- 1Zero Carbon Lab, School of Creative Arts, University of Hertfordshire, Hatfield, United Kingdom
- 2Centre for Future Societies Research, University of Hertfordshire, Hatfield, United Kingdom
Extreme weather conditions increase the frequency of regular maintenance on heritage buildings and cause erosion of traditional materials. Developments in bio-enhanced self-repair materials provide an opportunity to improve building performance and reduce the frequency of costly maintenance schedules. The microbial sequestration of carbon by bacteria, encapsulated and layered into several limewash coats, facilitates capturing atmospheric carbon and reduces carbon-generating maintenance regimes. The use of hydrogels, alginates and biofilm derived biopolymers as novel bacterial encapsulation and nutrient delivery vehicles is discussed and the opportunity to develop self-healing sacrificial limewash as a future research project. Microbial enhanced carbon-fixing limewash may also offer a broader application to improve the performance of sustainable materials such as hemp-lime bio-composites as a fast-forward projection of problems and solutions with these materials in the future.
1 Introduction
The goal of this review is to improve the long-term CO2 performance of lime substrates by applying a bioactive-carbonating limewash to protect the renders on heritage building which are at-risk from the effects of climate change. The objective is to propose a biological approach to extend limewash performance on building exteriors which are subjected to weather erosion and because of this approach improve long-term capture of atmospheric CO2.
Extreme weather patterns generate global droughts, floods, wind-driven rain, changes in pH and biological attack (Table 1) which threaten heritage building longevity. Climate change is challenging existing conservation policy for listed heritage buildings such as potential conflicts with zero carbon programmes and insulation retrofits (Brimblecombe, Grossi and Harris, 2011).
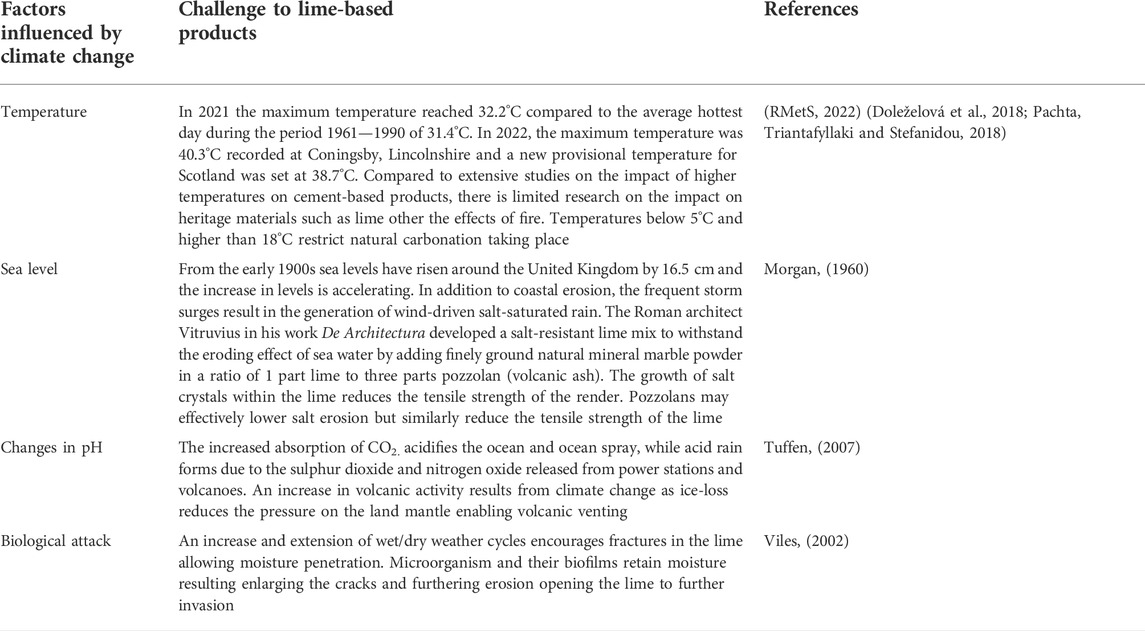
TABLE 1. Environmental factors influenced by climate change and their impact on lime-based products.
This study examines the effect climate change will have on heritage buildings particularly those buildings on at-risk registers and in private ownership. Maintenance and upgrade programme costs for listed buildings are rising rapidly, driven by limited artisan skills, traditional material shortages and increasing supplemental costs, such as building scaffolding and complying with health and safety regulations. A comparison of 30 listed churches in England revealed a range of expenditure for average cost of repairs in the region of £100–250 k while the speed of deterioration is so rapid such expenditure is insufficient to maintain building stability (Historic England, 2019). Without substantial changes to conservation policies, extreme weather events will ultimately result in a decline in built heritage assets. The development of more robust self-healing biomaterials such as bio-limewash, will help to address maintenance costs while improving the sequestering of atmospheric CO2 and extending the longevity of heritage assets.
2 Background
Aware of the challenge, the United Kingdom government, directed by the 2008 Climate Change Act, publishes a climate change risk assessment (CCRA) every 5 years (UK Government, 2008). Legislation directed toward moderating greenhouse gases and their contribution toward solar heat reflection, is under development. The CCRA3 Risk Independent Assessment 2021, is a result of more than 3 years of work based on the latest scientific evidence from over 450 experts on weather-related hazards informing the CCRA3 Government Report. The CCRA3 Report has published several themed factsheets including how cultural heritage has been assessed and the types of action necessary to adapt to climate change risk.
Pre-1919 homes constitute approximately 20% of the total housing stock across the United Kingdom. Unlisted pre-1919 buildings more readily accommodate a retrofit intervention to reduce the carbon footprint (Hamot and George, 2021). In contrast, listed properties as defined in Planning Legislation (HM Government, 1997) are governed by less-agile heritage policies restraining the adoption of zero carbon emission goals and adaptive strategies to climate change.
Biodesigned applications offer a sustainable approach to address this challenge by enhancing traditional construction materials. This paper considers how lime render can be modified to extend built heritage lifespan and performance, reduce maintenance costs and lower CO2 emissions. This review examines current microbe cementitious carbonation technologies and explores options to enhance the sacrificial role of limewash to slow down the speed of climate accelerated lime render deterioration.
Lime was originally selected on availability, aesthetic qualities, and a proven track record of preserving buildings (Carran et al., 2012). The microscopic structure of lime facilitates moisture permeability and when saturated forms a watertight outer skin limiting the accumulation of trapped water.
Ecologically sustainable, lime slowly absorbs CO2 hardening while it carbonates. It has a lower embodied energy than concrete and the potential to embed atmospheric carbon for the lifetime of the building.
The increase in anthropogenic airborne pollutants and extreme weather erosion undermine the long-term longevity of lime-based products. The physical degradation of lime render and mortar arises from the chemical removal of the calcium ions by dissolved atmospheric acidic gases and by chemical substitution with sulphates and chlorides. As erosion occurs, spaces form in the lime providing damp niches for chemotrophs which produce toxic compounds of ammonia and nitrite salts and as they die form a nutrient base for other organisms. Traditionally, limewash is applied to extend the life of the underlying render and mortar by providing a sacrificial surface which is more easily repaired under a regular maintenance schedule, protecting the building, and reducing ongoing costs. Extreme weather events result in more intense wind-driven rain, halving the lifespan of the limewash layer. Doubling the frequency of the maintenance schedule is likely to be cost prohibitive and raise the carbon footprint of the building. A bio-enhanced limewash layer can reduce underlying lime render damage and can also provide a protective layer to a wider range of materials such as hemp-lime.
Hemp-lime can reduce or eliminate carbon emissions from conventional construction processes which can shorten the time taken to achieve net zero targets (Bharadwaj, Jankovic and Carta, 2021). As hemp-lime bio-composite material comes with negative embodied emissions of -108 kg CO2/m3 (Bevan and Woolley, 2008) resulting from sequestration of carbon dioxide in the hemp plant during its growth, the use of this material leads to a significant reduction in embodied emissions. Building performance improvements resulting from hemp-lime bio-composites are stable internal air temperatures and relative humidity. The inclusion of hemp-lime into historic building repairs is of growing interest for low energy consumption and occupant health in housing (Eberlin and Jankovic, 2014), as well as in non-residential projects where stable temperatures and relative humidity help with the preservation of museum artefacts (Leskard, 2022) or pharmaceutical products (Couch, Perry and Wilkes, 2014).
3 Literature review
Carbonates in varying forms of limestone account for nearly 42% of the total carbon on the planet, a significant portion of which is biogenic in origin (Zhu and Dittrich, 2016). Carbon sequestration by microbial CO2 fixation is now recognised as an emerging and promising technology (Rossi et al., 2015). Photosynthetic microbes, such as cyanobacteria and microalgae, contribute to capturing CO2 (Kumar et al., 2011). In addition to the environmental benefits, the commercial opportunities of exploiting environmentally beneficial microbial products are significant. Microbial biologics in 2015 were valued at US$ 277 billion, estimated to reach US$ 400 billion by 2025 (Grand View Research, 2017). Microbials contribute toward generated lime-concrete CO2 micro-encapsulating pastes (Wang and Soens, 2014), biopolymers (Moradali and Rehm, 2020), biocides and biosurfactants (Fidanza and Caneva, 2019; Płaza and Achal, 2020), biofilm generated bioelectricity (Nealson, 2017), biofuels (Kumar et al., 2018) and brownfield site bioremediation (Megharaj and Naidu, 2017). The economic value of the carbon-fixing global market in the future is likely to be significantly greater than past estimates.
3.1 Lime render and weather erosion
Lime is produced from burning calcium-based rocks at a temperature of 900°C forming unstable calcium oxide (CaO) (Figure 1). Calcium oxide is “slaked” or hydrated with water, to form lime putty, or dampened aggregate to form “hot-lime.” The addition of water to calcium oxide is violently exothermic producing thixotropic wet hydrate, traditionally preferred by artisans. Hydrated lime undergoes induration from atmospheric carbon dioxide at a temperature above 5°C and with a residual moisture level. The carbonation process absorbing atmospheric CO2 occurs at 5 mm per month from the outer skin working inwards (Young, 2008).
It is the outer stone or render that is under threat from climate change (Table 1) causing extended drought and flood cycles, rain acidity (H2SO3, HNO3) resulting in changes in pH, increasing temperature, biological attack, and storm force winds (Sabbioni, Brimblecombe and Lefevre, 2008). Wind driven rain and rainwater salination in coastal areas drive salts which accumulate in weathered microcracks. During periods of drought, salt crystallisation exerts significant pressure within the spaces in the lime resulting in material failure.
By the 19th century use of lime mortar declined as Portland cement, an easy to use, fast curing and high compression strength material, became widely used. Cement has two drawbacks, it can fracture and become brittle, and it is a major contributor to atmospheric CO2 (Blankendaal, Schuur and Voordijk, 2014). Researchers are exploring ways to lower these CO2 emissions due to the extensive use of concrete in construction, though the costs remain prohibitive (Scrivener, John and Gartner, 2018).
Lime in comparison is a time-consuming material as the application requires a build of several layers which is dependent on weather conditions and availability of competent skills. Inappropriate conservation treatments such as epoxy resins and Ba(OH)2 solution are environmentally toxic and hamper moisture permeability which causes irreversible damage to the microstructure of the lime (Rodriguez-Navarro et al., 2003). Additives such as sealants and pozzolans attempt to enhance the adherence, waterproofing and antiseptic properties of the lime. Over the centuries the application of lime on buildings have included additives such as marine salt (1811), skimmed milk (1881), warm slaughterhouse blood mixed with stale beer (1883), flour (1887), sugar (1890), and molasses (1913) (Taliaferro, 2015). Artisans believed the addition of blood improved binding strength, weather resistance and carbonation. This may be a result of blood protein hydrolysis within the alkaline environment (Fangquiang et al., 2015).
Concrete despite the ease of application and high compressive strength, is subject to cracking. Increased brittleness generates microcracks allowing water and pollutants to entry undermining long-term structural integrity. Thermal expansion between different component materials reduces material strength which over time leads to environmental pollution (de Muynck et al., 2008).
Early attempts to incorporate microbial repair mechanisms to strengthen concrete, involved casting the concrete with Bacillus spores and calcium lactate (C6H10CaO2) as a nutrient embedded in clay pellets. As cracks form, water splits the pellets resuscitating the dormant bacteria. The bacteria then form calcium carbonate (CaCO3) deposits, sealing the cracks (Jonkers, 2007; Wiktor and Jonkers, 2011). During this microbial repair process, the compressive strength of the concrete was noticeably improved (Bang et al., 2010). However, anaerobes such as ureolytic bacteria generate eco-toxic by-products such as ammonia while precipitating calcium carbonate through biomineralisation, thereby contributing to toxic run-off. In contrast, autophototrophic bacteria fix atmospheric carbon forming strong calcite layers within the cracks and avoid the production of toxic by-products (Zhu and Dittrich, 2016).
3.2 Mechanisms of bacterial precipitation of calcium carbonate
Microbial precipitation results from metabolic activities which are either heterotrophic (e.g., urea hydrolysis) or autotrophic. The negatively charged outer cell membrane binds divalent cations such as Ca2+, resulting in the organism forming a crystal nucleation site. As urea hydrolyses into CO2 and ammonia, bio-deposition increases both the surrounding pH and resulting carbonate concentration (Chahal, Rajor and Sidique, 2011). Active microbial CaCO3 precipitation accelerates with cell metabolism at a faster rate than passive chemical precipitation (Stocks-Fischer, Galinat and Bang, 1999) demonstrated by improvements in CO2 sequestration in Chlorella sp. and Spirulina platensis up to 46% (Ramanan et al., 2010).
Three autotrophic metabolic pathways are involved in bacterial calcium carbonate formation (Castanier et al., 1999), non-methylotrophic methanogenesis, anoxygenic photosynthesis and oxygenic photosynthesis (Figure 2). All three pathways use CO2 as a carbon source and in the presence of calcium ions, produce a precipitation of calcium carbonate.
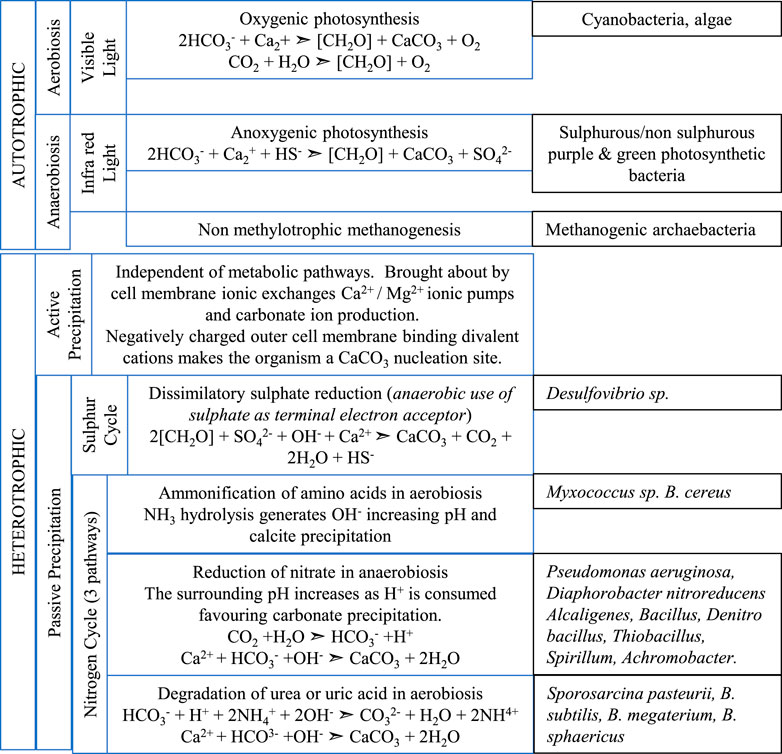
FIGURE 2. Comparison between autotrophic and heterotrophic bacterial production of CaCO3. A redox generated high environmental pH is common across metabolic pathways. Autotrophic bacteria: Aerobiosis (Dupraz and Visscher, 2005), Anaerobiosis (Baumgartner et al., 2006; Reeburgh, 2007), Heterotrophic bacteria: Active Precipitation (Stocks-Fischer, Galinat and Bang, 1999), Passive precipitation (sulphur cycle) (Baumgartner et al., 2006; Braissant et al., 2007), (nitrogen cycle pathways) (Lee, 2003; Rodriguez-Navarro et al., 2003; Kavazanjian and Karatas, 2008; González-Muñoz et al., 2010; Jroundi et al., 2010; Achal and Mukherjee, 2015; Erşan, de Belie and Boon, 2015; Wei et al., 2015).
Photosynthesis is the principal contributor to the production of carbonate rocks (Altermann et al., 2006) such as cyanobacteria formation of stromatolitic carbonate speleothems in the photic zone of carbonate caves (Léveillé, et al., 2007). Photosynthesis leads to calcite precipitation by conducting an HCO3−/OH− exchange across the cell membrane increasing the pH around the cells. By diffusion or via a symporter, CO2 enters the cell wall (Espie and Kandasamy, 1992), the CO2 is then synthesised into organic matter while bicarbonate is converted to CO2 and OH−, the latter released out of the cell increasing the pH of the external environment. Cyanobacteria are the only organism that utilise H2O as an electron donor during photosynthesis and the degree of light intensity is critical for this photosynthetic pathway (Kumar et al., 2011). Low intensity light limits the biomass productivity whereas high intensity can cause photo-inhibition. (Rubio Camacho et al., 2003).
When microbial carbonate is formed, the carbonate adheres to the original material while retaining moisture permeability (Rodriguez-Navarro et al., 2003). Importantly, microbial CaCO3 deposition conforms to conservation standards and does not alter the appearance of the stone (Jroundi et al., 2010).
3.3 Methods for bacterial inclusion into a cementitious matrix
Bacterial inclusion into a cement or lime material follows three widely used methods, direct application, immobilisation, and encapsulation (Table 2) (Griño, Daly and Ongpeng, 2020). The simplest method directly applies live bacterial cells or spores with or without supporting nutrients to the concrete mix. Any micro spaces in the concrete fill with calcite precipitate, improving the overall compression strength (Ghosh et al., 2005). Due to the high alkaline environment, researchers have used alkaliphilic or alkali-tolerant strains that are capable of spore formation such as Bacillus sphaericus, a ureolytic, alkali-tolerant spore forming microbe. However, unprotected cells cannot endure the harsh environmental conditions and may not survive long enough to provide sufficient calcite repair (Wang and Soens, 2014; Li et al., 2019).
To improve live cell viability and precipitation, bacterial cells can be immobilised within a protective material. Bacteria immobilised within graphite nano-platelets and light weight aggregates can extend calcite precipitation up to 28 days (Khaliq and Ehsan, 2016). Other protective materials include limestone powder (Shaheen, Khushnood and Ud Din, 2018), iron oxide nanoparticles (Seifan et al., 2018), polyurethane (Bang, Galinat and Ramakrishnan, 2001) and sepiolite (Sandalci, Tezer and Basaran Bundur, 2021) which, subject to availability of nutrients, can extend viability for up to a year. A third method for inclusion into a cement or lime paste is to encapsulate the bacteria or spores within a biodegradable capsule providing a mechanical buffer during application and enclosed nutrients to extend cell viability.
3.3.1 Bacterial encapsulation
Encapsulation reduces the risk of physical or chemical damage to cells or spores prior to release into the cementitious matrix. The design of the capsule material must ensure encapsulation does not hinder carbonate precipitation, access to water, deteriorate the lime or cement matrix chemical profile nor reduce compression strength. Successful cell encapsulation becomes a function of surface texture, shell thickness and diameter (Joseph et al., 2010). Changes to the wet/dry curing environments can also influence the self-healing response of encapsulated cells (Wang et al., 2012). Microbial immobilisation using encapsulation is a more robust approach compared to solid or fluid microbe inclusion. For the process of encapsulation to be economic, consideration must include consistent evidence of microbial survival, longevity in transportation and ease of usage at the site of application.
3.3.2 Cell encapsulation technologies
The food, medical and environmental sectors utilise encapsulation for the introduction of targeted microbial cells as a means of extending the life and effectiveness of the microbes beneficial metabolic processes, with each technology adapted to its specific application (Table 3).
Bashan et al. (2002) inoculated soil using microbeads produced by a low-pressure spray of suspended bacterial culture in a highly nutrient liquid base mixed into an alginate solution. The resulting suspension expressed as small diameter droplets, which when sprayed through a calcium chloride solution hardened to form 100–200 μm containing colony forming units. The microbeads produced were viable and when added to wet or dry mediums could resist a standard freeze-drying procedure. Within 15-days within a moist environment the microbeads successfully biodegraded within the medium.
This example illustrates just one of the methods used to immobilise and encapsulate at the micro level, others include flocculation, adsorption to surfaces, covalent bonding to a carrier, intercellular cross-linking, polymer-gel encapsulation, and matrix entrapment (Cassidy, Lee and Trevors, 1996). Each of these technologies require the selection of a polymer which will perform appropriately for the chosen application. There are a wide range of synthetic and natural polymers available. Natural biopolymers are more likely to be compatibility with environmentally sustainable goals than synthetics and better equipped to provide a supportive environment for microbial growth when used as the encapsulating medium.
3.4 Encapsulation polymers
3.4.1 Algal polysaccharides
Alginate and κ-carrageenan are two natural polymers, the guluronic acid in alginates for example, will readily form cross-linked polymer networks when exposed to Ca2+ ions. Alginates are linear polymers of β (1,4)—D-mannuronic acid and a (1,4)-L-guluronic acid monomers which are found in nature in varying configurations and displaying a wide range of properties (da Silva et al., 2014; Dhamecha et al., 2019). The cross-linked matrix encloses spaces which can entrap, protect, and immobilise cells. Calcium alginate has a thick, large pore alginate matrix ideal for bacterial occupation (Voo et al., 2016). Bacteria held within calcium alginate beads consistently generate calcite precipitation when compared to control groups (Soysal et al., 2020). As calcium alginate beads biodegrade, they provide sufficient time for a steady supply of nutrients and calcium ions for ongoing microbial carbonate precipitation. Microorganisms are encapsulated into the alginate using the traditional syringe method to produce the alginate beads which form in the range of 0.5–3.5 mm diameter (Lancy and Tuovinen, 1984). More advanced techniques can reduce the size of the beads down to 120 μm (Musgrave et al., 1983).
Algal polysaccharide beads increase the surface for cell attachment for encapsulated microorganisms allowing for a substantial increase in cellular metabolism. Alginate encapsulated Saccharomyces cerevisiae cells produced 80% more ethanol when compared to planktonic cells (Galazzo and Bailey, 1990).
Carrageenan produced by red algae such as Chondrus crispus offer a varied structural diversity composed of linear chains of β(1,3)-D-galactose and α(1–4)-D-galactose units which form a robust encapsulation gel (Perrechil et al., 2020). The encapsulation gel can be formed by extruding carrageenan and cell suspensions at a temperature of 42°C into a cold solution of potassium chloride. The risk of denaturing several of the temperature sensitive proteins within the cells can be mitigated through the addition of lotus bean or carob bean gum. This technique has been used for the large-scale production of encapsulated microorganisms for the treatment of contaminated soil sites (Hulst et al., 1985). Manufacturing techniques can generate industrial production capability of encapsulated alginate more than 24 lhr−1 using resonance nozzles, rotating disk atomisers, low pressure ultrasonic nozzles and parallel plate electrostatic droplet generators (Ogbonna et al., 1989; Stormo and Crawford, 1992). The alginate and carrageenan compounds improve encapsulation by providing chemical and mechanical stability, ensuring a more effective release of the capsule contents and protect the cells if exposed to freeze/thaw cycles (Poncelet et al., 1994; Malhotra and Basir, 2020; Sarıyer et al., 2020).
3.4.2 Pectin
Like alginate, pectin is an anionic polysaccharide derived from plant cell walls and is composed of long sequenced partially methyl-esterified (1–4)-linked a D-galactosyluronic acid which forms a natural hydrogel with the addition of Ca2+ divalent ions (Yang, Mu and Ma, 2018). A simple hydrogel encapsulation technique incorporates the cell suspension with CaCO3 and sodium alginate, which can be either extruded or applied as an emulsion (Liu, Xie and Nie, 2020). The thick stable wall of the pectin capsule exerts a controlled release and reducing the stress on the capsule contents.
3.4.3 Hydrogels
Initial encapsulation methods utilised a porous aggregate to encase the bacterial spores and nutrients (Jonkers, 2007). Hydrogels are a broader range of compounds which include alginate and pectin, and similarly consist of a hydrophilic gel of cross-linked polymer chains in which bacteria or spores are held and from which water is dispersed. Several hydrogels including calcium alginate provide a non-toxic, renewable natural source with properties such as a well-structured matrix and large pores, ideal for encapsulating bacterial cells (Voo et al., 2016). Hydrogel encapsulation mimics an intracellular environment by holding over 90% water (Oyen, 2014). The slow release of water held in the hydrogel matrix extends a protection to the cells from physical and chemical damage and provides water to facilitate metabolic CaCO3 precipitation (Wang and Snoeck, 2014).
3.4.4 Bacterially generated biopolymers—Biofilms
A challenge for biopolymer immobilisation technology is their relatively low mechanical strength. Instability in the protective capsule will result in untimely release of bacteria and premature cell death. This can be addressed by reducing the size of the capsule to nano or micro encapsulation which demonstrates improved cell survival rates and cell lifespan (Jampílek and Králová, 2017; Prasad, Bhattacharyya and Nguyen, 2017).
A key advantage provided by bacterial biopolymers is a three-dimensional space which can accommodate the microorganism. Bacteria produce four primary polymer classes, polysaccharides, polyesters, polyamides and inorganic polyanhydrides each expressing diverse properties. Microbial extracellular polymeric substance (EPS) or exopolymers, produced as a survival mechanism by bacterial cells, adhere to both hydrophobic and hydrophilic surfaces assisting in the formation of three-dimensional bacterial biofilm architectures (Decho and Gutierrez, 2017). As bacterial cells produce the biofilm it provides a highly effective barrier to toxic molecules. The barrier raises the minimum inhibitory concentration of cytotoxins compared to that needed to destroy planktonic cells. Biofilm biopolymers are more resistance to mechanical stress by utilising electrostatic and hydrophobic forces, offering structural protection to resist deforming forces (Billings et al., 2015).
3.5 Biopolymer enhancements
3.5.1 Additives
Introducing organic or inorganic additives to biopolymers during the encapsulation process can further enhance the physical properties for polymer encapsulation (Table 4). Additives are application specific, but if incompatible with the polymer may impede the encapsulation process (Viveganandan and Jauhri, 2000; Liu et al., 2015). The addition of the additive can enhance bio-carbonation and is an area for future research to advance encapsulation technologies. The addition of lectins -carbohydrate-binding proteins—to biofilm derived biopolymers improve the linkages between the bacterial cells and the exopolysaccharides in the capsule wall and improve the encapsulation success rate (Table 4).
3.5.2 Microfibres
The addition of microfibres together with the encapsulated bacteria to the lime medium can further advance the bio-carbonation process. The coupling effect between the added fibres loaded with encapsulated bacteria into the three-dimensional matrix of the fibre and the addition of calcium lactate as a precursor improves the efficiency and extends the bio-carbonation period (Luo, Qian and Li, 2015; Su et al., 2021). Environmentally compatible fibres such as cellulose also encourage the bacterial cells to produce EPS possibly by causing genomic or proteomic changes to the cells (Gupta, Kua and Tan Cynthia, 2017; Singh and Gupta, 2020).
3.5.3 Bacterial selection
The highest bio-carbonation efficiency can be determined by the encapsulation of the most appropriate non-pathogenic bacteria. Within a recent comprehensive review into the performance of several Bacillus sp. incorporated into concrete mixes, Bacillus halodurans demonstrated the highest efficiency in spore formation, survival, and calcium carbonate formation (Sri Durga et al., 2021).
4 Designing a limewash encapsulation technology
Souradeep and Kua, (2016) identified an eight-factor checklist to evaluate the effectiveness of a self-healing system in concrete substrates. Six of these factors can be adapted to evaluate the selection of an effective system for use in limewash encapsulation.
1. The capsule wall must be sufficiently robust to protect the capsule contents during mixing and sufficiently thin to trigger a timely release of the bacterial healing agent.
2. A uniform density of the capsules contained throughout the limewash will allow a consistent bacterial release across the application area.
3. The timing of the release of the healing agent must be sufficiently responsive to be available when and where the bio-carbonation is required.
4. As the capsules fracture and empty the bacterial contents, the capsule fragments must not impair the structural integrity of the limewash.
5. The release of the capsule contents must both maintain the viscosity of the limewash to allow for uniform distribution of the capsules and be sufficiently viscous for the bacteria to be retained at the point of application.
6. The survival rate of the bacteria directly relates to the stability and releasing mechanism of the capsule polymer. Spores provide a more robust bioactive content (available for up to 6 months) whereas active bacterial cells will respond immediately on release from the capsule but have a limited lifespan (Wiktor and Jonkers, 2011).
The merits of developing a bacterial-enabled limewash for use on lime render and lime composites are promising as a slower eroding limewash sacrificial layer when exposed to extreme weather events has environmental and economic benefits.
Based on this review, there are four areas which inform the design for limewash encapsulation and extend the natural carbonation process through bio-carbonation.
1. Selection of a micro or nano encapsulation technology of no more than 100–200 μm to protect the capsule contents from physical stress.
2. Development of an EPS derived biopolymer able to sustain living bacterial cells and trigger their timely release during the limewash application process.
3. Assess which additive, microfibre and bacterial species combine to maximise bio-carbonation as the limewash carbonates between applications to the stone or render.
4. Design a density formulation for the bacterial capsules which allows a uniform bacterial release which does not impair the characteristics of the limewash.
5 Next steps
Local authorities are bound by statutory obligations within heritage conservation policy that may limit local authority discretion to consider innovative alternatives within the consent process. Conservation principles are also challenged to acknowledge a key ambition of the United Kingdom Government set out by the Government Construction Strategy to systematically reduce carbon emissions. The resulting growing body of environmental legislation may be an opportunity for the introduction of new technologies and alternative conservation products to expand the portfolio of traditional materials needed to address extreme weather events. Any new conservation product must be subject to rigorous review resulting in the development of technical and safety data sheets, environmental product declarations and supported by building application guides.
Biodesigned materials for use within the construction industry can extend beyond enhanced traditional heritage materials, such as lime. This review recommends the advantages of bacterial encapsulated limewash and seeks to encourage ongoing investment in microbial self-repair technologies. The incorporation of microbial materials in building construction is likely to become a mainstream technology (Heveran et al., 2020).
No less important are the opportunities from this review to encourage development of alternative construction materials, such as hemp-lime bio-composites which can be protected by carbon sequestration layers. By extending the performance of microbe encapsulated limewash beyond listed buildings, developing innovative biodesigned active coatings to combat environmental pollutants and GHGs, could have global impact, environmentally and economically. The availability of genome databases and further investigation into secondary metabolic pathways will lead the way toward transformational microbial advances for environmental improvements for heritage buildings and within the wider construction industry.
Data availability statement
The original contributions presented in the study are included in the article/supplementary material, further inquiries can be directed to the corresponding author.
Author contributions
PB contributed to conception and design of the study. PB wrote the first draft of the manuscript. LJ wrote sections of the manuscript. All authors contributed to manuscript revision, read, and approved the submitted version.
Conflict of interest
The authors declare that the research was conducted in the absence of any commercial or financial relationships that could be construed as a potential conflict of interest.
Publisher’s note
All claims expressed in this article are solely those of the authors and do not necessarily represent those of their affiliated organizations, or those of the publisher, the editors and the reviewers. Any product that may be evaluated in this article, or claim that may be made by its manufacturer, is not guaranteed or endorsed by the publisher.
References
Abd El Kader, A. E., and Abu Hashish, H. M. (2020). Encapsulation techniques of food bioproduct. Egypt. J. Chem. 63 (5), 0. doi:10.21608/ejchem.2019.16269.1993
Achal, V., and Mukherjee, A. (2015). ‘A review of microbial precipitation for sustainable construction’, Construction and Building Materials. Elsevier Ltd., 1224–1235. doi:10.1016/j.conbuildmat.2015.04.051
Altermann, W., Kazmierczak, J., Oren, A., and Wright, D. T. (2006). Cyanobacterial calcification and its rock-building potential during 3.5 billion years of Earth history. Geobiology 4 (3), 147–166. doi:10.1111/j.1472-4669.2006.00076.x
Bamidele, O. P., and Emmambux, M. N. (2020). Encapsulation of bioactive compounds by “extrusion” technologies: A review. Crit. Rev. Food Sci. Nutr. 61 (18), 3100–3118. doi:10.1080/10408398.2020.1793724
Bang, S. S., Galinat, J. K., and Ramakrishnan, V. (2001). Calcite precipitation induced by polyurethane-immobilized Bacillus pasteurii. Enzyme Microb. Technol. 28 (4–5), 404–409. doi:10.1016/S0141-0229(00)00348-3
Bang, S. S., Lippert, J. J., Yerra, U., Mulukutla, S., and Ramakrishnan, V. (2010). Microbial calcite, a bio-based smart nanomaterial in concrete remediation. Int. J. Smart Nano Mater. 1 (1), 28–39. doi:10.1080/19475411003593451
Bashan, Y., Hernandez, J. P., Leyva, L., and Bacilio, M. (2002). Alginate microbeads as inoculant carriers for plant growth-promoting bacteria. Biol. Fertil. Soils 35 (5), 359–368. doi:10.1007/s00374-002-0481-5
Baumgartner, L. K., Reid, R., Dupraz, C., Decho, A., Buckley, D., Spear, J., et al. (2006). Sulfate reducing bacteria in microbial mats: Changing paradigms, new discoveries. Sediment. Geol. 185, 131–145. doi:10.1016/j.sedgeo.2005.12.008
Berger, L. R. R., Stamford, T., Stamford-Arnaud, T., de Alcantara, S., da Silva, A., da Silva, A., et al. (2014). Green conversion of agroindustrial wastes into chitin and chitosan by rhizopus arrhizus and cunninghamella elegans strains. Int. J. Mol. Sci. 15 (5), 9082–9102. doi:10.3390/ijms15059082
Bevan, R., and Woolley, T. (2008). Hemp lime construction: A guide to building with hemp lime composites. UK: IHS BRE Press. [Preprint].
Bharadwaj, P., Jankovic, L., and Carta, S. (2021). “How can UK housing projects be brought in line with net-zero carbon emission Targets?International conference held on 12-13th july 2021,” in Proceedings of pathways to resilient zero carbon cities (Hatfield, UK: University of Hertfordshire).
Billings, N., Birjiniuk, A., Samad, T. S., Doyle, P. S., and Ribbeck, K. (2015). Material properties of biofilms - a review of methods for understanding permeability and mechanics. Rep. Prog. Phys. 78 (3), 036601. doi:10.1088/0034-4885/78/3/036601
Blankendaal, T., Schuur, P., and Voordijk, H. (2014) ‘Reducing the environmental impact of concrete and asphalt: A scenario approach’, J. Clean. Prod., 66, pp. 27–36. doi:10.1016/j.jclepro.2013.10.012
Braissant, O., Decho, A. W., Dupraz, C., Glunk, C., Przekop, K. M., and Visscher, P. T. (2007). Exopolymeric substances of sulfate-reducing bacteria: Interactions with calcium at alkaline pH and implication for formation of carbonate minerals. Geobiology 5 (4), 401–411. doi:10.1111/j.1472-4669.2007.00117.x
Brimblecombe, P., Grossi, C. M., and Harris, I. (2011). “Climate change critical to cultural heritage,” in Environmental earth sciences (Springer-Verlag), 195–205. doi:10.1007/978-3-540-95991-5_20
Carran, D., Hughes, J., Leslie, A., and Kennedy, C. (2012). A short history of the use of lime as a building material beyond Europe and North America. Int. J. Archit. Herit. 6, 117–146. doi:10.1080/15583058.2010.511694
Casadesús, A., Polo, J., and Munné-Bosch, S. (2019). Hormonal effects of an enzymatically hydrolyzed animal protein-based biostimulant (pepton) in water-stressed tomato plants. Front. Plant Sci. 10, 758. doi:10.3389/fpls.2019.00758
Cassidy, M. B., Lee, H., and Trevors, J. T. (1996). Environmental applications of immobilized microbial cells: A review. J. Industrial Microbiol., 79–101. doi:10.1007/BF01570068
Castanier, S., le Métayer-Levrel, G., and Perthuisot, J. P. (1999). Ca-carbonates precipitation and limestone Genesis - the microbiogeologist point of view. Sediment. Geol. 126 (1–4), 9–23. doi:10.1016/S0037-0738(99)00028-7
Chahal, N., Rajor, A., and Sidique, R. (2011). Calcium carbonate precipitation by different bacterial strains. Afr. J. Biotechnol. 10, 8359–8372. doi:10.5897/ajb11.345
Colla, G., Hoagland, L., Ruzzi, M., Cardarelli, M., Bonini, P., Canaguier, R., et al. (2017). Biostimulant action of protein hydrolysates: Unraveling their effects on plant physiology and microbiome. Front. Plant Sci. 8, 2202. doi:10.3389/fpls.2017.02202
Couch, T., Perry, G., and Wilkes, G. (2014). Ellipta compliance building. Available at: http://www.cpwp.com/project/ellipta-compliance-building/.
da Silva, P. T., Fries, L. L. M., Menezes, C. R. d., Holkem, A. T., Schwan, C. L., Wigmann, E. F., et al. (2014). Microencapsulation: Concepts, mechanisms, methods and some applications in food technology. Cienc. Rural. 44 (7), 1304–1311. Available at:. doi:10.1590/0103-8478CR20130971
de Muynck, W., Cox, K., Belie, N. D., and Verstraete, W. (2008). Bacterial carbonate precipitation as an alternative surface treatment for concrete. Constr. Build. Mater. 22 (5), 875–885. Available at:. doi:10.1016/j.conbuildmat.2006.12.011
Decho, A. W., and Gutierrez, T. (2017). Microbial extracellular polymeric substances (EPSs) in ocean systems. Front. Microbiol. 8 (MAY), 922. Available at:. doi:10.3389/fmicb.2017.00922
Dhamecha, D., Movsas, R., Sano, U., and Menon, J. U. (2019). Applications of alginate microspheres in therapeutics delivery and cell culture: Past, present and future. Int. J. Pharm. 569, 118627. doi:10.1016/J.IJPHARM.2019.118627
Doleželová, M., Scheinherrova, L., Krejsova, J., and Vimmrova, A. (2018). Effect of high temperatures on gypsum-based composites. Constr. Build. Mater. 168, 82–90. Available at:. doi:10.1016/j.conbuildmat.2018.02.101
Dunkle, R. L., and Shasha, B. S. (1989). Response of starch-encapsulated Bacillus thuringiensis containing ultraviolet screens to sunlight. Environ. Entomol. 18 (6), 1035–1041. Available at:. doi:10.1093/ee/18.6.1035
Eberlin, C., and Jankovic, L. (2014). Proceedings of zero carbon buildings today and in the future. [Preprint].Exploring the energy performance of hemcrete in affordable housing and future implications for carbon reduction in the housing sector
Elzoghby, A. O., Elgohary, M. M., and Kamel, N. M. (2015). Implications of protein- and peptide-based nanoparticles as potential vehicles for anticancer drugs. Adv. Protein Chem. Struct. Biol. 98, 169–221. Available at:. doi:10.1016/BS.APCSB.2014.12.002
England, Historic (2019). Historic England commentary on the value of maintenance? Project Report. Available at: https://historicengland.org.uk/content/docs/caring-for-heritage/he-commentary-value-of-maintenance/.
Erşan, Y. Ç., de Belie, N., and Boon, N. (2015). Microbially induced CaCO3 precipitation through denitrification: An optimization study in minimal nutrient environment. Biochem. Eng. J. 101, 108–118. Available at:. doi:10.1016/j.bej.2015.05.006
Espie, G. S., and Kandasamy, R. A. (1992) ‘Na+-independent HCO3- transport and accumulation in the cyanobacterium synechococcus UTEX 625’, Plant Physiol., 98(2), pp. 560–568. Available at: doi:10.1104/pp.98.2.560
Estevinho, B. N., Rocha, F., Santos, L., and Alves, A. (2013). Microencapsulation with chitosan by spray drying for industry applications – a review. Trends Food Sci. Technol. 31 (2), 138–155. Available at:. doi:10.1016/J.TIFS.2013.04.001
Fangquiang, S., Zhang, K., Zhang, H., and Zhang, B. (2015). A study of traditional blood lime mortar for restoration of ancient buildings. Cem. Concr. Res. 76, 232–241. Available at:. doi:10.1016/j.cemconres.2015.06.006
Fidanza, M. R., and Caneva, G. (2019). Natural biocides for the conservation of stone cultural heritage: A review. Journal of cultural heritage. Elsevier Masson, 271–286. Available at:. doi:10.1016/j.culher.2019.01.005
Galazzo, J. L., and Bailey, J. E. (1990). Growing Saccharomyces cerevisiae in calcium‐alginate beads induces cell alterations which accelerate glucose conversion to ethanol. Biotechnol. Bioeng. 36 (4), 417–426. Available at:. doi:10.1002/bit.260360413
Gao, D., Sun, Y., Fong, A. M., and Gu, X. (2022). Mineral-based form-stable phase change materials for thermal energy storage: A state-of-the art review. Energy Storage Mater. 46, 100–128. doi:10.1016/j.ensm.2022.01.003
Ghosh, P., Mandal, S., Chattopadhyay, B., and Pal, S. (2005). Use of microorganism to improve the strength of cement mortar. Cem. Concr. Res. 35 (10), 1980. Available at:. doi:10.1016/j.cemconres.2005.03.005
González-Muñoz, M. T., Rodriguez-Navarro, C., Martinez-Ruiz, F., Arias, J. M., Merroun, M. L., and Rodriguez-Gallego, M. (2010). Bacterial biomineralization: New insights from Myxococcus-induced mineral precipitation. Geol. Soc. Spec. Publ. 336 (1), 31–50. Available at:. doi:10.1144/SP336.3
Grand View Research (2017). Biologics market size forecast, industry growth report 2018-2025. Available at: https://www.grandviewresearch.com/industry-analysis/biologics-market (Accessed: March 22, 2021).
Griño, A. A., Daly, M. K. M., and Ongpeng, J. M. C. (2020). Bio-influenced self-healing mechanism in concrete and its testing: A review. Switzerland: Applied Sciences (Switzerland)MDPI AG, 5161. Available at:. doi:10.3390/app10155161
Guo, J., Li, P., Kong, L., and Xu, B. (2020). Microencapsulation of curcumin by spray drying and freeze drying. LWT 132, 109892. Available at:. doi:10.1016/J.LWT.2020.109892
Gupta, S., Kua, H. W., and Tan Cynthia, S. Y. (2017). Use of biochar-coated polypropylene fibers for carbon sequestration and physical improvement of mortar. Cem. Concr. Compos. 83, 171–187. Available at:. doi:10.1016/J.CEMCONCOMP.2017.07.012
Hamot, L., and George, C. B. (2021). Heart of the matter: Calculating embodied carbon using TM65 – CIBSE journal. ICIBSE J. Available at: https://www.cibsejournal.com/technical/heart-of-the-matter-calculating-embodied-energy-using-tm65/(Accessed: April 6, 2021).
Heveran, C. M., Williams, S. L., Qiu, J., Artier, J., Hubler, M. H., Cook, S. M., et al. (2020). Biomineralization and successive regeneration of engineered living building materials. Matter 2 (2), 481–494. Available at:. doi:10.1016/j.matt.2019.11.016
Hm Government, (1997). Planning (listed buildings and conservation areas) Act 1997. Available at: http://www.legislation.gov.uk/ukpga/1990/9/pdfs/ukpga_19900009_en.pdf (Accessed December 6, 2019).
Hulst, A. C., Tramper, J., van't Riet, K., and Westerbeek, J. M. M. (1985). A new technique for the production of immobilized biocatalyst in large quantities. Biotechnol. Bioeng. 27 (6), 870–876. Available at:. doi:10.1002/bit.260270617
Jadhav, U. U., Lahoti, M., Chen, Z., Qiu, J., Cao, B., and Yang, E. H. (2018). Viability of bacterial spores and crack healing in bacteria-containing geopolymer. Constr. Build. Mater. 169, 716–723. Available at:. doi:10.1016/j.conbuildmat.2018.03.039
Jampílek, J., and Králová, K. (2017). “Nanomaterials for delivery of nutrients and growth-promoting compounds to plants,” in Nanotechnology: An agricultural paradigm (Springer Singapore), 177–226. Available at:. doi:10.1007/978-981-10-4573-8_9
Jankowski, T., Zielinska, M., and Wysakowska, A. (1997). Encapsulation of lactic acid bacteria with alginate/starch capsules. Biotechnol. Tech. 11 (1), 31–34. Available at:. doi:10.1007/BF02764447
Jonkers, H. M. (2007). “Self-healing concrete: A biological approach,” in Springer series in materials science (Springer-Verlag), 195–204. Available at:. doi:10.1007/978-1-4020-6250-6_9
Joseph, C., Jefferson, A., Isaacs, B., Lark, R., and Gardner, D. (2010). Experimental investigation of adhesive-based self-healing of cementitious materials. Mag. Concr. Res. 62 (11), 831–843. Available at:. doi:10.1680/macr.2010.62.11.831
Jroundi, F., Fernandez-Vivas, A., Rodriguez-Navarro, C., Bedmar, E. J., and Gonzalez-Munoz, M. T. (2010). Bioconservation of deteriorated monumental calcarenite stone and identification of bacteria with carbonatogenic activity. Microb. Ecol. 60 (1), 39–54. Available at:. doi:10.1007/s00248-010-9665-y
Kavazanjian, E., and Karatas, I. (2008). ‘Microbiological improvement of the physical properties of soil’, international Conference on case Histories in geotechnical engineering, 1–10. Available at: https://search.proquest.com/openview/e911bbd4881768c073e54a82df893526/1?pq-origsite=gscholar&cbl=18750&diss=y (Accessed: April 9, 2021).
Khaliq, W., and Ehsan, M. B. (2016). Crack healing in concrete using various bio influenced self-healing techniques. Constr. Build. Mater. 102, 349–357. Available at:. doi:10.1016/j.conbuildmat.2015.11.006
Khotimchenko, M. (2020). Pectin polymers for colon-targeted antitumor drug delivery. Int. J. Biol. Macromol. 158, 1110–1124. Available at:. doi:10.1016/J.IJBIOMAC.2020.05.002
Kim, Y. J., Park, H. G., Yang, Y. L., Yoon, Y., Kim, S., and Oh, E. (2005). Multifunctional drug delivery system using starch-alginate beads for controlled release. Biol. Pharm. Bull. 28 (2), 394–397. Available at:. doi:10.1248/bpb.28.394
Kumar, K., Dasgupta, C., Nayak, B., Lindblad, P., Das, D., et al. (2011). Development of suitable photobioreactors for CO2 sequestration addressing global warming using green algae and cyanobacteria. Bioresource Technology 102 (8), 4945–4953. doi:10.1016/j.biortech.2011.01.054
Kumar, M., Sundaram, S., Gnansounou, E., Larroche, C., and Thakur, I. S. (2018) ‘Carbon dioxide capture, storage and production of biofuel and biomaterials by bacteria: A review’, Bioresour. Technol., 247, pp. 1059–1068. Available at: doi:10.1016/j.biortech.2017.09.050
Lancy, E. D., and Tuovinen, O. H. (1984). Ferrous ion oxidation by Thiobacillus ferrooxidans immobilized in calcium alginate. Appl. Microbiol. Biotechnol. 20 (2), 94–99. Available at:. doi:10.1007/BF00252584
Lee, Y.-N. (2003). Calcite production by Bacillus amyloliquefaciens CMB01. J. Microbiol. 41 (4), 345–348.
Leskard, M. (2022). A sustainable storage solution for the Science Museum Group. Sci. Mus. Group J. 4 (4). Available at:. doi:10.15180/150405
Léveillé, R. J., Longstaffe, F. J., and Fyfe, W. S. (2007). An isotopic and geochemical study of carbonate-clay mineralization in basaltic caves: Abiotic versus microbial processes. Geobiology 5 (3), 235–249. Available at:. doi:10.1111/j.1472-4669.2007.00109.x
Li, L., Zheng, Q., Li, Z., Ashour, A., Han, B., et al. (2019). ‘Bacterial technology-enabled cementitious composites: A review’, composite structures. Elsevier. 111170, Available at:. doi:10.1016/j.jbiotec.2018.04.019
Liffourrena, A. S., and Lucchesi, G. I. (2018). Alginate-perlite encapsulated Pseudomonas putida A (ATCC 12633) cells: Preparation, characterization and potential use as plant inoculants. J. Biotechnol. 278, 28–33. Available at:. doi:10.1016/j.jbiotec.2018.04.019
Liu, Hongxia, Zhao, X., Guo, M., and Zheng, Z. (2015). Growth and metabolism of Beauveria bassiana spores and mycelia. BMC Microbiol. 15, 267. doi:10.1186/s12866-015-0592-4
Liu, H., Xie, M., and Nie, S. (2020). Recent trends and applications of polysaccharides for microencapsulation of probiotics. Food Front. 1 (1), 45–59. Available at:. doi:10.1002/fft2.11
Luo, M., Qian, C. X., and Li, R. Y. (2015). Factors affecting crack repairing capacity of bacteria-based self-healing concrete. Constr. Build. Mater. 87, 1–7. Available at:. doi:10.1016/J.CONBUILDMAT.2015.03.117
Malhotra, I., and Basir, S. F. (2020). Immobilization of invertase in calcium alginate and calcium alginate-kappa-carrageenan beads and its application in bioethanol production. Prep. Biochem. Biotechnol. 50 (5), 494–503. Available at:. doi:10.1080/10826068.201910.1080/10826068.2019.1709979
Megharaj, M., and Naidu, R. (2017). ‘Soil and brownfield bioremediation’, microbial biotechnology. John Wiley & Sons, 1244–1249. Available at:. doi:10.1111/1751-7915.12840
Milián, Y. E., Gutierrez, A., Grageda, M., and Ushak, S. (2017). A review on encapsulation techniques for inorganic phase change materials and the influence on their thermophysical properties. Renew. Sustain. Energy Rev. 73, 983–999. Available at:. doi:10.1016/j.rser.2017.01.159
Moradali, M. F., and Rehm, B. H. A. (2020). Bacterial biopolymers: From pathogenesis to advanced materials. Nat. Rev. Microbiol., 195–210. Available at:. doi:10.1038/s41579-019-0313-3
Morgan, C. A., Herman, N., White, P. A., Vesey, G., et al. (2006). Preservation of micro-organisms by drying; A review. J. Microbiol. Methods, 183–193. Available at:. doi:10.1016/j.mimet.2006.02.017
Musgrave, S. C., Kerby, N. W., Codd, G. A., and Stewart, W. D. P. (1983). Structural features of calcium alginate entrapped cyanobacteria modified for ammonia production. Eur. J. Appl. Microbiol. Biotechnol. 17 (2), 133–136. Available at:. doi:10.1007/BF00499865
Muxika, A., Etxabide, A., Uranga, J., Gujerrero, P., de la Caba, K., et al. (2017). Chitosan as a bioactive polymer: Processing, properties and applications. International journal of biological macromolecules. Elsevier, 1358–1368. Available at:. doi:10.1016/j.ijbiomac.2017.07.087
Nah, J. W., and Jeong, G. W. (2021). Preparation and encapsulation techniques of chitosan microsphere for enhanced bioavailability of natural antioxidants. Carbohydr. Res. 500, 108218. Available at:. doi:10.1016/j.carres.2020.108218
Nealson, K. H. (2017). Bioelectricity (electromicrobiology) and sustainability. Microb. Biotechnol. 10 (5), 1114–1119. Available at:. doi:10.1111/1751-7915.12834
Nesterenko, A., Alric, I., Silvestre, F., Durrieu, V., et al. (2013). Vegetable proteins in microencapsulation: A review of recent interventions and their effectiveness’, industrial crops and products. Elsevier 42, 469–479. Available at:. doi:10.1016/j.indcrop.2012.06.035
Ogbonna, J. C., Matsumura, M., Yamagata, T., Sakuma, H., and Kataoka, H. (1989). Production of micro-gel beads by a rotating disk atomizer. J. Ferment. Bioeng. 68 (1), 40–48. Available at:. doi:10.1016/0922-338X(89)90212-2
Oyen, M. L. (2014). Mechanical characterisation of hydrogel materials. Int. Mater. Rev. 59 (1), 44–59. Available at:. doi:10.1179/1743280413Y.0000000022
Pachta, V., Triantafyllaki, S., and Stefanidou, M. (2018). Performance of lime-based mortars at elevated temperatures. Constr. Build. Mater. 189, 576–584. Available at:. doi:10.1016/j.conbuildmat.2018.09.027
Perrechil, F. A., Maximo, G. J., Sato, A. C. K., and Cunha, R. L. (2020). Microbeads of sodium caseinate and κ-carrageenan as a β-carotene carrier in aqueous systems. Food bioproc. Tech. 13 (44), 661–669. Available at:. doi:10.1007/S11947-020-02426-9
Płaza, G., and Achal, V. (2020). Biosurfactants: Eco-friendly and innovative biocides against biocorrosion. Int. J. Mol. Sci. 21, 2152. Available at:. doi:10.3390/ijms21062152
Poncelet, D., Neufeld, R., Bugarski, B., Amsden, B. G., Zhu, J., and Goosen, M. F. A. (1994). A Parallel plate electrostatic droplet generator: Parameters affecting microbead size. Appl. Microbiol. Biotechnol. 42 (2–3), 251–255. Available at:. doi:10.1007/BF00902725
Poornima, K., and Sinthya, R. (2017). Application of various encapsulation techniques in food industries. IJLERA: International Journal of Latest Engineering Research and Applications.
Power, B., Liu, X., Germaine, K., Ryan, D., Brazil, D., and Dowling, D. (2011). Alginate beads as a storage, delivery and containment system for genetically modified PCB degrader and PCB biosensor derivatives of Pseudomonas fluorescens F113. J. Appl. Microbiol. 110 (5), 1351–1358. Available at:. doi:10.1111/j.1365-2672.2011.04993.x
Prasad, R., Bhattacharyya, A., and Nguyen, Q. D. (2017). Nanotechnology in sustainable agriculture: Recent developments, challenges, and perspectives. Front. Microbiol., 1014. Available at:. doi:10.3389/fmicb.2017.01014
Qi, X., and Tester, R. F. (2019). Starch granules as active guest molecules or microorganism delivery systems. Food Chem. 271, 182–186. Available at:. doi:10.1016/J.FOODCHEM.2018.07.177
Ramanan, R., Kannan, K., Deshkar, A., Yadav, R., and Chakrabarti, T. (2010). Enhanced algal CO2 sequestration through calcite deposition by Chlorella sp. and Spirulina platensis in a mini-raceway pond. Bioresour. Technol. 101 (8), 2616–2622. Available at:. doi:10.1016/j.biortech.2009.10.061
Reeburgh, W. S. (2007). Oceanic methane biogeochemistry’, chemical reviews. Am. Chem. Soc. 107, 486–513. Available at:. doi:10.1021/cr050362v
Rekha, P. D., Lai, W. A., Arun, A., and Young, C. C. (2007). Effect of free and encapsulated Pseudomonas putida CC-FR2-4 and Bacillus subtilis CC-pg104 on plant growth under gnotobiotic conditions. Bioresour. Technol. 98 (2), 447–451. Available at:. doi:10.1016/j.biortech.2006.01.009
RmetS, (2022). UK climate continues to change in 2021. State U. K. Clim. 42 (1), 1–80. doi:10.1002/joc.7787
Rodriguez-Navarro, C., Rodriguez-Gallego, M., Ben Chekroun, K., and Gonzalez-Muñoz, M. T. (2003). Conservation of ornamental stone by Myxococcus xanthus-induced carbonate biomineralization. Appl. Environ. Microbiol. 69 (4), 2182–2193. Available at:. doi:10.1128/AEM.69.4.2182-2193.2003
Rossi, F., Olguin, E. J., Diels, L., and De Philippis, R. (2015). Microbial fixation of CO2 in water bodies and in drylands to combat climate change, soil loss and desertification. New Biotechnol. 32 (1), 109–120. Available at:. doi:10.1016/j.nbt.2013.12.002
Rubio Camacho, F., Sevilla, J. M. F., Chisti, Y., and Grima, E. M. (2003). A mechanistic model of photosynthesis in microalgae. Biotechnol. Bioeng. 81 (4), 459–473. Available at:. doi:10.1002/bit.10492
Sabbioni, C., Brimblecombe, P., and Lefevre, R. A. (2008). European and mediterranean major hazards agreement (EUR-OPA) vulnerability of cultural heritage to climate change. Available at: https://www.coe.int/t/dg4/majorhazards/activites/2009/Ravello15-16may09/Ravello_APCAT2008_44_Sabbioni-Jan09_EN.pdf (Accessed: September 11, 2019).
San Keskin, N. O., Celebioglu, A., Sarioglu, O. F., Uyar, T., and Tekinay, T. (2018). Encapsulation of living bacteria in electrospun cyclodextrin ultrathin fibers for bioremediation of heavy metals and reactive dye from wastewater. Colloids Surfaces B Biointerfaces 161, 169–176. Available at:. doi:10.1016/J.COLSURFB.2017.10.047
Sandalci, I., Tezer, M. M., and Basaran Bundur, Z. (2021). Immobilization of bacterial cells on natural minerals for self-healing cement-based materials. Front. Built Environ. 7, 46. Available at:. doi:10.3389/fbuil.2021.655935
Sarıyer, S., Duranoglu, D., Dogan, O., and Kucuk, I. (2020). pH-responsive double network alginate/kappa-carrageenan hydrogel beads for controlled protein release: Effect of pH and crosslinking agent. J. Drug Deliv. Sci. Technol. 56, 101551. Available at:. doi:10.1016/J.JDDST.2020.101551
Sarma, S. J., Pakshirajan, K., and Mahanty, B. (2011). Chitosan-coated alginate–polyvinyl alcohol beads for encapsulation of silicone oil containing pyrene: A novel method for biodegradation of polycyclic aromatic hydrocarbons. J. Chem. Technol. Biotechnol. 86 (2), 266–272. Available at:. doi:10.1002/JCTB.2513
Schoebitz, M., López, M. D., and Roldán, A. (2013). Bioencapsulation of microbial inoculants for better soil–plant fertilization. A review. Agron. Sustain. Dev. 3333 (44), 751–765. Available at:. doi:10.1007/S13593-013-0142-0
Scrivener, K. L., John, V. M., and Gartner, E. M. (2018). Eco-efficient cements: Potential economically viable solutions for a low-CO2 cement-based materials industry. Cem. Concr. Res. 114, 2–26. Available at:. doi:10.1016/j.cemconres.2018.03.015
Seifan, M., Sarmah, A. K., Samani, A. K., Ebrahiminezhad, A., Ghasemi, Y., and Berenjian, A. (2018). Mechanical properties of bio self-healing concrete containing immobilized bacteria with iron oxide nanoparticles. Appl. Microbiol. Biotechnol. 102 (10), 4489–4498. Available at:. doi:10.1007/s00253-018-8913-9
Shaheen, N., Khushnood, R. A., and Ud Din, S. (2018). Bioimmobilized limestone powder for autonomous healing of cementitious systems: A feasibility study. Adv. Mater. Sci. Eng. 2018, 1–9. doi:10.1155/2018/7049121
Singh, H., and Gupta, R. (2020). Cellulose fiber as bacteria-carrier in mortar: Self-healing quantification using UPV. J. Build. Eng. 28, 101090. Available at:. doi:10.1016/J.JOBE.2019.101090
Souradeep, G., and Kua, H. W. (2016). Encapsulation technology and techniques in self-healing concrete. J. Mat. Civ. Eng. 28 (12), 04016165. Available at:. doi:10.1061/(asce)mt.1943-5533.0001687
Soysal, A., Milla, J., King, G. M., Hassan, M., and Rupnow, T. (2020). Evaluating the self-healing efficiency of hydrogel-encapsulated bacteria in concrete. Transp. Res. Rec. 2674 (6), 113–123. Available at:. doi:10.1177/0361198120917973
Sri Durga, C. S., Ruben, N., Sri Rama Chand, M., Indira, M., and Venkatesh, C. (2021). Comprehensive microbiological studies on screening bacteria for self-healing concrete. Materialia 15, 101051. Available at:. doi:10.1016/j.mtla.2021.101051
Stocks-Fischer, S., Galinat, J. K., and Bang, S. S. (1999). Microbiological precipitation of CaCO3. Soil Biol. Biochem. 31 (11), 1563–1571. Available at:. doi:10.1016/S0038-0717(99)00082-6
Stormo, K. E., and Crawford, R. L. (1992). Preparation of encapsulated microbial cells for environmental applications. Appl. Environ. Microbiol. 58, 727–730. Available at:. doi:10.1128/aem.58.2.727-730.1992
Su, Y., Qian, C., Rui, Y., and Feng, J. (2021). Exploring the coupled mechanism of fibers and bacteria on self-healing concrete from bacterial extracellular polymeric substances (EPS). Cem. Concr. Compos. 116, 103896. Available at:. doi:10.1016/j.cemconcomp.2020.103896
Taliaferro, S. (2015). Documentation and testing of nineteenth-century LImewash recipes in the United States. Columbia: Columbia University.
Tuffen, H. (2007). Models of ice melting and edifice growth at the onset of subglacial basaltic eruptions. J. Geophys. Res. 112 (3), B03203. Available at:. doi:10.1029/2006JB004523
Uk Government, (2008). The climate change Act 2008 (2020 target, credit limit and definitions) order 2009. Available at: https://www.legislation.gov.uk/ukdsi/2009/9780111478523/contents (Accessed: April 10, 2021).
Valdivia-Rivera, S., Ayora-Talavera, T., Lizardi-Jimenez, M. A., Garcia-Cruz, U., Cuevas-Bernardino, J. C., and Pacheco, N. (2021). Encapsulation of microorganisms for bioremediation: Techniques and carriers. Rev. Environ. Sci. Biotechnol. 20, 815–838. Available at:. doi:10.1007/s11157-021-09577-x
Vejan, P., Abdullah, R., Khadiran, T., and Ismail, S. (2019). Encapsulation of Bacillus salmalaya 139SI using double coating biopolymer technique. Lett. Appl. Microbiol. 68 (1), 56–63. Available at:. doi:10.1111/lam.13088
Viles, H. A. (2002). Implications of future climate change for stone deterioration. Geol. Soc. Spec. Publ. 205, 407–418. Available at:. doi:10.1144/GSL.SP.2002.205.01.29
Viveganandan, G., and Jauhri, K. S. (2000). Growth and survival of phosphate-solubilizing bacteria in calcium alginate. Microbiol. Res. 155 (3), 205–207. Available at:. doi:10.1016/S0944-5013(00)80033-6
Voo, W. P., Ooi, C. W., Islam, A., Tey, B. T., and Chan, E. S. (2016). Calcium alginate hydrogel beads with high stiffness and extended dissolution behaviour. Eur. Polym. J. 75, 343–353. Available at:. doi:10.1016/j.eurpolymj.2015.12.029
Wang, J., Van Tittelboom, K., De Belie, N., and Verstraete, W. (2012). Use of silica gel or polyurethane immobilized bacteria for self-healing concrete. Constr. Build. Mater. 26 (1), 532–540. Available at:. doi:10.1016/j.conbuildmat.2011.06.054
Wang, J. Y., Snoeck, D., Van Vlierberghe, S., Verstraete, W., and De Belie, N. (2014). Application of hydrogel encapsulated carbonate precipitating bacteria for approaching a realistic self-healing in concrete. Constr. Build. Mater. 68, 110–119. Available at:. doi:10.1016/j.conbuildmat.2014.06.018
Wang, J. Y., Soens, H., Verstraete, W., and De Belie, N. (2014). Self-healing concrete by use of microencapsulated bacterial spores. Cem. Concr. Res. 56, 139–152. Available at:. doi:10.1016/J.CEMCONRES.2013.11.009
Wei, S., Cui, H., Jiang, Z., Liu, H., He, H., and Fang, N. (2015). Biomineralization processes of calcite induced by bacteria isolated from marine sediments. Braz. J. Microbiol. 46 (2), 455–464. Available at:. doi:10.1590/S1517-838246220140533
Wiktor, V., and Jonkers, H. M. (2011). Quantification of crack-healing in novel bacteria-based self-healing concrete. Cem. Concr. Compos. 33 (7), 763–770. Available at:. doi:10.1016/j.cemconcomp.2011.03.012
Yang, J. S., Mu, T. H., and Ma, M. M. (2018). Extraction, structure, and emulsifying properties of pectin from potato pulp. Food Chem. 244, 197–205. Available at:. doi:10.1016/j.foodchem.2017.10.059
Young, R. (2008). “Lime-based plasters, renders and washes,” in Materials & skills for historic building conservation. Editor M. Forsyth (Chichester, UK: Blackwell Publishing Ltd), 56–91.
Yu, W. K., Yim, T. B., Lee, K. Y., and Heo, T. R. (2001). Effect of skim milk-alginate beads on survival rate of bifidobacteria. Biotechnol. Bioprocess Eng. 6 (2), 133–138. Available at:. doi:10.1007/BF02931959
Zhu, T., and Dittrich, M. (2016). Carbonate precipitation through microbial activities in natural environment, and their potential in biotechnology: A review. Frontiers in bioengineering and biotechnology, 4. doi:10.3389/fbioe.2016.00004
Keywords: carbon-fixing, limewash, hemp-lime, heritage, conservation, encapsulation
Citation: Booth P and Jankovic L (2022) Novel biodesign enhancements to at-risk traditional building materials. Front. Built Environ. 8:766652. doi: 10.3389/fbuil.2022.766652
Received: 16 November 2021; Accepted: 15 September 2022;
Published: 03 October 2022.
Edited by:
Prannoy Suraneni, University of Miami, United StatesReviewed by:
Neven Ukrainczyk, Darmstadt University of Technology, GermanySivakumar Ramanathan, Oregon State University, United States
Copyright © 2022 Booth and Jankovic. This is an open-access article distributed under the terms of the Creative Commons Attribution License (CC BY). The use, distribution or reproduction in other forums is permitted, provided the original author(s) and the copyright owner(s) are credited and that the original publication in this journal is cited, in accordance with accepted academic practice. No use, distribution or reproduction is permitted which does not comply with these terms.
*Correspondence: Peter Booth, cGIxOWFidUBoZXJ0cy5hYy51aw==