- 1School of Civil and Construction Engineering, Oregon State University, Corvallis, OR, United States
- 2Caribbean Green Technology Center, University of the Virgin Islands, St. Thomas, United States Virgin Islands
- 3Applied Technology and Management/Geosyntec Consultants, Inc, West Palm Beach, FL, United States
- 4Department of Naval Architecture and Ocean Engineering, United States Naval Academy, Annapolis, MD, United States
Natural and Nature-Based Features (NNBF) are promoted as alternatives to structural flood protection measures. Progress has been made in understanding the physics and engineering of these systems; however, engineering, ecological, and social barriers to implementation remain. This paper identifies these barriers using the results of a literature review and summary of expert opinion; contrasts the state of the practice of NNBF with traditional structures; and details the main engineering challenges to NNBF implementation, including the uncertainty in current calculation techniques and lack of engineering design guidelines. We suggest that emergent vegetation systems can be designed with the current body of information, and an example framework is proposed for assessing these systems for their wave attenuation performance. The framework is discussed in the context of risk, and future research priorities are presented.
Introduction
Over the last few decades, ecosystems have been promoted as viable alternatives to conventional (structural or gray) coastal protection structures (Arkema et al., 2013; Silver et al., 2019). Several studies have demonstrated the protective and restorative values of wetlands, reefs, seagrass beds, and/or vegetated dunes (Scyphers et al., 2011; Anderson and Smith, 2014; Ozeren et al., 2014; Taylor et al., 2015; Guannel et al., 2016; Narayan et al., 2016; Chang et al., 2019; Lei and Nepf, 2019; Maza et al., 2019; Tomiczek et al., 2020a, 2020b, 2022; Elko et al., 2021; Kelty et al., 2022). These types of solutions for shoreline protection are termed “Natural and Nature-Based Features (NNBF),” and are landscape features that are used to provide engineering functions, while producing additional economic, environmental, and/or social benefits. There are many definitions, but the common element among all of these definitions is the focus on conserving, restoring, and engineering natural systems for the benefit of people and the ecosystems they inhabit (Bridges et al., 2021). NNBF for flood and erosion protection include natural features such as emergent vegetation, beaches and dunes, reefs, or islands, and nature-based features (i.e., engineered ecosystems that mimic characteristics of natural features) such as constructed wetlands, nourished beaches, and artificial reefs (Bridges et al., 2015). These systems have also been referred to as “Nature-Based Solutions (NbS),” “Natural Infrastructure,” or “Green Infrastructure,” among other terms (Bridges et al., 2021). NNBF solutions are attractive because they have the potential to provide ecological, social, and economic benefits in addition to shoreline protection services (Barbier et al., 2011; Arkema et al., 2015; Ruckelshaus et al., 2016), and are often viewed as a “win-win” approach to coastal engineering (Hochard et al., 2019; Menéndez et al., 2020; Cunha et al., 2021; Feagin et al., 2021). As a result, several major initiatives by U.S. government agencies (Bridges et al., 2015, 2021; Webb et al., 2019), non-profit groups (Sarasota Bay Estuary Program, 2018; Narayan et al., 2019), and international organizations (PIANC, 2018; Browder et al., 2019; UNDRR, 2020; Castellari et al., 2021; Science for Environment Policy, 2021), have focused on leveraging NNBF as resilient adaptation alternatives for shoreline protection.
One type of natural habitat widely discussed by practitioners and in the literature is emergent vegetation, which includes mangroves (e.g., Rhizophora sp., Avicennia sp., Laguncularia racemosa) and marsh vegetation such as grasses, rushes, or reeds (e.g., Spartina sp., Juncus sp., Phragmites sp.). Among other benefits, these intertidal ecosystems have been noted for their wave and storm surge attenuation capabilities (Mazda et al., 1997; McIvor et al., 2012; Zhang et al., 2012; Montgomery et al., 2019; Chen et al., 2021), carbon sequestration (Alongi, 2008; Sanderman et al., 2018), habitat services for native fauna (Odum et al., 1982; USFWS, 1999), and cultural and recreational values (Uddin et al., 2013; Spalding and Parrett, 2019). However, the quantification and prediction of engineering performance (e.g., wave height attenuation) of emergent vegetation to inform design lags behind the quantification of hydraulic responses for conventional engineering systems. Indeed, practicing engineers may be hesitant to design NNBF due to the lack of design standards and differences with the traditional design process for gray infrastructure. The coastal engineering practice, as well as civil engineering in general, is guided by established manuals of practice, design standards, and guidance documents (e.g., USACE, 2002; FEMA, 2011; ASCE, 2014, 2022; Bridges et al., 2021). Although recent efforts have made strides in developing general guidelines for NNBF at international, national, state, and local levels (e.g., Miller et al., 2015; World Bank, 2017; Webb et al., 2019; Bridges et al., 2021), current guidance documents do not provide the NNBF equivalent of the comprehensive calculation methodologies common in traditional engineering design manuals (Bridges et al., 2021). Moreover, compared with conventional systems, NNBF have unique concerns, because their performance may be affected by biological factors and physical events. For example, although scientists have found evidence of engineering benefits provided by emergent vegetation under specific circumstances (e.g., McIvor et al., 2012), few conclusions are applicable for storm conditions (Pinsky et al., 2013), and only recently has research been focused on storm performance (e.g., Vuik et al., 2016; Kelty et al., 2022). Therefore, it is important that guidance on coastal protection benefits be provided, clearly identifying the range of applicability of expected benefits.
Even with recent advancements in knowledge about utilizing emergent vegetation for coastal risk mitigation in hydraulics or engineering models, barriers to implementation remain (e.g., Close et al., 2017; Cherry et al., 2018). These barriers exist throughout the implementation process as identified by Bridges et al. (2021), with challenges noted for technical design, socioeconomic considerations, financing, permitting, construction, and maintenance (Close et al., 2017; Cherry et al., 2018; Zuniga-Teran et al., 2020; King et al., 2021). A broad set of conditions needs to be addressed to facilitate and promote the appropriate use of NNBF for coastal protection, which requires not only coastal engineers, but also experts in other disciplines in engineering, ecology, and social science.
To improve our abilities to predict the engineering performance of NNBF, there is a need to 1) develop technical recommendations on how to incorporate NNBF as part of coastal hazard mitigation solutions; 2) quantify wave attenuation performance; and 3) establish prescriptive standards for design, construction, and monitoring of projects to create, restore, or enhance NNBF systems.
This paper addresses the various issues raised above by summarizing the state of the practice and providing practical guidance for the design of NNBF, based on recent advances in the quantification of wave attenuation by emergent vegetation. We also describe the engineering, ecological, and social conditions that influence the use of these systems for coastal protection. Based on these considerations, we propose a conceptual engineering framework for evaluating existing natural systems or designing new NNBF or hybrid systems, and make recommendations in the engineering, ecological, and social dimensions to facilitate and promote the appropriate use of these systems.
State of the practice
Expert opinion
To gain a deeper insight into the use of emergent vegetation in engineering design, implementation, and construction, we asked practitioners in different fields working with NNBF projects to comment on the state of the practice by answering the following questions:
1) In your experience, what is the current level of understanding regarding the performance of emergent vegetation in coastal protection applications? Is available information applied adequately for analysis of alternatives and for design?
2) What additional progress from a scientific, engineering, or design standpoint is needed to encourage adequate consideration and better implementation of these types of nature-based solutions?
3) What steps from a policy or regulatory standpoint could be taken to encourage adequate consideration and better implementation of these solutions?
4) Please share any other thoughts/comments/concerns about the present status and future needs regarding the use of nature-based solutions (especially emergent vegetation) for coastal hazards mitigation and climate adaptation.
A total of 32 professionals responded, representing academia (6), consulting (9), government (13), and nonprofit organizations (4), resulting in a variety of perspectives and experience on emergent vegetation projects. The responses primarily inform on the existing knowledge in the field and progress needed for NNBF strategies to be more widely adopted. Responses were organized according to two broad themes: 1) current knowledge and 2) future needs, and within these themes, responses were separated into engineering, ecology, and social categories.
While many respondents recognize that there is ample information supporting NNBF performance, designers are unable to apply it in a quantitative way needed for design (19/32). Two consultants and one government professional note that the information requires expertise to understand, making it difficult to access (3/32). Furthermore, experience from case studies is highly location-specific and unable to be extrapolated (5/32). Rather than designed solutions, many NNBF projects are structured as vulnerability studies (1/32). Physical space is also a concern, as there may not be enough space for emergent vegetation in urban environments or on steep and narrow banks (2/32). From a socioeconomic perspective, respondents identify the current regulatory framework as inadequate for NNBF, with many suggesting changes to policies and permitting (21/32). Stakeholders may advocate against or be reluctant to implement NNBF projects due to loss of space and view, attraction of mosquitos, and maintenance considerations (5/32). There are concerns about a perception among some stakeholders that vegetation can always be used as a solution, even though many situations call for another strategy or multi-tiered solution (2/32). Responses from academia, government, and consulting describe a disconnect between the engineering, architecture, and environmental disciplines, with some noting that engineers tend to ignore NNBF solutions, while architects and environmental professionals tend to overestimate the protection that vegetation can provide and do not understand the need for quantitative design guidelines for engineers (4/32).
Responses identify future engineering needs; a summary of broad categories is shown in Figure 1. The most frequently cited need is the further development of engineering design standards (19/32), an observation that agrees with previous studies (Cherry et al., 2018; Zuniga-Teran et al., 2020). Some responses describe specific criteria that would be needed in engineering design guidelines: 1) coastal geomorphology considerations, including sediment; 2) nearshore conditions, such as bathymetry, tidal range, and wave conditions; 3) vegetation considerations, such as species, age, number of plants, density, height, and width of patch, and under what environmental conditions and in what locations they would be able to thrive; 4) contrasting design considerations for gray, green, and hybrid systems; 5) maintenance requirements, including best management practices; 6) performance over the design life, including recovery time between storms and changes in protection as vegetation ages; and 7) survivability, especially in the face of climate and environmental changes such as water quality, salinity, diseases, and extreme weather events. These criteria should be predictive and could consider navigation.
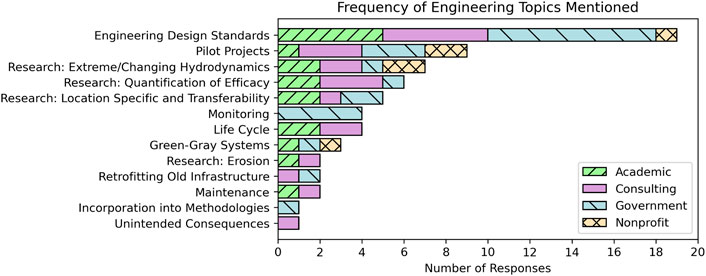
FIGURE 1. Frequency of categories of future engineering needs for emergent vegetation implementation cited by experts in survey responses (N=32).
Additional engineering challenges remain beyond the development of comprehensive engineering design guidelines. For example, a broader consensus is required on how to incorporate emergent vegetation into varied methodologies of calculating wave runup and total water level (1/32). Government respondents identify a need for monitoring criteria to show success, such as 1) metrics for reporting vegetation density, areal extent, and root structure of plants; and 2) guidelines for duration and spatial resolution of the monitoring program (4/32). There is also a need for additional pilot projects in all types of locations that measure efficacy and detail designs (9/32). Similarly, research should include field experiments especially in locations where NNBF projects may be implemented, such as tropical environments, and develop a greater understanding of the applicability of solutions from one location to another (5/32). This response echoes the need to understand transferability of NNBF results between locations (Close et al., 2017). Future research should also characterize the performance of emergent vegetation under extreme events, higher tidal ranges, and future relative sea level rise scenarios (7/32), and provide a better quantification of erosive processes (2/32). Finally, respondents found that experiments are needed to further quantify and prove efficacy, including the translation of results from flume experiments to field parameters (6/32).
Many responses mention the necessity of ecological research advances for successful implementation of NNBF (6/32), including studies that assess connectivity, comprise a wide range of habitat types and environmental conditions, monitor interaction with substrate and changes over the project’s life cycle, and characterize the impacts of invasive species. In order to obtain the best performance of a restored or hybrid system, projects should imitate the ecology of nearby natural systems (4/32). This observation is consistent with the findings of Waryszak et al. (2021), who determined that the designers of most successful hybrid projects have a strong knowledge of site hydrological and ecological history. Furthermore, vegetation may be optimized based on the carbon cycle (3/32). Materials scientists and engineers can be brought into interdisciplinary teams for design (1/32). The engineering and ecology responses are highly related, with species considerations, performance over design life, maturation, and survivability requiring ecological expertise. One future research topic is converting existing ecological parameters, such as basal area, to engineering parameters, such as projected area (1/32). NNBF designs may also create unintended consequences (1/32).
We also asked subject matter experts about sociopolitical barriers to NNBF implementation (Figure 2). The most cited recommendations center on policies (21/32), including 1) prioritizing green solutions over traditional (structural) alternatives; 2) allowing NNBF projects to count for other environmental credits such as for stormwater; 3) requiring vegetation experts or plans to be included in projects; 4) encouraging redundancy in planning; 5) considering longer life cycles of up to 100 years; and 6) developing regulations specifically for hybrid systems. Of these policy responses, multiple suggest permitting modernization (5/32), which is also identified in the literature (Cherry et al., 2018). Suggestions include fast-tracking NNBF permits and modifying USACE Nationwide Permits to prioritize NNBF over gray infrastructure. Permitting modernization would focus on the removal of artificial barriers to NNBF project approval, allowing NNBF to be given equal consideration with traditional solutions. One potential area of conflict within policy arises from habitat regulations, as one government professional and two consultants note the need for flexibility with habitat conversion regulations (3/32), while an academic and nonprofit representative state the need for policies to protect existing emergent vegetation from degradation (2/32).
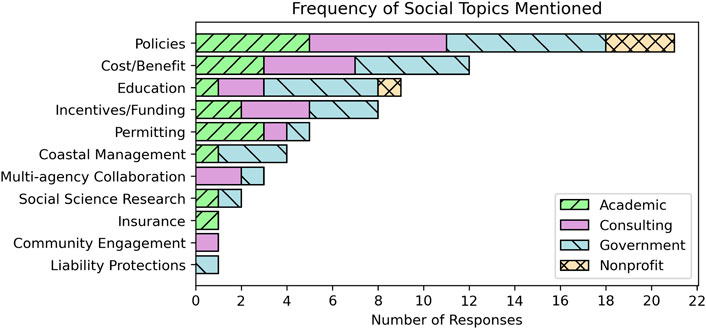
FIGURE 2. Frequency of categories of future socioeconomic and policy needs for emergent vegetation implementation cited by experts in survey responses (N=32).
In addition to policies, responses describe other social considerations that would catalyze future progress in NNBF implementation. Incentives should be created to encourage NNBF projects, including additional dedicated sources of funding (8/32). Additionally, cost-benefit analyses are in need of improvement, and should consider the full lifecycle cost of the project (12/32). These observations agree with recent studies that have identified challenges in quantifying costs, benefits, and co-benefits of NNBF (Close et al., 2017; Cherry et al., 2018; Zuniga-Teran et al., 2020). Collaboration across multiple government agencies is needed for effective projects (3/32), a recommendation which supports findings from previous workshops (Cherry et al., 2018; Zuniga-Teran et al., 2020). Community engagement is cited as an important component to creating a successful project (1/32), a finding also highlighted in the literature (Zuniga-Teran et al., 2020; Waryszak et al., 2021). Responses note the need for broader coastal management, such as 1) considering regional planning and retreat, perhaps utilizing a different term than the politicized “retreat”; 2) having government agencies acquire vegetated lands; and 3) developing tools specifically for coastal management (4/32). Education and updated materials for the public, project applicants, regulators, maintenance workers, students, and engineers are needed (9/32).
NNBF in the context of traditional structures
To elucidate the challenges of incorporating emergent vegetation systems in coastal infrastructure design, it is helpful to compare requirements for NNBF with practices for conventional infrastructure. In traditional civil engineering design, a coastal protection structure is sized and justified by performance objectives, such as flood risk management, erosion control, and/or wave and current mitigation under both extreme design events and normal operational conditions (e.g., USACE, 2002). In the design process, a clear understanding emerges on how the structure accomplishes its purpose, how success is measured, and the length of time the structure can maintain its desired performance. The structure’s performance is predictive, that is, based on a set of widely accepted, controllable assumptions and uncontrollable hazards. The structure’s performance and failure limits can also be determined, such as the storm surge height that can overwhelm the structure, wave types that can damage the structure, or storm conditions and durations that can generate significant erosion.
Established design methods exist for one form of NNBF: beach nourishment (e.g., USACE, 2002; Elko et al., 2021). Engineers select a grain size to be compatible with the existing geomorphological processes of the native beach, and calculate the volume of sand that can provide an acceptable dynamic response under a set of design parameters. Additional design decisions may include adding vegetation and widening the beach in front of a dune. However, since the “structure” (i.e., beach profile) dynamically adjusts through time to environmental conditions, performance factors are harder to control, predict, and improve, and nourished beaches are usually adaptively managed through monitoring, maintenance, or renourishment works.
The design considerations are more complicated for types of NNBF that consist of living systems, such as wetlands or reefs. From a large body of evidence based on field observations, physical modeling, and numerical modeling, engineers have been able to characterize key variables for specific ecosystems that control wave, water level, and erosion mitigation. After decades of observations, it emerges that different NNBF provide different types of coastal protection benefits. Table 1 builds off previous work (e.g., Cunniff and Schwartz, 2015; Bridges et al., 2021) to summarize current knowledge on protection mechanisms, performance, and services of NNBF. In this paper, we focus on emergent vegetation systems (salt marshes and mangroves).
Emergent vegetation can provide protection to inland areas by affecting nearshore hydrodynamics and attenuating wave heights (e.g., NAS, 1977; McIvor et al., 2012), nearshore currents (e.g., Guannel et al., 2015), and storm surge heights (e.g., Zhang et al., 2012). As a result, emergent vegetation may reduce the risk of erosion (Coops et al., 1996) and flooding (e.g., Narayan et al., 2017, 2019; Dong et al., 2020), as well as wave forces and resulting damage to coastal structures (Kyprioti et al., 2021; Mitchell, 2021) and ecosystems, both in response to chronic (La et al., 2015; Thuy et al., 2017; Tomiczek et al., 2022) and acute hazards (Narayan et al., 2019; Tomiczek et al., 2020a; Menéndez et al., 2020). Additionally, emergent vegetation can dynamically respond to increases in sea level by trapping sediment and moving landward, unless it is squeezed by development or rapid rates of submergence (Borchert et al., 2018; Saintilan et al., 2020). It is important to note that all protection services are relative and may be significantly reduced depending on various factors.
Despite this large body of evidence, evaluating the performance of NNBF is more complicated than for conventional systems. The protection services delivered by vegetation arise due to the drag force they exert on nearshore waters, and are a function of the morphology of emergent vegetation and the hydrodynamic forcing offshore (e.g., NAS, 1977; Dalrymple et al., 1984). Guannel et al. (2015) showed how the choice of a drag coefficient is sensitive to wave model formulation, and Kelty et al. (2022) were among the few to test such models under storm conditions. Additionally, contrary to conventional systems, the performance of NNBF is determined by ecological factors, which engineers cannot fully control, and can positively or negatively impact the performance of the system. For example, the ability of natural systems and their constituent species to grow, increase in density, and survive can be influenced by local or global processes like local climate, sea level rise, ocean acidification and warming, water quality, sedimentation rates, or the spread of diseases (Ross and Adam, 2013; Salimi et al., 2021). These factors, which are often influenced by humans (IPCC, 2013), impact the physical characteristics of natural systems (e.g., stem density and diameter) and hence their ability to moderate coastal hazards.
While traditional structural components may have controllable design parameters (e.g., rock weight for a rubble mound breakwater), emergent vegetation systems have design parameters that change both spatially (e.g., natural variability in trunk or prop root diameters, prop root distribution, and stem densities) and temporally (e.g., vegetation may grow and forest density may increase or recede over a system’s life cycle (e.g., Maza et al., 2021)). Moreover, while traditional projects can be built, maintained, and repaired immediately according to set specifications, NNBF need time to grow into the morphology that provides the desired protection benefits. For NNBF projects, engineers have less control of the performance of the system and contend with a higher level of uncertainty than for traditional coastal protection structures; a range of design parameters must be evaluated for NNBF systems.
2.3 Characterizing the performance of NNBF
To compute traditional design metrics in the presence of NNBF–overtopping, runup, wave force on inland structures, or cross-shore erosion–engineers incorporate vegetation modules in wave or nearshore circulation models, and couple these outputs with other established performance metrics models. For example, forces on structures behind emergent vegetation can be calculated using formulas such as Goda (2010) by accurately accounting for wave height attenuation due to vegetation (Mitchell, 2021).
Table 2 provides an overview of existing wave and hydrodynamic models available for emergent vegetation (see also Suzuki et al., 2019; Piercy et al., 2021). This table shows the wealth of numerical models that are now available, including Reynolds Averaged Navier Stokes (RANS) models, which resolve the highest level of physics, phase-averaged models, which summarize the wave conditions as wave spectra, and 1-Dimensional (1D) models, which use representative values of wave height and period. Details of vegetation implementation in each model are described in the references listed in the respective row of Table 2. Other models exist beyond those listed in Table 2, such as the Boussinesq-type model FUNWAVE (Blackmar et al., 2014), and the 1D phase-averaged wave and nearshore current model CSHORE, which can incorporate stem flexibility (Ding et al., 2022). Progress in computer modeling has allowed for a better understanding and quantification of the effects of vegetation on nearshore hydrodynamics.
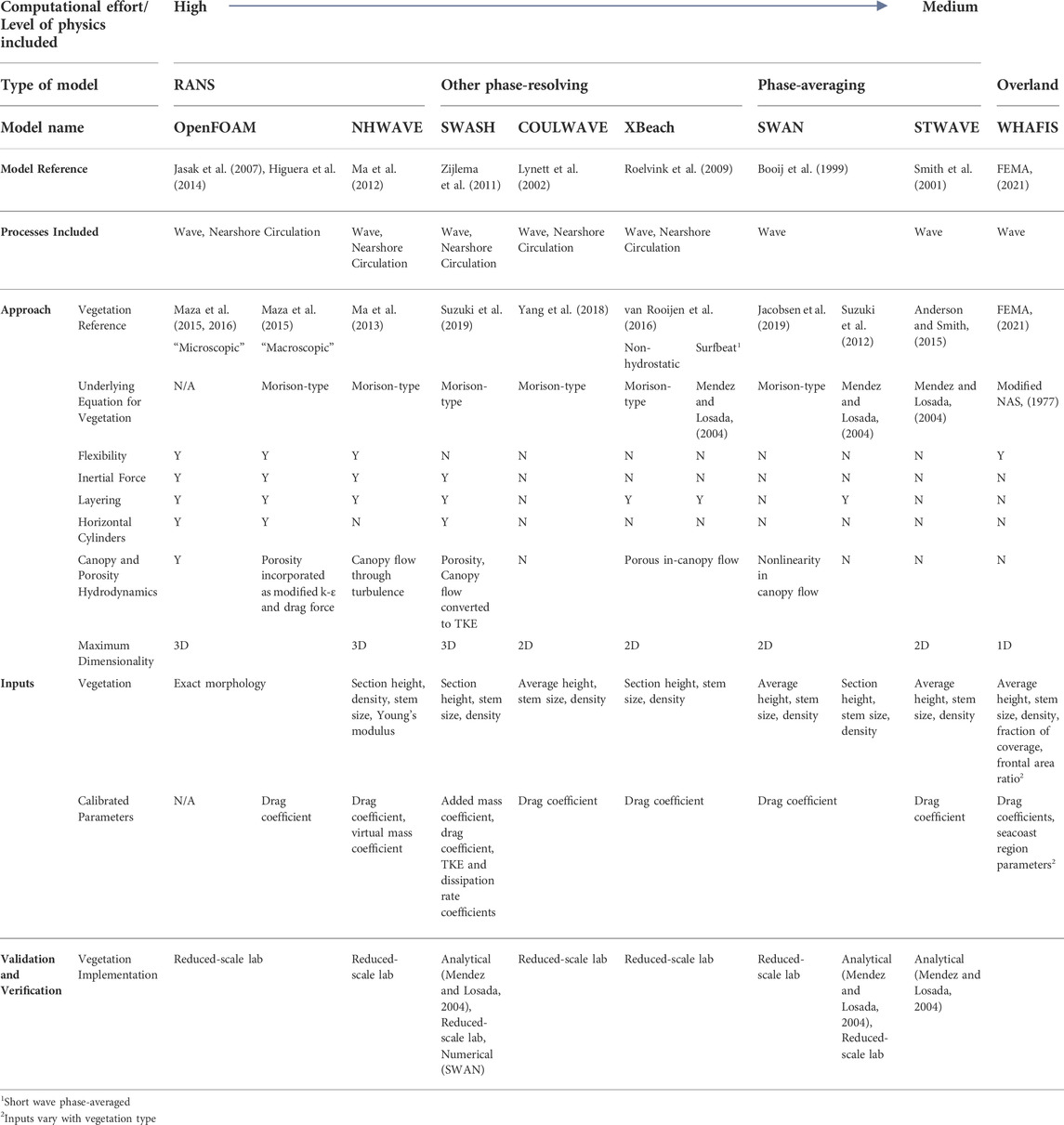
TABLE 2. Commonly used computational and analytical models for determining wave height attenuation through emergent vegetation.
Most of the models in Table 2 incorporate the effects of vegetation using Morison-type equations, which require information on the system’s morphological and hydrodynamic parameters. The accuracy of these parameters will determine the quality of the results (i.e., relying on drag coefficients from reduced scale laboratory studies may result in inaccurate amounts of wave attenuation). By necessity, models make simplifications or idealizations to the system to allow the model to run; however, the more physics that a model simplifies, the more uncertain the outputs. For example, many models neglect flexibility, an important parameter for marshes (e.g., van Veelen et al., 2020; Ding et al., 2022). Models also vary in their ability to layer different characteristics of vegetation elements in the water column, an important characteristic of mangroves such as Rhisophora sp. (e.g., Suzuki et al., 2019; Kelty et al., 2022), and to represent other fluid mechanics properties such as porosity (important in denser forests (Suzuki et al., 2019)), turbulence, and wave nonlinearity (Maza et al., 2015). Many models also do not reproduce wave transformation and water level changes in intertidal zones (Guannel et al., 2015; van Rooijen et al., 2016).
Importantly, although recent studies have validated some numerical models under certain storm and field conditions (e.g., Vuik et al., 2016; Baron-Hyppolite et al., 2019; Garzon et al., 2019), to the authors’ knowledge, the models are only validated under limited conditions, that is, against reduced-scale laboratory studies that do not consider storm conditions (see “Validation and Verification” in Table 2 and associated references). In fact, only one full-scale laboratory study has been carried out for storm wave attenuation of mangroves (Kelty et al., 2022). This study shows that, for the tested conditions, to have wave height attenuation of order 25%, an 18-m-wide forest needs to have a high density and still water elevation lower than the root system. Conversely, low density, high still water elevations with respect to the root system, and narrow fringes provide wave height attenuation on the order of 5% or less. While more research is needed to generalize these results, the data support the assertion that mangroves can provide storm wave attenuation, but not under every incident condition.
Beyond modeling the hydrodynamics, the ecological performance of NNBF systems must be characterized. During storms, trees bend and break, reducing the capacity of the forest to attenuate waves compared with the ideal conditions modeled in the design phase. Storms may also create conditions such as ponding, leading to delayed mortality of vegetation (e.g., Craighead and Gilbert, 1962; Lagomasino et al., 2021). Even if the emergent vegetation is successful at its purpose of protecting the built environment during a storm, the delayed mortality will cause the decomposing forest to break down and not provide the same level of service during the next storm. Likewise, damages to the built environment may occur even without a failure of the emergent vegetation itself. It is therefore important to distinguish between “engineering” failure (i.e., failure to provide the required hydraulic response) and “ecological” failure (i.e., inability to withstand the environmental conditions during a storm or owing to longer-term changes) in the design of NNBF; current approaches do not incorporate the latter.
As shown above, advances in computational methods allow for the improved quantification of emergent vegetation’s engineering performance. For example, based on information from results such as those by Kelty et al. (2022) and Maza et al. (2019), engineers may assess either 1) the cross-shore distance required to achieve a desired wave height reduction for a design condition, or 2) hybrid alternatives (e.g., structural measures) that can provide a second line of defense to provide the remaining required wave height attenuation. Engineers may also be able to assess expected wave height reduction, lowering design requirements on inland structural measures or near-coast structures. However, these models have limitations (Table 2), and designers should consider the impact these limitations have on the ability to design systems to meet performance requirements. Professional practice dictates that engineers have a primary responsibility to “protect the health, safety, and welfare of the public” (ASCE, 2022b). In traditional design, engineers rely on engineering design standards to produce design parameters that have a low, commonly accepted probability of failure, allowing engineers to have a high level of confidence that their designs will protect the public. Such standards do not exist for emergent vegetation, and questions about the uncertainty of the results, such as those raised above, linger. Therefore, it is difficult for engineers to have a high level of confidence in NNBF designs, and engineering design standards for NNBF are needed (Figure 1).
As a step toward design standards, we propose a framework to evaluate NNBF in such a way that engineers can ensure that lives and properties are protected, while simultaneously accounting for the engineering performance of natural systems following engineering design principles.
Evaluation and design framework
Even though there are many uncertainties that remain in the quantification of the physical behavior of emergent vegetation under hydrodynamic loads and their long-term performance in the face of uncontrollable ecological variables, the existing body of knowledge can be used for practical purposes (Figure 3). Since wave impact forces can generate significant damage to near-coast infrastructure (Robertson et al., 2007; FEMA, 2011; Duncan et al., 2021), this framework focuses on providing a methodology to quantify wave attenuation performance. The proposed framework can be used for the assessment of existing wetlands and for the design of new features. It should be integrated in a comprehensive process that includes other engineering evaluations (e.g., overtopping) as well as ecological (Piercy et al., 2021) and social dimensions (King et al., 2021), as suggested in Figure 4.
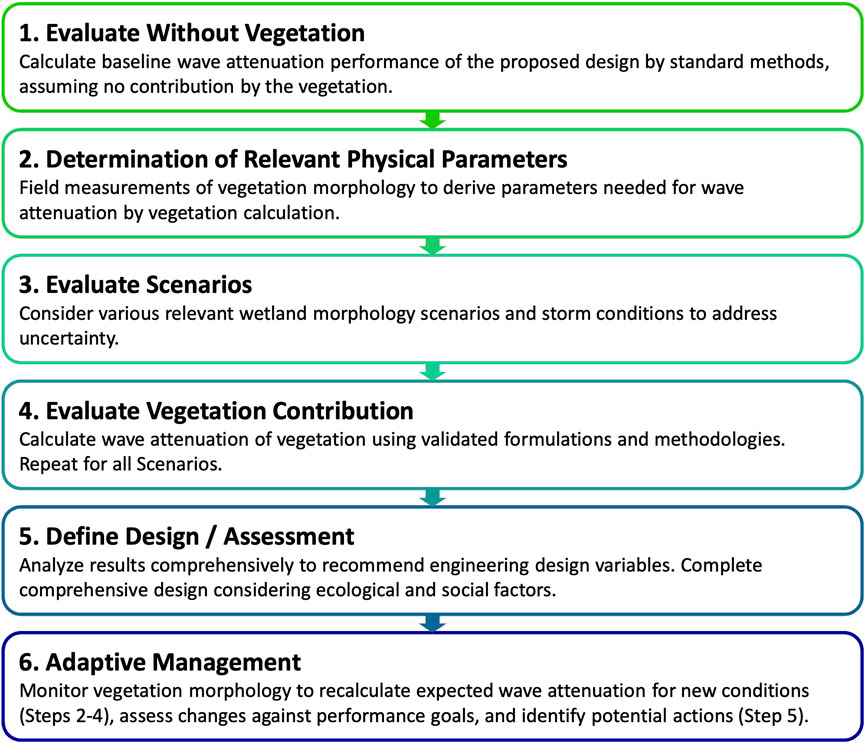
FIGURE 3. Framework for characterizing the wave attenuation performance of an emergent vegetation system.
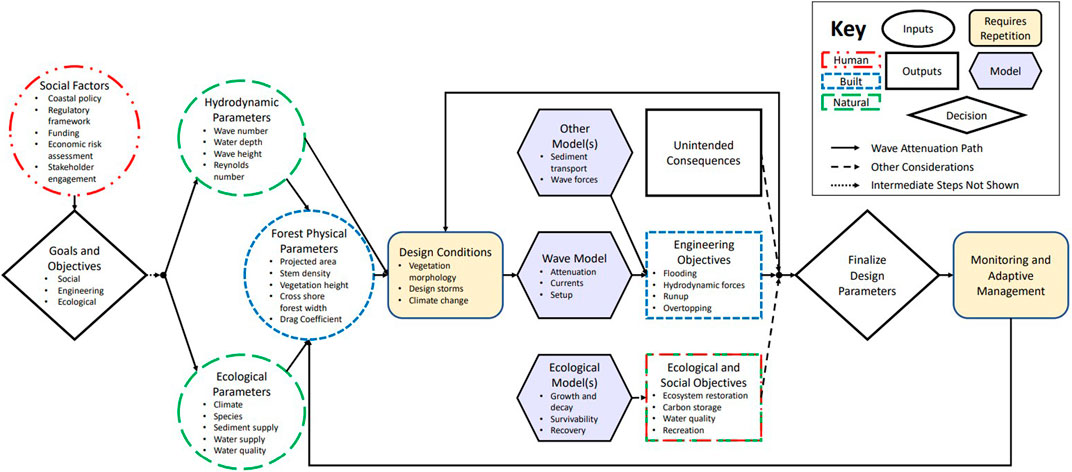
FIGURE 4. Expanded framework for NNBF describing objectives, variables affecting system performance, models for assessing performance, and engineering, ecological, social, and unintended outcomes.
The proposed analytical approach for the design of new emergent vegetation systems considers five key points (Figure 3). Step 1 involves calculating a baseline performance of the system without the contribution of vegetation (e.g., USACE, 2002). The quantification of this baseline is recommended because engineering design standards do not yet prescribe a method of calculating wave attenuation for emergent vegetation, and newly planted NNBF may perform as if no vegetation is present. The system including NNBF will therefore be overdesigned, as vegetation is expected to moderate forcing parameters over its lifetime.
The second step comprises of determining relevant physical parameters that will allow for the quantification of wave attenuation performance, for example, by measuring forest morphological parameters in the field (Figure 4). Stem density and height can be measured through traditional ecological methods (Cintrón and Schaeffer-Novelli, 1984). A variety of methods have been developed to characterize projected area (see Yoshikai et al., 2021 for an overview), including empirical models (e.g., Ohira et al., 2013; Mori et al., 2022), 3D laser scanning (e.g., Chang et al., 2019; Kelty et al., 2022), photogrammetry (e.g., Zhang et al., 2015; Maza et al., 2019), and remote sensing (Figueroa-Alfaro et al., 2022). Eventually, field measurement of engineering morphological parameters could be integrated with ecological field work. For new plantings, the framework recommends measuring the physical parameters of a benchmark nearby forest. This is analogous to the standard ecological design of NNBF, which includes the thorough understanding of ecological variables (e.g., terrain elevation, water elevation ranges, vegetation species composition) of a nearby wetland community (UNEP-Nairobi Convention/USAID/WIOMSA, 2020). The reference forest’s capability of representing a future condition of the proposed wetland should be validated through an ecological evaluation. The field measurement collection process can be simplified by considering a set of scenarios that are relevant to the study goals. For example, to quantify the economic benefit of an existing, healthy mangrove forest, a scenario with a degraded forest may be used for comparison. The framework conservatively neglects the forest canopy when designing for storm conditions, assuming all leaves are gone and small branches have broken. For engineering purposes, the minimum attenuation performance is more important than average conditions, and should be the aim of measurements. For the drag coefficient, estimates vary widely (Pinsky et al., 2013) as relationships for coefficients based on the Reynolds number (Re) derived from small-scale flume studies do not match with recent full-scale studies (Kelty et al., 2022), owing to kinematic scaling differences between the Froude and Reynolds numbers under Froude similitude (Heller, 2011). Recent prototype-scale physical models have suggested equations for the drag coefficient as a function of Re, with the coefficient approaching 0.6 for large values (Kelty et al., 2022).
Because the actual wetland vegetation morphology cannot be fully predicted and future storm conditions have increasing uncertainty (IPCC, 2013), the third step defines various scenarios of vegetation morphology, storm parameters (e.g., storm surge, wave heights) and sea levels. Emergent vegetation consists of, by definition, living systems that grow and adapt to changing environmental conditions at various time scales; the physical structure of the wetland at the design storm’s time of impact is likely to be different from the conditions at the time of design, and is uncertain and uncontrollable to a certain extent. In addition, storm conditions may cause emergent vegetation to fail during the event (e.g., Doyle, 1995), meaning it no longer has its protective capabilities (Table 1). This uncertainty can be accounted for by quantifying the performance of alternative (but similar) ecosystems, assessing possible growth rates, stressors, and more. This understanding should inform the adoption of a set of representative conditions for calculation (scenarios), and analysis of performance results for a given design storm under each scenario. Additionally, this step should be used to assess the resilience of NNBF to climate change stressors and to explore potential adaptation scenarios. At a minimum, alternative design storm parameters (e.g., different return periods) and the influence of sea level rise on storms should be evaluated (Biondi and Guannel, 2018). It may also be appropriate to qualitatively consider a broader range of other potential conditions, but a quantitative calculation may only be required for a limited number of selected scenarios, depending on the design goals.
Step 4 involves using validated tools to quantify wave attenuation based on the physical parameters and scenarios identified in Steps 2 and 3. Multiple tools exist for completing these calculations in Step 4 (Table 2), but it is recommended to use tools that have been validated for prototype-scale laboratory studies or field studies that cover a wide range of initial conditions, such as the Mendez and Losada (2004) equation for the conditions in Kelty et al. (2022). In models, the spatial scale should be sufficient to evaluate a forest between tens and hundreds of meters wide, and the vertical structure of the forest should be reproduced and sensitive to changing water elevations. Once wave attenuation by the emergent vegetation is calculated, other engineering performance parameters of the original design, such as overtopping, wave forces, and runup, can be assessed using appropriate engineering tools. Based on these analyses, a range of performance results under different conditions and assumptions can be identified. These quantitative data should be adequate for the engineer to make appropriate design decisions, weighing uncertainties, costs, performance, and risk. Engineering, ecological, and social benefits can also be evaluated across different types of solutions. With these results, engineering criteria can be used to justify a design of an emergent vegetation system (Step 5).Due to the living nature of emergent vegetation, the morphological parameters of a system will change over time for both new designs and already existing marshes and forests. As the built wetland changes over time (e.g., growth), or responds to acute disturbances (e.g., storm events) or ecological changes (e.g., disease), monitoring of physical morphology can be used to update expected wave attenuation performance. After the project has been implemented, Steps 2 and 4 should be repeated to obtain updated morphological parameters and calculation results, which should be evaluated by the engineer as part of a revisited Step 3. In a created wetland, the analysis should use measurements from the wetland itself, removing uncertainty derived from using parameters of a reference forest. In existing, restored, or created forests, calculation updates can be done in response to observed changes in the forest structure, either due to growth, ecological stress, or storm damage. Given the biological and engineering performance of NNBF, additional actions may be taken over the project lifetime to improve its performance of overall benefits. This can be part of an adaptive management approach, as described in NNBF design guidance (de Looff et al., 2021; Piercy et al., 2021).
Discussion
The framework presented in this paper provides ways for engineers, designers, and stakeholders to include emergent vegetation in coastal infrastructure design in a way that both demonstrates the value of the protection services delivered and creates a pathway for the creation of rigorous design standards in the future. To some extent, the present state of the practice of engineering with nature for emergent vegetation is reminiscent of the development of rubble mound structure methodologies, which began in the 1950s with limited data available and evolved over time to have well-established standards (Hudson, 1958, 1974; USACE, 1977, 1984, 2002). Alternative formulations and coefficients were used by engineers to inform a decision-making process, even with uncertainty of the structural performance.
The proposed framework allows for prudent, conservative approaches to incorporating NNBF in coastal engineering designs. This approach is also appropriate for engineers to be in compliance with ASCE guidance. Currently, to the authors’ knowledge, the only mention of NNBF in existing engineering design standards in the United States is in ASCE 24 (ASCE, 2014). ASCE 24-14 4.3 states that projects “shall not remove or otherwise alter sand dunes and mangrove stands, unless an engineering report documents that the alterations will not increase potential flood damage by reducing the wave and flow dissipation characteristics of the sand dunes or mangrove stands” (ASCE, 2014). Notwithstanding environmental regulations that would typically prevent the removal of mangroves, the burden of proof requires that the engineer prove that alterations would not exacerbate wave impacts. Therefore, from an engineering perspective (i.e., ASCE compliance) no removal of natural features can be justified because research demonstrates that a mangrove stand always provides some level of wave attenuation, and therefore removal will cause some increase in the potential damage. Even under unfavorable circumstances, mangrove protection can increase over time (e.g., growth in height, root density), so present conditions cannot be used to justify that the removal will not increase potential damage.
However, expanding upon the spirit of ASCE 24-14, it is also worth considering how wetlands provide protection under future climate change scenarios. Rising sea level and changes to the frequency, intensity, and speed of storms (Emanuel, 2005; Mendelsohn et al., 2012; Kossin, 2018; Sweet et al., 2022) will affect the performance of mangroves and wetlands as their footprint and composition are required to adapt to changing conditions (Hagen et al., 2013; Lovelock et al., 2015; Woodroffe et al., 2016). These changes will in turn impact the performance of coastal infrastructure (Biondi and Guannel, 2018). While these facts are not yet part of engineering design standards, they should be accounted for by practicing engineers and considered in the definition of scenarios (Figure 3). The wave protection afforded by emergent vegetation should be considered as a part of resilience and adaptation strategies where these systems are a viable alternative from a physical and ecological standpoint.
In addition to providing an opportunity to improve engineering guidance, the proposed framework creates opportunities for convergence among academic and professional disciplines. First, the plants themselves could have unintended consequences (Figure 4). For example, emergent vegetation can modify nearshore currents and sediment transport, which may be detrimental for a particular site (e.g., Allen, 1998), become refuge for mosquitos (e.g., Rey et al., 2012), contribute to trash and debris buildup (Cunniff and Schwartz, 2015), or become projectile debris during an extreme storm event. Edge effects should be characterized at locations where emergent vegetation integrates with other shoreline typologies. These issues can be addressed if considered as part of the design, and the inclusion of researchers, practitioners, and stakeholders during monitoring efforts can help direct future guidance that includes both engineering and ecological dimensions.
Furthermore, implementation of NNBF must consider the sociopolitical context in the locations in which they are deployed, requiring skills beyond pure engineering and environmental sciences. Implementing NNBF projects requires navigating the factors that were cited as barriers to implementation (Figure 2; see also Cherry et al., 2018; Zuniga-Teran et al., 2020) such as convoluted permitting processes, limited funding streams, public perception, and enhanced coordination. Community engagement and appropriate socioeconomic analyses over a project’s life cycle are also cited as critical for successful implementation of projects but are often insufficiently considered (Zuniga-Teran et al., 2020).
One way to garner public support is to properly account for the full value of NNBF. NNBF projects, which often include public access and amenity features (e.g., boardwalks, kayak trails, kayak launches), can have significant economic, recreational, and aesthetic value (Prato and Hey, 2006; Pueyo-Ros et al., 2018), and provide habitat and improved water quality that can support fisheries and biodiversity (Odum et al., 1982; USFWS, 1999; Struve and Falconer, 2001; Wang et al., 2010). Consequently, the full evaluation of the benefits delivered by emergent vegetation used for coastal protection requires a solid understanding of the relationship between the engineering, ecological, and social dimensions at play at a particular site. While coastal protection may be a benefit driving a particular project, all potential benefits should be pursued. These multiple performance objectives must be evaluated during the planning and design process, and subsequently monitored along with engineering performance objectives to assess the system’s overall performance (van Zanten et al., 2021).
Future research priorities
In this paper, we summarized a state of the practice through a review of the literature and elicitation of expert opinion, and proposed a framework that can increase the adoption of NNBF by various stakeholders. We identify three main areas of focus for more widespread implementation of emergent vegetation systems:
1) Validating existing models and characterizing uncertainty in ecological and engineering parameters;
2) Understanding lifecycle performance, including factors that affect survivability and relevant time scales; and
3) Anticipating unintended social, environmental, and engineering consequences.
Engineers require comprehensive validation of the methodology to quantify wave attenuation and develop design standards. This validation would involve blind model studies; presently, most validation studies tune model parameters to fit data. Furthermore, work is needed to determine conservative values for, and uncertainties associated with, input parameters in the wave height attenuation and/or other models for engineering performance output variables. This knowledge would help to characterize the reliability of NNBF systems. Further quantification needs to be determined for forests with a mixed composition of species, hybrid systems, and 3-Dimensional effects.
Future work should also quantify the lifecycle performance and survivability of emergent vegetation with respect to acute stressors and long-term changes. Recovery after a storm should be analyzed to determine what human interventions are needed and over what time scales recovery occurs. Currently, no model in Table 2 incorporates survivability; mangroves are assumed to survive no matter how severe the event and associated environmental conditions. However, damage assessments show that mangrove tree limbs break during extreme events due to wind or debris impact, and can be at risk of delayed mortality due to extreme ponding or other ecological stressors (Tomiczek et al., 2020a; Radabaugh et al., 2020). In the future, process-based ecological models (Charbonneau et al., 2022) could be adapted for emergent vegetation. These models could also be coupled with wave and nearshore hydrodynamic models for better predictions over time (Hagen et al., 2013).
Conclusion
This paper presents a review of the state of the art in leveraging emergent vegetation for coastal engineering design through a synthesis of expert opinion and recent literature. It further provides a design framework for emergent vegetation, identifying critical ecological and morphological parameters affecting system evolution and capability, required variables for wave height attenuation calculations, selection criteria for wave numerical models used for evaluating system performance, and scenarios to build up a set of performance outputs that can be evaluated based on project requirements to make design decisions. Following the methodology presented in Figures 3 and 4 is anticipated to yield estimates of wave attenuation to adequately inform the design and assessment of wave attenuation engineering performance of emergent vegetation NNBF.
Future research priorities are outlined to advance scientific knowledge and to reduce the uncertainty associated with the engineering performance of these systems, which can result in the development of design standards for emergent vegetation. While additional work is needed to provide the same level of detail as for conventional engineering systems, engineers must start broadening the implementation of emergent vegetation and other NNBF systems in the near-term future with the existing knowledge in systems engineering performance. Engineering coastal feasibility studies and design should also broaden the definition of performance objectives from solely engineering requirements to include ecological and social objectives. In the face of sea level rise and climate change, a paradigm shift is required in engineering design to embrace risk management methodologies and propose projects within a long-term adaptive management strategy.
Data availability statement
The raw data supporting the conclusions of this article will be made available by the authors, without undue reservation.
Author contributions
Conceptualization, EB, DC, GG, KO, and TT; Methodology, EB, DC, GG, KO, and TT; Formal Analysis, KO; Investigation, EB, DC, GG, KO, and TT; Writing–Original Draft Preparation, EB, DC, GG, KO, and TT; Writing–Review & Editing, EB, DC, GG, KO, and TT; Visualization, KO, GG; Supervision, EB, DC, GG, KO, and TT; Project Administration, EB, DC, GG, KO, and TT; Funding Acquisition, DC, GG, TT.
Funding
This project was supported by funding from the National Science Foundation CBET Grant #s 2110262 and 2110439, National Science Foundation OIA Grant #1946412, and from the US Army Corps of Engineers through Sponsor Award Number W912HZ2120045. Any opinions, findings, and conclusions or recommendations expressed in this material are those of the authors and do not necessarily reflect the views of the National Science Foundation, the US Army Corps of Engineers, or United States Naval Academy.
Acknowledgments
The authors are grateful to those individuals who provided expert opinion on the state of the art in emergent vegetation.
Conflict of interest
Author EB was employed by Applied Technology and Management/Geosyntec Consultants, Inc.
The remaining authors declare that the research was conducted in the absence of any commercial or financial relationships that could be construed as a potential conflict of interest.
Publisher’s note
All claims expressed in this article are solely those of the authors and do not necessarily represent those of their affiliated organizations, or those of the publisher, the editors and the reviewers. Any product that may be evaluated in this article, or claim that may be made by its manufacturer, is not guaranteed or endorsed by the publisher.
References
Allen, J. (1998). Mangroves as alien species: The case of Hawaii. Glob. Ecol. Biogeogr. Lett. 7, 61–71. doi:10.2307/2997698
Alongi, D. M. (2008). Mangrove forests: Resilience, protection from tsunamis, and responses to global climate change. Estuar. Coast. Shelf Sci. 76, 1–13. doi:10.1016/j.ecss.2007.08.024
Anderson, M. E., and Smith, J. M. (2015). Implementation of wave dissipation by vegetation in STWAVE. Vicksburg, MS. U.S: U.S. Army Engineer and Research Development Center.
Anderson, M. E., and Smith, J. M. (2014). Wave attenuation by flexible, idealized salt marsh vegetation. Coast. Eng. 83, 82–92. doi:10.1016/j.coastaleng.2013.10.004
Arkema, K. K., Guannel, G., Verutes, G., Wood, S. A., Guerry, A., Ruckelshaus, M., et al. (2013). Coastal habitats shield people and property from sea-level rise and storms. Nat. Clim. Chang. 3, 913–918. doi:10.1038/nclimate1944
Arkema, K. K., Verutes, G. M., Wood, S. A., Clarke-Samuels, C., Rosado, S., Canto, M., et al. (2015). Embedding ecosystem services in coastal planning leads to better outcomes for people and nature. Proc. Natl. Acad. Sci. U. S. A. 112, 7390–7395. doi:10.1073/pnas.1406483112
ASCE (2022b). Code of ethics. Am. Soc. Civ. Eng. Available at: https://www.asce.org/career-growth/ethics/code-of-ethics (Accessed April 18, 2022).
ASCE (2014). Flood resistant design and construction. Reston, VA: American Society of Civil Engineers. ASCE/SEI 24-14. doi:10.1061/9780784413791
ASCE (2022a). Minimum design loads and associated criteria for buildings and other structures. ASCE/SEI 7-22: ASCE 7 standard. doi:10.1061/9780784415788
Barbier, E. B., Hacker, S. D., Kennedy, C., Koch, E. W., Stier, A. C., and Silliman, B. R. (2011). The value of estuarine and coastal ecosystem services. Ecol. Monogr. 81, 169–193. doi:10.1890/10-1510.1
Baron-Hyppolite, C., Lashley, C. H., Garzon, J., Miesse, T., Ferreira, C., and Bricker, J. D. (2019). Comparison of implicit and explicit vegetation representations in SWAN hindcasting wave dissipation by coastal wetlands in chesapeake Bay. Geosciences 9, 8. doi:10.3390/geosciences9010008
Biondi, E. L., and Guannel, G. (2018). Practical tools for quantitative analysis of coastal vulnerability and sea level rise impacts—Application in a caribbean island and assessment of the 1.5 °C threshold. Reg. Environ. Change 18, 2227–2236. doi:10.1007/s10113-018-1397-4
Blackmar, P. J., Cox, D. T., and Wu, W.-C. (2014). Laboratory observations and numerical simulations of wave height attenuation in heterogeneous vegetation. J. Waterw. Port. Coast. Ocean. Eng. 140, 56–65. doi:10.1061/(ASCE)WW.1943-5460.0000215
Booij, N., Ris, R. C., and Holthuijsen, L. H. (1999). A third-generation wave model for coastal regions: 1. Model description and validation. J. Geophys. Res. 104, 7649–7666. doi:10.1029/98JC02622
Borchert, S. M., Osland, M. J., Enwright, N. M., and Griffith, K. T. (2018). Coastal wetland adaptation to sea level rise: Quantifying potential for landward migration and coastal squeeze. J. Appl. Ecol. 55, 2876–2887. doi:10.1111/1365-2664.13169
Bridges, T. S., King, J. K., Simm, J. D., Beck, M. W., Collins, G., Lodder, Q., et al. (2021). International guidelines on natural and nature-based features for flood risk management. (U.S.): Engineer Research and Development Center. Available at: https://hdl.handle.net/11681/41946.
Bridges, T. S., Wagner, P. W., Burks-Copes, K. A., Bates, M. E., Collier, Z. A., Fischenich, C. J., et al. (2015). Use of natural and nature-based features (NNBF) for coastal resilience. Vicksburg, MS. US: U.S. Army Engineer and Research Development Center. Available at: https://hdl.handle.net/11681/4769.
Browder, G., Ozment, S., Rehberger Bescos, I., Gartner, T., and Lange, G.-M. (2019). Integrating green and gray: Creating next generation infrastructure. Washington, DC: World Bank Group. doi:10.1596/978-1-56973-955-6
Castellari, S., Zandersen, M., Davis, M., Veerkamp, C., Förster, J., Marttunen, M., et al. The European Environment Agency (2021). Nature-based solutions in Europe policy, knowledge and practice for climate change adaptation and disaster risk reduction. Luxembourg: Publications Office. doi:10.2800/919315
Chang, C.-W., Mori, N., Tsuruta, N., and Suzuki, K. (2019). Estimation of wave force coefficients on mangrove models. J. Jpn. Soc. Civ. Eng. Ser. B2 75, I_1105–I_1110. doi:10.2208/kaigan.75.I_1105
Charbonneau, B. R., Duarte, A., Swannack, T. M., Johnson, B. D., and Piercy, C. D. (2022). Doonies: A process-based ecogeomorphological functional community model for coastal dune vegetation and landscape dynamics. Geomorphology 398, 108037. doi:10.1016/j.geomorph.2021.108037
Chen, Q., Li, Y., Kelly, D. M., Zhang, K., Zachry, B., and Rhome, J. (2021). Improved modeling of the role of mangroves in storm surge attenuation. Estuar. Coast. Shelf Sci. 260, 107515. doi:10.1016/j.ecss.2021.107515
Cherry, C., Dix, B., Asam, S., Webb, B., and Douglass, S. (2018). Peer exchange summary report: Nature-based solutions for coastal highway resilience. Washington, DC: Federal Highway Administration. Available at: https://trid.trb.org/view/1539901.
Cintrón, G., and Schaeffer-Novelli, Y. (1984). “Methods for studying mangrove structure,” in The mangrove ecosystem: Research methods monographs on oceanographic methodology. Editors S. C. Snedaker, and J. G. Snedaker (Paris: The United Nations Educational, Scientific and Cultural Organization), 251.
Close, S. L., Montalto, F., Orton, P., Antoine, A., Peters, D., Jones, H., et al. (2017). Achieving sustainability goals for urban coasts in the US northeast: Research needs and challenges. Local Environ. 22, 508–522. doi:10.1080/13549839.2016.1233526
Coops, H., Geilen, N., Verheij, H. J., Boeters, R., and van der Velde, G. (1996). Interactions between waves, bank erosion and emergent vegetation: An experimental study in a wave tank. Aquat. Bot. 53, 187–198. doi:10.1016/0304-3770(96)01027-3
Craighead, F. C., and Gilbert, V. C. (1962). The effects of hurricane donna on the vegetation of southern Florida. Q. J. Fla. Acad. Sci. 25, 1–28.
Cunha, J., Cardona, F. S., Bio, A., and Ramos, S. (2021). Importance of protection service against erosion and storm events provided by coastal ecosystems under climate change scenarios. Front. Mar. Sci. 8. doi:10.3389/fmars.2021.726145
Cunniff, S., and Schwartz, A. (2015). Performance of natural infrastructure and nature-based measures as coastal risk reduction features. New York: Environmental Defense Fund.
Dalrymple, R. A., Kirby, J. T., and Hwang, P. A. (1984). Wave diffraction due to areas of energy dissipation. J. Waterw. Port. Coast. Ocean. Eng. 110, 67–79. doi:10.1061/(asce)0733-950x
de Looff, H., Welp, T., Snider, N., and Wilmink, R. (2021). “Chapter 7: Adaptive management,” in International guidelines on natural and nature-based features for flood risk management. T. S. Bridges, J. K. King, J. D. Simm, M. W. Beck, G. Collins, Q. Lodderet al. (Vicksburg, MS: U.S. Army Engineer Research and Development Center).
Ding, Y., Chen, Q. J., Zhu, L., Rosati, J. D., and Johnson, B. D. (2022). Implementation of flexible vegetation into CSHORE for modeling wave attenuation. Vicksburg, MS: US Army Engineer Research and Development Center. doi:10.21079/11681/43220
Dong, S., Abolfathi, S., Salauddin, M., Tan, Z. H., and Pearson, J. M. (2020). Enhancing climate resilience of vertical seawall with retrofitting - a physical modelling study. Appl. Ocean Res. 103, 102331. doi:10.1016/j.apor.2020.102331
Doyle, T. W. (1995). Wind damage effects of hurricane andrew on mangrove communities along the southwest coast of Florida, USA. J. Coast. Res. 11, 159–168.
Duncan, S., Cox, D., Barbosa, A. R., Lomónaco, P., Park, H., Alam, M. S., et al. (2021). Physical modeling of progressive damage and failure of wood-frame coastal residential structures due to surge and wave forces. Coast. Eng. 169, 103959. doi:10.1016/j.coastaleng.2021.103959
Elko, N., Briggs, T. R., Benedet, L., Robertson, Q., Thomson, G., Webb, B. M., et al. (2021). A century of U.S. beach nourishment. Ocean. Coast. Manag. 199, 105406. doi:10.1016/j.ocecoaman.2020.105406
Emanuel, K. (2005). Increasing destructiveness of tropical cyclones over the past 30 years. Nature 436, 686–688. doi:10.1038/nature03906
IPCC (2013). in Climate change 2013: The physical science basis. Contribution of working group I to the fifth assessment report of the intergovernmental panel on climate change. T. F. Stocker, D. Qin, G.-K. Plattner, M. Tignor, S. K. Allen, J. Boschunget al. (Cambridge, United Kingdom and New York, NY, USA: Cambridge University Press). doi:10.1017/CBO9781107415324
Feagin, R. A., Bridges, T. S., Bledsoe, B., Losos, E., Ferreira, S., Corwin, E., et al. (2021). Infrastructure investment must incorporate Nature’s lessons in a rapidly changing world. One Earth 4, 1361–1364. doi:10.1016/j.oneear.2021.10.003
Fema, (2021). Guidance for flood risk analysis and mapping: Coastal overland wave propagation. India: FEMA.
Fema, (2011). Principles and practices of planning, siting, designing, constructing, and maintaining residential buildings in coastal areas. 4th Edition. India: FEMA.
Figueroa-Alfaro, R. W., van Rooijen, A., Garzon, J. L., Evans, M., and Harris, A. (2022). Modelling wave attenuation by saltmarsh using satellite-derived vegetation properties. Ecol. Eng. 176, 106528. doi:10.1016/j.ecoleng.2021.106528
Foster-Martinez, M. R., Alizad, K., and Hagen, S. C. (2020). Estimating wave attenuation at the coastal land margin with a GIS toolbox. Environ. Model. Softw. 132, 104788. doi:10.1016/j.envsoft.2020.104788
Garzon, J. L., Miesse, T., and Ferreira, C. M. (2019). Field-based numerical model investigation of wave propagation across marshes in the Chesapeake Bay under storm conditions. Coast. Eng. 146, 32–46. doi:10.1016/j.coastaleng.2018.11.001
Goda, Y. (2010). Random seas and design of maritime structures. 3rd ed. Singapore: World Scientific. doi:10.1142/7425
Guannel, G., Arkema, K., Ruggiero, P., and Verutes, G. (2016). The power of three: Coral reefs, seagrasses and mangroves protect coastal regions and increase their resilience. PLOS ONE 11, e0158094. doi:10.1371/journal.pone.0158094
Guannel, G., Ruggiero, P., Faries, J., Arkema, K., Pinsky, M., Gelfenbaum, G., et al. (2015). Integrated modeling framework to quantify the coastal protection services supplied by vegetation. J. Geophys. Res. Oceans 120, 324–345. doi:10.1002/2014JC009821
Hagen, S. C., Morris, J. T., Bacopoulos, P., and Weishampel, J. F. (2013). Sea-level rise impact on a salt marsh system of the lower st. Johns river. J. Waterw. Port. Coast. Ocean. Eng. 139, 118–125. doi:10.1061/(ASCE)WW.1943-5460.0000177
Heller, V. (2011). Scale effects in physical hydraulic engineering models. J. Hydraulic Res. 49, 293–306. doi:10.1080/00221686.2011.578914
Higuera, P., Lara, J. L., and Losada, I. J. (2014). Three-dimensional interaction of waves and porous coastal structures using OpenFOAM®. Part I: Formulation and validation. Coast. Eng. 83, 243–258. doi:10.1016/j.coastaleng.2013.08.010
Hochard, J. P., Hamilton, S., and Barbier, E. B. (2019). Mangroves shelter coastal economic activity from cyclones. Proc. Natl. Acad. Sci. U. S. A. 116, 12232–12237. doi:10.1073/pnas.1820067116
Hudson, R. Y. (1974). Concrete armor units for protection against wave attack. Vicksburg, MS: U.S. Army Engineer Waterways Experiment Station.
Hudson, R. Y. (1958). Design of quarry-stone cover layers for rubble-mound breakwaters. Vicksburg, MS: U.S. Army Engineer Waterways Experiment Station.
Jacobsen, N. G., McFall, B. C., and van der A, D. A. (2019). A frequency distributed dissipation model for canopies. Coast. Eng. 150, 135–146. doi:10.1016/j.coastaleng.2019.04.007
Jasak, H., Jemcov, A., and Tukovic, Z. (2007). “OpenFOAM: A C++ library for complex physics simulations,” in International workshop on coupled methods in numerical dynamics (Dubrovnik, Croatia), 20.
Kelty, K., Tomiczek, T., Cox, D. T., Lomonaco, P., and Mitchell, W. (2022). Prototype-scale physical model of wave attenuation through a mangrove forest of moderate cross-shore thickness: LiDAR-based characterization and Reynolds scaling for engineering with nature. Front. Mar. Sci. 8. doi:10.3389/fmars.2021.780946
King, J. K., Simm, J. D., and Bridges, T. S. (2021). “Chapter 2: Principles, frameworks, and outcomes,” in International guidelines on natural and nature-based features for flood risk management. T. S. Bridges, J. K. King, J. D. Simm, M. W. Beck, G. Collins, Q. Lodderet al. (Vicksburg, MS: U.S. Army Engineer Research and Development Center).
Kobayashi, N., Raichle, A. W., and Asano, T. (1993). Wave attenuation by vegetation. J. Waterw. Port. Coast. Ocean. Eng. 119, 30–48. doi:10.1061/(asce)0733-950x
Kossin, J. P. (2018). A global slowdown of tropical-cyclone translation speed. Nature 558, 104–107. doi:10.1038/s41586-018-0158-3
Kyprioti, A. P., Taflanidis, A. A., and Kennedy, A. B. (2021). Dissipation effects of coastal vegetation on nearshore structures under wave runup loading. J. Struct. Eng. 147, 06020010. doi:10.1061/(asce)st.1943-541x.0002902
La, T. V., Yagisawa, J., and Tanaka, N. (2015). Efficacy of Rhizophora apiculata and Nypa fruticans on attenuation of boat-generated waves under steep slope condition. Int. J. Ocean. Water Resour. 19, 1103–1111.
Lagomasino, D., Fatoyinbo, T., Castañeda-Moya, E., Cook, B. D., Montesano, P. M., Neigh, C. S. R., et al. (2021). Storm surge and ponding explain mangrove dieback in southwest Florida following Hurricane Irma. Nat. Commun. 12, 4003. doi:10.1038/s41467-021-24253-y
Lei, J., and Nepf, H. (2019). Wave damping by flexible vegetation: Connecting individual blade dynamics to the meadow scale. Coast. Eng. 147, 138–148. doi:10.1016/j.coastaleng.2019.01.008
Lovelock, C. E., Cahoon, D. R., Friess, D. A., Guntenspergen, G. R., Krauss, K. W., Reef, R., et al. (2015). The vulnerability of Indo-Pacific mangrove forests to sea-level rise. Nature 526, 559–563. doi:10.1038/nature15538
Lynett, P. J., Wu, T.-R., and Liu, P. L.-F. (2002). Modeling wave runup with depth-integrated equations. Coast. Eng. 46, 89–107. doi:10.1016/S0378-3839(02)00043-1
Ma, G., Kirby, J. T., Su, S.-F., Figlus, J., and Shi, F. (2013). Numerical study of turbulence and wave damping induced by vegetation canopies. Coast. Eng. 80, 68–78. doi:10.1016/j.coastaleng.2013.05.007
Ma, G., Shi, F., and Kirby, J. T. (2012). Shock-capturing non-hydrostatic model for fully dispersive surface wave processes. Ocean. Model. (Oxf). 43, 22–35. doi:10.1016/j.ocemod.2011.12.002
Maza, M., Lara, J. L., and Losada, I. J. (2019). Experimental analysis of wave attenuation and drag forces in a realistic fringe Rhizophora mangrove forest. Adv. Water Resour. 131, 103376. doi:10.1016/j.advwatres.2019.07.006
Maza, M., Lara, J. L., and Losada, I. J. (2021). Predicting the evolution of coastal protection service with mangrove forest age. Coast. Eng. 168, 103922. doi:10.1016/j.coastaleng.2021.103922
Maza, M., Lara, J. L., and Losada, I. J. (2016). Solitary wave attenuation by vegetation patches. Adv. Water Resour. 98, 159–172. doi:10.1016/j.advwatres.2016.10.021
Maza, M., Lara, J. L., and Losada, I. J. (2015). Tsunami wave interaction with mangrove forests: A 3-D numerical approach. Coast. Eng. 98, 33–54. doi:10.1016/j.coastaleng.2015.01.002
Mazda, Y., Magi, M., Kogo, M., and Hong, P. N. (1997). Mangroves as a coastal protection from waves in the Tong King delta, Vietnam. Mangroves Salt Marshes 1, 127–135. doi:10.1023/A:1009928003700
McIvor, A. L., Möller, I., Spencer, T., and Spalding, M. (2012). Reduction of wind and swell waves by mangroves. Natural Coastal Protection Series: Report 1. Cambridge Coastal Research Unit Working Paper 40. The Nature Conservancy and Wetlands International, 27. Available at: https://www.conservationgateway.org/ConservationPractices/Marine/crr/library/Documents/wind-and-swell-wave-reduction-by-mangroves.pdf.
Mendelsohn, R., Emanuel, K., Chonabayashi, S., and Bakkensen, L. (2012). The impact of climate change on global tropical cyclone damage. Nat. Clim. Chang. 2, 205–209. doi:10.1038/nclimate1357
Mendez, F. J., and Losada, I. J. (2004). An empirical model to estimate the propagation of random breaking and nonbreaking waves over vegetation fields. Coast. Eng. 51, 103–118. doi:10.1016/j.coastaleng.2003.11.003
Menéndez, P., Losada, I. J., Torres-Ortega, S., Narayan, S., and Beck, M. W. (2020). The global flood protection benefits of mangroves. Sci. Rep. 10, 4404. doi:10.1038/s41598-020-61136-6
Miller, J. K., Rella, A., Williams, A., and Sproule, E. (2015). Living shorelines engineering guidelines. New Jersey: New Jersey Department of Environmental Protection.
Mitchell, W. T. (2021). Effect of an idealized mangrove forest of moderate cross-shore width on loads measured on a sheltered structure and comparison with predicted forces. Corvallis, OR.
Montgomery, J. M., Bryan, K. R., Mullarney, J. C., and Horstman, E. M. (2019). Attenuation of storm surges by coastal mangroves. Geophys. Res. Lett. 46, 2680–2689. doi:10.1029/2018GL081636
Mori, N., Chang, C.-W., Inoue, T., Akaji, Y., Hinokidani, K., Baba, S., et al. (2022). Parameterization of mangrove root structure of Rhizophora stylosa in coastal hydrodynamic model. Front. Built Environ. 7, 782219. doi:10.3389/fbuil.2021.782219
Narayan, S., Beck, M. W., Reguero, B. G., Losada, I. J., Wesenbeeck, B. V., Pontee, N., et al. (2016). The effectiveness, costs and coastal protection benefits of natural and nature-based defences. PLOS ONE 11, e0154735. doi:10.1371/journal.pone.0154735
Narayan, S., Beck, M. W., Wilson, P., Thomas, C. J., Guerrero, A., Shepard, C. C., et al. (2017). The value of coastal wetlands for flood damage reduction in the northeastern USA. Sci. Rep. 7, 9463. doi:10.1038/s41598-017-09269-z
Narayan, S., Thomas, C., Matthewman, J., Shepard, C. C., Geselbracht, L., Nzerem, K., et al. (2019). Valuing the flood risk reduction benefits of Florida’s mangroves. Arlington, VA: The Nature Conservancy.
NAS (1977). Methodology for calculating wave action effects associated with storm surges. Washington, DC: National Academy of Sciences.
Odum, W. E., McIvor, C. C., and Smith, T. J. (1982). The ecology of the mangroves of south Florida: A community profile. US: U.S. Fish and Wildlife Service.
Ohira, W., Honda, K., Nagai, M., and Ratanasuwan, A. (2013). Mangrove stilt root morphology modeling for estimating hydraulic drag in tsunami inundation simulation. Trees 27, 141–148. doi:10.1007/s00468-012-0782-8
Ozeren, Y., Wren, D. G., and Wu, W. (2014). Experimental investigation of wave attenuation through model and live vegetation. J. Waterw. Port. Coast. Ocean. Eng. 140, 04014019. doi:10.1061/(ASCE)WW.1943-5460.0000251
PIANC (2018). Environmental commission guide for applying working with nature to navigation infrastructure projects. Brussels: PIANC.
Piercy, C. D., Pontee, N., Narayan, S., Davis, J., and Meckley, T. (2021). “Chapter 10: Coastal wetlands and tidal flats,” in International guidelines on natural and nature-based features for flood risk management. T. S. Bridges, J. K. King, J. D. Simm, M. W. Beck, G. Collins, Q. Lodderet al. (Vicksburg, MS: U.S. Army Engineer Research and Development Center).
Pinsky, M. L., Guannel, G., and Arkema, K. K. (2013). Quantifying wave attenuation to inform coastal habitat conservation. Ecosphere 4, art95. doi:10.1890/ES13-00080.1
Prato, T., and Hey, D. (2006). Economic analysis of wetland restoration along the Illinois river. J. Am. Water Resour. Assoc. 42, 125–131. doi:10.1111/j.1752-1688.2006.tb03828.x
Pueyo-Ros, J., Garcia, X., Ribas, A., and Fraguell, R. M. (2018). Ecological restoration of a coastal wetland at a mass tourism destination. Will the recreational value increase or decrease? Ecol. Econ. 148, 1–14. doi:10.1016/j.ecolecon.2018.02.002
Radabaugh, K. R., Moyer, R. P., Chappel, A. R., Dontis, E. E., Russo, C. E., Joyse, K. M., et al. (2020). Mangrove damage, delayed mortality, and early recovery following hurricane irma at two landfall sites in southwest Florida, USA. Estuaries Coast. 43, 1104–1118. doi:10.1007/s12237-019-00564-8
Rey, J. R., Walton, W. E., Wolfe, R. J., Connelly, C. R., O’Connell, S. M., Berg, J., et al. (2012). North American wetlands and mosquito control. Int. J. Environ. Res. Public Health 9, 4537–4605. doi:10.3390/ijerph9124537
Robertson, I. N., Riggs, H. R., Yim, S. C., and Young, Y. L. (2007). Lessons from hurricane katrina storm surge on Bridges and buildings. J. Waterw. Port. Coast. Ocean. Eng. 133, 463–483. doi:10.1061/(asce)0733-950x
Roelvink, D., Reniers, A., van Dongeren, A., van Thiel de Vries, J., McCall, R., and Lescinski, J. (2009). Modelling storm impacts on beaches, dunes and barrier islands. Coast. Eng. 56, 1133–1152. doi:10.1016/j.coastaleng.2009.08.006
Ross, P. M., and Adam, P. (2013). Climate change and intertidal wetlands. Biology 2, 445–480. doi:10.3390/biology2010445
Ruckelshaus, M. H., Guannel, G., Arkema, K., Verutes, G., Griffin, R., Guerry, A., et al. (2016). Evaluating the benefits of green infrastructure for coastal areas: Location, location, location. Coast. Manage. 44, 504–516. doi:10.1080/08920753.2016.1208882
Saintilan, N., Khan, N. S., Ashe, E., Kelleway, J. J., Rogers, K., Woodroffe, C. D., et al. (2020). Thresholds of mangrove survival under rapid sea level rise. Science 368, 1118–1121. doi:10.1126/science.aba2656
Salimi, S., Almuktar, S. A. A. A. N., and Scholz, M. (2021). Impact of climate change on wetland ecosystems: A critical review of experimental wetlands. J. Environ. Manage. 286, 112160. doi:10.1016/j.jenvman.2021.112160
Sanderman, J., Hengl, T., Fiske, G., Solvik, K., Adame, M. F., Benson, L., et al. (2018). A global map of mangrove forest soil carbon at 30 m spatial resolution. Environ. Res. Lett. 13, 055002. doi:10.1088/1748-9326/aabe1c
Sarasota Bay Estuary Program (2018). Living shorelines: Guidance for sarasota Bay watershed. FL: Sarasota.
Science for Environment Policy, (2021). The solution is in nature. Bristol: Science Communication Unit.
Scyphers, S. B., Powers, S. P., Jr, K. L. H., and Byron, D. (2011). Oyster reefs as natural breakwaters mitigate shoreline loss and facilitate fisheries. PLOS ONE 6, e22396. doi:10.1371/journal.pone.0022396
Silver, J. M., Arkema, K. K., Griffin, R. M., Lashley, B., Lemay, M., Maldonado, S., et al. (2019). Advancing coastal risk reduction science and implementation by accounting for climate, ecosystems, and people. Front. Mar. Sci. 6. doi:10.3389/fmars.2019.00556
Smith, J. M., Sherlock, A. R., and Resio, D. T. (2001). Stwave: Steady-state spectral wave model user’s manual for STWAVE, version 3.0. Vicksburg, MS: U.S. Army Engineer and Research Development Center.
Spalding, M., and Parrett, C. L. (2019). Global patterns in mangrove recreation and tourism. Mar. Policy 110, 103540. doi:10.1016/j.marpol.2019.103540
Struve, J., and Falconer, R. A. (2001). Hydrodynamic and water quality processes in mangrove regions. J. Coast. Res., 65–75.
Suzuki, T., Hu, Z., Kumada, K., Phan, L. K., and Zijlema, M. (2019). Non-hydrostatic modeling of drag, inertia and porous effects in wave propagation over dense vegetation fields. Coast. Eng. 149, 49–64. doi:10.1016/j.coastaleng.2019.03.011
Suzuki, T., Zijlema, M., Burger, B., Meijer, M. C., and Narayan, S. (2012). Wave dissipation by vegetation with layer schematization in SWAN. Coast. Eng. 59, 64–71. doi:10.1016/j.coastaleng.2011.07.006
Sweet, W. V., Hamlington, B. D., Kopp, R. E., Weaver, C. P., Barnard, P. L., Bekaert, D., et al. (2022). Global and regional sea level rise scenarios for the United States: Updated mean projections and extreme water level probabilities along U.S. Coastlines. Silver spring, MD. Spring, MD: National Oceanic and Atmospheric Administration. National Ocean Service Available at: https://oceanservice.noaa.gov/hazards/sealevelrise/noaa-nostechrpt01-global-regional-SLR-scenarios-US.pdf.
Taylor, E. B., Gibeaut, J. C., Yoskowitz, D. W., and Starek, M. J. (2015). Assessment and monetary valuation of the storm protection function of beaches and foredunes on the Texas coast. J. Coast. Res. 31, 1205–1216. doi:10.2112/JCOASTRES-D-14-00133.1
Thuy, N. B., Nandasena, N. A. K., Dang, V. H., Kim, S., Hien, N. X., Hole, L. R., et al. (2017). Effect of river vegetation with timber piling on ship wave attenuation: Investigation by field survey and numerical modeling. Ocean. Eng. 129, 37–45. doi:10.1016/j.oceaneng.2016.11.004
Tomiczek, T., O’Donnell, K., Furman, K., Webbmartin, B., and Scyphers, S. (2020a). Rapid damage assessments of shorelines and structures in the Florida keys after hurricane irma. Nat. Hazards Rev. 21, 05019006. doi:10.1061/(ASCE)NH.1527-6996.0000349
Tomiczek, T., Wargula, A., Lomónaco, P., Goodwin, S., Cox, D., Kennedy, A., et al. (2020b). Physical model investigation of mid-scale mangrove effects on flow hydrodynamics and pressures and loads in the built environment. Coast. Eng. 162, 103791. doi:10.1016/j.coastaleng.2020.103791
Tomiczek, T., Wargula, A., O’Donnell, K., LaVeck, V., Castagno, K. A., and Scyphers, S. (2022). Vessel-generated wake attenuation by Rhizophora mangle in key west, Florida. J. Waterw. Port. Coast. Ocean. Eng. 148, 04022002. doi:10.1061/(ASCE)WW.1943-5460.0000704
Uddin, Md. S., de Ruyter van Steveninck, E., Stuip, M., and Shah, M. A. R. (2013). Economic valuation of provisioning and cultural services of a protected mangrove ecosystem: A case study on sundarbans reserve forest, Bangladesh. Ecosyst. Serv. 5, 88–93. doi:10.1016/j.ecoser.2013.07.002
UNDRR (2020). Ecosystem-based disaster risk reduction: Implementing nature-based solutions for resilience. Bangkok, Thailand: United Nations Office for Disaster Risk Reduction – Regional Office for Asia and the Pacific.
UNEP-Nairobi Convention/USAID/WIOMSA (2020). Guidelines on mangrove ecosystem restoration for the western Indian ocean region. Nairobi: UNEP. Available at: https://www.nairobiconvention.org/CHM%20Documents/WIOSAP/guidelines/GuidelinesonMangroveRestorationForTheWIO.pdf.
USACE (1977). Shore protection manual. Vicksburg, MS: U.S. Army Coastal Engineering Research Center.
USACE (1984). Shore protection manual. Vicksburg, MS: U.S. Army Engineer Waterways Experiment Station.
USFWS (1999). South Florida multi-species recovery plan. Atlanta, GA: U.S. Fish and Wildlife Service.
van Rooijen, A. A., McCall, R. T., van Thiel de Vries, J. S. M., van Dongeren, A. R., Reniers, A. J. H. M., and Roelvink, J. A. (2016). Modeling the effect of wave-vegetation interaction on wave setup: Wave Setup Damping by Vegetation. J. Geophys. Res. Oceans 121, 4341–4359. doi:10.1002/2015JC011392
van Veelen, T. J., Fairchild, T. P., Reeve, D. E., and Karunarathna, H. (2020). Experimental study on vegetation flexibility as control parameter for wave damping and velocity structure. Coast. Eng. 157, 103648. doi:10.1016/j.coastaleng.2020.103648
van Zanten, B., Arkema, K., Swannack, T., Griffin, R., Narayan, S., Penn, K., et al. (2021). “Chapter 6: Benefits and costs of NNBF,” in International guidelines on natural and nature-based features for flood risk management. T. S. Bridges, J. K. King, J. D. Simm, M. W. Beck, G. Collins, Q. Lodderet al. (Vicksburg, MS: U.S. Army Engineer Research and Development Center).
Vuik, V., Jonkman, S. N., Borsje, B. W., and Suzuki, T. (2016). Nature-based flood protection: The efficiency of vegetated foreshores for reducing wave loads on coastal dikes. Coast. Eng. 116, 42–56. doi:10.1016/j.coastaleng.2016.06.001
Wang, M., Zhang, J., Tu, Z., Gao, X., and Wang, W. (2010). Maintenance of estuarine water quality by mangroves occurs during flood periods: A case study of a subtropical mangrove wetland. Mar. Pollut. Bull. 60, 2154–2160. doi:10.1016/j.marpolbul.2010.07.025
Waryszak, P., Gavoille, A., Whitt, A. A., Kelvin, J., and Macreadie, P. I. (2021). Combining gray and green infrastructure to improve coastal resilience: Lessons learnt from hybrid flood defenses. Coast. Eng. J. 63, 335–350. doi:10.1080/21664250.2021.1920278
Webb, B. M., Dix, B., Douglass, S. L., Asam, S., Cherry, C., Buhring, B., et al. (2019). Nature-based solutions for coastal highway resilience: An implementation guide. Washington, DC.
Woodroffe, C. D., Rogers, K., McKee, K. L., Lovelock, C. E., Mendelssohn, I. A., and Saintilan, N. (2016). Mangrove sedimentation and response to relative sea-level rise. Annu. Rev. Mar. Sci. 8, 243–266. doi:10.1146/annurev-marine-122414-034025
World Bank (2017). Implementing nature-based flood protection: Principles and implementation guidance. Washington, DC: World Bank.
Yang, Z., Tang, J., and Shen, Y. (2018). Numerical study for vegetation effects on coastal wave propagation by using nonlinear Boussinesq model. Appl. Ocean Res. 70, 32–40. doi:10.1016/j.apor.2017.09.001
Yoshikai, M., Nakamura, T., Suwa, R., Rollon, R., and Nadaoka, K. (2021). “Measurement and modeling of above-ground root systems as attributes of flow and wave attenuation function of mangroves,” in Mangroves: Ecology, biodiversity and management. Editors R. P. Rastogi, M. Phulwaria, and D. K. Gupta (Singapore: Springer), 279–303. doi:10.1007/978-981-16-2494-0_12
Zhang, K., Liu, H., Li, Y., Xu, H., Shen, J., Rhome, J., et al. (2012). The role of mangroves in attenuating storm surges. Estuar. Coast. Shelf Sci. 102–103, 11–23. doi:10.1016/j.ecss.2012.02.021
Zhang, X., Chua, V. P., and Cheong, H.-F. (2015). Hydrodynamics in mangrove prop roots and their physical properties. J. Hydro-environment Res. 9, 281–294. doi:10.1016/j.jher.2014.07.010
Zijlema, M., Stelling, G., and Smit, P. (2011). Swash: An operational public domain code for simulating wave fields and rapidly varied flows in coastal waters. Coast. Eng. 58, 992–1012. doi:10.1016/j.coastaleng.2011.05.015
Keywords: engineering with nature, natural and nature-based features, working with nature, building with nature, emergent vegetation, wave attenuation, design guidelines, nature-based solutions
Citation: Ostrow K, Guannel G, Biondi EL, Cox DT and Tomiczek T (2022) State of the practice and engineering framework for using emergent vegetation in coastal infrastructure. Front. Built Environ. 8:923965. doi: 10.3389/fbuil.2022.923965
Received: 20 April 2022; Accepted: 03 August 2022;
Published: 31 August 2022.
Edited by:
Spyros Hirdaris, Aalto University, FinlandReviewed by:
Matthew V. Bilskie, University of Georgia, United StatesSooyoul Kim, Kumamoto University, Japan
Copyright © 2022 Ostrow, Guannel, Biondi, Cox and Tomiczek. This is an open-access article distributed under the terms of the Creative Commons Attribution License (CC BY). The use, distribution or reproduction in other forums is permitted, provided the original author(s) and the copyright owner(s) are credited and that the original publication in this journal is cited, in accordance with accepted academic practice. No use, distribution or reproduction is permitted which does not comply with these terms.
*Correspondence: Kayla Ostrow, b3N0cm93a0BvcmVnb25zdGF0ZS5lZHU=