- 1Department of Biology, University of British Columbia, Kelowna, BC, Canada
- 2Independent researcher, Revelstoke, BC, Canada
- 3Miistakis Institute, Mount Royal University, Calgary, AB, Canada
- 4Western Transportation Institute, Montana State University, Bozeman, MT, United States
Wildlife exclusion fencing has become a standard component of highway mitigation systems designed to reduce collisions with large mammals. Past work on the effectiveness of exclusion fencing has relied heavily on control–impact (i.e., space-for-time substitutions) and before–after study designs. These designs limit inference and may confound the effectiveness of mitigation with co-occurring process that also changes the rate of collisions. We used a replicated (n = 2 sites monitored for over 1000 km years combined) before-after-control-impact study design to assess fencing effectiveness along the Trans-Canada Highway in the Rocky Mountains of Canada. We found that collisions declined for common ungulates species (elk, mule deer, and white-tailed deer) by up to 96% but not for large carnivores. The weak response of carnivores is likely due to the combination of fence intrusions and low sample sizes. We calculated realized fencing effectiveness by applying the same change in collision rates observed at control (unfenced) sites as the expected change for adjacent fenced sections. Compared with the apparent fencing effectiveness (i.e., the difference in WVCs rates before and after fencing was installed), the realized estimates of fencing effectiveness declined by 6% at one site and increased by 10% at another site. When factoring in the cost of ungulate collisions to society, fencing provided a net economic gain within 1 year of construction. Over a 10-year period, fencing would provide a net economic gain of > $500,000 per km in reduced collisions. Our study highlights the benefits of long-term monitoring of road mitigation projects and provides evidence of fencing effectiveness for reducing wildlife–vehicle collisions involving large mammals.
Introduction
Roads are a ubiquitous feature of human activity across the earth, with ~21 million km built as of 2018 and upwards of an additional 25 million km of new roads developed by 2050 (Dulac, 2013; Meijer et al., 2018). Central to commerce, resource extraction, and human connectivity, roads also induce several negative impacts on biodiversity. These impacts include barrier effects to animal movement (Epps et al., 2005; Jaeger et al., 2005; Ford and Fahrig, 2008), changes to ecological interactions (Whittington et al., 2011; Dickie et al., 2017), increased access by people to intact habitats (Laurance et al., 2009; Carter et al., 2020), and increase mortality from wildlife–vehicle collisions (WVCs) (Trombulak and Frissell, 2000; Ford and Fahrig, 2007; Clevenger et al., 2015). WVCs not only harm biodiversity but they can also jeopardize human safety, particularly for larger species such as ungulates. For example, 2–3% of WVCs involving deer result in human injury, and this increases to ~20% for WVCs involving larger species such as moose (Huijser et al., 2009).
To counteract the negative effects of WVCs on wildlife and people, transportation and wildlife agencies have employed several mitigation strategies and technologies. These strategies include reduced speed limits (Riginos et al., 2019), road closures (Lamb et al., 2018; Whittington et al., 2019), road density planning (Carter et al., 2020), and technologies such as warning signs for drivers (Huijser et al., 2015). One of the more common tools is wildlife-exclusion fencing, which is designed to prevent large animals from accessing the road right-of-way (but see Ford and Clevenger, 2019). Fence designs vary—and may include shorter “drift” fences for amphibians (Boyle et al., 2021) and taller wire fences designed for large mammals (Ford et al., 2011). Fencing may be accompanied by wildlife crossing structures, which are intended to maintain or restore the safe movement of wildlife across the highway via underpasses or overpasses. Because of its cost, fencing is usually prioritized along particular road segments or “hotspots” of WVCs (Ford et al., 2011; Huijser et al., 2016; Lee et al., 2020).
Despite the widespread use of technologies such as fencing and crossing structures, monitoring and evaluation of mitigation often suffer from lack of experimental control. For example, study designs may compare WVC rates before and after the construction of mitigation (e.g., Clevenger et al., 2001); however, as wildlife populations fluctuate over time independently of road mitigation, such a before–after (“BA”) design may confound changes in the wildlife population with mitigation effectiveness. Likewise, study designs that compare mitigation across nearby areas (i.e., control–impact or CI) may confound local variation in wildlife population size, road design, or driver behavior with mitigation (Rytwinski et al., 2015). Indeed the biophysical process that contribute to WVCs are highly localized even for similar species within a region or watershed (Clevenger et al., 2015; Lee et al., 2020).
To support evidence based approaches to the design and management of road mitigation, it is critical that more rigorous, quantitative approaches be used to assess mitigation effectiveness (Rytwinski et al., 2016). Upon experimental testing, putatively effective technologies may not work as intended at the scales or intensity needed to reduce WVC rates. For example, vehicle speed reductions did not affect WVCs involving deer in Wyoming (Riginos et al., 2019). Similarly, a meta-analysis indicated that wildlife reflector systems are equivocal in their effect on WVC reductions for large mammals, whereas crossings and fencing have a strong negative effect on WVCs across studies (Rytwinski et al., 2016). In addition, meta-analysis revealed that studies using BA and combined BACI (before-after-control-impact) designs detected a stronger effect of mitigation on WVC rates than CI studies alone (Rytwinski et al., 2016). This suggests that opportunities to assess road mitigation through BACI designs are not only more rigorous (Boyle et al., 2021) but also likely to reduce the probability of a Type I error (e.g., there is no effect of mitigation per se but some unmeasured process that led to the decline in WVCs following mitigation) and Type II error (i.e., there is an effect of mitigation on WVCs, but it was not detected).
The Bow Valley of Alberta, Canada includes some of the most well-studied and long-term monitoring of WVCs and road mitigation (Ford et al., 2010; Lee et al., 2012; Clevenger et al., 2015). Spurred by the upgrade of Canada’s major transportation corridor (the Trans-Canada Highway, i.e., the “TCH”) in the 1970s, coupled with a hyperabundant elk population, Banff National Park undertook one of the earliest and most expansive mitigation efforts in the world. Beginning with a series of underpasses and wildlife-exclusion fencing, this system of mitigation expanded along a series of phases (~15–30 km each) from the early 1980s to the present day (Ford et al., 2010). This phased approach has enabled managers to prioritize higher risk road segments for mitigation (e.g., Ford et al., 2011; Lee et al., 2020), but it—along with long-term monitoring—has also facilitated opportunistic field experiments in road ecology. For example, Ford and Clevenger (2010) used a BACI design across highway mitigation phases to test the prey-trap hypothesis, that is, that wildlife crossing structures facilitate predation by large carnivores.
Here, we used an opportunistic field experiment to employ a BACI-design to measure the effectiveness of wildlife-exclusion fencing at reducing large mammal WVCs. We integrate long-term monitoring of WVCs (Gunson et al., 2009; Clevenger et al., 2015) over a 12- to 15-year period covering in two regions of the TCH. We focused on the occurrence of ungulate and large carnivore WVCs. Building on published values that assess the cost of a WVCs to society in terms of property damage, injury, and lost opportunity costs of an animal (Huijser et al., 2009; Ford et al., 2011), we also measured the number of years from the completion of mitigation to reach a cost recovery in reduced WVCs.
Methods
The TCH runs along the Bow River Valley and is a major east–west aligned transportation corridor spanning from Atlantic to Pacific coast. In Alberta, the TCH traverses the Bow River watershed on the east side of the Continental Divide. The TCH is a four-lane highway with an estimated annual average daily traffic (AADT) volume of 10,000–20,000 vehicles per day, with peaks of more than 30,000 vehicles per day during summer (Highway Service Center, Parks Canada, unpublished data; Edwards et al., 2022) during the study period. In addition to the TCH, the Bow River Valley includes portions of the Canadian Pacific Railway, outlying commercial areas (ski hills and golf courses), and the towns of Lake Louise, Banff, Canmore, and Dead Man’s Flats (Barrueto et al., 2014). In general, the Bow River Valley represents high-quality, low-elevation wildlife habitat in this mountain landscape. The region is a vital movement corridor for large mammals, including grizzly bears (Ursus artcos), wolves (Canis lupus) elk (Cervus elaphus), deer (Odocoileus sp.), moose (Alces alces), and Rocky Mountain bighorn sheep (Ovis canadensis).
Within the Bow River watershed, we assess the performance of mitigation measures to reduce WVCs in two study areas of the TCH based on biophysical transition, Banff National Park (BNP) and Dead Man’s Flats (DMF) (Figure 1). BNP is characterized by higher elevation, greater precipitation, and lower human population density compared with the DMF study area.
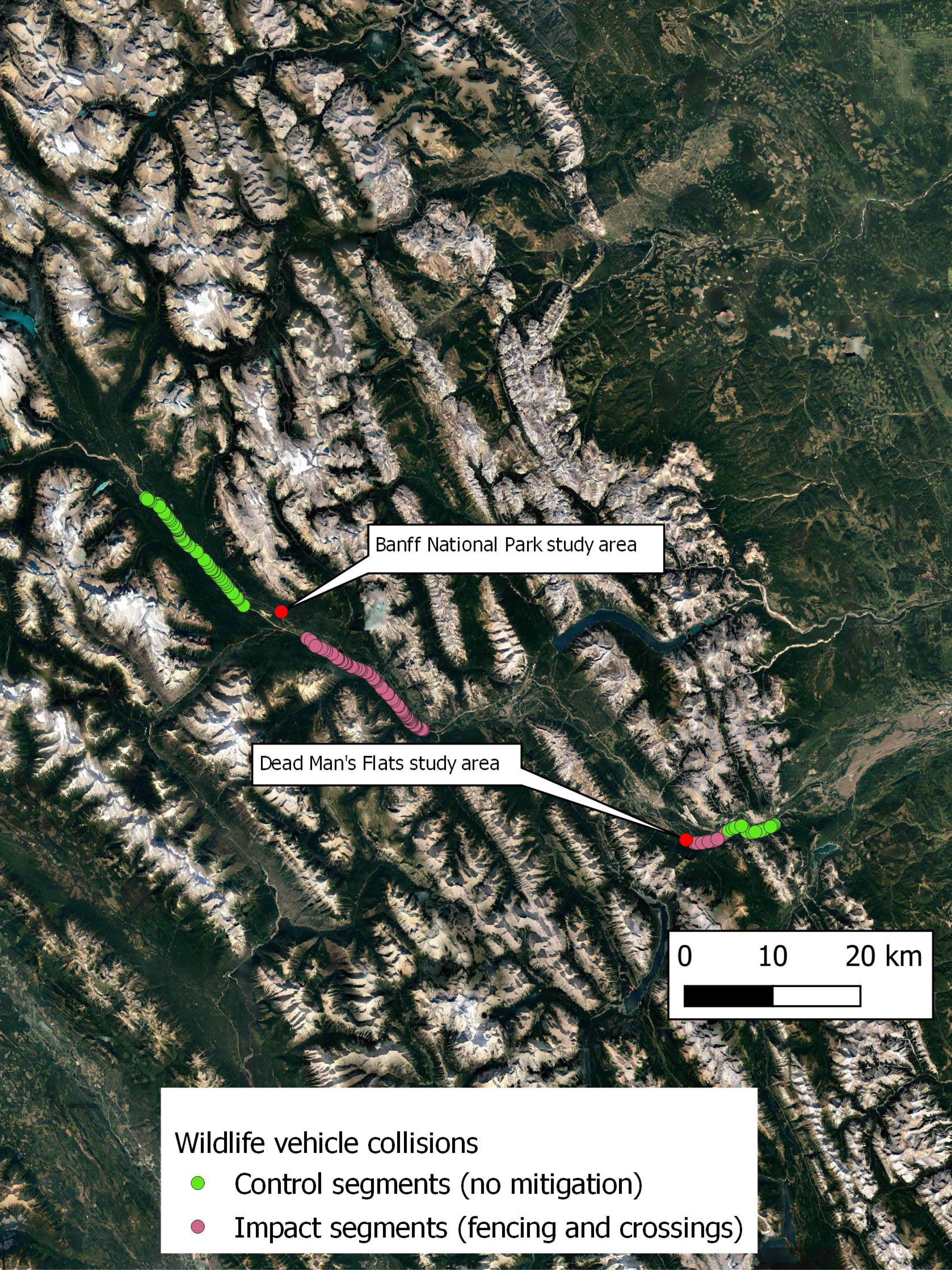
Figure 1 Map showing the location of wildlife vehicle collisions along the Trans-Canada Highway in Banff National Park [top] and Dead Mans Flats [bottom], AB, Canada between 1998 and 2010. Green points represent the location of collisions in the control section and purple points are the location of collisions in the impact section.
Field data collection
WVC data were collected year-round as part of routine wildlife observation reporting by staff from BNP. Each accident site was visited, and the date of the WVC was recorded along with information about the species and the number of individuals. For each WVC location, a Universal Transverse Mercator (UTM) coordinate was obtained using a handheld, global positioning system (GPS) unit accurate to < 5 m (Gunson et al., 2009). For the DMF study area, WVC data were synthesized from four provincial datasets over a 10-year period (Lee et al., 2012). These data were not systematically collected (i.e., scheduled transects) but based on opportunistic data entry from a combination of highway maintenance contractors, provincial biologists, and the public.
In BNP, both the unfenced (i.e., control) and impact (i.e., fenced) segments of the highway were 17-km long. We did not analyze buffer zones around fence ends for potential fence end effects, because the control segment was at least 3 km from any fence end during the entire analysis period. As a result, 34 km of highway was analyzed during a 12-year period (6 years before and 6 years after). Wildlife-exclusion fencing required 3 years to completely install, so those years were excluded from the analysis for both the fenced and unfenced segments. Fenced sections included a number of wildlife underpasses (Ford et al., 2009). For each of 6 years prior to mitigation (November 1988–November 1994) and 6 years following mitigation (November 1998–November 2003), data were compiled by species and by kilometer.
In DMF, mitigation measures consisted of a wildlife underpass flanked by 1.5 km of fencing to the east and 4 km of fencing to the west, with fencing constructed on both sides of the highway (Lee et al., 2012). We compiled counts of WVCs for each of the 6 years prior to mitigation (1998–2004) and 6 years following mitigation (2005–2010). The “impact” segment was defined as the portion of highway associated with the underpass and fencing described above as well as a buffer zone extending 1 km beyond the east end of the fence to account for potentially heightened WVCs at the fence end (Huijser et al., 2016). The western end is contiguous with an existing fence and therefore was not assigned as a buffer zone. WVC data were compiled for this 4-km fenced segment and a contiguous 6-km unmitigated control segment to the east.
For both the BNP and DMF sections, fencing accompanied crossing structures installed below the road surface. The DMF section was more urbanized and had a higher speed limit (110 km/h) and at lower elevation than the BNP section. This lower elevation is typically associated with lower snow accumulation and changes in species abundance, as determined by WVC records. The BNP section was inside a national park, had a lower speed limit (90 km/h), and parks’ staff are assigned to help reduced WVCs in the event of a fence intrusion by an animal onto the right of way. Thus, while we view these two sites as “replicates” along the same stretch of highway, within the same biophysical valley, there are some variables that we cannot control from an experimental perspective. Variance caused by these differences are addressed in the mixed effects model structure described below. Likewise, the “control” sections in this analysis are not actual controls in a true experimental sense. For example, the fenced segments likely had higher WVC rates (i.e., higher risk) so were prioritized for fencing ahead of lower risk segments that we adopted as control for our study.
Data analysis
Collision data were annualized and assigned to one of four experimental groups: before-control, after-control, before-impact, and after-impact. The control segments were not fenced during the study period; the impact segments were fenced in the “after” period only. We separately analyzed elk, mule deer, and white-tailed deer, as well as separate analyses for pooled ungulates and pooled carnivores. The pooled ungulate analysis included elk, mule deer, white-tailed deer and taxa which occurred too infrequently to be analyzed independently: unidentified deer species, moose, and bighorn sheep. Similarly, we pooled carnivores because low numbers across experimental groups made species-specific analyses impossible in the mixed-effect modeling framework that we used.
We used a Poisson mixed effects models to analyze these data. We first confirmed that the count data (collisions per year per segment) were not overdispersed or had too many zeros using the functions testDispersion() and testZeroInflation() in the R package, DHARMa (v 0.4.5). We used the default settings for both functions. Test statistics that were not significant (P > 0.10) were considered not to be overdispersed or zero inflated. We used a main-effects factor for the fenced versus unfenced road segment (i.e., “road segment”), a main-effects factor for the before versus after period (i.e., “time sequence”), and an interaction term between road segment and time sequence. We included study area (i.e., BNP or DMF) as a random intercept to account for variation among study areas (e.g., elevation, traffic volume, and vehicles), with an autoregressive (AR1) term nested within study area to account for temporal autocorrelation. We included an offset term for effort, that is, the number of kilometers sampled in each of the four experimental groups.
We report on apparent and realized measures of fencing effectiveness. Apparent effectiveness is the difference in WVCs prior to and following mitigation for the fenced road segment only. Realized effectiveness first calculates the difference in WVCs prior to and following mitigation for the control sections and applies that difference to the change in WVCs prior to and following mitigation for the fenced sections. For example, consider that there were 10 fewer WVCs along a section of road following the installation the fencing and three fewer WVCs along an adjacent unfenced section during that same period. In this example, the apparent effectiveness is 10 and the realized effectiveness is 7.
For the cost-benefit analysis, we assigned elk, moose, sheep, and deer species a cost-per-collision based on Huijser et al. (2009). We summed these costs for each road segment. We then examined the cost of fencing based published values for this area ($60/m; Ford et al., 2011). We then calculated the annual reduction in collision costs caused by mitigation and any changes in collision costs for unfenced segments. Finally, we estimated the number of years to cost recovery for fencing by dividing the total cost of fencing by the annualized cost reduction in collisions.
Results
We compiled the data from 376 WVCs, including 151 from the two control road segments and 225 from the two impact segments (Table 1). Most WVCs (92%) involved ungulates, and 43% of all ungulate collisions involved elk. Among 31 carnivore WVCs, most (65%) were black bears. Both study areas had comparable total WVCs (i.e., BNP = 183; DMF = 193); however, when accounting for road length of each study area and years of monitoring, DMF had 1.06–3.63 collisions per km per year (for control and impact road segments, respectively), whereas BNP had 0.33–0.85 collisions per km per year (for control and impact road segments, respectively) prior to mitigation. The impact road segments had roughly twice the rate of WVCs compared with the control segments in both study areas. Following mitigation, there were 1.25 WVCs per km per year for fenced segments at DMF and 0.06 WVCs per km per year for fenced segments in BNP.
At a species-specific level, there was a significant effect of the interaction between road segment (control vs. impact) and time sequence (before vs. after) for elk, mule deer, and white-tailed deer (Figure 2 and Table 2). Across all species and study areas, there was a 2% increase (from 0.50 to 0.52 WVCs per km per year) in WVCs from the before to the after period for unfenced control segments. In contrast, there was a 78% reduction in WVCs following fencing segments (from 1.32 to 0.28 WVCs per km per year). For ungulates specifically, there was a 6% decrease in WVCs BNP (0.33–0.31 WVCs per km per year) and a 9% increase in WVCs in DMF (0.86–0.94 WVCs per km per year) between the before and after periods at control segments. For ungulates on fenced highway segments, mitigation was associated with a 96% decrease in the BNP study area (0.96–0.04 WVCs per km per year) and a 73% decrease in the DMF study area (3.50–0.96 WVCs per km per year).
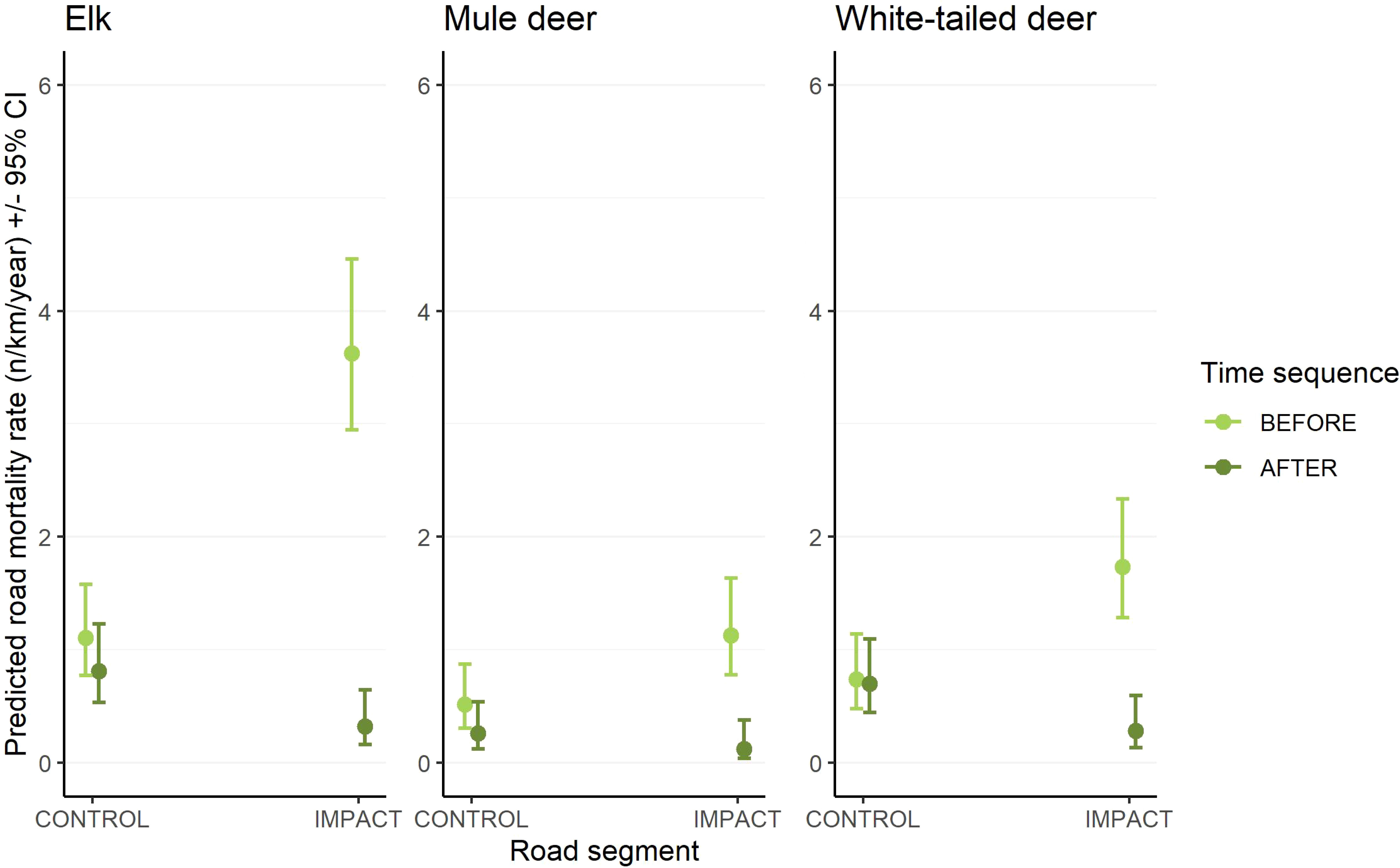
Figure 2 Predicted effects of the mixed-effects, Poisson regression showing the interaction between road segment (control vs. impact) and time sequence (before vs. after) on the collision rate of elk (left panel), white-tailed deer (center panel), and mule deer (right panel). For ease of illustration, only the main effects of the model are shown here. The complete statistical model included an AR1 autoregressive term for year nested within study area to account for temporal autocorrelation and a random intercept for the study area.
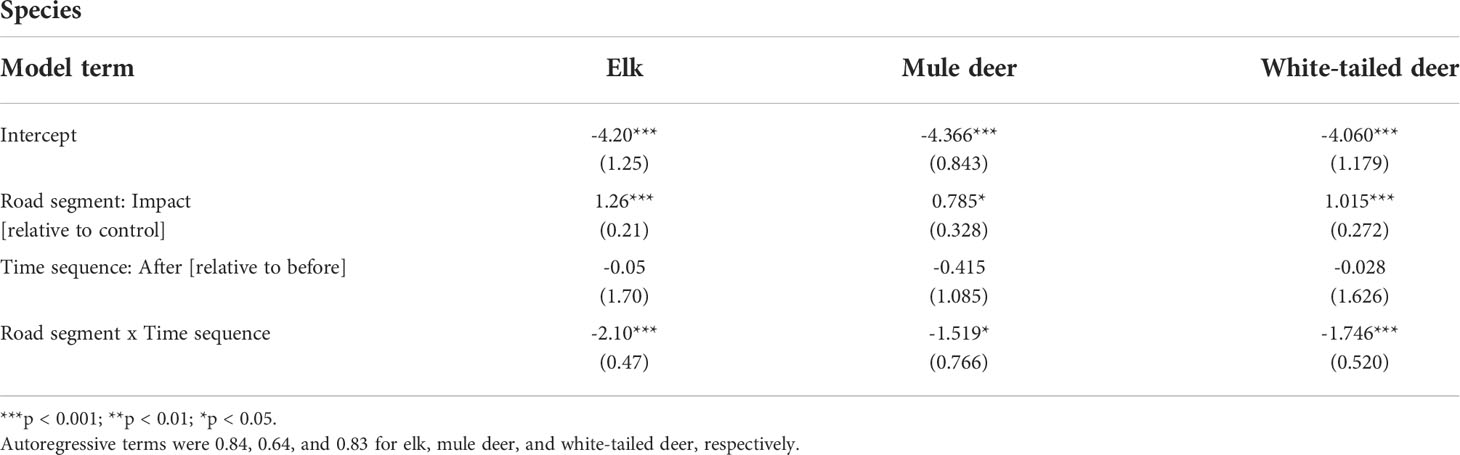
Table 2 Summary of the mixed-effect Poisson models to test for the interaction between road segments (control vs. impact) and time sequence (before vs. after) on elk, mule deer, and white-tailed deer.
Reductions in WVCs were greatest for elk (81–96% decline for fenced segments vs. 9–33% decline in control, for BNP and DMF, respectively), white-tailed deer (100–74% decline for fenced segments vs. 0–3% change in control, for BNP and DMF, respectively), and mule deer (96–60% decline for fenced segments vs. 75–30% decline in control, for BNP and DMF, respectively) in the after period relative to the before period. Both elk and mule deer WVCs declined in unfenced control segments. At the taxa level, there was a significant interaction of road segment and time sequence for ungulates but not carnivores (Figure 3 and Table 3).
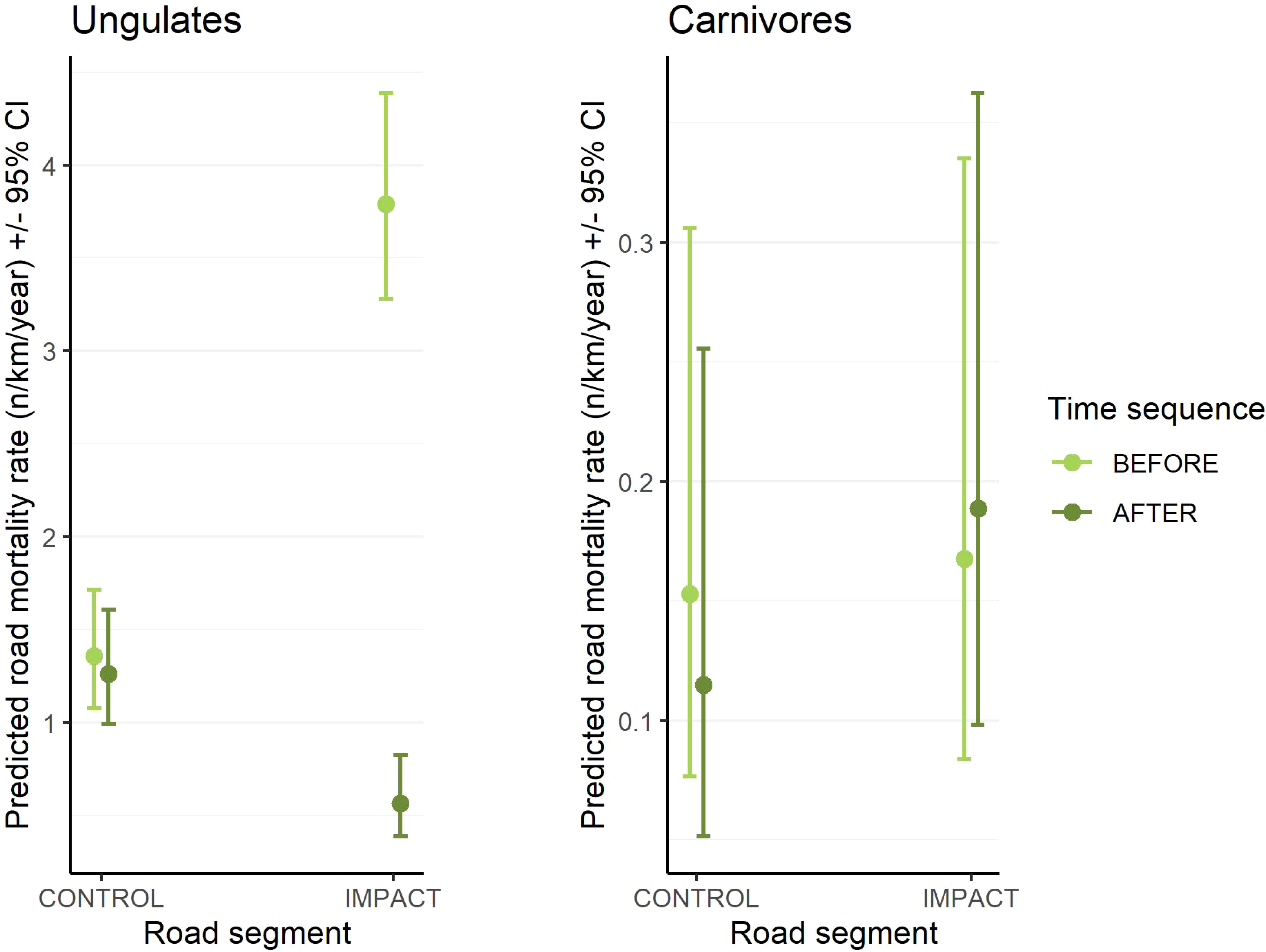
Figure 3 Predicted effects of the mixed-effects, Poisson regression showing the interaction between road segment (control vs impact) and time sequence (before vs after) on the collision rate of all ungulates ppoled together (left panel) and all carnivore species pooled together (right panel). For ease of illustration, only the main effects of the model are shown here. The complete statistical model included an AR1 autogressive term for year nested within study area to account for temporal autocorrelation and a random intercept for study area. Note different scales on the y-axis.
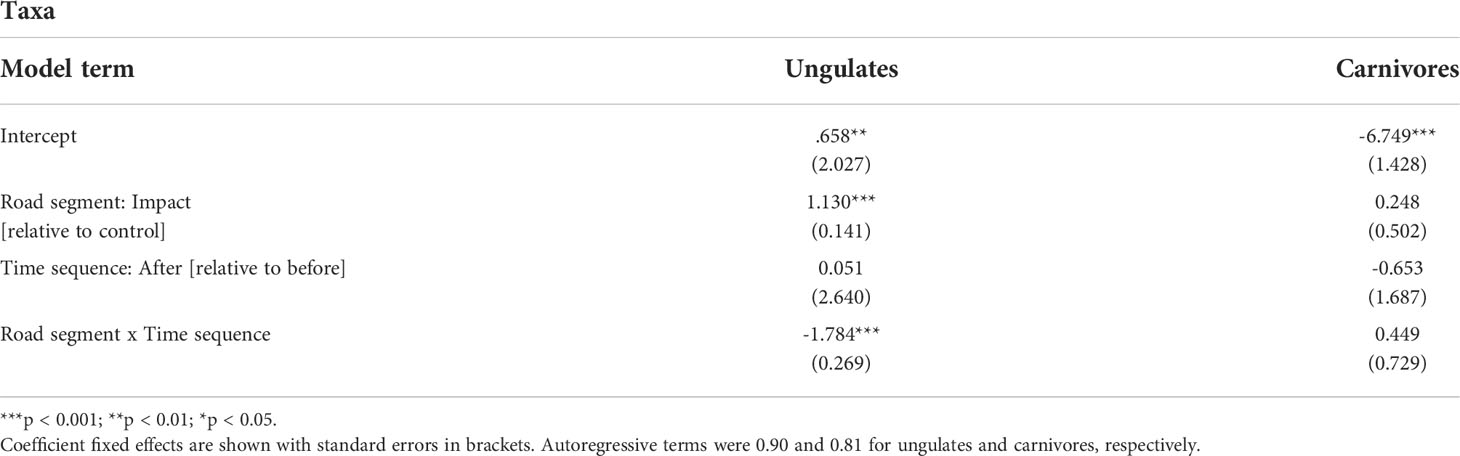
Table 3 Summary of the mixed-effect Poisson models to test for the interaction between road segment (control vs. impact) and time sequence (before vs. after) for all ungulate species pooled together and for all carnivores.
Prior to mitigation, WVCs incurred a total cost of $24,131 to $69,325 per km per year for control and fenced road segments (pooled across study areas), respectively Figure 4. Following mitigation, WVCs incurred a cost of $21,387 to $8,783 per km per year for control and fenced road segments, respectively. In other words, the cost of WVCs across control segments declined by $2,743 per km per year, even though these segments were never fenced during the study. This outcome is likely driven by the observed decline in elk and mule deer WVCs at control segments. Over the same period, the cumulative cost of WVCs in fenced segments declined by $60,541 per km per year. When accounting for both the cost of fencing and the benefits of WVC reduction, fenced segments delivered a net benefit of $3,287 in the first year. Assuming a one-time fixed cost of installing fences, mitigation is estimated to provide a net benefit of $572,872 per km at fenced segments over a 10-year period. Because there were generally fewer WVCs at control segments, fencing them will take longer to reach a net economic gain. As a thought experiment, we assumed that all other factors affecting WVCs are stable (e.g., animal population size, traffic volume, and driver behavior) and projected the observed background declining cost of WVCs of 11% per km per year. Under these conditions, it would take 88 years to reach a net cost of < $1 per km per year for control segments in the absence of fencing. Thus, the time required to reach a financial break-even point for fenced segments (< 1 year) and unfenced control segments (~70 years) is roughly 90× greater without fencing.
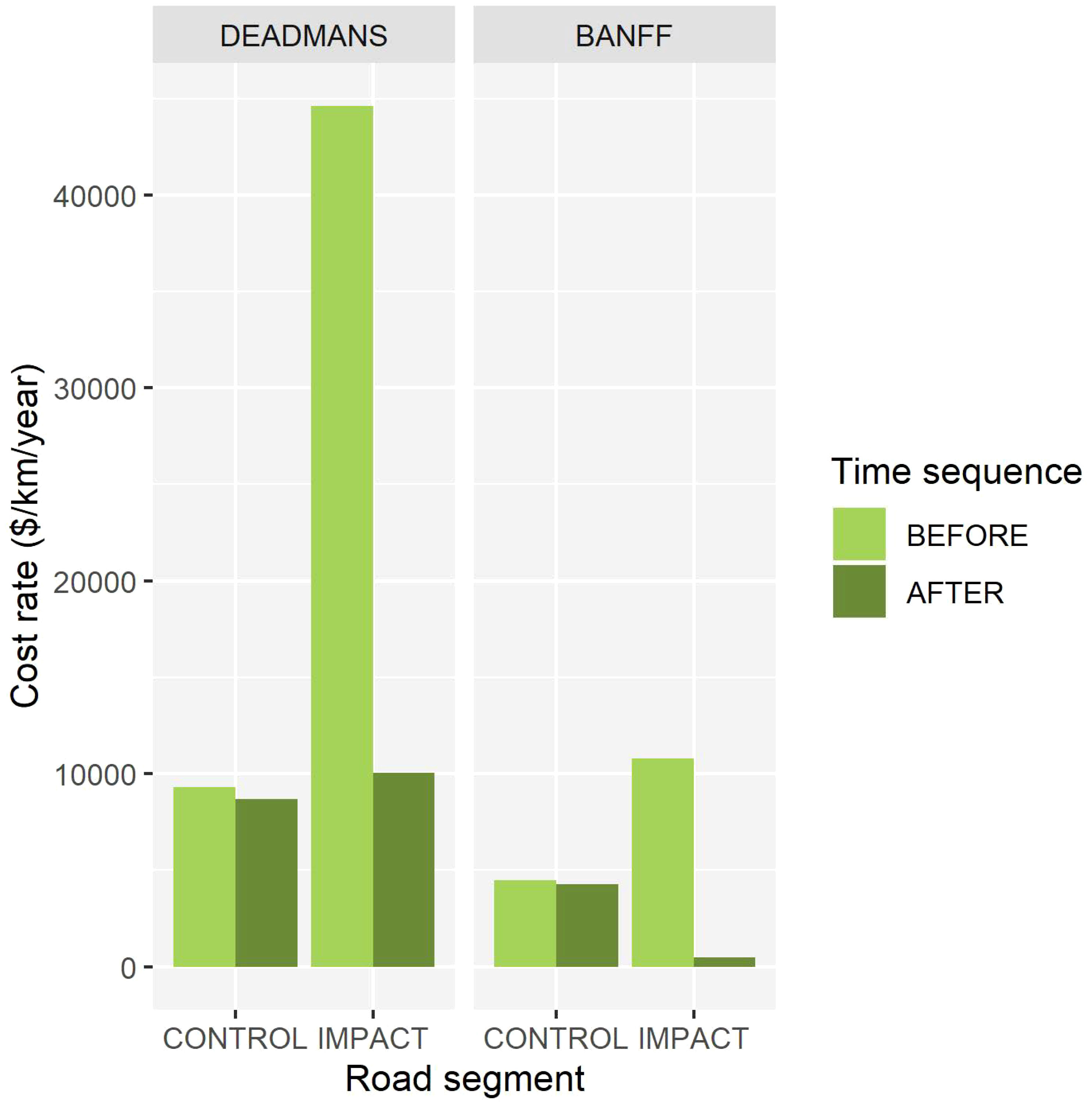
Figure 4 Estimated cost of collisions occurring within each road segment and time sequence for the two study areas, Dead Mans Flats and Banff National Park. Cost estimates per collision are based on Huijser et al (2009).
Discussion
Through a combination of long-term monitoring and phased highway mitigation, we conducted an opportunistic, BACI study design in two adjacent areas of the Canadian Rocky Mountains. We found that wildlife-exclusion fencing led to a significant (> 80%) reduction in WVCs for common ungulate species. We did not detect a change in carnivore-vehicle collisions following mitigation. Our results indicate that that societal-wide economic benefits of mitigation can be realised in less than 2 years for high-risk WVC road segments and in 6 years for lower risk areas. While the effect sizes that we observed are consistent with related studies (e.g., Clevenger et al., 2001; Rytwinski et al., 2015; Rytwinski et al., 2016), the BACI study design that we employed revealed confounding variation in WVC rates that more accurately portrays the costs and benefits of wildlife-exclusion fencing.
Consistent with previous studies in this region (Clevenger et al., 2001; Gilhooly et al., 2019) and abroad (McCollister and van Manen, 2010), wildlife exclusion fencing is highly effective at reducing vehicle collisions with ungulates. The reduction in WVCs was greatest in the BNP study area, likely because of the additional effects of staff at Banff National Park who will actively direct animals off the highway right-of-way through a series of large (4-m wide) swing gates and conduct fence repairs when damaged (Clevenger et al., 2009). In addition, the BNP study area of the highway was more than twice as long as the DMF study area, possibly indicating that “fence end” intrusions could be reducing the effectiveness of this shorter section of fencing (see Huijser et al., 2016).
Unlike ungulates, carnivore mortality rates were low but did not change following mitigation. The lack of response by carnivores can be explained by both biological and statistical reasons. From a biological perspective, page wire fences can be climbed by black bears – the most common carnivore in our study (Serrouya, 1999; Hebblewhite et al., 2003). Downed trees on top of the fence and soil erosion under the fences can also open gaps in the fence that lead to intrusions onto the right of way. Consequently, carnivore intrusions may remain an issue for the TCH, particularly if there are food attractants such as road-killed ungulates and herbaceous plants (i.e., for bears). In addition to these biological explanations, our study may not have the statistical power to detect a change in carnivore collisions. For example, we detected no cougar mortalities in BNP and just four in the DMF area. Of those four, only one occurred in the control segment. Similarly, low observations occurred for wolves and lynx, making it impossible to conduct species-specific comparisons among carnivores. We know from other studies that carnivores are frequently observed near the highway and use nearby wildlife crossing structures many hundreds of times annually, thus significantly reducing mortality risk compared with an unfenced TCH (Clevenger et al., 2009; Ford and Clevenger, 2010; Ford et al., 2010). From a road safety perspective, our results suggest that carnivore-vehicle collisions are low risk. However, the per capita effect of even rare mortality, especially for adult females, can be profound for low fecund, low density species such as carnivores (Lamb et al., 2020).
For the public, the return on investment from highway mitigation can be realized within a few years providing that a few conditions are met. First, the fencing needs to be placed in an area with a high risk of collisions. While intuitive, identifying area of high risk also requires adequate data: long-term, consistent, and accurate data collection protocols. Variation in such protocols, such as GPS versus landmark-located carcases can profoundly affect where and why roadkill hotspots occur (Gunson et al., 2009). Prior to mitigation in our study area, high-risk road segments of the TCH incurred close to $70,000 per km per year in damage to vehicles, human health, and lost opportunity costs of wildlife mortality (sensu Huijser et al., 2009). With an estimated cost of $60,000 per km for fencing alone (Ford et al., 2011), benefits to society at large are realized almost immediately. For the return-on-investment analysis to function, we assumed that both funders and benefactors of fencing share a common economic pool. However, the BNP study area was part of a national parks system that provides ecosystem services to visitors from all over the world, most of whom place transportation infrastructure as a highly ranked part of their experience (Geng et al., 2021). In this case, the funders of the BNP mitigation costs are “national” or international in scope, and benefactors include a largely non-resident population of national park visitors and people passing through Banff National Park. In contrast, the highway in the DMF study area was funded through a mix of provincial and federal funding (Lee et al., 2012), with benefits accruing to local traffic and a smaller tourism sector (Hu et al., 2022). Thus, the payers and benefactors of the economic analyses that we conducted can vary significantly at different segments of the same highway. Nonetheless, for nationally significant infrastructure such as the TCH, the assumptions that we made based on Huijser et al. (2009) for a net societal benefit seem reasonable. For more localized mitigation projects, such as secondary roads, such cost-benefit calculations of fencing require further exploration of these assumptions.
Following perspectives shared in Rytwinski et al. (2015), our study was one of few to conduct a BACI analyses to test for the effectiveness of fencing to reduce WVCs. This approach was made possible by long-term monitoring of WVCs conducted along sequential phases of highway mitigation. Such a phased approach can be very cost effective by targeting high-risk areas with more efficient and shorter road segments (Ford et al., 2011; Huijser et al., 2016). Indeed, the “before” periods for the fenced segments had much higher rates of WVCs than the before period for the nonfenced segments. This means that fencing was appropriately installed at higher risk areas. High-risk locations can be identified through WVC monitoring, as in our study system (e.g., Clevenger et al., 2015) and through various connectivity algorithms that simulate likely road crossing locations (Clevenger et al., 2002; Quaglietta et al., 2019; Zeller et al., 2020).
Another important outcome of our study was the small decline in WVCs rates among elk and deer along unfenced road segments. Given the short distance between fenced and unfenced segments, the mechanisms leading to the decline of WVCs at control segments were likely also present at the fenced segments. We speculate that the background decline in WVCs could be driven by a declining population abundance of elk (Hebblewhite et al., 2002, Zimmermann Teixeira et al., 2017), changing animal movement patterns near roads (Seiler, 2005), or changing driver behavior associated with other mitigation efforts in the area, such as signage (Huijser et al., 2009). Traffic volume in this area has increased over the duration of the study area from 10,000 AADT in the early 1980s to ~20,000 AADT in 2014 (Gilhooly et al., 2019)—the volumes are much higher than reported thresholds of avoidance for ungulates (Seiler, 2005). In other words, it is unlikely that the added traffic volume created a stimulus that led to fewer attempts by individual animals to cross the road. Thus, we do not believe that the increase in traffic volume is causing ungulates to avoid crossing the road more in the after period than during the before period. Changes in driver behavior may be linked to fewer WVCs at unfenced segments; however, it is difficult to imagine a mechanism that altered driver behavior in such a significant manner over a short period of time and for the BNP study area of the TCH but not the DMF study area.
Regardless of the mechanism for the decline in WVCs at control segments, this signal confounds the magnitude of the fencing effect per se and is the reason for future work to report both apparent and realized effectiveness. Apparent effectiveness does not consider background changes of WVCs at control segments and may over or underestimate fencing effectiveness. For example, the apparent effectiveness of fencing for ungulate WVCs in BNP is a 96% decline (i.e., a decline of 0.96–0.04 WVCs per km per year). However, when considering that WVCs dropped by 6% at control segments over this same period, the realized effectiveness is 90%. At the DMF study area, the apparent effectiveness of fencing was a 73% decline in ungulate WVCs. During this same period, controls had a 10% increase in ungulate WVCs. Thus, the realized effectiveness of fencing on ungulate WVCs in DMF is 82%. In sum, the realized effectiveness of fencing the TCH in the Rocky Mountains is an 80–90% reduction in ungulate WVCs.
The BACI design that we used is not only associated with stronger effect sizes than “before–after” or “control–impact” (space for time substitutions) studies (Rytwinski et al., 2016), but it is also critical for developing a rigorous understanding of how large, free-ranging animals interact with transportation infrastructure. In a few cases, such as road closures (Whittington et al., 2019) or lockdowns associated with the COVID-19 pandemic (Shilling et al., 2021; Abraham and Mumma, 2021), there is limited opportunity to perform replicated field experiments in road ecology for large mammals. Investments in long-term monitoring of WVCs (Gunson et al., 2009; Gilhooly et al., 2019), animal movement at crossing structures (Clevenger et al., 2009; Ford et al., 2017), and predator–prey interactions (Ford and Clevenger, 2010) are a key feature of BACI designs when infrastructure cannot be readily moved or altered following construction. As part of this long-term approach to monitoring, accounting for temporal autocorrelation will become a more reliable means to meet the assumptions of statistical analyses (Boyle et al., 2021). However, these approaches may not be reliable for short-time series data, even if the variances in the data are temporally structured in the statistical model. Depending on the other variables of interest in a study, time series analysis may require a large number (> 10 years) of observations or resampling methods to estimate effect sizes (e.g., Hervieux et al., 2014; McNay et al., 2022).
Fencing is used for a variety of purposes in conservation and science, including human–wildlife coexistence (Bauer, 1964; Packer et al., 2013), disease mitigation (Mysterud and Rolandsen, 2019), basic ecological research (Goheen et al., 2018), and ecological restoration (Laskin et al., 2020; Ford, 2021). Indeed, with the growing use of fencing in conservation, specialized analytical tools are being used to asses the response of wildlife movement (Xu et al., 2021) amid broader calls for developing the science of “fence ecology” (Jakes et al., 2018; McInturff et al., 2020). Our work extends these studies by showing how fencing technology (i.e., high page wire) reduces ~70–90% of ungulate-vehicle collisions. Further research on fencing effectiveness for large carnivores and other species such as small mammals (Ford and Clevenger, 2019) will be needed, particularly given their infrequent occurrence in WVC studies (Ford and Fahrig, 2007).
Data availability statement
The original contributions presented in the study are included in the article/Supplementary Material. Further inquiries can be directed to the corresponding author.
Ethics statement
We used public data on roadkills. We did not harm or handle animals during this study.
Author contributions
All authors listed have made a substantial, direct, and intellectual contribution to the work, and approved it for publication.
Acknowledgments
Funding for this research came from a variety of sources including Parks Canada and Public Works and Government Services Canada, Parks Canada’s Ecological Integrity Innovation and Leadership Fund, the Parks Canada Ecosystem Science Office (National Office) and the Woodcock Foundation. We thank the Transportation Pooled Fund Study TPF 5(358) committee and the Nevada Department of Transportation for funding and valuable contributions to this paper.
Conflict of interest
The authors declare that the research was conducted in the absence of any commercial or financial relationships that could be construed as a potential conflict of interest.
Publisher’s note
All claims expressed in this article are solely those of the authors and do not necessarily represent those of their affiliated organizations, or those of the publisher, the editors and the reviewers. Any product that may be evaluated in this article, or claim that may be made by its manufacturer, is not guaranteed or endorsed by the publisher.
References
Abraham J. O., Mumma M. A. (2021). Elevated wildlife-vehicle collision rates during the COVID-19 pandemic. Sci. Rep. 11, 20391. doi: 10.1038/s41598-021-99233-9
Bauer F. H. (1964). Queensland’s new dingo fence. Aust. Geographer 9, 244–246. doi: 10.1080/00049186408702431
Barrueto M., Ford A. T., Clevenger A. P. (2014). Anthropogenic effects on activity patterns of wildlife at crossing structures. Ecosphere 5(3), 1–19.
Boyle S. P., Keevil M. G., Litzgus J. D., Tyerman D., Lesbarrères D. (2021). Road-effect mitigation promotes connectivity and reduces mortality at the population-level. Biol. Conserv. 261, 109230. doi: 10.1016/j.biocon.2021.109230
Carter N., Killion A., Easter T., Brandt J., Ford A. (2020). Road development in Asia: Assessing the range-wide risks to tigers. Sci. Adv. 6 (18), eaaz9619. doi: 10.1126/sciadv.aaz9619
Clevenger A. P., Barrueto M., Gunson K. E., Caryl F. M., Ford A. T. (2015). Context-dependent effects on spatial variation in deer-vehicle collisions. Ecosphere 6, 1–20. doi: 10.1890/ES14-00228.1
Clevenger A. P., Chruszcz B., Gunson K. E. (2001). “Highway mitigation fencing reduces wildlife-vehicle collisions.” In Wildlife Society Bulletin (1973-2006) 29 (2), 646–653. JSTOR, http://www.jstor.org/stable/3784191. Accessed 5 Oct. 2022.
Clevenger A. P., Ford A. T., Sawaya M. A. (2009). Banff wildlife crossings project: integrating science and education in restoring population connectivity across transportation corridors (Bozeman, MT United States: Western Transportation Institute Montana State University, Bozeman), 59717–4250.
Clevenger A. P., Wierzchowski J., Chruszcz B., Gunson K. (2002). GIS-generated, expert-based models for identifying wildlife habitat linkages and planning mitigation passages. Conserv. Biol. 16, 503–514. doi: 10.1046/j.1523-1739.2002.00328.x
Dickie M., Serrouya R., McNay R. S., Boutin S. (2017). Faster and farther: wolf movement on linear features and implications for hunting behaviour. J. Appl. Ecol. 54, 253–263. doi: 10.1111/1365-2664.12732
Dulac J. (2013). Global land transport infrastructure requirements Vol. 20 (Paris: International Energy Agency), 2014.
Edwards H. A., Lebeuf-Taylor E., Busana M., Paczkowski J. (2022). Road mitigation structures reduce the number of reported wildlife-vehicle collisions in the bow valley, Alberta, Canada. Conserv. Sci. Pract. 4, e12778. doi: 10.1111/csp2.12778
Epps C. W., Palsbøll P. J., Wehausen J. D., Roderick G. K., Ramey R. R., McCullough D. R. (2005). Highways block gene flow and cause a rapid decline in genetic diversity of desert bighorn sheep. Ecol. Lett. 8, 1029–1038. doi: 10.1111/j.1461-0248.2005.00804.x
Ford A. T. (2021). Operationalizing process‐based restoration for terrestrial communities. Restoration Ecology 29 (8), e13457. doi: 10.1111/rec.13457
Ford A. T., Barrueto M., Clevenger A. P. (2017). Road mitigation is a demographic filter for grizzly bears. Wildlife Soc. Bull. 41, 712–719. doi: 10.1002/wsb.828
Ford A. T., Clevenger A. P. (2010). Validity of the prey-trap hypothesis for carnivore-ungulate interactions at wildlife-crossing structures. Conserv. Biol. 24, 1679–1685. doi: 10.1111/j.1523-1739.2010.01564.x
Ford A. T., Rettie K., Cleavenger A. P. (2009). Fostering ecosystem function through an international public–private partnership: A case study of wildlife mitigation measures along the Trans-Canada Highway in Banff National Park, Alberta, Canada. International Journal of Biodiversity Science & Management 5(4), 181–189.
Ford A. T., Clevenger A. P. (2019). Factors affecting the permeability of road mitigation measures to the movement of small mammals. Can. J. Zoology 97, 379–384. doi: 10.1139/cjz-2018-0165
Ford A. T., Clevenger A. P., Huijser M. P., Dibb A. (2011). Planning and prioritization strategies for phased highway mitigation using wildlife-vehicle collision data. Wildlife Biol. 17, 253–265. doi: 10.2981/09-051
Ford A. T., Clevenger A. P., Rettie K. (2010). The banff wildlife crossings project: an international public-private partnership (Washington, DC: Island Press).
Ford A. T., Fahrig L. (2007). Diet and body size of north American mammal road mortalities. Transport. Res. Part D: Transport. Environ. 12, 498–505. doi: 10.1016/j.trd.2007.07.002
Ford A. T., Fahrig L. (2008). Movement patterns of eastern chipmunks (Tamias striatus) near roads. J. Mammalogy 89, 895–903. doi: 10.1644/07-MAMM-A-320.1
Geng D. C., Innes J. L., Wu W., Wang W., Wang G. (2021). Seasonal variation in visitor satisfaction and its management implications in Banff National Park. Sustainability 13 (4), 1681. doi: 10.3390/su13041681
Gilhooly P. S., Nielsen S. E., Whittington J., St. Clair C. C. (2019). Wildlife mortality on roads and railways following highway mitigation. Ecosphere 10, e02597. doi: 10.1002/ecs2.2597
Goheen J. R., Augustine D. J., Veblen K. E., Kimuyu D. M., Palmer T. M., Porensky L. M., et al. (2018). Conservation lessons from large-mammal manipulations in East African savannas: the KLEE, UHURU, and GLADE experiments. Ann. New York Acad. Sci 1429 (1), 31–49. doi: 10.1111/nyas.13848
Gunson K. E., Clevenger A. P., Ford A. T., Bissonette J. A., Hardy A. (2009). A comparison of data sets varying in spatial accuracy used to predict the occurrence of wildlife-vehicle collisions. Environ. Manage. 44, 268–277. doi: 10.1007/s00267-009-9303-y
Hebblewhite M., Percy M., Serrouya R. (2003). Black bear (Ursus americanus) survival and demography in the bow valley of banff national park, Alberta. Biol. Conserv. 112, 415–425. doi: 10.1016/S0006-3207(02)00341-5
Hebblewhite M., Pletscher D. H., Paquet P. C. (2002). Elk population dynamics in areas with and without predation by recolonizing wolves in banff national park, Alberta. Can. J. Zoology 80, 789–799. doi: 10.1139/z02-058
Hervieux D., Hebblewhite M., Stepnisky D., Bacon M., Boutin S. (2014). Managing wolves (Canis lupus) to recover threatened woodland caribou (Rangifer tarandus caribou) in Alberta. Can. J. Zoology 1037, 1029–1037. doi: 10.1139/cjz-2014-0142
Huijser M. P., Duffield J. W., Clevenger A. P., Ament R. J., McGowen P. T. (2009). Cost-benefit analyses of mitigation measures aimed at reducing collisions with large ungulates in the united states and canada: A decision support tool. Ecol. Soc. 14(2). Available at: https://www.jstor.org/stable/26268301
Huijser M. P., Fairbank E. R., Camel-Means W., Graham J., Watson V., Basting P., et al. (2016). Effectiveness of short sections of wildlife fencing and crossing structures along highways in reducing wildlife-vehicle collisions and providing safe crossing opportunities for large mammals. Biol. Conserv. 197, 61–68. doi: 10.1016/j.biocon.2016.02.002
Huijser M. P., Mosler-Berger C., Olsson M., Strein M. (2015). “Wildlife warning signs and animal detection systems aimed at reducing wildlife-vehicle collisions,” In van der Ree R., Smith D. J., Grilo C. Handbook of road ecology, 198–212. doi: 10.1002/9781118568170.ch2
Hu F., Wang Z., Sheng G., Lia X., Chen C., Geng D., et al. (2022). Impacts of national park tourism sites: a perceptual analysis from residents of three spatial levels of local communities in Banff National Park. Environment Dev. Sustainability 24 (3), 3126–45. doi: 10.1007/s10668-021-01562-2
Jaeger J. A., Bowman J., Brennan J., Fahrig L., Bert D., Bouchard J., et al. (2005). Predicting when animal populations are at risk from roads: an interactive model of road avoidance behavior. Ecol. Model. 185, 329–348. doi: 10.1016/j.ecolmodel.2004.12.015
Jakes A. F., Jones P. F., Paige L. C., Seidler R. G., Huijser M. P. (2018). A fence runs through it: A call for greater attention to the influence of fences on wildlife and ecosystems. Biol. Conserv. 227, 310–318. doi: 10.1016/j.biocon.2018.09.026
Lamb C. T., Ford A. T., McLellan B. N., Proctor M. F., Mowat G., Ciarniello L., et al. (2020). The ecology of human–carnivore coexistence. Proc. Natl. Acad. Sci. United States America 117, 17876–17883. doi: 10.1073/pnas.1922097117
Lamb C. T., Mowat G., Reid A., Smit L., Proctor M., McLellan B. N., et al. (2018). Effects of habitat quality and access management on the density of a recovering grizzly bear population. J. Appl. Ecol. 55, 1406–1417. doi: 10.1111/1365-2664.13056
Laskin D. N., Watt D., Whittington J., Heuer K. (2020). Designing a fence that enables free passage of wildlife while containing reintroduced bison: a multispecies evaluation. Wildlife Biol. 2020 (4), 1–4. doi: 10.2981/wlb.00751
Laurance W. F., Goosem M., Laurance S. G. W. (2009). Impacts of roads and linear clearings on tropical forests. Trends Ecol. Evol. 24, 659–669. doi: 10.1016/j.tree.2009.06.009
Lee T., Clevenger A. P., Ament R. J. (2012). Highway wildlife mitigation opportunities for the TransCanada Highway in the Bow Valley. Report to Alberta Ecotrust Foundation, Calgary, Alberta.
Lee T. S., Creech T. G., Martinson A., Nielsen S. E., Jakes A. F., Jones P. F., et al. (2020). Prioritizing human safety and multispecies connectivity across a regional road network. Conserv. Sci. Pract., e327. doi: 10.1111/csp2.327
McCollister M. F., van Manen F. T. (2010). Effectiveness of wildlife underpasses and fencing to reduce wildlife–vehicle collisions. J. Wildlife Manage. 74, 1722–1731. doi: 10.2193/2009-535
McInturff A., Xu W., Wilkinson C. E., Dejid N., Brashares J. S. (2020). Fence ecology: Frameworks for understanding the ecological effects of fences. BioScience 70, 971–985. doi: 10.1093/biosci/biaa103
McNay R. S., Lamb C. T., Giguere L., Williams S. H., Martin H., Sutherland G. D., et al. (2022). Demographic responses of nearly extirpated endangered mountain caribou to recovery actions in central British Columbia. Ecol. Appl. 32, e2580. doi: 10.1002/eap.2580
Meijer J. R., Huijbregts M. A. J., Schotten K. C. G. J., Schipper A. M. (2018). Global patterns of current and future road infrastructure. Environ. Res. Lett. 13, 064006. doi: 10.1088/1748-9326/aabd42
Mysterud A., Rolandsen C. M. (2019). Fencing for wildlife disease control. J. Appl. Ecol. 56, 519–525. doi: 10.1111/1365-2664.13301
Packer C., Loveridge A., Canney S., Caro T., Garnett S. T., Pfeifer M., et al. (2013). Conserving large carnivores: Dollars and fence. Ecol. Lett. 16, 635–641. doi: 10.1111/ele.12091
Quaglietta L., Porto M., Ford A. T. (2019). Simulating animal movements to predict wildlife-vehicle collisions: illustrating an application of the novel r package SiMRiv. Eur. J. Wildlife Res. 65, 100. doi: 10.1007/s10344-019-1333-z
Riginos C., Fairbank E., Hansen E., Kolek J., Huijser M. P. (2019). Effectiveness of night-time speed limit reduction in reducing wildlife-vehicle collisions (5300 Bishop Blvd., Cheyenne, WY 82009: Page Wyoming Department of Transportation).
Rytwinski T., Soanes K., Jaeger J. A. G., Fahrig L., Findlay C. S., Houlahan J., et al. (2016). How effective is road mitigation at reducing road-kill? a meta-analysis. Plos One 11, e0166941. doi: 10.1371/journal.pone.0166941
Rytwinski T., van der Ree R., Cunnington G. M., Fahrig L., Findlay C. S., Houlahan J., et al. (2015). Experimental study designs to improve the evaluation of road mitigation measures for wildlife. J. Environ. Manage. 154, 48–64. doi: 10.1016/j.jenvman.2015.01.048
Seiler A. (2005). Predicting locations of moose–vehicle collisions in Sweden. J. Appl. Ecol. 42, 371–382. doi: 10.1111/j.1365-2664.2005.01013.x
Serrouya R. (1999). Permeability of the Trans-Canada highway to black bear movements in the Bow River Valley of Banff National Park (Columbia: Doctoral dissertation, University of British Columbia).
Shilling F., Nguyen T., Saleh M., Kyaw M. K., Tapia K., Trujillo G., et al. (2021). A reprieve from US wildlife mortality on roads during the COVID-19 pandemic. Biol. Conserv. 256, 109013. doi: 10.1016/j.biocon.2021.109013
Trombulak S. C., Frissell C. A. (2000). Review of ecological effects of roads on terrestrial and aquatic communities. Conserv. Biol. 14, 18–30. doi: 10.1046/j.1523-1739.2000.99084.x
Whittington J., Hebblewhite M., Decesare N. J., Neufeld L., Bradley M., Wilmshurst J., et al. (2011). Caribou encounters with wolves increase near roads and trails: A time-to-event approach. J. Appl. Ecol. 48, 1535–1542. doi: 10.1111/j.1365-2664.2011.02043.x
Whittington J., Low P., Hunt B. (2019). Temporal road closures improve habitat quality for wildlife. Sci. Rep. 9, 3772. doi: 10.1038/s41598-019-40581-y
Xu W., Dejid N., Herrmann V., Sawyer H., Middleton A. D. (2021). Barrier behaviour analysis (BaBA) reveals extensive effects of fencing on wide-ranging ungulates. J. Appl. Ecol. 58, 690–698. doi: 10.1111/1365-2664.13806
Zeller K. A., Wattles D. W., Destefano S. (2020). Evaluating methods for identifying large mammal road crossing locations: black bears as a case study. Landscape Ecol. 35, 1799–1808. doi: 10.1007/s10980-020-01057-x
Keywords: mitigation, ungulate, carnivore, road, study design
Citation: Ford AT, Dorsey B, Lee TS and Clevenger AP (2022) A before-after-control-impact study of wildlife fencing along a highway in the Canadian Rocky Mountains. Front. Conserv. Sci. 3:935420. doi: 10.3389/fcosc.2022.935420
Received: 03 May 2022; Accepted: 20 September 2022;
Published: 19 October 2022.
Edited by:
Christine Wilkinson, University of California, Berkeley, United StatesReviewed by:
Anders Flykt, Mid Sweden University, SwedenGreta Schmidt, San Diego State University, United States
Copyright © 2022 Ford, Dorsey, Lee and Clevenger. This is an open-access article distributed under the terms of the Creative Commons Attribution License (CC BY). The use, distribution or reproduction in other forums is permitted, provided the original author(s) and the copyright owner(s) are credited and that the original publication in this journal is cited, in accordance with accepted academic practice. No use, distribution or reproduction is permitted which does not comply with these terms.
*Correspondence: Adam T. Ford, adam.ford@ubc.ca