interventions that have turned it into the town house, after the 1980 Earthquake. These operations have irreversibly compromised the original 18th-century morphology of which the only remains are concentrated in the inner courtyard where there are still visible remains of the decorative apparatuses for which restoration is planned. For the restoration of the decorations, a careful mapping of the identified and classified degradation has been drawn up according to the categories codified by the UNI 11182 (2006) standard. The surveys showed situations of extreme degradation of some vertical structures, whose roofs and internal horizontals are largely collapsed. Figure 2 shows the degradation analysis results for one of the frontal views of the building.
Moreover, particular attention was dedicated to the plant system upgrading. All the solutions respected the fundamental principles of containment of consumption, modularity, maintainability, and reversibility. Then, regarding the respect of the legislation for the overcoming of architectural barriers, all the provisions of the current legislation have been satisfied. Finally, Figure 3 shows a typical floor view of the building. It is possible to see that different thicknesses of the masonry walls are present in the building, varying from about 0.5–1.0 m. The stairs have been realized in masonry. Finally, the slabs are done with a composite system of steel joists and thin hollow bricks and cast in place of concrete.
With respect to the characterization of the mechanical material properties, Table C8.5.1 of the Circolare (2019) has been used. The mechanical properties of the masonry related to “irregular masonry of soft stone (tuff, calcarenite, etc.)” have been chosen. Conservatively, while waiting for specific destructive/non-destructive tests on materials, the minimum value in the range proposed by the code has been taken. The used mechanical material properties are described as follows:
• fm = average compressive strength of the masonry: 140 N/cm2
• τ0 = average shear strength of the masonry: 2.8 N/cm2
• E = average value of the Young modulus: 900 N/mm2
• G = average value of the tangential elasticity modulus 300 N/mm2
• w = average specific weight of the masonry: 16 kN/m3
Finally, it is to note that in the upgrading design, the mechanical properties values have been improved based on the designed operations on the masonry, such as cracks injections, joints restillation, and the scuci-cuci method of patching.
Introduction to the Assessment and Upgrading Operations
The technique used to improve the structural performance of the masonry is the CAM technique. The CAM consists of a “packing” of the masonry with stainless steel strips arranged in the horizontal and vertical directions, passing through the masonry thickness and closed on themselves after applying a pretension. The adoption of a system of tensioning diffused in the three orthogonal directions, in particular also in the transversal direction, improves the monolithic and the mechanical behaviors of the masonry body, increasing its shear strength and bending in plane and out of plane. Through the special connection elements (stainless steel plates, equipped with a funnel-shaped hole), the stainless steel strips allow to create a continuous system of trusses, able to retrace all the irregularities of the masonry, both horizontally and vertically. Moreover, in the third floor, RC jacketing is used for the columns, while steel jacketing is used for the beams.
Nonlinear Static Analysis Procedure
Nonlinear static analysis (e.g., Pushover Analysis) is proposed herein. The CDS (CDS, 2018) software has been used. The modeling of masonry structures is strictly related to the choice of modeling single units or more complex aggregates (Chiumiento and Formisano, 2019). This issue is out of the scope of this paper. Moreover, the identification and calibration of the structural model of masonry buildings are fundamental issues (for example, see Catinari et al., 2017; Clementi et al., 2017, to name a few), but are outside the goals of this work. The structure has been modeled using the finite element method (FEM), inserting two types of elements: (1) one-dimensional line element for RC members and (2) the two-dimensional shell (quad) element for the masonry structure. With respect to masonry structure, initially, a first model schematizes the masonry walls with rectangular two-dimensional elements representative of single-membrane stresses with deformability in their own plane, as plate, therefore, with shear, extensional, and flexion deformability typologies. Then, a second model known as the “equivalent frame,” schematizes the panels and the floor strips with beam-type finite elements with two nodes and rigid offsets to model the panels or the floor band. With respect to RC line members, their force-deformation behavior is evaluated considering that, for each structural member, inelastic action is considered at the ends while the middle remains elastic. The nonlinear moment–curvature relationship in the element ends is obtained through section analysis. The safety verifications are proposed using the limit state method with the partial coefficients (NTC, 2018). LS limit state results are presented herein, while in the design phase, also serviceability limit states have been verified. The LS limit state is achieved when the maximum shear strength (brittle safety verifications) or the maximum rotational capacity (ductile safety verifications) is reached for a structural element. The safety checks for the joints are also considered. Pushover Analysis is implemented based on the code recommendations (NTC, 2018). Vertical loads analysis is done before Pushover Analysis. Then, 32 pushovers are implemented, using two force distributions with an eccentricity of 5% in each direction. The first distribution of the forces is proportional to the floor masses. The second one is proportional to the shape of the first vibration mode (NTC, 2018; Circolare, 2019). Figures 4A,B presents the pushover results (base shear or acceleration vs. top displacement). The 32 pushovers are presented by color in groups of four. Each group corresponds to a certain direction of the forces application ±30% of the orthogonal force. The four pushovers for each direction refer to the two force distributions with the application of ±5% eccentricity associated to the structure geometrical barycenter. The red curves refer to forces parallel to the horizontal positive X-Direction (Figure 3); the blue curves refer to forces parallel to the horizontal negative X-Direction; the black curves refer to forces parallel to horizontal positive Y-Direction; the green curves refer to forces parallel to horizontal negative Y-Direction. For the LS limit state, the verifications are not satisfied. Figure 5A presents for the LS limit state with red color the elements for which the safety checks are not verified and with green color the ones for which the safety checks are verified. A consisting portion of masonry panels, columns, and beams are not verified. The failures of the masonry panels are mainly axial-bending crises. RC columns and beams suffer premature flexural and shear failures. The choice and the investigation of the right retrofit methods are very discussed topics inside the scientific community (Moehle, 2000; Thermou and Elnashai, 2006; Calvi, 2013; Formisano and Mazzolani, 2015; Formisano et al., 2016b, 2017b; Miano et al., 2019b). Herein, the choice has been as a compromise between architectural and structural recommendations, remaining in the economical funding established for this design project. Then, the same procedure has been repeated after the implementation on the upgrading operations. The same modeling approach presented before is used for the upgraded structure. Pushover Analysis has been implemented. A set of 32 pushovers has been implemented using two different distributions of forces and using a 5% eccentricity in each direction. Figures 4C,D present the pushover results (base shear or acceleration vs. top displacement). The red curves refer to forces parallel to horizontal positive X-Direction (Figure 3); the blue curves refer to forces parallel to horizontal negative X-Direction; the black curves refer to forces parallel to horizontal positive Y-Direction; the green curves refer to forces parallel to horizontal negative Y-Direction.
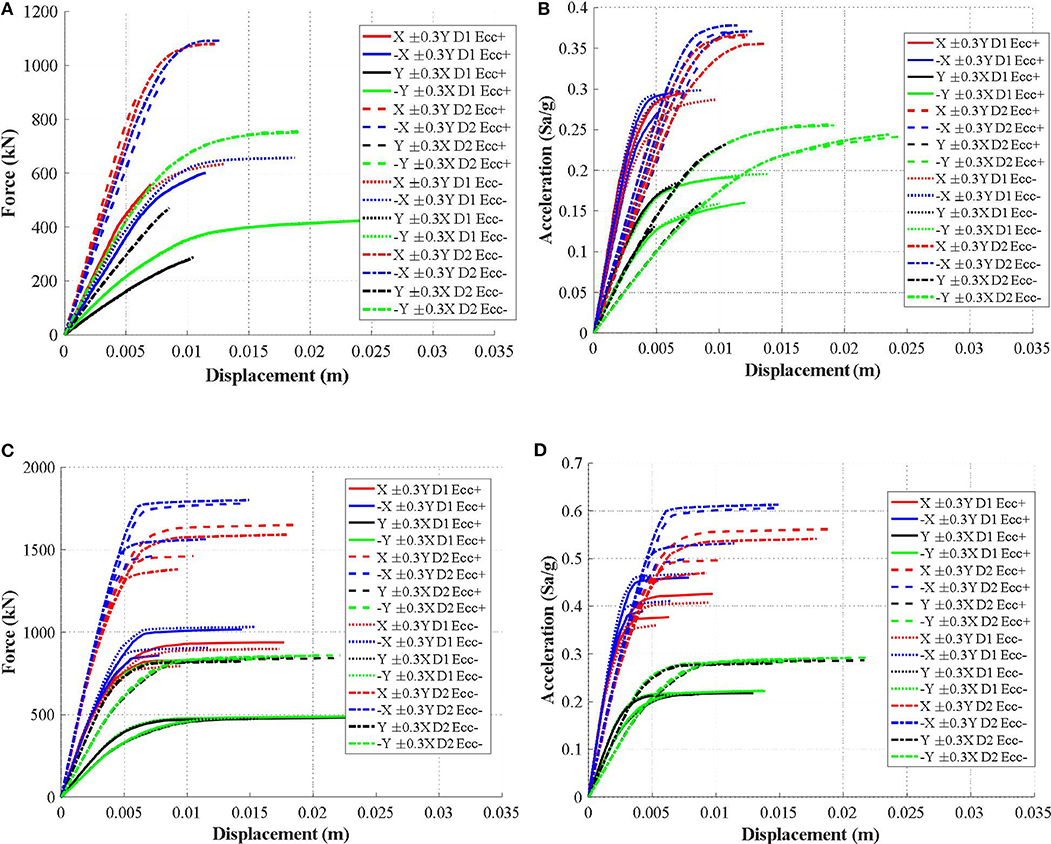
Figure 4. Pushover curves before upgrading operations in terms of (A) base shear/displacement and (B) acceleration/displacement. Pushover curves after upgrading operations in terms of (C) base shear/displacement and (D) acceleration/displacement.
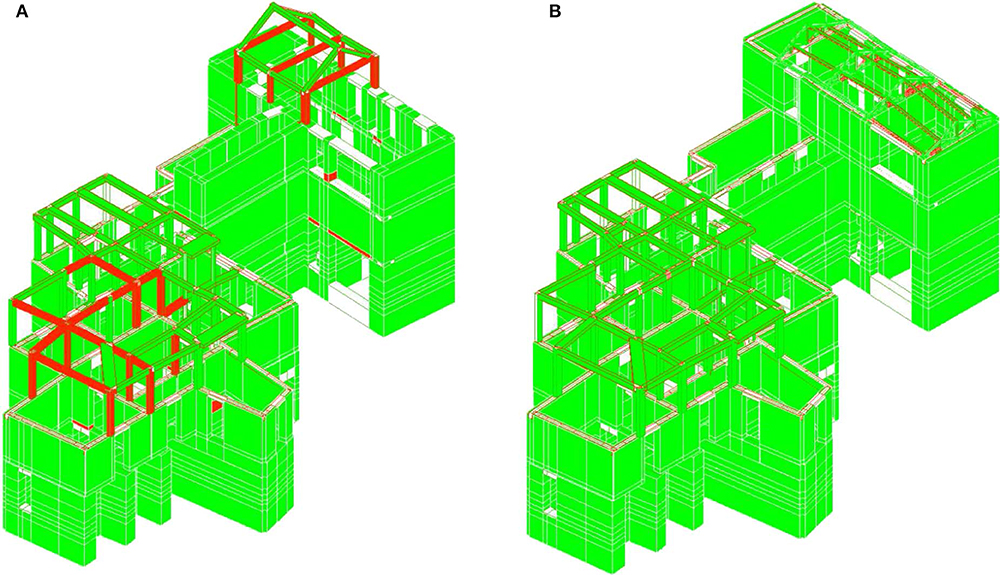
Figure 5. Safety verifications after Pushover Analysis (A) before upgrading operations and (B) after upgrading operations.
Figure 5B presents the LS limit state with red color and the elements for which the safety checks are not verified and with green color the ones for which the safety checks are verified. The number of unverified elements strongly decreases after the upgrading operations. It is to note that some panels are still unsafe, while all the premature failures for the RC members are solved.
Vulnerability Index Calculation
The Italian code (NTC, 2018) recommends to verify the seismic vulnerability before and after upgrading with respect to LS limit state. This process has been followed for the case study structure. The calculation of the vulnerability index (ζE) is defined and discussed in the literature (Frascadore et al., 2015; Cosenza et al., 2018). The ζE is an efficient parameter to evaluate the vulnerability of the building. In this section, a brief summary of the procedure to calculate ζE is presented. For more details, see Frascadore et al. (2015). Based on the pushover response for the Multi Degree of Freedom (MDOF) system, it is possible to have the response for the corresponding Single Degree of Freedom (SDOF). The SDOF is characterized by the period T*, yield strength , and ultimate displacement . The calculation of the capacity in terms of return period and then of peak ground acceleration (PGA), for which the failure is achieved, is based on these parameters. The quantification of the ζE is obtained in the Acceleration Displacement Response Spectrum (ADRS) space (Fajfar, 1999, 2000), in which the abscissas are the spectral displacements and the ordinates are the spectral accelerations. The elastic spectrum has to be scaled until the spectrum containing the point performance (Sae; Sde) of the SDOF is intercepted. It is identified by the inclination line T* and the displacement . The ζE is the ratio between the demand PGA (based on the code recommendations for the LS limit state) and the capacity PGA of the structure:
where PGALS_Capacity is the PGA corresponding to the achievement of the first failure at the LS limit state inside the structure, while PGALS_Demand is obtained from the elastic code spectrum for the specific site for the LS limit state. The ratio between PGALS_Capacity and PGALS_Demand is a measure of the seismic vulnerability of the building with respect to the achievement of the failure condition for the LS limit state. Then, 32 SDOF systems are obtained for the 32 pushovers. The minimum value of these 32 ζE values is considered for the building. The final value of the ζE is 0.19. The building is very vulnerable, and upgrading operations were needed for it. The final value of ζE after the implementation of the upgrading operations described in the previous sections is, instead, 0.60.
Cloud Analysis and Fragility Estimation
The estimation of the analytical fragility curves through nonlinear dynamic analyses can be very useful to have a more complete measure of the vulnerability. In this section, the fragility curves are presented for the investigated structure both before and after the upgrading operations. A group of 30 ground motion records has been applied to the SDOF previously described (e.g., among the 32 SDOF systems, the most penalized SDOF, that minimizes ζE, has been used). A brief summary of Cloud Analysis is proposed. More details about the application of the Cloud Analysis for SDOF systems are provided in Jalayer et al. (2019). The first step of the procedure is to identify the structural response parameter. As presented in Jalayer et al. (2007), the critical demand to capacity ratio for the LS limit state (DCRLS), equal to the mechanism that brings the structure closest to the onset of the limit state, is chosen as the structural response parameter. It can be calculated for each nonlinear time-history analysis. The DCRLS parameter, equal to one at the achieving of the LS limit state, is obtained for the SDOF system as the ratio between the maximum demand in terms of displacement for each record among all the steps of the nonlinear dynamic analysis and the limit state capacity, calculated based on Italian code (NTC, 2018). The LS capacity corresponds to the ultimate displacement of the bilinear system of the SDOF. The ratio between demand and capacity for SDOF and MDOF is equal because both the demand and the capacity are multiplied by the modal participation factor to pass from SDOF to MDOF. Herein, LS limit state is considered, but the procedure can be used for other limit states. This choice allows to have a comparison with the trend of ζE before and after the upgrading operations. The record selection is important to have a proper implementation of Cloud Analysis. A set of 30 records is chosen from both the NGA-West2 and RESORCE database (see Jalayer et al., 2017; Miano et al., 2018b, and Miano et al., 2019a, about the record selection recommendations). This records set has a range of magnitudes between 5.2 and 7.2, and closest distance-to-ruptured area (RRUP) up to around 50 km. The shear wave velocity in the upper 30 m of soil, Vs30, at the structure's site is in higher than 800 m/s (e.g., soil class A, accordingly to Italian code, NTC, 2018). Then, the selected records are mainly on NTC 2018 site classes A and B. Only one of the two horizontal components of each record is considered. The records are free field or measured on the ground level. Table 1 presents the records set.
The structural fragility is estimated through Cloud Analysis. It considers a structure subjected to a group of unscaled records of specific values of the first-mode spectral acceleration Sa(T), adopted herein as the intensity measure (IM) parameter. The records are applied to the structure, and the DCRLS is estimated. This gives a set of values that form the basis for the Cloud Analysis calculations. The statistical properties of the Cloud response are calculated through a logarithmic linear regression applied to the response. This is equivalent to fitting a power-law curve to the cloud response in the original (arithmetic) scale. The result is a curve that finds the median drift demand for a given level of Sa:
where ln(a) and b are the constants of the regression. The logarithmic standard deviation βDCR|Sa is the root mean sum of the square of the residuals:
where DCRi and Sa,i are the DCR values and the corresponding Sa for record number i within the cloud response set, and N is total number of records. In conclusion, the fragility curve based on the Cloud Analysis is:
Figures 6A,B shows the Cloud regression results for the LS limit state with reference to the pre and post upgrading structure. The scatter plots for Cloud Analysis data D = {(Sa, i, DCRLS,i), i = 1:30, for the group of records outlined in Table 1, are shown in Figures 6A,B. For each data point (cyan-colored squares), the corresponding record number is shown. The figures illustrate also the Cloud Analysis regression prediction model (i.e., regression line and the estimated parameters a and b) fitted to the data. The line DCRLS =1, for which LS limit state is achieved, is proposed with a red dashed line. Figure 6C shows the resulting Cloud Analysis-based fragility curve with reference to the bare and the upgraded building. The values of the median and the logarithmic standard deviation of fragility curves are, respectively, 0.25 and 0.32 before upgrading and 0.40 and 0.39 after the upgrading.
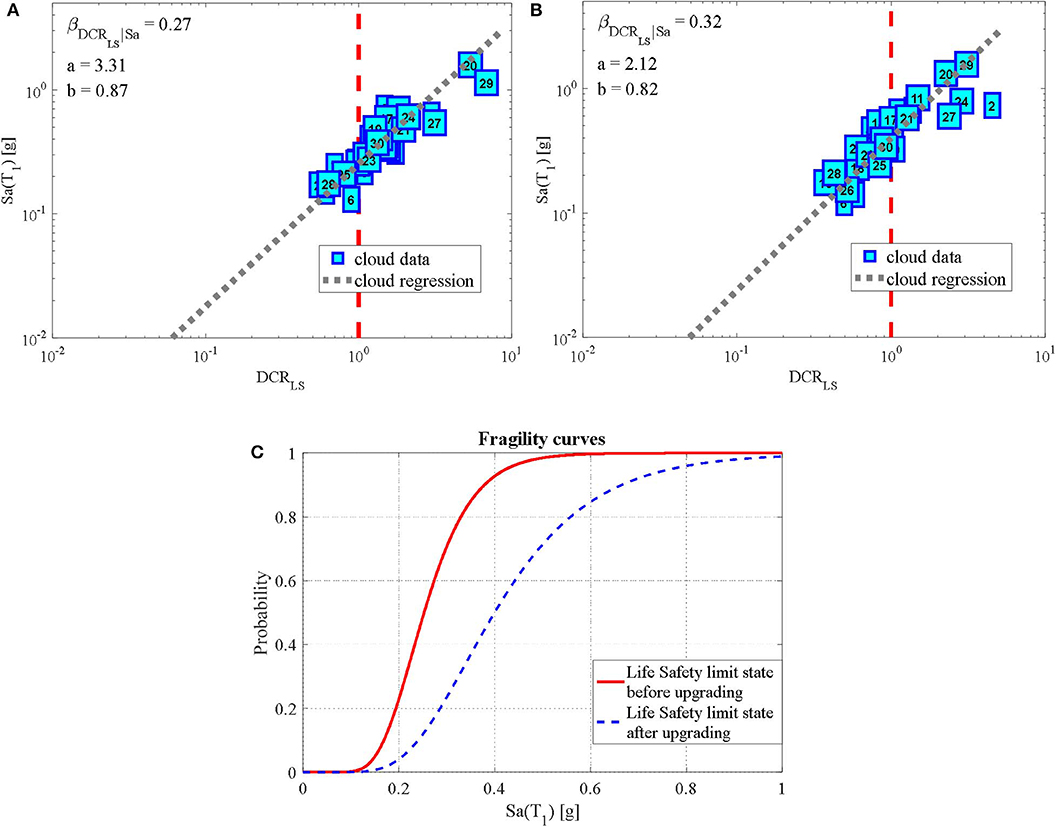
Figure 6. Cloud regression for life safety (LS) (A) before upgrading and (B) after upgrading. (C) Fragility curves before and after upgrading.
Calculation of the Class of Risk Before and After the Upgrading
The class of risk (as suggested in the Italian Guidelines on the seismic classification, Guidelines, 2017 and L.205/17, 2017) is evaluated for the investigated structure before and after the upgrading based on two parameters derived after the assessment process of the building. The economical parameter is the expected annual mean losses (PAM). The structural safety parameter is the Life Safety Index (IS-V). The 2017 Italian law (Balance Law 2017) proposed a campaign for the seismic upgrading of existing buildings (e.g., “Sismabonus” plan). This plan stimulated the evaluation of the existing building and the prevention of the seismic risk. In relation to this, the Italian guidelines provided operational tools for the seismic risk classification of the structures. Eight risk classes, with increasing risk from letter A+ to G, are considered. Two methods for evaluating the class of risk for a structure are considered: the conventional and the simplified methods (Guidelines, 2017). The conventional method is presented for this case study. The steps for determining the class of risk are the following:
• PGA evaluation: the PGAs at the building site, PGAD, for achieving the various limit states are estimated;
• Analysis of the structure: the capacity PGA values, PGAC, for which LS and damage limitation (DL) limit states are reached, are estimated;
• IS-V class definition, as relation between PGAC (for LS limit state) and PGAD of the site. The percentage value, calculated based on Table 2.1 defined by the guidelines, defines the seismic risk class as a consequence of vulnerability index;
• Vulnerability analysis: it defines the damages (structural and not structural) based on the achievement of predefined levels by the response parameters, calculating return periods, TrC, and its inverse annual average frequency of exceeding λ; reconstruction cost percentage (CR%) associated with λ values for the limit states considered;
• PAM class definition as the area under the curve of the direct economic losses, calculated as function of the annual average frequency of exceeding the events that produce the achievement of a predefined limit state. The PAM Class definition is based on Table 2.2 of the guidelines.
Then, the risk class is defined as the worst class between the PAM and the IS-V classes. Figure 7 shows risk class evaluation pre and post upgrading.
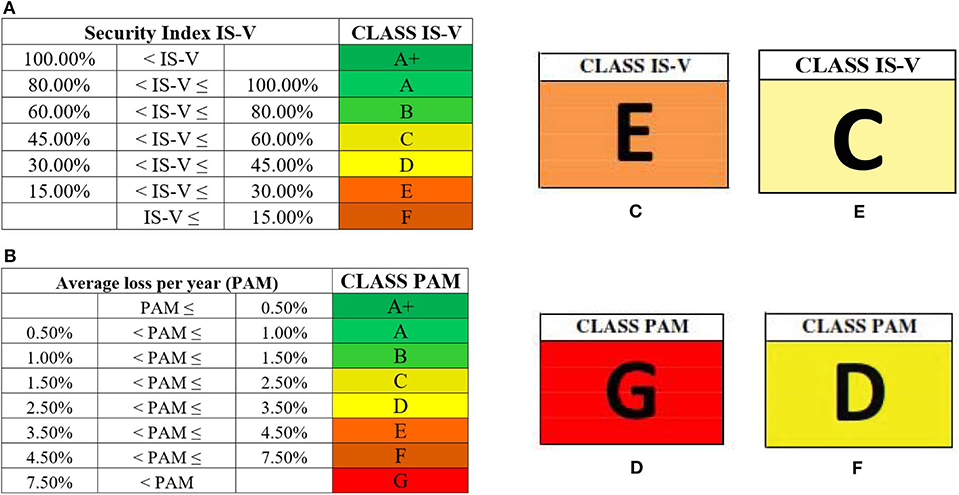
Figure 7. (A) Life Safety Index (Indice di sicurezza della vita, IS-V) risk classes definition. (B) Expected annual mean losses (Perdite Annue medie, PAM) risk classes definition. (C) IS-V class before upgrading. (D) PAM class before upgrading. (E) IS-V class after upgrading. (F) PAM class after upgrading.
Fire Resistance Assessment
Structural fire safety is one of the primary aspects that has to be considered in the design of buildings especially where the intended of use involves particularly fire-risky activities (e.g., elevated fire loads) or the structure materials are vulnerable to fire (e.g., steel or wood). Indeed, given its nature, the most vulnerable material to fire is steel, where sometimes the passive fire protections are necessary (de Silva et al., 2017, 2019), while the fire performance of the masonry and concrete has often been taken for granted considering its noncombustible nature and ability to function as a thermal barrier, preventing heat and fire spread. The low concrete thermal conductivity, compared to steel, cause inside the fire-exposed concrete members, strong temperature gradients and the core region may take a long time to heat up. Thus, while the compressive strength of concrete is rapidly lost beyond a critical temperature, which is not too dissimilar to the equivalent temperature for loss of steel strength, structural effectiveness is not affected until the bulk of the material reaches the same temperature. This requires an analysis of the thermal response of the entire structural element (Fletcher et al., 2007).
At present, structural fire safety (fire resistance) of members is generally achieved through prescriptive approaches, which are based on standard fire resistance tests or empirical calculation methods. These prescriptive based approaches (PBAs) are design approaches that will allow the building to meet a preset standard when it comes to fire safety. Applying already deemed to satisfy solutions to the design of the building will inherently be safe, it is a code-compliant building. Therefore, a building that is designed in accordance with the code is considered an acceptable building from a fire risk perspective.
Sometimes, the building deviates from a “standard” building, and it is very difficult to impossible to apply the standard set of rules set out in the code. This of course does not necessarily mean that the building is unsafe; it just means that it cannot comply with the deemed to satisfy solutions. In these cases, the Fire Engineering Approach (FEA) could be useful for the evaluation of the real level of building fire safety. Indeed, the recent move toward performance-based fire design, by using the Fire Safety Engineering (FSE) criteria, has increased the focus on the use of advanced computer simulations for evaluating fire resistance of structure. In particular, the FEA consists of detailed analysis of the fire, considering natural fire curves, which combine more sophisticated calculation (advanced methods) for structural models and the temperature distribution in the elements, the mechanical and geometric nonlinear structural responses are taken into account.
This requirement, implemented in the National Codes of European member countries, is explained by achieving the following five performance levels:
- the load-bearing capacity of the construction can be assumed for a specific period of time
- the generation and spread of both fire and smoke within the works are limited
- the spread of fire to neighboring construction works must be limited
- occupants have to be able to leave the works or be rescued by other means
- the safety of rescue teams must be taken into consideration.
The results of each application of the FEA to the fire safety should be evaluated through the analysis of the achievement of these objectives. Figure 8 explains the steps for applying the FEA.
In the following, the PBA and the FEA were applied to the case study of Palazzo Colonna in Caggiano. In particular, since the main structure of Palazzo Colonna in Caggiano is in masonry, the concrete part of the structure is analyzed, with the aim of observing all the effects that may arise in the structural elements during fire.
Fire Modeling
The fire event represents a very complex phenomenon involving different interacting physical and chemical processes, such as combustion, radiation, and transport phenomena under turbulent conditions. Fire evolution and the severity of the consequences depend on the boundary conditions, such as the confined or unconfined environment. Given the complexity of the scenarios, the simulation tools are becoming fundamental for design purposes of buildings in case of fire. Indeed, numerical modeling approaches allow to obtain cost-effective and reproducible information, with a degree of confidence dependent on the quality of the input information and the assumptions made by the model itself. For a building-confined fire, like in this case, the most accredited models are the zone models. Zone models are numerical tools used for the evaluation of the gases temperature within a compartment during a fire, and they are based on several hypothesis. The main hypothesis in zone models is that the compartment is vertically divided into zones (Figure 9), and each zone has homogeneous properties in terms of temperature and composition: a hot layer with combustion products, located near the ceiling, and a cold layer with fresh clean air at the bottom, separated by a moving interface, which is considered an adiabatic surface. The zone properties (and the layer height) can vary over time according to global conservation equations (mass and energy). In particular, in one zone model (Figure 9B), the temperature is considered to be uniform within the whole compartment. This type of model is thus valid in case of generalized fires, contrary to two zones models, which are valid in case of localized fires.
These models are implemented in the software (Cadorin and Franssen, 2003), which is used for the simplified fluid-dynamic analyses in this case study. In the following, all the details are described.
Performance Level, Fire Scenarios, and Fire Models
The main objective of fire safety checks concerns the mechanical resistance and stability of the building in case of fire. In the case of PBA, the required time of fire resistance is 90 min of standard fire curve, while for the FEA, the safety performance level required for the structure is maintaining the fire resistance requirements for the duration of the natural fires. For the definition of the natural fire curves, the description of the structure fire scenarios is necessary. The design fire scenario is a qualitative description of the fire development during the time, identifying key events that characterize the fire (Del Prete et al., 2016). Since each floor has a different occupancy like museum and offices, the scenario is chosen to maximize the fire risk based on engineering judgment. In particular, the analyzed fire scenario consists of the library located at the third floor of the structure. Furthermore, this part of the structure is in concrete, while the other parts are in masonry.
The library was considered a fire compartment of 36 m2, and the post-flashover fire is modeled by two-zone model, which assumes homogeneous temperature, density, internal energy, and pressure of the gas in the compartment. The combustion model was considered as “extended fire duration,” which supposes that the release of mass may be limited by the quantity of oxygen available in the compartment. The total mass of fuel is burnt inside the compartment (safe procedure) then the fire duration is increased compared to the input one (Cadorin et al., 2001). In this model, no external combustion is assumed, the entire fire load delivers its energy inside the compartment. If the fire is ventilation controlled, the pyrolysis rate is proportional to the oxygen coming in the library and is not a physical model because pyrolysis is not directly dependent on oxygen concentration. It has been established for design procedures in order to avoid uncertainties on the maximum pyrolysis rate per unit floor area and therefore to be on the safe side concerning the fire duration. In this case, the fire load was estimated equal to 500 MJ/m2 of paper.
Figure 10A shows the data RHR, obtained in accordance with EN 1991-1-2 (2002) and the RHR computed obtained as a result of Ozone. Figure 10A shows that the fire is ventilation-controlled, indeed, the fire is limited by the availability of oxygen in the compartment and the RHR computed has a lower stationary phase than the RHR data. This is confirmed also by the comparison between the ventilation factor (Ocomp) of the compartment and the critic ventilation factor (Ocr) (Compagnone et al., 2017):
Equation (5) shows that Ocomp < Ocr; this is the case in which the ventilation controls the fire.
Figure 10B shows the natural fire curve of the library compared with the standard fire curve, which gives higher temperatures and therefore more demanding for the structure.
Thermomechanical Analyses
As said before, the thermomechanical analyses purpose concerns the mechanical resistance and stability of the building in fire. In the case of PBA, the required time of fire resistance is 90 min of standard fire, while for the FEA, the safety performance level required for the structure is maintaining the structural stability for the duration of the library natural fire. In the case of PBA, the simplified tabular method is considered, while applying the FEA criteria, advanced thermomechanical analyses were conducted on the concrete part of the building, selected as significant substructure (Figure 11).
Prescriptive Based Analyses Results
The PBA approach was considered applying the simplified tabular data according with Table 5.2a and Table 5.5 of the Eurocode (EN 1992-1-2, section 5).
Table 2 shows that the beam “B1” does not satisfy the required resistance time of 90 min, while for the other elements, both the bmin and a dimensions, allow to reach 90 min.
Since the tabular data are often very conservative, an analysis of the substructure subjected to the ISO 834 curve was carried out in order to check the eventual differences and to take into account the structural thermal effects, which are neglected in the tabular method. The results are shown in Figure 12. Figure 12 shows the variation of the bending moment during the thermal transitory due to the statically redundancies and restraints to thermal expansions, which causes the emergence of additional stresses in structural elements. As Table 2 shows, the only element which does not satisfy the 90 min of fire resistance is the beam “B1” with tabular data; furthermore, the same beam satisfies the request if modeled within the entire structure always with ISO834 curve, with a sufficient margin of safety (Figure 13A); this is the demonstration of the conservative of the simplified tabular data. Furthermore, Figure 13 shows that, on the one hand, the resistance of the beam decreases with the increase of time (because the temperature is increasing), and on the other hand, the stress bending moment increases due to the structure redundancies.
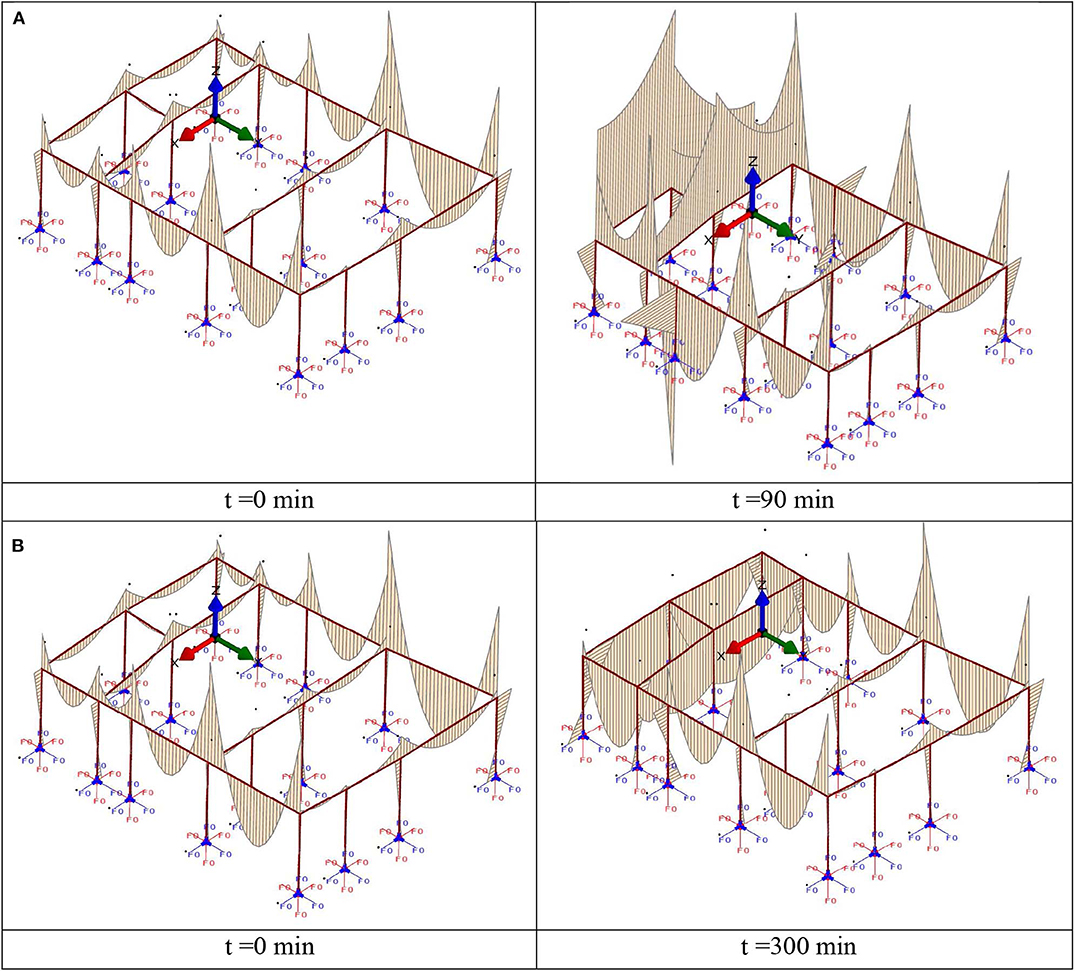
Figure 12. (A) Bending moment on the substructure with ISO834 fire curve. (B) Bending moment on the substructure with library fire curve.
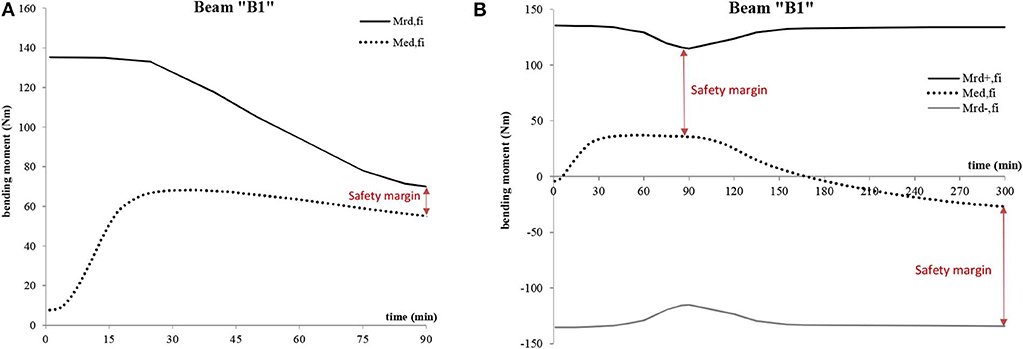
Figure 13. (A) Stress and strain on beam “B1” with ISO834 fire. (B) Stress and strain on beam “B1” with library fire curve.
Performance-Based Analyses Results
In order to evaluate the real level of fire safety of the concrete substructure, an advanced thermomechanical analysis was conducted with the library fire curve, checking the stability of the substructure for the entire duration of the library fire (about 300 min).
In addition, in this case, there is a variation of the bending moment during the thermal transitory due to the structural redundancy, but without causing the structural collapse. The beam “B1,” which is the weakest element in the tabular data analyses, is verified also in this case, with a greater safety margin than the case of the ISO834 fire curve (Figure 13B).
Conclusions
The assessment and retrofit of existing masonry structures with historical and cultural value in highly seismic zones are challenging issues in earthquake engineering. A historical masonry palace, located in Caggiano (Salerno, Italy), currently hosting a museum of the country culture of the town and previously a location of the town municipality, is used herein as a case study to show the vulnerability assessment and the seismic upgrading process for the existing masonry structures with historical and cultural value. The case study building has a masonry structural type at the first two floors, while there is a third floor realized in reinforced concrete and a fourth floor realized with a wood structure. The masonry palace has a configuration of the structural system similar to the big part of the masonry buildings of the historical center of different Italian towns. In fact, most of these Italian masonry buildings were built in a time period where the knowledge about the seismic behavior of structures, performance of materials, and earthquake actions was very limited. As a consequence, these are characterized by a remarkable seismic vulnerability and need seismic upgrading operations compatibility with existing spaces and the “Italian authority for the preservation of cultural heritage” recommendations. After the vulnerability assessment process, some design suggestions are proposed for the seismic upgrading of the building. The structure before and after the upgrading operations has been checked through nonlinear static and dynamic analyses. Then, coherently with the “Sismabonus” approach, the attribution of the seismic risk class, performed through numerical analyses, is founded on two parameters, namely, the expected annual mean losses (PAM), related to economic factors, and the Life Safety Index (IS-V), related to the structure seismic safety. Finally, the overcoming of the different classes of risk is shown and compared with the amount of the retrofit operations, their costs, and the impact on the existing space. Moreover, fire assessment has been investigated. In fact, in many cases, the buildings such as the case study structure are intended for public activities such as museums, so specific fire requirements, like fire resistance, are necessary. This topic became relevant especially if the structure is equipped with particular structural retrofit interventions which can be altered and modified in case of a fire. The paper presents the results of advanced thermomechanical analyses of the historical masonry palace, located in Caggiano (Salerno, Italy), hosting a museum. Since the case study building has a masonry structural type at the first two floors while there is a third floor realized in reinforced concrete, where a library is located, the fire analyses were conducted on the third floor, which may be more vulnerable to fire, making the fire load considerable. The structural fire analyses show that the simplified tabular analyses are conservative, indeed, the beam, which does not satisfy the required R90, is verified if an advanced analysis of a substructure is conducted, referring to the ISO834 fire curve.
The safety check is even more precise in the case of a natural fire curve analysis, where the library fire curve is considered. In this case, the safety margin is greater than the ISO834, since the input temperatures and therefore the temperatures in the structural elements are, on average, lower. This result underlines the efficacy of using the PBA, which certainly returns more reliable results.
Data Availability Statement
The datasets generated for this study are available on request to the corresponding author.
Author Contributions
AM is responsible for the organization and implementation of this research. GC is responsible for the structural calculations. MC is responsible for the architectural design project. DS is responsibile for the fire assessment.
Conflict of Interest
The authors declare that the research was conducted in the absence of any commercial or financial relationships that could be construed as a potential conflict of interest.
References
Aslani, H., and Miranda, E. (2005). Probabilistic Earthquake Loss Estimation and Loss Disaggregation in Buildings (dissertation), Stanford University, Stanford, CA, United States.
Cadorin, J.-F., and Franssen, J.-M. (2003). A tool to design steel elements submitted to compartment fires—OZone V2. Part 1: pre- and post-flashover compartment fire model. Fire Saf. J. 38, 395–427. doi: 10.1016/S0379-7112(03)00014-6
Cadorin, J. F., Pintea, D., and Franssen, J. M. (2001). The Design Fire Tool OZone V2.0 - Theoretical Description and Validation On Experimental Fire Tests. Liègi: University of Liegi.
Calvi, G. M. (2013). Choices and criteria for seismic strengthening. J. Earth Eng. 17, 769–802. doi: 10.1080/13632469.2013.781556
Catinari, F., Pierdicca, A., Clementi, F., and Lenci, S. (2017). “Identification and calibration of the structural model of historical masonry building damaged during the 2016 Italian earthquakes: the case study of Palazzo del Podestà in Montelupone,” in AIP Conference Proceedings, Vol. 1906 (Thessaloniki: AIP Publishing LLC), 090013.
Celik, O. C., and Ellingwood, B. R. (2010). Seismic fragilities for non-ductile reinforced concrete frames–Role of aleatoric and epistemic uncertainties. Struct. Safety 32, 1–12. doi: 10.1016/j.strusafe.2009.04.003
Chieffo, N., Clementi, F., Formisano, A., and Lenci, S. (2019a). Comparative fragility methods for seismic assessment of masonry buildings located in Muccia (Italy). J. Build Eng. 25:100813. doi: 10.1016/j.jobe.2019.100813
Chieffo, N., Formisano, A., and Miguel Ferreira, T. (2019b). Damage scenario-based approach and retrofitting strategies for seismic risk mitigation: an application to the historical Centre of Sant'Antimo (Italy). Eur. J. Env. Civ. Eng. 1–20. doi: 10.1080/19648189.2019.1596164
Chiumiento, G., and Formisano, A. (2019). Simplified and refined analyses for seismic investigation of historical masonry clusters: Comparison of results and influence of the structural units position. Built. Environ. 5:4. doi: 10.3389/fbuil.2019.00084
Circolare (2019). NTC 2018 Commentario: Supplemento ordinario alla Gazzetta Ufficiale, n. 35 del 11 Febbraio 2019. Ministero delle Infrastrutture e dei Trasporti, Circolare 21 Gennaio 2019 “Istruzioni per applicare dell'≪Aggiornamento delle Norme tecniche per le costruzioni≫.
Clementi, F., Ferrante, A., Giordano, E., Dubois, F., and Lenci, S. (2020). Damage assessment of ancient masonry churches stroked by the Central Italy earthquakes of 2016 by the non-smooth contact dynamics method. Bull. Earthq. Eng. 18, 455–486. doi: 10.1007/s10518-019-00613-4
Clementi, F., Mezzapelle, A., Cocchi, G., and Lenci, S. (2017). “Global analyses of historical masonry buildings: equivalent frame vs. 3D solid models,” in AIP Conference Proceedings, Vol. 1863 (Rhodes: AIP Publishing LLC) 450006.
Compagnone, A., Bilotta, A., and Nigro, E. (2017). “Fire resistance evaluation through fragility curves,” in IFireSS 2017 – 2nd International Fire Safety Symposium (Naples).
Cosenza, E., Del Vecchio, C., Di Ludovico, M., Dolce, M., Moroni, C., Prota, A., et al. (2018). The Italian guidelines for seismic risk classification of constructions: technical principles and validation. Bull. Earthq. Eng. 16, 5905–5935. doi: 10.1007/s10518-018-0431-8
de Silva, D., Bilotta, A., and Nigro, E. (2017). “Effect of the thermal input on the behaviour of intumescent coatings,” in Proocedings of ASFE (2017) - Applications of Structural Fire Engineering (Manchester), 325–334.
de Silva, D., Bilotta, A., and Nigro, E. (2019). Experimental investigation on steel elements protected with intumescent coating. Constr. Build. Mat. 205, 232–244. doi: 10.1016/j.conbuildmat.2019.01.223
de Silva, F. (2020). Influence of soil-structure interaction on the site-specific seismic demand to masonry towers. Soil Dyn. Earthq. Eng. 131:106023. doi: 10.1016/j.soildyn.2019.106023
Del Prete, I., Cefarelli, G., and Nigro, E. (2016). Application of criteria for selecting fire scenarios for structures within fire safety engineering approach. J. Build. Eng. 8, 208–217. doi: 10.1016/j.jobe.2016.10.010
EN 11991-1-2 (2002). Eurocode 1: Actions on structures - Part 1-2: General Actions – Actions on Structures Exposed to Fire. Brussels.
Fajfar, P. (1999). Capacity spectrum method based on inelastic demand spectra. Earth Eng. Struct. Dyn. 28, 979–994. doi: 10.1002/(SICI)1096-9845(199909)28:9<979::AID-EQE850>3.0.CO;2-1
Fajfar, P. (2000). A nonlinear analysis method for performance-based seismic design. Earth Spectra 16, 573–592. doi: 10.1193/1.1586128
Ferrante, A., Clementi, F., and Milani, G. (2019). Dynamic behavior of an inclined existing Masonry tower in Italy. Front. Built Environ. 5:33. doi: 10.3389/fbuil.2019.00033
Fletcher, I. A., Welch, F., Torero, J. L., Carvel, R. O., and Usmani, A. (2007). The behavior of concrete structures in fire. Thermal Sci. 11, 37–52 doi: 10.2298/TSCI0702037F
Formisano, A., Castaldo, C., and Chiumiento, G. (2017a). Optimal seismic upgrading of a reinforced concrete school building with metal-based devices using an efficient multi-criteria decision-making method. Struct. Infrastruct. Eng. 13, 1373–1389. doi: 10.1080/15732479.2016.1268174
Formisano, A., Chiumiento, G., Fabbrocino, F., and Landolfo, R. (2017b). “Comparative seismic evaluation between numerical analysis and Italian Guidelines on Cultural Heritage applied to the case study of a masonry building compound,” in AIP Conference Proceedings, Vol. 1863, (Rhodes: AIP Publishing), 450008.
Formisano, A., De Matteis, G., Panico, S., and Mazzolani, F. M. (2008). Seismic upgrading of existing RC buildings by slender steel shear panels: A full-scale experimental investigation. Adv. Steel Constr. 4, 26–45. doi: 10.18057/IJASC.2008.4.1.3
Formisano, A., Lombardi, L., and Mazzolani, F. M. (2016a). Perforated metal shear panels as bracing devices of seismic-resistant structures. J. Constr. Steel Res. 126, 37–49. doi: 10.1016/j.jcsr.2016.07.006
Formisano, A., Lombardi, L., and Mazzolani, F. M. (2016b). Full and perforated metal plate shear walls as bracing systems for seismic upgrading of existing rc buildings. Ingegneria Sismica 33, 16–34.
Formisano, A., and Mazzolani, F. M. (2015). On the selection by MCDM methods of the optimal system for seismic retrofitting and vertical addition of existing buildings. Comput. Struct. 159, 1–13. doi: 10.1016/j.compstruc.2015.06.016
Formisano, A., and Sahoo, D. R. (2015). Steel shear panels as retrofitting system of existing multi-story RC buildings: Case studies. Adv. Struct. Eng. Mech. 1, 495–512. doi: 10.1007/978-81-322-2190-6_41
Frascadore, R., Di Ludovico, M., Prota, A., Verderame, G. M., Manfredi, G., Dolce, M., et al. (2015). Local strengthening of reinforced concrete structures as a strategy for seismic risk mitigation at regional scale. Earth Spectra 31, 1083–1102. doi: 10.1193/122912EQS361M
Giordano, E., Clementi, F., Nespeca, A., and Lenci, S. (2019). damage assessment by numerical modeling of Sant'Agostino's sanctuary in offida during the Central Italy 2016–2017 seismic sequence. Front. Built Environ. 4:87. doi: 10.3389/fbuil.2018.00087
Guidelines (2017). Guidelines for the Seismic Risk Classification of Constructions. Decreto del Ministero delle Infrastrutture e dei Trasporti.
Jalayer, F., Beck, J., and Zareian, F. (2012). Analyzing the sufficiency of alternative scalar and vector intensity measures of ground shaking based on information theory. J. Eng. Mech. 138, 307–316. doi: 10.1061/(ASCE)EM.1943-7889.0000327
Jalayer, F., De Risi, R., and Manfredi, G. (2015). Bayesian Cloud Analysis: efficient structural fragility assessment using linear regression. Bull. Earth Eng. 13, 1183–1203. doi: 10.1007/s10518-014-9692-z
Jalayer, F., and Ebrahimian, H. (2017). Seismic risk assessment considering cumulative damage due to aftershocks. Earthq. Eng. Struct. Dyn. 46, 369–389. doi: 10.1002/eqe.2792
Jalayer, F., Ebrahimian, H., and Miano, A. (2019). “N2 with cloud: a non-linear dynamic analysis procedure for the equivalent SDOF system,” in XVIII Convegno ANIDIS “L'ingegneria sismica in Italia” (Ascoli Piceno).
Jalayer, F., Ebrahimian, H., Miano, A., Manfredi, G., and Sezen, H. (2017). Analytical fragility assessment using un-scaled ground motion records. Earth Eng. Struct. Dyn. 46, 2639–2663. doi: 10.1002/eqe.2922
Jalayer, F., Franchin, P., and Pinto, P. E. (2007). A scalar damage measure for seismic reliability analysis of RC frames. Earthq. Eng. Struct. Dyn. 36, 2059–2079. doi: 10.1002/eqe.704
L.205/17 (2017). Balance Law 2018, Official Gazette n. 302 of 29/12/17. Ordinary Supplement n. 62 (in Italian).
Liel, A. B., and Deierlein, G. G. (2013). Cost-benefit evaluation of seismic risk mitigation alternatives for older concrete frame buildings. Earth Spectra 29, 1391–1411. doi: 10.1193/030911EQS040M
Miano, A., Jalayer, F., Ebrahimian, H., and Prota, A. (2018a). Cloud to IDA: efficient fragility assessment with limited scaling. Earth Eng. Struct. Dyn. 47, 1124–1147. doi: 10.1002/eqe.3009
Miano, A., Jalayer, F., Ebrahimian, H., and Prota, A. (2019a). “Nonlinear dynamic analysis procedure with limited number of analyses and scaling,” in Proceedings of 7th ECCOMAS Thematic Conference on Computational Methods in Structural Dynamics and Earthquake Engineering (COMPDYN)(Crete).
Miano, A., Jalayer, F., and Prota, A. (2017a). “Considering structural modeling uncertainties using bayesian cloud analysis,” in Proceeding of 6th ECCOMAS Thematic Conference on Computational Methods in Structural Dynamics and Earthquake Engineering (COMPDYN) (Rhodes).
Miano, A., Sezen, H., Jalayer, F., and Prota, A. (2017b). “Performance based comparison of different retrofit methods for reinforced concrete structures,” in Proceeding of the 6th ECCOMAS Thematic Conference on Comp Methods in Struct Dyn and Earth Eng (COMPDYN) (Rhodes).
Miano, A., Sezen, H., Jalayer, F., and Prota, A. (2018b). “Performance Based Assessment and Retrofit of Non ductile Existing Reinforced Concrete Structures,” in Proceeding of the Structures Conference (Fort Worth).
Miano, A., Sezen, H., Jalayer, F., and Prota, A. (2019b). Performance-based assessment methodology for retrofit of buildings. ASCE J. Struct. Eng. 145:04019144. doi: 10.1061/(ASCE)ST.1943-541X.0002419
Moehle, J. P. (2000). “State of research on seismic retrofit of concrete building structures in the US,” in US-Japan Symposium and Workshop on Seismic Retrofit of Concrete Structures (Tokio).
NTC (2018). D. M. Infrastrutture Trasporti 17 gennaio 2018, Norme tecniche per le Costruzioni. G.U. 20. Suppl. Ord.
Terracciano, G., Di Lorenzo, G., Formisano, A., and Landolfo, R. (2015). Cold-formed thin-walled steel structures as vertical addition and energetic retrofitting systems of existing masonry buildings. Eur. J. Environ. Civil Eng. 19, 850–866. doi: 10.1080/19648189.2014.974832
Thermou, G. E., and Elnashai, A. S. (2006). Seismic retrofit schemes for RC structures and local-global consequences. Prog. Struct. Eng. Mater. 8.1, 1–15. doi: 10.1002/pse.208
Keywords: historical buildings, seismic vulnerability, fire resistance, upgrading, masonry buildings, Sismabonus
Citation: Miano A, de Silva D, Chiumiento G and Capasso ML (2020) Seismic and Fire Assessment and Upgrading Process for Historical Buildings: The Case Study of Palazzo Colonna in Caggiano. Front. Built Environ. 6:22. doi: 10.3389/fbuil.2020.00022
Received: 24 December 2019; Accepted: 17 February 2020;
Published: 12 March 2020.
Edited by:
Francesco Clementi, Marche Polytechnic University, ItalyReviewed by:
Nicola Chieffo, Politehnica University of Timişoara, RomaniaAngela Ferrante, Marche Polytechnic University, Italy
Ersilia Giordano, Marche Polytechnic University, Italy
Copyright © 2020 Miano, de Silva, Chiumiento and Capasso. This is an open-access article distributed under the terms of the Creative Commons Attribution License (CC BY). The use, distribution or reproduction in other forums is permitted, provided the original author(s) and the copyright owner(s) are credited and that the original publication in this journal is cited, in accordance with accepted academic practice. No use, distribution or reproduction is permitted which does not comply with these terms.
*Correspondence: Andrea Miano, andrea.miano@unina.it