- 1Department of Material Science, Faculty of Mechanical Engineering, Technical University of Liberec, Liberec, Czech Republic
- 2Department of Materials Technology and Production Systems, Faculty of Mechanical Engineering, Lodz University of Technology, Lodz, Poland
- 3Faculty of Civil Engineering, Mechanics and Petrochemistry, Warsaw University of Technology, Płock, Poland
- 4Department of Acoustics, Multimedia and Signal Processing, WroclawUniversity of Science and Technology, Wrocław, Poland
This paper deals with investigation of changes in geopolymer wettability with increasing mass fraction of high-carbon fly ash and surface treatment by cold atmospheric plasma (CAP) to determine the influence of fly ash on wettability and whether it is a viable method to increase surface wettability for further surface treatment. In this study, multiple samples of geopolymers were prepared, including those with 16% and 32% of high-carbon fly ash from coal-fired power station. Wettability of samples was then measured before and after plasma treatment, both on surface and cut surface by using static sessile drop method to measure the differences in contact angle. While addition of fly ash only had low effect on the wettability, as in most cases, it only lowered the initial contact angle without speeding up the speed of soaking for compact geopolymer and actually slowed the soaking for foamed geopolymer, plasma treatment had significant impact and made the geopolymer completely hydrophobic, making plasma treatment a viable method to increase geopolymer wettability.
1 Introduction
Geopolymers, also called geopolymer gels (Nenadović et al., 2021), are materials made from alumino-silicate materials, such as metakaolin (MEFISTO L05, 2022), furnace slag (Trinh et al., 2020) fly ash (Kristály et al., 2020) or precursors prepared synthetically via sol-gel method (Tsai et al., 2010), which are primarily composed of silicon dioxide, aluminium oxide, iron oxide and other oxides. They are formed by polycondensation in strongly basic environment, usually in hydroxide or silicate solution. During the reaction, aluminosilicates are transformed to polysialates and form zeolitic (microporous) structure (Růžek, 2020). In comparison with ordinary Portland cement (OPC), most widely used construction material, geopolymers exhibit higher compressive strength (Gailitis et al., 2020a), resistance against high temperatures (Heah et al., 2017), resistance against chemicals and lower thermal conductivity (Prałat et al., 2021). Their manufacturing also requires less energy than OPC. The disadvantages include lower tensile strength and higher price. They may also be used for deposition of waste, such as high-carbon fly ash from coal power stations (Buczkowska et al., 2020) (Ambrus et al., 2019) or glass (Kristály et al., 2020). Using such commodities as geopolymer filler may also lower the price or geopolymers and lower the amount of landfilled waste (Buczkowska et al., 2020). Mechanical properties of compact geopolymer with high carbon fly ash are marginally lower than in pure geopolymer (Dufková et al., 2020), while mechanical properties of foamed geopolymer are improved by high carbon fly ash (Buczkowska et al., 2020). Geopolymers are a potential alternative to OPC as a building material (Nguyen et al., 2022) as well as useful material for other special application where their properties may be used, such as passive fire protection (Łach et al., 2018), where foamed geopolymer is used, as foaming decreases its thermal conductivity and weight, while also increasing their resistance against fire and high temperatures. Geopolymer foam may also be used as insulation for buildings. (Le et al., 2020a) (Le et al., 2020b) (Le and Louda, 2021). Geopolymers are also a viable material for 3D printing (Korniejenko et al., 2020a; Korniejenko et al., 2020d).
Geopolymers, as well as OPC, are also commonly reinforced by various types of fibers (Korniejenko et al., 2020b) (Ranjbar and Zhang, 2019; Korniejenko and Łach, 2020), to compensate for their low tensile and flexural strength (Ciemnicka et al., 2021) (Abbas et al., 2022), as well as to enhance their high compressive strength (Gailitis et al., 2021a), although some materials, such as common glass or light metals cannot be used, due to high geopolymer basicity (Růžek, 2020). Fibers also reduce internal cracking (Kozub et al., 2021a). Materials used to reinforce geopolymers (and other materials) include carbon (Gailitis et al., 2021a) (Le Chi et al., 2021) (Łach et al., 2019), basalt (Le Chi et al., 2019a) (Le Chi et al., 2019b) (Korniejenko et al., 2020c), microspheres (Ciemnicka et al., 2021), various polymers (Łach et al., 2019) (Kozub et al., 2021b) (Gailitis et al., 2021b) etc. Geopolymers may also be reinforced with biological materials, such as hemp (Taye et al., 2021), flax (Bazan et al., 2021) fibers, wooden biomass (Ambrus et al., 2020) or waste materials (such as glass wool) (Kozub et al., 2021b). Rubber and steel fibers recycled from car tires were also investigated as possible additive to geopolymers (Mucsi et al., 2018).
Plasma, an ionized gas exhibiting quasineutrality and collective behaviour, is used for cleaning, etching and activation of surfaces (Yaghoubi and Taghavinia, 2011), thin layer formation and other purposes. Unlike other methods, it does not require high temperatures and aggressive or toxic chemicals like solvents or catalysts (Tendero et al., 2006), and can therefore be used to treat materials with low durability or in medicine (Kowalonek et al., 2010). When used to activate a surface to increase its wettability, reactivity etc., plasma may change the chemical properties of the surface (Tesař, 2008) by depositing functional groups, such as hydroxyl groups, or by changing composition of the surface layer (Tesař, 2008). Plasma may also change the surface morphology by the process of plasma etching, which increases coarseness and surface area (Wang, 2012). If the surface is contaminated, plasma may also be used to clean it. Plasma treatments commonly used for activating and hydrophilizing various types of surfaces, being universal and efficient, while doing no to minimal damage to the surface, especially when compared to treatments using chemicals, such as acids, and leaving no toxic residue (Tesař, 2008). This makes plasma pretreatment a viable method of improving the properties of materials used in medicine, such as scaffolds or implants (Volokhova et al., 2022). Regarding cementitious materials, plasma may be used to enhance their mechanical properties, when used to treat their aggregates or reinforcing elements, such as fibers (Thibodeaux et al., 2021) or fiberglass waste (Lupu et al., 2021).
As geopolymers may be used as an alternative to OPC, they may also be coated or modified with various types of coatings, including penetration coatings, hydrophobic coatings, antimicrobial coatings (Le Chi et al., 2019b) etc. Geopolymer coatings are commonly based on epoxy resin, polyester and vinil ester (Kong et al., 2019) (Nodehi, 2022) However, these coatings, especially when used on industrial scale, may require the geopolymer surface to be hydrophilic and wettable, so that optimal spread and adhesion of the coating is ensured. Geopolymers may also form variable surfaces, depending on their composition, geopolymerization time etc. and may not always be completely wettable. Other properties are also influenced by these parameters (Nodehi et al., 2022). It is also possible to achieve hydrophobic properties in geopolymers, such as by adding hydrophobic reagents into the geopolymer mixture, such as polydimethylsiloxane (Ruan et al., 2021) This study therefore aims to investigate the wettability of various types of geopolymers, including those with high-carbon fly ash (also called coke dust waste) additive (as using geopolymers to encapsulate the fly ash is a potential viable method to dispose of it, but the ash might have negative impact on geopolymer properties), and the influence of CAP (cold atmospheric plasma) treatment on their wettability, to determine the possibility of using CAP to activate geopolymer surface for the purpose of further functionalization by varnishes, chemical treatments etc. A commercial waterproofing varnish (Revacry Ultrafine 4210, 2022) was also tested the possibility of waterproofing various geopolymer materials.
2 Materials and methods
2.1 Materials used
The samples were made using the following materials:
• Baucis LNa, component A (metakaolin) and B (liquid activator), České lupkové závody a. s
• Baucis LK component A (metakaolin) and B (liquid activator), České lupkové závody a. s
• Quartz sand, Sklopísek Střeleč–filler material
• Silica fumes RW-Füller, AMG Silicon–improve mechanical properties and chemical resistance
• Aluminium powder AIPRA, PK Chemie–foaming agent
• Basalt fibers (length 6 mm, width 13 µm) - ORLIMEX CZ, s. r.o.—improve flexural strength
• Revacryl UF 4210 varnish, Synthomer
• Fly ash - Polska Grupa Energetyczna
Baucis LNa and Baucis LK are geopolymer precursor materials made of locally manufactured metakaolin, whose composition is shown in Table 1, as geopolymer base (MEFISTO L05, 2022) and alkaline activator (LNa activator is sodium-based, while LK activator is potassium-based) (Baucis LNa, 2021). Other materials include quartz sand, silica fumes with mean particle size of 0.1–0.3 µm and 96% silicon dioxide content (RW-Füller, 2018) aluminium powder used to foam certain samples, basalt fibers with 6 mm length and 13 µm width and high carbon fly ash. Revacryl Ultrafine 4210, an acrylate polymer water dispersion, is a commercially available waterproofing varnish used for hydrophobization (Revacry Ultrafine 4210, 2022).
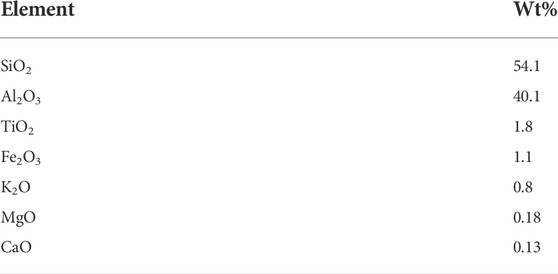
TABLE 1. Metakaolin composition (MEFISTO L05, 2022)
High-carbon fly ash, used as a geopolymer additive, was produced by PGE Polska Grupa Energetyczna and came from an electrostatic precipitator of a coal-fired power station. According to performed analysis, it contains 76.89 wt% of carbon. It also contains oxygen, iron, calcium and other elements (presumably in oxide form). Its elemental composition is shown in Table 2.
2.2 Geopolymer samples
Geopolymer samples were prepared from sodium metakaolin-based geopolymer Baucis LNa and its water glass and sodium hydroxide-based activator, both manufactured by České lupkové Závody a. s (Baucis LNa, 2021). Quartz sand and silica fumes were added to all samples (in accordance with geopolymer recipe used at Technical university of Liberec for research), while the samples differed by other additives. From each set, both compact and foamed samples were prepared, with aluminium powder serving as foaming agent. The base composition of samples without additives is shown in Table 3. In total, 5 sets of samples were prepared. The materials are listed by weight proportions to geopolymer base, which is used as reference. For example, number 1 represents 100% of the weight of geopolymer base.
• First set: Addition of basalt fibers with 0.07 weight proportion to geopolymer base (7% of the mass of geopolymer base).
• Second set: Only the materials specified in Table 3.
• Third set: Addition of high carbon fly ash with 0.16 weight proportion to geopolymer base.
• Fourth set: Addition of high carbon fly ash with 0.32 weight proportion to geopolymer base.
• Fifth set: Base set of materials, potassium-based activator used instead of sodium-based activator.
2.3 Sample preparation
First, the metakaolin geopolymer base and sand were mixed together for 1 min, before adding liquid activator and mixing the paste for 2 min. Then silica fumes the other materials for each respective set were added (first silica fumes, then the other materials) and the paste was mixed for 5 min, for foamed samples, aluminium powder was also added after mixing and the paste was mixed for an additional minute, until a homogenous gel formed, after which it was poured into wooden (for experiments) or plastic (for display) molds, wrapped with polyvinyl sheet and left for 1 day. The samples were then cured at 45°C for 5 h, unwrapped and cured at 82°C for additional 15 h. Samples were then cut to smaller pieces suitable for testing, cleaned with water and dried at 45°C for 5 h. This procedure is standard for geopolymer laboratory at Technical university of Liberec and provides adequate time and curing temperatures for geopolymerization and subsequent drying, while minimizing risk of developing cracks. All tests were performed on both the uncut surface of geopolymer and on the cut surface. Addition of high-carbon fly ash coloured geopolymers into shades of dark grey, as seen in Figure 1. Geopolymer made from potassium base foamed more significantly with the addition of aluminium powder. The difference can be seen in Figure 2.
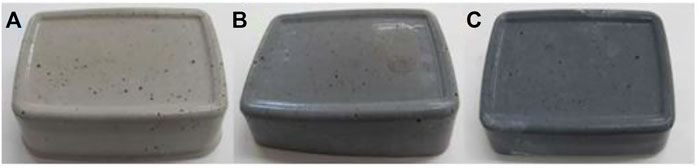
FIGURE 1. Geopolymers with 0 (A), 0.16 (B) and 0.32 (C) of high-carbon fly ash in weight proportion to geopolymer base.
2.4 Plasma pretreatment
For the purpose of testing plasma activation of geopolymer surface, samples were treated with Piezobrush PZ2, which produces cold atmospheric plasma (CAP, nitrogen-oxygen plasma) with temperature below 50°C and operating frequency of 50 kHz (RF equivalent). Its maximum output energy is 10 W. The device was fitted with near-field nozzle used for conductive surfaces, as using the standard nozzle caused visible discharges into the surface of geopolymer. The schematics of near-field nozzle used to treat geopolymer surface is shown in Figure 3. (TDK Electronics, 2022)
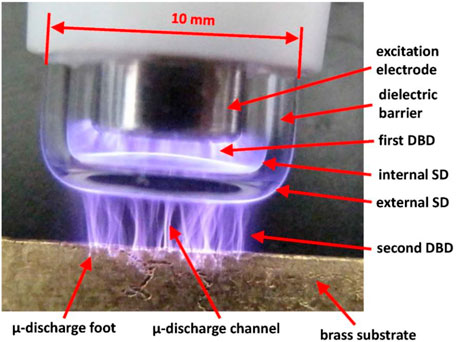
FIGURE 3. Example of Piezobrush PZ2 Near-field nozzle used on conductive surface with Surface discharges (SD) and Dielectric barrier discharges (DBD) denoted (Korzec et al., 2021)
The geopolymer surfaces were first cleared with textile and treated by cold atmospheric plasma jet for 5 s and the surface was treated like this two times, to ensure consistent surface activation. The distance between Piezobrush and sample was around 1 mm or below during surface treatment, as the near-field nozzle does not produce plasma unless in close proximity of the sample. Samples from each set were treated by plasma, both on uncut and cut surface.
2.5 Waterproofing varnish application
Commercially available Revacryl UF 4210, a waterproofing varnish for mineral surfaces, was used to waterproof geopolymer samples. The varnish was applied by a paintbrush and multiple layers were applied to ensure optimal surface coverage and to prevent the geopolymer from penetrating the surface. Samples from all sets and both cut and uncut surfaces were waterproofed.
2.6 Wettability testing
All wettability tests were performed on Surface Energy Evaluation System, a device used to measure the contact angle of a droplet of liquid. In this study, 3.5 µl were used for each measurement. For all samples, the contact angle was measured 3 times during each measurement and mean value was used for calculation. The interval between measurements and number of measurements taken differed for each surface modification or type of surface (uncut or cut). Contact angle was measured at t = 0 as well. The speed of water soaking into the surface was approximated as a change in contact angle in time.
• For untreated and uncut surface, the measurement took 2 min with 20 s intervals and 10 samples were tested to calculate standard deviation calculated in Microsoft Excel. The standard deviation is represented by error bars in Figures 4–8.
• For surface, both cut and uncut, treated with varnish, the measurement took 7 min with 1 min intervals and one sample was tested to verify its waterproofing.
• For cut surface and both cut and uncut surface treated with plasma, the interval was 1 s, contact angle was measured until the droplet soaked into the geopolymer and one sample was tested from each set, to verify the hydrophobic nature of the sample.
3 Results and discussion
In this paper, two main results were produced, first one determined the influence of various geopolymer additives on the wettability of resulting geopolymer composite, with the fly ash being the most influential additive, while the second one determined, and whether geopolymer surface treatment using low-energy, low-temperature atmospheric plasma makes the surface of geopolymers of various composition completely hydrophobic. As a secondary result, commercial waterproofing varnish was also tested on all geopolymer samples.
3.1 Influence of fly ash and basalt fibers on wettability
As seen in Figure 4, addition of basalt fibers in compact geopolymer (geopolymer without added aluminium powder and therefore unfoamed) only marginally quickened soaking of water into the geopolymer when compared to geopolymer without fibers, with the change of initial contact angle being low and within the error margin. However, as seen in Figure 5, foaming the geopolymer containing basalt fibers caused it to retain the wettability of a compact sample, with even slower soaking of water into its surface and similar initial contact angle. Without fibers, however, the geopolymer became more wettable, with nearly 3 times faster soaking and lower initial contact angle. Unlike the compact samples or foamed sample with basalt fibers, the droplet had also soaked into the sample completely before reaching 100 s during some measurements. The effect of basalt fibers is likely caused by hydrophilic and polar properties of basalt fibers (Khandelwal and Yop Rhee, 2020).
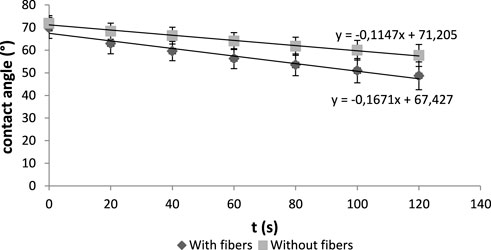
FIGURE 4. Wettability of compact geopolymer with and without basalt fibers (sodium-based geopolymer).
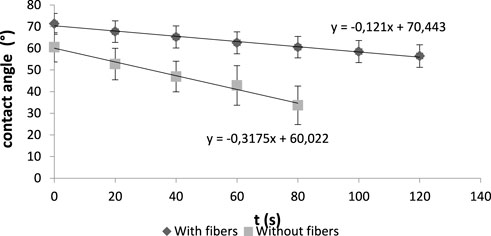
FIGURE 5. Wettability of foamed geopolymer with and without basalt fibers (sodium-based geopolymer).
As seen in Figure 6, adding high-carbon fly ash into the compact geopolymer mix significantly lowered the initial contact angle, but did not accelerate soaking of water into the geopolymer. At 16 % of the weight of geopolymer base, the speed of soaking is nearly identical to the sample with no fly ash. At 32 %, the speed of soaking seemed to be marginally higher. However, as seen in the graph, the difference is within the margin of error for both sets of measurements and may not exist at all. For the foamed samples, as seen in Figure 7, adding fly ash, despite lowering the initial contact angle, slowed down soaking of water into the samples, although higher mass fraction of fly ash caused faster soaking than the smaller mass fraction.
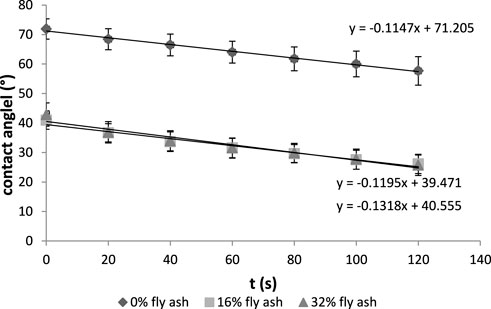
FIGURE 6. Wettability of compact geopolymer with and without high-carbon fly ash (sodium-based geopolymer).
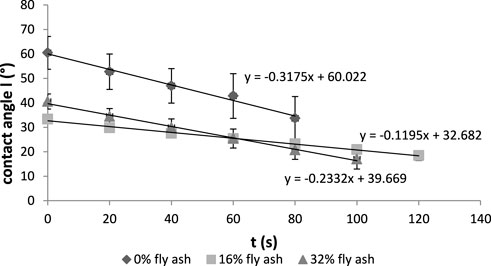
FIGURE 7. Wettability of foamed geopolymer with and without high-carbon fly ash (sodium-based geopolymer).
These effects therefore make high-carbon fly ash a helpful additive, when treating geopolymers with water-based solutions, as it decreases wettability of both compact and foamed geopolymers. As high-carbon fly ash also improves other properties of geopolymer composites, such as thermal resistance or compressive strength in foamed geopolymers (Buczkowska et al., 2020), while not significantly reducing mechanical properties of compact geo-polymers (Dufkova et al., 2020) further confirms it as a useful filler material and deposition method for this kind of waste. These improved properties are likely a result of high surface to volume ratio and “whisker clusters” on the surface of high-carbon fly ash particles, as investigated in different study using the same fly ash (Buczkowska et al., 2020), including the increase in wettability.
3.2 Influence of potassium base
When compared to sodium base, using potassium base to prepare compact geopolymer samples lowered the initial contact angle and slightly increased the speed of soaking into the surface, as seen in Figure 8, while using it to prepare foamed geopolymer samples led to more significant foaming and caused the sample surface to be completely hydrophilic, as water soaked in nearly immediately, similarly to cut surface or surface treated with plasma. Geopolymers made using different activators exhibit different properties, including mechanical, thermal and dielectric properties (El Alouani et al., 2020) (Sitarz et al., 2022).
For all samples, however, the changes were insignificant in the long term applications, such as when using geopolymer as construction material, as the contact angle steadily decreased and water eventually soaked into all of the samples (except those treated by waterproofing varnish) despite some of them being seemingly hydrophobic, as the initial contact angle was nearly 90° during some measurements. All cut surfaces were highly wettable and soaked the water in almost immediately. The contact angle measurements also varied greatly even for samples of the same composition, so using varnishes and other coatings on geopolymer surfaces may not always produce even results.
Geopolymer wettability was therefore influenced mostly by using fly ash and potassium base, with basalt fibers only having small impact on the wettability.
3.3 Influence of plasma treatment on wettability
Treating the geopolymer surface with cold atmospheric plasma (CAP) significantly increased its wettability, with water droplet soaking into the surface in less than 3 s. This result was the same for all samples, with plasma treated cut surface having highest wettability, as it was impossible to even measure the droplet on the surface, as it soaked in nearly instantly.
Plasma activation was therefore successful at making the geopolymer surface hydrophilic by increasing its surface energy, regardless of additives. Effect of plasma treatment therefore matches its effect on other materials and may be used for surface pretreatment and activation (Tesař, 2008). There are two possible explanations for this. Plasma may have changed the surface composition of the surface, as nitrogen plasma is known to nitride the surface by replacing oxygen with nitrogen on the surface (Seino et al., 2002), which may be possible on geopolymer surface, as geopolymers are composed of aluminosilicates, which are composed of oxygen (Tesař, 2008) and deposited reactive groups, such as hydroxyl groups. The other possible explanation is change in the morphology of the surface by etching, as it is possible for plasma be used to etch surfaces, however, it usually requires heavier nonreactive elements, such as argon, to sputter away material from the surface without reacting with it, and CAP only contains roughly 1% of Argon (Plasma, 2021).
3.4 Waterproofing
Applying waterproofing varnish on geopolymer samples prevented water from soaking into them, with the exception of foamed potassium-based geopolymer, as it was impossible to properly coat it due to presence of large cavities. While the contact angle was still getting lower, it can be attributed to water evaporating, rather than soaking into the geopolymer, as the varnish produced uniform layer with no visible pores or cracks, there were no signs of water infiltration on geopolymer itself (surface darkening) and similar results being observed on microscope slide tested by the same method. The initial contact angles were also very similar and there were no observable correlations between surface treatment or additive and the contact angle. Acrylate-based coatings are therefore usable to hydrophobize geopolymers, along with other types of coatings, including methyl silicone resins (Le Chi et al., 2019b) or, potentially, coatings for mineral-based (especially silicone and aluminium oxide based) materials, such as OPC based concrete or stones, due to similar composition to geopolymers (Hussin et al., 2014)
4 Conclusion
Multiple additives were tested for their influence on geopolymer wettability. While there were differences, most of them were insignificant. The only exception was foamed potassium-based geopolymer, which became completely hydrophilic, with contact angle measurement being impossible due to water nearly instantly soaking into geopolymer surface. Using high-carbon fly ash does increase wettability of geopolymers, but does not accelerate soaking of water into them. Commercial waterproofing varnish was successful at making all samples waterproof, although it was also confirmed that without pretreatment, the uncut geopolymer surface exhibits variable wettability, as the contact angle measured varied significantly, even for samples with the same composition, and hydrophobic coatings may not always spread and adhere to the surface evenly.
Cold atmospheric plasma was successful at activating the surface (both cut and uncut) of geopolymer and making it hydrophilic. It is therefore a viable method for pretreatment of geopolymer surfaces before applying water-based varnishes or functionalization solutions, although it was necessary to use special systems for treating conductive surfaces.
In future studies, mechanism of hydrophilization of geopolymers should be investigated, as the effect may have been achieved either by changing the surface composition of geopolymer material, depositing reactive groups (such as hydroxyl groups) or changing the morphology of the surface, as well as by combination of more changes. Influence of high-carbon fly ash additives on geopolymer should also be investigated further, as using it as geopolymer filler and colouring is a potentially viable method to dispose of this kind of waste. Further types of coatings should also be tested to determine their suitability for usage on geopolymers, for example those designed for use on concrete or natural stones.
Data availability statement
The original contributions presented in the study are included in the article/supplementary material, further inquiries can be directed to the corresponding author.
Author contributions
Formal analysis, VR, PJ and PP, Funding acquisition, PL, Investigation, VR, Project administration, PL, Resources, VR and JC, Supervision, PL and KB, Visualization, KP, Writing–original draft, VR, Writing–review and editing, VR and KB. All authors contributed to manuscript revision, read, and approved the submitted version.
Funding
This publication was written at the Technical University of Liberec, Faculty of Mechanical Engineering with the support of the Institutional Endowment for the Long Term Conceptual Development of Research Institutes, as provided by the Ministry of Education, Youth and Sports of the Czech Republic in the year 2022. This work was supported by the Ministry of Education, Youth and Sports of the Czech Republic and the European Union–European Structural and Investment Funds in the frames of Operational Programme Research, Development and Education–project Hybrid Materials for Hierarchical Structures (HyHi, Reg. No. CZ.02.1.01/0.0/0.0/16_019/0000843). This work was partly supported by the Student Grant Scheme at the Technical University of Liberec through project nr. SGS-2022-5066.
Conflict of interest
The remaining authors declare that the research was conducted in the absence of any commercial or financial relationships that could be construed as a potential conflict of interest.
The handling editor KK declared a past co-authorship with the author PL.
Publisher’s note
All claims expressed in this article are solely those of the authors and do not necessarily represent those of their affiliated organizations, or those of the publisher, the editors and the reviewers. Any product that may be evaluated in this article, or claim that may be made by its manufacturer, is not guaranteed or endorsed by the publisher.
References
Abbas, A.-G. N., Aziz, F. N. A. A., Abdan, K., Nasir, N. A. M., and Huseien, G. F. (2022). A state-of-the-art review on fibre-reinforced geopolymer composites. Constr. Build. Mat. 330, 127187. doi:10.1016/j.conbuildmat.2022.127187
Ambrus, M., Papné Halyag, N., Czupy, I., Szalay, D., and Mucsi, G. (2020). Mechanical and structural properties of biomass-geopolymer composites. Geosci. Engin. 8 (12), 47–60.
Ambrus, M., Szabó, R., and Mucsi, G. (2019). Utilisation and quality management of power plant fly ash. Int. J. Eng. Manag. Sci. 4, 329–337. doi:10.21791/IJEMS.2019.4.37
Baucis LNa (2021). České lupkové závody a.s. Available at: https://www.cluz.cz/cz/baucis-lna (accessed 2 17, 2021).
Bazan, P., Kozub, B., Korniejenko, K., Gailitis, R., and Sprince, A. (2021). Tribo-mechanical behavior of geopolymer composites with wasted flax fibers. IOP Conf. Ser. Mat. Sci. Eng. 1190, 012030. doi:10.1088/1757-899X/1190/1/012030
Buczkowska, K., Le, C. H., Louda, P., Michał, S., Bakalova, T., Tadeusz, P., et al. (2020). The fabrication of geopolymer foam composites incorporating coke dust waste. Processes 8, 1052. doi:10.3390/pr8091052
Ciemnicka, J., Prałat, K., Koper, A., Makomaski, G., Majewski, Ł., Wójcicka, K., et al. (2021). Changes in the strength properties and phase transition of gypsum modified with microspheres, aerogel and HEMC polymer. Materials 14, 3486. doi:10.3390/ma14133486
Dufkova, I., Kovacic, V., and Kejzlar, P. (2020). Mechanical properties of geopolymer filled with from coke production, 591–595. doi:10.37904/nanocon.2019.8786
Dufková, I., Kovačič, V., and Kejzlar, P. (2020). “Mechanical properties of geopolymer filled with from coke production,” in Nanocon 2019 conference proeedings (Brno, Czech Republic: Tanger Ltd). Nanocon 2019. doi:10.37904/Nanocon.2019.8786
El Alouani, M., Alehyen, S., el Achouri, M., Hajjaji, A., Ennawaoui, C., and Taibi, M. (2020). Influence of the nature and rate of alkaline activator on the physicochemical properties of fly ash-based geo-polymers. Adv. Civ. Eng. 2020, 1–13. doi:10.1155/2020/8880906
Gailitis, R., Korniejenko, K., Sprince, A., and Pakrastins, L. (2020a). Comparison of the long-term properties of foamed concrete and geopolymer concrete in compression. AIP Conf. Proc. 2239, 020012. doi:10.1063/5.0007787
Gailitis, R., Sliseris, J., Korniejenko, K., Mikuła, J., Łach, M., Pakrastins, L., et al. (2020b). Long-term deformation properties of a carbon-fiber-reinforced alkali-activated cement composite. Mech. Compos. Mat. 56, 85–92. doi:10.1007/s11029-020-09862-w
Gailitis, R., Sprince, A., Kozlovskis, T., Radina, L., Pakrastins, L., and Vatin, N. (2021b). Long-term properties of different fiber rein-forcement effect on fly ash-based geopolymer composite. Crystals 11, 760. doi:10.3390/cryst11070760
Gailitis, R., Sprince, A., Pakrastins, L., Bazan, P., and Korniejenko, K. (2021a). Plain and PVA fibre-reinforced geopolymer compact tension specimen critical area surface composition. Environ. Technol. Resour. Proc. Int. Sci. Pract. Conf. 3, 72–77. doi:10.17770/etr2021vol3.6569
Heah, C. Y., Liew, Y. M., Mohd, M. A. B. A., and Kamarudin, H. (2017). Thermal resistance variations of fly ash geopolymers: Foaming responses. Sci. Rep. 7, 45355. doi:10.1038/srep45355
Hussin, M., Ramadhansyah, P. J., Mirza, J. R., Ariffin, M. A., and Bhutta, A. (2014). Performance of blended ash geopolymer concrete at elevated temperatures. Mat. Struct. 48, 709–720. doi:10.1617/s11527-014-0251-5
Khandelwal, S., and Yop Rhee, K. (2020). Recent advances in basalt-fiber-reinforced composites: Tailoring the fiber-matrix interface. Compos. Part B Eng. 192, 108011. ISSN 1359-8368. doi:10.1016/j.compositesb.2020.108011,
Kong, L., Fang, J., and Zhang, B. (2019). Effectiveness of surface coatings against intensified sewage corrosion of concrete. J. Wuhan. Univ. Technol. -Mat. Sci. Ed. 34, 1177–1186. doi:10.1007/s11595-019-2175-y
Korniejenko, K., Łach, M., Chou, S.-Y., Lin, W.-T., Cheng, A., Hebdowska-Krupa, M., et al. (2020d). Mechanical properties of short fiber-reinforced geopolymers made by casted and 3D printing methods: A comparative study. Materials 13, 579. doi:10.3390/ma13030579
Korniejenko, K., and Łach, M. (2020). Geopolymers reinforced by short and long fibres – innovative materials for additive manufacturing. Curr. Opin. Chem. Eng. 28, 167–172. doi:10.1016/j.coche.2020.06.005
Korniejenko, K., Łach, M., Mikuła, J., Hebdowska-Krupa, M., Mierzwiński, D., Gądek, S., et al. (2020a). Development of 3D printing Technology for geopolymers. Eb. Proc. 1. doi:10.23967/dbmc.2020.033
Korniejenko, K., Mierzwiński, D., Szabó, R., Halyag, N. P., Louda, P., Thorhallsson, E. R., et al. (2020c). The impact of the curing process on the efflorescence and mechanical properties of basalt fibre reinforced fly ash-based geopolymer composites. MATEC Web Conf. 322, 01004. doi:10.1051/matecconf/202032201004
Korniejenko, K., Mucsi, G., Halyag, N. P., Szabó, R., Mierzwiński, D., and Louda, P. (2020b). Mechanical properties of basalt fiber reinforced fly ash-based geopolymer composites. KnE Eng., 86–100. doi:10.18502/keg.v5i4.6800
Korzec, D., Hoppenthaler, F., and Nettesheim, S. (2021). Piezoelectric direct discharge: Devices and applications. Plasma 4, 1–41. doi:10.3390/plasma4010001
Kowalonek, J., Kaczmarek, H., and Dąbrowska, A. (2010). Air plasma or UVirradiation applied to surface modification of pectin/poly(vinyl alcohol) blends. Appl. Surf. Sci. 257 (1), 325–331. doi:10.1016/j.apsusc.2010.07.005
Kozub, B., Bazan, P., Gailitis, R., Korniejenko, K., and Mierzwiński, D. (2021b). Foamed geopolymer composites with the addition of glass wool waste. Materials 14, 4978. doi:10.3390/ma14174978
Kozub, B., Bazan, P., Mierzwiński, D., and Korniejenko, K. (2021a). Fly-ash-based geopolymers reinforced by melamine fibers. Materials 14, 400. doi:10.3390/ma14020400
Kristály, F., Szabó, R., Mádai, F., Debreczeni, Á., and Mucsi, G. (2020). Lightweight composite from fly ash geo-polymer and glass foam. J. Sustain. Cement-Based Mater. 10, 1–22. doi:10.1080/21650373.2020.1742246
Łach, M., Hebdowska-Krupa, M., Mierzwiński, D., and Korniejenko, K. (2019). Mechanical properties of geopolymers reinforced with carbon and aramid long fibers. IOP Conf. Ser. Mat. Sci. Eng. 706, 012011. doi:10.1088/1757-899X/706/1/012011
Łach, M., Mierzwiński, D., Korniejenko, K., and Mikuła, J. (2018). Geopolymer foam as a passive fire protection. MATEC Web Conf. 247, 00031. doi:10.1051/matecconf/201824700031
Le Chi, H., Hájková, P., Van, S. L., Louda, P., and Voleský, L. (2019b). Water absorption properties of geopolymer foam after being impregnated with hydrophobic agents. Materials 12, 4162. doi:10.3390/ma12244162
Le Chi, H., Louda, P., Ewa Buczkowska, K., and Dufkova, I. (2021). Investigation on flexural behavior of geopolymer-based carbon textile/basalt fiber Hybrid composite. Polymers 13, 751. doi:10.3390/polym13050751
Le Chi, H., Louda, P., Le Van, S., Volesky, L., Kovacic, V., and Bakalova, T. (2019a). Composite performance evaluation of basalt textile-reinforced geopolymer mortar. Fibers 7, 63. doi:10.3390/fib7070063
Le, V. S., Szczypinski, M., Hájková, P., Kovacic, V., Bakalova, T., Volesky, L., et al. (2020a). Mechanical properties of geopolymer foam at high temperature. Sci. Eng. Compos. Mater. 27, 129–138. doi:10.1515/secm-2020-0013
Le, V. S., and Louda, P. (2021). Research of curing time and temperature-dependent strengths and fire resistance of geopolymer foam coated on an aluminum plate. Coatings 11, 87. doi:10.3390/coatings11010087
Le, V. S., Louda, P., Tran, H. N., Nguyen, P. D., Bakalova, T., Ewa Buczkowska, K., et al. (2020b). Study on temperature-dependent properties and fire resistance of metakaolin-based geopolymer foams. Polymers 12, 2994. doi:10.3390/polym12122994
Lupu, M. L., Isopescu, D. N., Tuns, I., Baciu, I.-R., and Maxineasa, S. G. (2021). Determination of physicomechanical characteristics of the cement mortar with added fiberglass waste treated with hydrogen plasma. Materials 14, 1718. doi:10.3390/ma14071718
MEFISTO L05 (2022). MEFISTO L05. Available at: https://www.cluz.cz/en/mefisto-l05 (accessed 3 1, 2022).
Mucsi, G., Szenczi, Á., and Nagy, S. (2018). Fiber reinforced geopolymer from synergetic utilization of fly ash and waste tire. J. Clean. Prod. 178, 429–440. doi:10.1016/j.jclepro.2018.01.018
Nenadović, S. S. S., Kljajević, L. M., Ivanović, M. M., Mirković, M. M., Radmilović, N., Rakočević, L. Z., et al. (2021). Structural and chemical properties of geopolymer gels incorporated with neodymium and samarium. Gels 7, 195. doi:10.3390/gels7040195
Nguyen, V. V., Le, V. S., Louda, P., Szczypiński, M. M., Ercoli, R., Vojtěch, R., et al. (2022). Low-density geopolymer composites for the construction industry. Polymers 14, 304. doi:10.3390/polym14020304
Nodehi, M. (2022). Epoxy, polyester and vinyl ester based polymer concrete: a review. Innov. Infrastruct. Solut. 7, 64. doi:10.1007/s41062-021-00661-3
Nodehi, M., Ozbakkaloglu, T., Gholampour, A., Mohammed, T., and Shi, X. (2022). The effect of curing regimes on physico-mechanical, microstructural and durability properties of alkali-activated materials: A review. Constr. Build. Mater. 321, 126335. doi:10.1016/j.conbuildmat.2022.126335
Plasma (2021). Plasma etching. Available at: https://www.plasma.com/en/applications/plasma-etching/(accessed May 9, 2021).
Prałat, K., Ciemnicka, J., Koper, A., Buczkowska, K. E., and Łoś, P. (2021). Comparison of the thermal properties of geopolymer and modified gypsum. Polymers 13, 1220. doi:10.3390/polym13081220
Ranjbar, N., and Zhang, M. (2019). Fiber reinforced geopolymer composites: A review. Cem. Concr. Compos. 107, 103498. doi:10.1016/j.cemconcomp.2019.103498
Revacry Ultrafine 4210 (2022). Revacryl™Ultrafine 4210 technical sheet. Available at: https://www.synthomer.com/fileadmin/files/tds/REVACRYL%20ULTRAFINE%204210%20(Coatings).pdf/(accessed January 16, 2022).
Ruan, S., Chen, S., Zhu, X., Zeng, Q., Liu, Y., Lai, J., et al. (2021). Matrix wettability and mechanical properties of geopolymer cement-polydimethylsiloxane (PDMS) hybrids. Cem. Concr. Compos. 124, 104268. doi:10.1016/j.cemconcomp.2021.104268
Růžek, V. (2020). Vliv spalinových příměsí a plazmatické úpravy na povrchové vlastnosti geopolymerů. Diploma thesis. Liberec, Czech Republic: Technical University of Liberec.
RW-Füller (2018). Technical data sheet. SiO2, RW-Füller. Available at: https://www.silicium.de/fileadmin/user_upload/Produktspezifikation_RWF_QSt_11_Rev._11_23.07.18.pdf.
Seino, T., Matsuura, T., and Murota, J. (2002). Atomic-order nitridation of SiO2 by nitrogen plasma. Surf. Interface Anal. 34, 451–455. doi:10.1002/sia.1336
Sitarz, M., Castro-Gomes, J., and Hager, I. (2022). Strength and microstructure characteristics of blended fly ash and ground granulated blast furnace slag geopolymer mortars with Na and K silicate solution. Materials 15, 211. doi:10.3390/ma15010211
Taye, E. A., Roether, J. A., Schubert, D. W., Redda, D. T., and Boccaccini, A. R. (2021). Hemp fiber reinforced red mud/fly ash geo-polymer composite materials: Effect of fiber content on mechanical strength. Materials 14, 511. doi:10.3390/ma14030511
TDK Electronics (2022). Cold plasma from a single component. Available at: https://www.tdk-electronics.tdk.com/en/373562/tech-library/articles/applications-cases/applications-cases/cold-plasma-from-a-single-component/1109546 (accessed January 12 2022).
Tendero, C., Tixier, C., Tristant, P., Desmaison, J., and Leprince, P. (2006). Atmospheric pressure plasmas: A review. Spectrochim. Acta Part B At. Spectrosc. 61 (1), 2–30. doi:10.1016/j.sab.2005.10.003
Tesař, J. (2008). Plazmochemická aktivace povrchu skla v povrchovém výboji ve vzduchu za atmosférického tlaku. Bachelor thesis. Brno: Masaryk university.
Thibodeaux, N., Guerrero, D. E., Lopez, J. L., Bandelt, M. J., and Adams, M. P. (2021). Effect of cold plasma treatment of polymer fibers on the mechanical behavior of fiber-reinforced cementitious composites. Fibers 9, 62. doi:10.3390/fib9100062
Trinh, Q. V., Mucsi, G., Dang, T. V., Le, L. P., Bui, V. H., and Nagy, S. (2020). The influence of process conditions on ground coal slag and blast furnace slag based geopolymer properties. Rudarsko-geološko-naftni Zb. 35, 15–20. doi:10.17794/rgn.2020.4.2
Tsai, Y., Hanna, J. V., Lee, Y., Smith, M. E., and Chan, J. C. C. (2010). Solid-state NMR study of geopolymer pre-pared by sol–gel chemistry. J. Solid State Chem. 183 (12), 3017–3022. ISSN 0022-4596. doi:10.1016/j.jssc.2010.10.008
Volokhova, A. A., Fedorishin, D. A., Khvastunova, A. O., Spiridonova, T. I., Kozelskaya, A. I., Kzhyshkowska, J., et al. (2022). Reactive magnetron plasma modification of electrospun PLLA scaffolds with incorporated chloramphenicol for controlled drug release. Polymers 14, 373. doi:10.3390/polym14030373
Wang, J. H. (2012). Surface preparation techniques for biomedical applications, Coatings for Biomedical Applications. Sawston, United Kingdom: Woodhead Publishing, 143–175. ISBN 9781845695682. doi:10.1533/9780857093677.1.143
Keywords: geopolymer, plasma, surface treatment, high-carbon fly ash, coke dust waste, wettability
Citation: Růžek V, Louda P, Buczkowska K, Just P, Prałat K, Ciemnicka J and Przemysław P (2022) Modifying geopolymer wettability by plasma treatment and high-carbon fly ash. Front. Built Environ. 8:991496. doi: 10.3389/fbuil.2022.991496
Received: 11 July 2022; Accepted: 03 August 2022;
Published: 31 August 2022.
Edited by:
Kinga Korniejenko, Cracow University of Technology, PolandReviewed by:
Romisuhani Binti Ahmad, Universiti Malaysia Perlis, MalaysiaRoland Szabó, University of Miskolc, Hungary
Copyright © 2022 Růžek, Louda, Buczkowska, Just, Prałat, Ciemnicka and Przemysław. This is an open-access article distributed under the terms of the Creative Commons Attribution License (CC BY). The use, distribution or reproduction in other forums is permitted, provided the original author(s) and the copyright owner(s) are credited and that the original publication in this journal is cited, in accordance with accepted academic practice. No use, distribution or reproduction is permitted which does not comply with these terms.
*Correspondence: Vojtěch Růžek, dm9qdGVjaC5ydXpla0B0dWwuY3o=