- 1Department of Pharmacy, Faculty of Health and Medical Sciences, University of Copenhagen, Copenhagen, Denmark
- 2Department of Drug Design and Pharmacology, Faculty of Health and Medical Sciences, University of Copenhagen, Copenhagen, Denmark
Thermostable dry powder formulations with high aerosol performance are attractive inhalable solid dosage forms for local treatment of lung diseases. However, preserved long-term physical stability of dry powder inhaler (DPI) formulations is critical to ensure efficient and reproducible delivery to the airways during the shelf life of the drug product. Here, we show that ternary excipient mixtures of the disaccharide trehalose (Tre), the polysaccharide dextran (Dex), and the shell-forming dispersion enhancer leucine (Leu) stabilize siRNA-loaded lipid-polymer hybrid nanoparticles (LPNs) during spray drying into nanocomposite microparticles, and result in inhalable solid dosage forms with high aerosol performance and long-term stability. The stabilizing roles of Tre and Dex were also studied separately by investigating DPI formulations containing binary mixtures of Leu/Tre and Leu/Dex, respectively. DPI formulations containing binary Leu/Dex mixtures were amorphous and displayed preserved long-term physical stability of LPNs and chemical stability of siRNA in accelerated stability studies under exaggerated storage conditions (ambient temperature and relative humidity). In contrast, powders containing binary Leu/Tre mixtures were amorphous, and hence metastable, and were recrystallized after six months of storage. Ternary mixtures of Tre, Leu, and Dex provided the most efficient protection of the LPNs during the spray drying process and prevented recrystallization of amorphous Tre. Hence, in ternary mixtures, Leu, Tre, and Dex have the following functions: the shell-forming Leu functions as a dispersion enhancer and is essential for high aerosol performance, the disaccharide Tre provides LPN protection during manufacturing and storage due to efficient coverage of the LPN surface, and the polysaccharide Dex promotes the formation of porous particles and prevents recrystallization of Tre during long-term storage. Therefore, the use of ternary excipient mixtures composed of Leu, Tre, and Dex, may prevent instability problems of DPI formulations and preserve the aerosol performance during long-term storage, which is essential for effective pulmonary drug delivery.
Introduction
Thermostable dry powder inhaler (DPI) formulations with aerosol properties customized for deep lung delivery are attractive solid dosage forms for local treatment of inflammatory lung conditions, for example, chronic obstructive pulmonary disease (COPD) and asthma. In addition, pulmonary administration is widely accepted by patients for the treatment of respiratory disorders (Xu et al., 2021). Inhalation therapy is frequently used because 1) it allows for local drug delivery directly to the pharmacological target, which enables dose reduction, and 2) it reduces systemic drug exposure. Hence, inhalation therapy often displays reduced side effects, as compared to systemic administration (Shaffer, 2020). However, designing DPI formulations is an inherently complex process that requires fine tuning of the formulation properties (Xu et al., 2021). For example, a high aerosol performance is a prerequisite for efficacious DPI formulations.
The stability of DPI formulations is critical to ensuring the delivery of a reproducible drug dose to the airways. The physical stability of DPI formulations during manufacture and storage is closely related to the physicochemical properties, for example, 1) the moisture content, 2) the density, 3) the aerodynamic particle size, 4) the surface roughness, 5) the particle shape, 6) the solid-state properties, 7) interparticulate forces, and 8) the aerosol performance (Xu et al., 2021). In addition, the chemical stability of the active pharmaceutical ingredient (API), for example, RNA, must be preserved during manufacturing and storage. Common preparation methods of DPI formulations include spray drying, spray freeze drying, and supercritical fluid technology (Shetty et al., 2020). A systematic examination, understanding, and optimization of the physical stability of dry powder–based RNA formulations and the chemical stability of the encapsulated RNA are required during formulation development to design DPI formulations with satisfactory long-term storage stability. However, little is known about physical instability mechanisms that may compromise the long-term stability of inhalable dry powder–based RNA therapeutics, including small interfering RNA (siRNA).
DPI formulations are usually manufactured by spray drying using stabilizing excipients to protect the API during the processing and long-term storage. Trehalose (Tre) is widely used as an excipient to stabilize the API during the drying process by forming hydrogen bonds with the API, or by vitrifying the API in a glassy state (Bosquillon et al., 2001; Ingvarsson et al., 2011; Mah et al., 2019). However, DPI formulations containing Tre are highly hygroscopic, are prone to deliquescence, and recrystallize from a metastable amorphous state to a crystalline state when exposed to a high moisture environment, which eventually impairs the aerosol performance (Mah et al., 2019). It is well-known that combining carbohydrates, for example, Tre, with amino acids, for example, leucine (Leu), increases the aerosol performance by improving the dispersibility of the DPI formulation, which is attributed to its surfactant-like properties and rapid crystallization, eventually forming an outer shell on the particles. In particular, the use of binary lyoprotectant mixtures of Tre and Leu has been shown to result in DPI formulations with preserved dry powder aerosolization properties during storage (Lechanteur and Evrard, 2020). However, our understanding of the effect of the Leu content in DPI formulations on the recrystallization of amorphous Tre during storage is still incomplete.
In contrast, spray drying with polysaccharide excipients, especially dextran (Dex), has been reported to result in porous particles with a hydrophobic surface (Kadota et al., 2019; Tarara et al., 2022), which is a critical quality attribute for high aerosol performance. However, there are no studies on the use of Dex as a stabilizing agent in combination with the anti-adherent Leu for pulmonary delivery. In addition, the long-term stability of Leu and saccharides, for example, Dex, remains to be addressed.
In this study, we examined the effect of the excipients Tre and Dex, in combination with Leu, on the aerosol performance and the long-term storage stability of spray-dried DPI formulations of siRNA-loaded lipid-polymer hybrid nanoparticles (LPNs) under different storage conditions. The LPNs consist of the ionizable cationic lipid-like material referred to as lipidoid L5N12 (Akinc et al., 2008), which displays a tetraamine backbone linked to five 12 carbon long alkyl chains, providing efficient interaction with polyanionic nucleic acids via electrostatic attractive interactions, and mediating cellular uptake, endosomal escape, and cytosolic delivery. They also contain the biodegradable polymer poly (D,L-lactic-co-glycolic acid) (PLGA), which we hypothesized forms the polymeric core of the LPNs enabling a sustained release of siRNA, and it thus constitutes an inherent part of the LPN architecture, whereas L5N12 interacts with the PLGA core and forms a membrane shell structure around the core (Thanki et al., 2017; Thanki et al., 2019). Previously, we showed that LPNs mediate efficient and safe intracellular delivery of siRNA and gene silencing in vitro and in vivo (Jansen et al., 2019). Recently, we designed a system based on ternary mixtures of the stabilizing excipients Tre and Dex, in combination with the shell-forming dispersion enhancer Leu, which stabilizes siRNA-loaded LPNs during spray drying into nanocomposite microparticles, and results in inhalable solid dosage forms with high aerosol performance (Xu et al., 2022). However, the long-term storage stability of the ternary system was not investigated. Hence, in this study, we aimed to investigate the stabilizing roles of Tre and Dex and explore the possibility of simplifying the ternary system by investigating DPI formulations containing binary mixtures of Leu/Tre and Leu/Dex, respectively. Our previous data also showed that inclusion of more than 60% Leu in the DPI formulations resulted in fragmented particles with a high aerosol performance (Xu et al., 2022). Hence, 60% (w/w) of Leu represents the upper limit, and the Leu content was, therefore, investigated systematically at 0%, 20%, 40%, and 60% (w/w) in the present study. The difference between DPI formulations of siRNA-loaded LPNs, co-spray–dried with binary mixtures of Leu/Dex and Leu/Tre, respectively, were investigated in detail with respect to 1) the particle size, 2) the residual moisture content, 3) crystallinity, and 4) surface morphology. The aerosol performance of the DPI formulations was examined using the PreciseInhale® (PI) dispensing system (Selg et al., 2013) and the Next Generation Impactor (NGI), respectively. The chemical stability of siRNA was measured by high-performance liquid chromatography (HPLC). The long-term storage stability was studied by evaluating the solid state and the aerosol performance of the DPI formulations, and the chemical stability of the siRNA cargo, in accelerated stability studies under exaggerated storage conditions (ambient temperature and relative humidity).
Materials and Methods
Materials
The 2'-O-Methyl-modified dicer substrate asymmetric siRNA duplex directed against tumor necrosis factor-alpha (TNF-α, 17,928 g/mol) was generously provided by GlaxoSmithKline (Stevenage, United Kingdom) as a dried, purified, and desalted duplex. The duplex was re-annealed according to a standard protocol recommended by Integrated DNA Technologies (Coralville, IA, United States ). The siRNA had the following sequence and modification pattern: TNF-α sense 5'-pGUCUCAGCCUCUUCUCAUUCCUGct-3' and antisense 5'-AGCAGGAAUGAGAAGAGGCUGAGACAU-3', where lower case letters represent deoxyribonucleotides, underlined capital letters represent 2'-O-methylribonucleotides, and p represents a phosphate residue. Lipidoid L5N12 was synthesized, purified, and characterized as reported previously (Thanki et al., 2017). PLGA (lactide:glycolide molar ratio 75:25, Mw: 20 kDa) was obtained from Wako Pure Chemical Industries (Osaka, Japan). Heparin was acquired from Biochrom (Berlin, Germany). Tris-EDTA buffer (TE-buffer, 10 mM Tris, 1 mM EDTA, pH 7.5) and Quant-iT™ RiboGreen® reagent were purchased from Molecular Probes, Invitrogen (Paisley, United Kingdom). Dextran (6 kDa) was from Alfa Aesar (Haverhill, MA, United States ). Glass microfiber filters used for the PI system were procured from GE Healthcare (Chicago, IL, United States ). Octyl β-D-glucopyranoside (OG), poly (vinyl alcohol) (PVA), Leu, Tre dehydrate, and additional chemicals (analytical grade) were provided by Sigma-Aldrich (St. Louis, MO, United States ). RNase-free diethyl pyrocarbonate–treated Milli-Q water (DEPC-water) was used for all buffers and dilutions.
Preparation of siRNA-Loaded LPNs and Physicochemical Characterization
L5N12-based LPNs loaded with siRNA were prepared using the double emulsion solvent evaporation method, essentially as reported previously (Thanki et al., 2019). The L5N12 content relative to the total solid content (L5N12 and PLGA) was 15% (w/w), and the L5N12:siRNA ratio was 15:1 (w/w), which was optimized previously (Lokras et al., 2020). The physicochemical properties, that is, the z-average, the polydispersity index (PDI), the zeta-potential, and the siRNA encapsulation efficiency were measured as previously described (Lokras et al., 2020).
Spray Drying
The DPI formulations were prepared by co-spray drying the LPN dispersions with co-dissolved mixtures of Leu and saccharides (Dex or Tre, or a combination of both) as excipients at different weight ratios (0:100, 20:80, 40:60, and 60:40, Supplementary Table S1). The excipients were dissolved, and the LPNs were dispersed at 5% (w/w) loading and 25 mg/ml stock concentration in DEPC-treated water to a total volume of 10 ml, and the dispersions were spray-dried using a co-current Büchi B-290 spray dryer (Büchi Labortechnik, Flawil, Switzerland) equipped with a nozzle atomizer with an orifice diameter of 2.0 mm by applying previously optimized process parameters (Dormenval et al., 2019). The spray dryer was operated at a feed rate of 1.53 ml/min, an atomizing airflow of 721 L/h, an aspirator capacity of 90%, and an outlet temperature of 50°C (Dormenval et al., 2013). The DPI formulations were separated from the drying gas by centrifugal forces using a high-performance cyclone (Büchi Labortechnik). Further on, all percentages in the DPI formulations are presented as mass percentages between the different excipients. The excipient percentage provided for all DPI formulations represents the mass percentage (w/w) of Leu and saccharide (excluding the siRNA-loaded LPNs).
Yield, Aerodynamic Particle Size, and Morphology of DPI Formulations
The mass of the DPI formulation deposited in the collection vessel after spray drying was weighed to determine the yield of the spray drying process, which was calculated as the difference in the mass of the collection vessel before and after spray drying, divided by the initial total solid mass Eq. 1.
The aerodynamic particle size, that is, the mass median aerodynamic diameter (MMAD), of the nanocomposite microparticles was measured using an Aerodynamic Particle Sizer (APS) Spectrometer 3321 (TSI, Shoreview, MN, United States ) equipped with a Small-Scale Powder Disperser (TSI) to generate the aerosols, as described previously (Jensen et al., 2012). The Aerosol Instrument Manager® Software, Release Version 8.1.0.0 (TSI), was used for data acquisition and analysis. The morphology of the microparticles was investigated by scanning electron microscopy (SEM, TM3030, Hitachi High-Tech Europe, Krefeld, Germany) at an accelerating voltage of 15 kV, a working distance of 5.8 mm, and an emission current of 53.5 A. Prior to imaging, the samples were coated under vacuum with gold in an argon atmosphere with a Sputter Coater 108 auto (Cressington Scientific Instruments, Watford, United Kingdom). A low scanning speed was used to maximize the resolution, and images were acquired at × 4,000 magnification. The samples were analyzed in triplicates.
Moisture Content and Solid-State Properties of DPI Formulations
The moisture content of the DPI formulations was determined using thermogravimetric analysis (TGA). Approximately 10–15 mg DPI formulation was loaded into platinum pans and heated at a rate of 30–300°C/min using a Discovery TGA 550 (Perkin Elmer, Waltham, MA, United States ) with nitrogen purging. The weight loss, caused by evaporation of the water in the sample, was calculated in percent using the TRIOS software (version 4.3) and defined as the moisture content. Thermal analysis was performed using a Discovery DSC (TA instruments, New Jersey, United States ) by filling 5 mg powder into aluminum Tzero pans, which were sealed with aluminum Tzero lids (TA instruments). The samples were heated from −20°C to 160°C at a heating rate of 2 K/min and a modulation amplitude of 0.2129°C for a period of 40 s. The glass transition temperature (
Aerodynamic Properties of DPI Formulations
The PI dispensing system (Inhalation Sciences, Huddinge, Sweden) containing an active aerosol dosing system integrated into the exposure control program was used to examine the aerosol performance of the DPI formulations (Fioni et al., 2018). The PI system includes the data logging instrument CEL-712 Microdust Pro Real-time Dust Monitor (Casella, Bedford, United Kingdom), which displays real-time graphical dust levels. The PI system was used to generate the aerosols, as previously described (Selg et al., 2013). The purpose of the aerosol generation procedure was to test the flowability of the DPI formulations, which was measured as the Casella maximum concentration (Cmax) and the amount of aerosol likely to deposit on the inhalation filter (
The ex vivo lung deposition behavior of the DPI formulations loaded into a clinical inhaler was investigated using an NGI (Copley Scientific, Nottingham, United Kingdom) equipped with a mouth piece adapter and an induction throat (USP throat). An equivalent amount of 50.0 ± 0.5 mg powder was loaded manually in a size 3 capsule (Capsugel, Bornem, Belgium) and dispersed through an Aerolizer® DPI (Novartis, Basel, Switzerland). A standard pharmacopeia dispersion procedure was used (USP 39 ⟨601⟩), where 4 L of air was passed through the inhaler at an airflow rate of 100 L/min for 2.4 s, with a pressure drop of approximately 4 kPa across the device. The DPI formulations either remained in the capsule and the inhaler, or were emitted onto the different stages of the impactor. The powder mass deposited onto each stage was calculated as the mass difference between the filter before and after exposure to the DPI formulations. The samples were analyzed in triplicate. The fine particle fraction (FPF%) and the emitted dose (ED%) were calculated according to Eqs. 3, 4.
Surface Composition of DPI Formulations
X-ray photoelectron spectroscopy (XPS) was performed using the XPS-Nexsa surface analysis system (Thermo Fisher Scientific, East Grinstead, United Kingdom). A 400 µm diameter spot of monochromatized Al Kα radiation and charge composition was employed to avoid charging of the insulating powder samples. The survey spectra were obtained at a pass energy of 200 eV, a step size of 1 eV, a scan number of 5, and a dwell time of 10 ms per step. High-resolution spectra were acquired at a pass energy of 50 eV, a step size of 0.1 eV, a scan number of 20, and a dwell time of 50 ms per step. The sample composition was determined from the survey spectra using the Avantage software package (Thermo Fisher Scientific). Dynamic vapor sorption (DVS) analysis was performed using VTI-SA + (TA instruments). The samples were prepared by weighing 3–5 mg amorphous or 10 mg crystalline DPI formulations, respectively, in a quartz sample holder. The samples were dried at 40°C for 240 min, or until a constant mass was achieved, at a flow rate of 2°C/min (0.0010 wt% in 5 min). After drying, the samples were cooled at 25°C and exposed to the sorption–desorption cycle [from 0% to 90% relative humidity (RH) with a 10% RH of each step]. Each step was in cycle for 240 min or until a constant mass was achieved (0.0010 wt% in 5 min). The data were logged every 2 min or ≥0.0100 wt%, and water sorption–desorption profiles were plotted for each DPI formulation.
Long-Term Storage Stability
The DPI formulations were stored for 6 months under three different storage conditions, that is, 1) 25°C/3% RH, 2) 25°C/58% RH, and 3) 40°C/3% RH. One desiccator with silica gel (3% RH) was stored at 25°C (RT), one desiccator with silica gel (3% RH) was stored at 40°C, and the third desiccator with sodium bromide (58% RH) was stored at 25°C. The hydrodynamic diameter of the LPNs was measured using DLS after storage for 1, 3, and 6 months, respectively. The crystallinity, morphology, moisture content, and aerodynamic properties of the DPI formulations upon storage were investigated using XRPD, SEM, TGA, APS, and PI, respectively. The crystallinity of the DPI formulations was analyzed at days 0, 1, 3, 7, and 14, and after 1, 3, and 6 months.
Chemical Stability of siRNA
The chemical stability of the siRNA cargo of the LPNs in the DPI formulations was evaluated at day 0, and after 3 and 6 months, respectively. An Agilent 1260 Infinity (Santa Clara, CA, United States ) HPLC system equipped with a Phenomenex Luna C18 (2) column (Torrance, CA, United States ) of dimensions 150 × 4.6 mm, a particle size of 3 μm, and a pore size of 100 Å was used for siRNA quantification. The setting parameters used for quantification were essential as described previously (Xu et al., 2022). The DPI formulations were reconstituted by dissolving 5 mg powder in 100 μL DEPC-water. Subsequently, a volume of 50 μL LPN dispersion was mixed with 200 μL CHCl3 by vortexing for 5 min. A volume of 100 μL HD solution was added, and the mixture was rotated for 5 min to extract the siRNA into the aqueous phase. The water phase and the organic phase were separated by centrifugation at 4°C and 22,000 × g for 12 min, and the supernatant was used for quantification. The standard curve (retention time 11 min) was linear in the investigated siRNA concentration range of 1.13–18.0 μg/ml.
Statistical Analysis
Data were analyzed using GraphPad Prism (version 8.4.2, La Jolla, CA, United States ). Data are represented as mean values ± standard deviation. The aerosol performance of the DPI formulations was compared by one-way analysis of variance (ANOVA), and pair-wise comparison was performed using Tukey’s post hoc test. A p-value ≤ 0.05 was considered statistically significant, p < 0.05 (*), p < 0.01 (**), and p < 0.001 (***).
Results
DPI Formulations Containing 40% Leu Display Optimal Performance When Combined With Saccharides
Dispersions of siRNA-loaded LPNs were prepared and co-spray–dried with co-dissolved mixtures of Leu and saccharides (Tre and Dex) at different weight ratios (Supplementary Table S1). The resulting DPI formulations were characterized with respect to physicochemical properties, solid-state properties, and aerosol performance. Before spray drying, the siRNA-loaded LPNs displayed a z-average of 193.9 nm, a PDI of 0.109, a zeta-potential of 24.7 mV, and an siRNA encapsulation efficiency (EE%) of 61.5% (Supplementary Table S1), as reported previously (Lokras et al., 2020). After spray drying, the LPNs reconstituted from the DPI formulations showed an increased z-average (Supplementary Table S1), and the z-averages of LPNs co-spray–dried with Leu/Tre mixtures (approximately 326 nm) were generally lower than the z-averages of LPNs co-spray–dried with Leu/Dex (approximately 253 nm). After spray drying, the EE (%) was approximately 50% for all DPI formulations (Supplementary Table S1). The yield of the spray drying process was above 50%, and the MMAD was in the range of 1–5 µm for all DPI formulations (Supplementary Table S2), which indicates that they have the potential to deposit in the deep lungs (Xu et al., 2021). For DPI formulations containing Leu/Dex excipient mixtures, the moisture content decreased from 5.2% to 1.4% when the Leu content was increased from 0% (w/w) to 60% (w/w) and the Dex content was decreased from 100% (w/w) to 40% (w/w) (Supplementary Table S2), whereas the moisture content of all DPI formulations containing Leu/Tre mixtures was very low (0.2%–1.2%).
The surface morphology of the DPI formulations was investigated using SEM (Figure 1). There was a pronounced difference in the microparticle morphology between DPI formulations containing Leu/Dex excipient mixtures (Figure 1, upper panel) and the DPI formulations containing Leu/Tre excipient mixtures (Figure 1, lower panel). It is apparent from the images that DPI formulations containing Dex (Leu/Dex 0:100, w/w) displayed a corrugated surface (Figure 1, upper panel), whereas the DPI formulation containing Tre (Leu/Tre 0:100, w/w) was characterized by spherical and smooth microparticles (Figure 1, lower panel, left). The addition of Leu did not influence the morphology of the Dex-containing DPI formulations, which displayed a wrinkled morphology at all investigated ratios (Figure 1, upper panel, right). However, the DPI formulation containing Leu/Tre displayed hollow, doughnut-like microparticles upon addition of Leu [Leu/Tre 40:60 (w/w) and Leu/Tre 60:40 (w/w)], and at the highest Leu content investigated [Leu/Tre 60:40 (w/w)], a collapsed and fragmented morphology was apparent (Figure 1, lower panel, right).
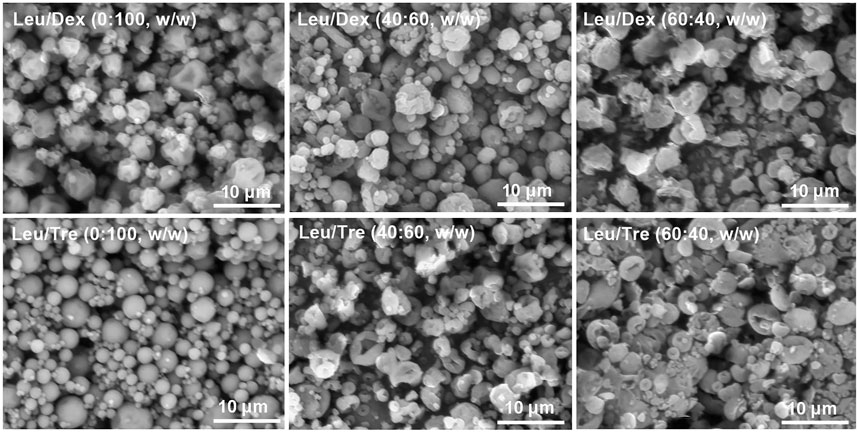
FIGURE 1. Representative SEM images (magnification 4,000 × ) of DPI formulations of siRNA-loaded LPNs, co-spray–dried with selected mixtures of excipients. Upper panel: Leu/Dex excipient mixtures (0:100, 40:60, and 60:40, w/w). Lower panel: Leu/Tre excipient mixtures (1:100, 40:60, and 60:40, w/w).
The X-ray diffractograms of DPI formulations of siRNA-loaded LPNs containing Leu/Dex 0:100 (w/w) (Figure 2A) and Leu/Tre 0:100 (w/w) (Figure 2B) displayed a halo, which suggests an amorphous state. DPI formulations containing Leu/Dex showed distinct peaks at a Leu content of 20% (w/w) representing a crystalline state of Leu, but powders containing Leu/Tre were largely amorphous, which indicates that replacing Tre with Dex prevents crystallization of Leu at a lower Leu content. The peaks observed for all other DPI formulations with higher content of Leu correspond to a partially crystalline state of Leu [6.00, 19.04, 24.41, 31.04, and 33.64 2(θ)], which is distinct from the crystalline state of unprocessed Leu (Suplementary Figure S1). This suggests that the DPI formulations are not in a fully crystalline state, as compared to the crystalline state of unprocessed Leu.
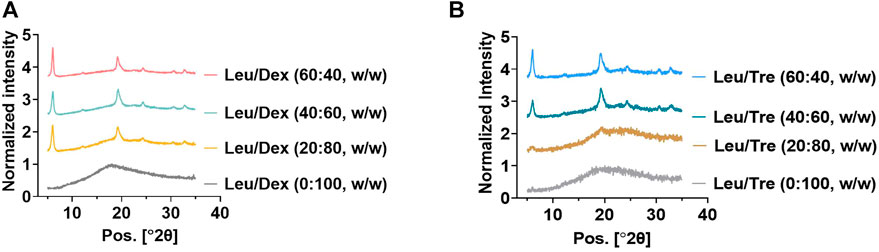
FIGURE 2. Representative diffractograms of DPI formulations of siRNA-loaded LPNs, which have been spray-dried with excipient mixtures of (A) Leu and Dex (Leu/Dex) and (B) Leu and Tre (Leu/Tre) at different weight ratios (0:100, 20:80, 40:60, and 60:40, w/w).
The aerosol performance of the DPI formulations of siRNA-loaded LPNs was investigated using the PI system, and the
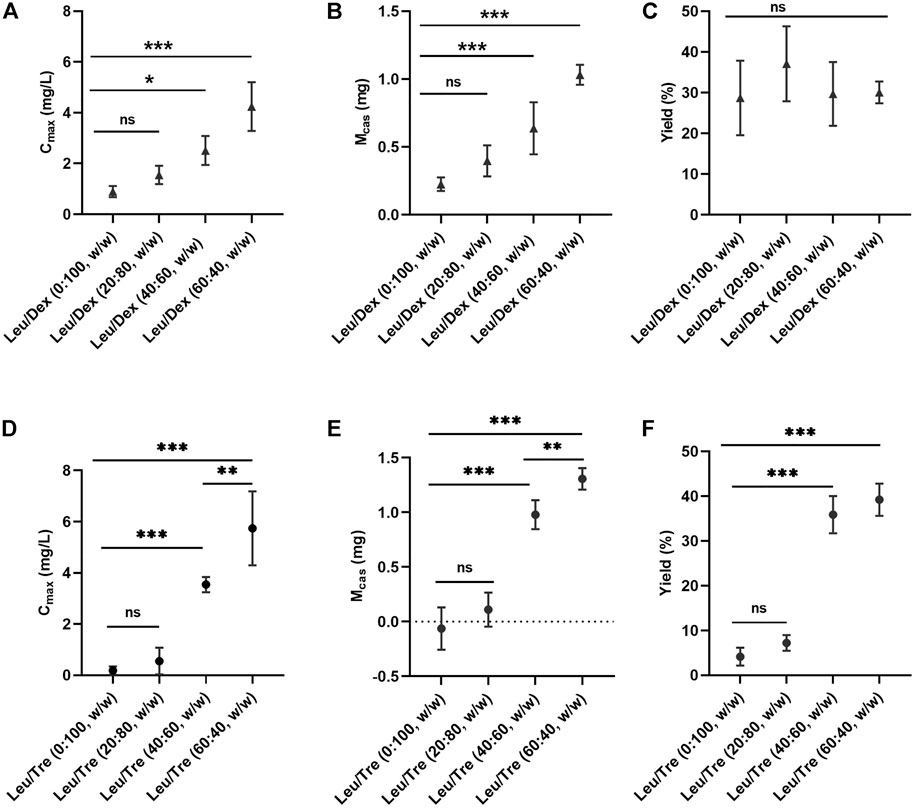
FIGURE 3. Aerosol performance, that is, Cmax (A,D), Mcas (B,E), and aerosol yield (C,F), of DPI formulations of siRNA-loaded LPNs, which have been co-spray–dried using excipient mixtures of Leu and saccharide (Dex or Tre) at different weight ratios (0:100, 20:80, 40:60, and 60:40, w/w) of Leu/Dex (A–C) and Leu/Tre (D–F). Data represent mean values ± standard deviation (n = 3). Results significantly different from the 0:100 (w/w) ratio indicate: p < 0.05 (*), p < 0.01 (**), and p < 0.001 (***).
For DPI formulations of siRNA-loaded LPNs containing Leu/Dex and Leu/Tre, the excipient ratio (w/w) influenced the physiochemical properties of the LPNs and dry powders after spray drying. Based on the physicochemical properties of the LPNs before and after spray drying, the solid state, and the aerodynamic properties of the DPI formulations of siRNA-loaded LPNs (Supplementary Table S3), the DPI formulations containing Leu/Dex (40:60, w/w) and Leu/Tre (40:60, w/w) were selected for further analysis. The resulting DPI formulations displayed FPFs of 56% and 58% for formulations containing Leu/Dex and Leu/Tre, respectively, thus exhibiting promising aerosol performance. The ED of the DPI formulations containing Leu/Dex (40:60, w/w) and Leu/Tre (40:60, w/w) were 93% and 91%, respectively (Supplementary Table S3). In addition, DPI formulations containing 40% Leu and a Tre/Dex ratio of 10:90 (w/w) optimized previously (Xu et al., 2022) were used to study the effect of trinary excipient mixtures on the long-term stability.
Leu Is Enriched on the Microparticle Surface and Decreases Water Sorption
The surface composition of the microparticles was determined using XPS. The DPI formulations exhibited XPS-detectable atom signals from the surface of the microparticles, that is, C, O, and N. No N was detected from the DPI formulations containing Dex 100% and Tre 100%. As a control, Leu 100% was tested where the theoretical and measured N values were similar. The main N contribution comes from Leu, which is likely present on the surface of the microparticles, because the measured N content was higher than the theoretical N content (Supplementary Table S4). For DPI formulations in combination of Leu with saccharides, Leu/Tre (40:60, w/w), Leu/Dex (40:60, w/w), and Leu/(Tre/Dex) [(40:60 w/w (10:90, w/w)], the measured N concentration was 7.33%, 7.21%, and 7.73%, respectively, where the N was detected from the surface of the DPI formulations. The measured N values were higher than the theoretical values for all three formulations.
The behavior of DPI formulations of siRNA-loaded LPNs upon exposure to different RH conditions at 25°C was investigated by exposing the samples to a sorption cycle (from 0% to 90% RH with a 10% RH step size), followed by an immediate desorption cycle. DPI formulations containing excipient mixtures of Leu/Tre (40:60, w/w), Leu/Dex (40:60, w/w), and Leu/(Tre/Dex) [(40:60 w/w (10:90, w/w)] were analyzed (Figure 4A). DPI formulations co-spray–dried with Leu, Tre, and Dex, respectively, were used as controls. DPI formulations containing Leu displayed very low water adsorption (approximately 0.9% water at 90% RH), because Leu crystallizes early in the drying process and forms a shell on the microparticle surface. For Dex, the maximum weight gain was 36% at 90% RH, which indicates that the moisture uptake capacity of this formulation is rather large. When using Tre as an excipient, the DPI formulations gradually adsorbed moisture when the RH was increased from 0% to 50%. As the RH increased to 50%, there was a small drop in the mass of the formulation, which is the critical RH. The DPI formulations containing Leu/Tre (40:60, w/w) demonstrated similar sorption and desorption characteristics as the DPI formulation containing 100% Tre, but these formulations can withstand a higher RH, that is, increase in the critical RH to 70% with only 5.2% weight gain of the DPI formulation at 90% RH. For DPI formulations containing Leu/Dex (40:60, w/w), the sample is still efficient in preventing moisture intake compared to Dex 100% (Figure 4A), and 19% weight gain of the DPI formulation was observed after the sorption process. Co-spray-drying with a ternary excipient mixture of Leu/(Tre/Dex) [40:60 w/w (10:90 w/w)] resulted in a DPI formulation displaying a low moisture uptake (20% weight gain of the formulation) and no moisture-induced recrystallization of Tre.
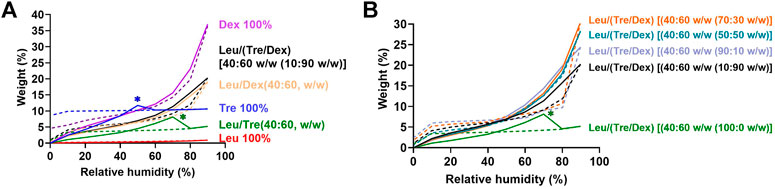
FIGURE 4. Representative DVS isotherms of control DPI formulations co-spray–dried with the excipients Leu 100% (red), Tre 100% (blue), and Dex 100% (purple), and excipient mixtures Leu/Tre (40:60, w/w, green), Leu/Dex (40:60, w/w, yellow), and Leu/(Tre/Dex) [40:60 w/w (10:90 w/w)], black (A). DVS isotherms of DPI formulations prepared with different mass ratios of Leu, Tre, and Dex (B). The “*” represents the critical relative humidity for powdered water adsorption. When this value is exceeded, crystallization of the sample and expulsion of excess water occur. The solid and dotted lines represent sorption and desorption processes, respectively.
The influence of the Tre content in the Leu/(Tre/Dex) formulations on the recrystallization of Tre was investigated further (Figure 4B). The formulations were prepared at mass ratios of Leu/(Tre/Dex) [40:60 w/w (10:90 w/w)], [40:60 w/w (70:30 w/w)], [40:60 w/w (50:50 w/w)], [40:60 w/w (90:10 w/w)], and [40:60 w/w (100:0 w/w)]. The results showed no recrystallization of Tre with an increased Tre content in the formulations, even at 90% Tre. The maximum weight gain increased from 20% to 30% when increasing the Tre content at 90% RH.
Addition of Leu to Tre-Containing DPI Formulations Increases
The thermal properties of the DPI formulations were investigated using DSC, and thermograms were recorded for control DPI formulations co-spray–dried with Leu 100%, Tre 100%, and Dex 100%, respectively, and binary/ternary mixtures of Leu/Tre (40:60, w/w), Leu/Dex (40:60, w/w), and Leu/(Tre/Dex) [40:60 w/w (10:90, w/w)] (Figure 5A). No
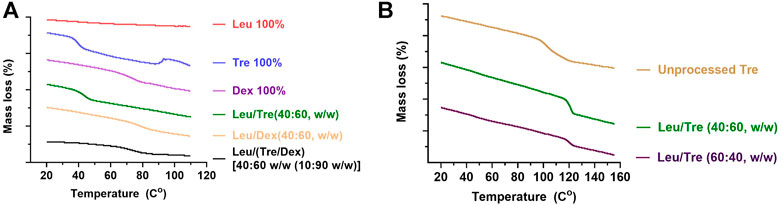
FIGURE 5. Representative thermograms of control LPN formulations co-spray–dried with the excipients Leu 100% (red), Tre 100% (blue), and Dex 100% (purple), and excipient mixtures Leu/Tre (40:60, w/w, green), Leu/Dex (40:60, w/w, yellow), and Leu/(Tre/Dex) [40:60 w/w (10:90 w/w), black] (A). Thermograms for the “heat–cool–heat” method used to completely dry the DPI formulations. Unprocessed Tre (yellow), Leu/Tre (40:60, w/w, green), and Leu/Tre (60:40, w/w, purple) (B).
The Physical Stability of LPNs and the Chemical integrity of siRNA Is Preserved After Storage for 6 months
The DPI formulations of siRNA-loaded LPNs co-spray–dried with Leu and saccharide mixtures displayed preserved physicochemical properties upon reconstitution of the DPI formulations after storage for 6 months (Supplementary Figure S2). The z-average of the LPNs reconstituted from DPI formulations containing Tre 100% stored at 25°C/58% RH was increased after 1 month of storage, and also displayed a higher z-average after 6 months of storage at 25°C/3% RH. In contrast, there were no pronounced differences in the z-average, PDI, and zeta-potential of LPNs co-spray–dried with Leu/Tre (40:60, w/w), Leu/Dex (40:60, w/w), and Leu/(Tre/Dex) [40:60 w/w (10:90 w/w)] excipient mixtures after storage for 6 months at 25°C/3% RH, 25°C/58% RH, and 40°C/3% RH, respectively (Supplementary Figure S2).
The EE (%) of siRNA in LPNs, measured using HPLC, was 47.24 ± 2.12% before spray drying (Supplementary Table S5). After 3 months of storage, the siRNA encapsulated in DPI formulations prepared with single excipients was susceptible to both high temperature and moisture environments, while formulations prepared with combinations of Leu and saccharides maintained higher EE (%). However, 44%, 39%, and 32% of siRNA were maintained after 3 months of storage at 25°C/3% RH, 25°C/58% RH, and 40°C/3% RH, respectively.
The Solid-State of DPI Formulations Is Preserved in the Presence of Dex
The MMAD of the DPI formulations during 6 months of storage was investigated using APS (Supplementay Figure S3). The MMAD of the formulations containing Tre was 100% increased after 1 month of storage at 25°C/58% RH. Since this formulation was agglomerated after 6 months of storage at 25°C/3% RH, the MMAD was not measured at this time point. The MMAD of DPI formulations prepared with Leu 100%, Dex 100%, Leu/Tre (40:60, w/w), Leu/Dex (40:60, w/w), and Leu/(Tre/Dex) [40:60 w/w (10:90 w/w)] ranged from 2 to 5 µm and remained unchanged during 6 months of storage under three different conditions. For DPI formulations containing Leu 100% and Tre 100%, the morphology of DPI formulations changed significantly during storage under all investigated conditions (Figure 6). The DPI formulations containing Leu 100% displayed more fragmented particles after storage. Formulations containing Tre 100% tend to form aggregates after 6 months of storage at 25°C/3% RH and 25°C/58% RH. However, formulations prepared with Dex 100%, Leu/Tre (40:60, w/w), Leu/Dex (40:60, w/w), and Leu/(Tre/Dex) [40:60 w/w (10:90 w/w)] displayed a well-protected particle morphology without visible aggregates under all three environmental conditions.
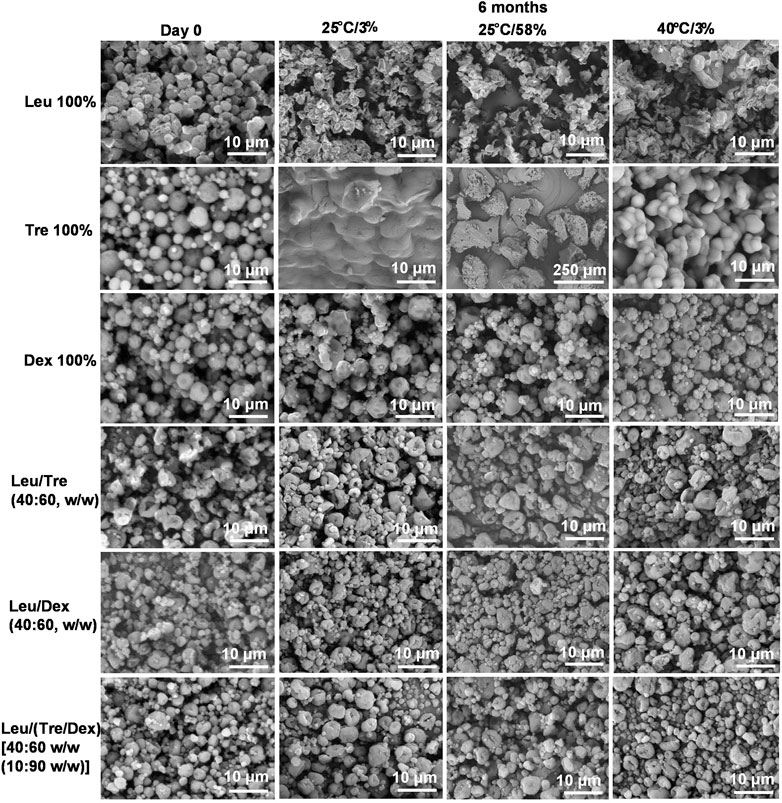
FIGURE 6. Representative SEM images of DPI formulations of siRNA-loaded LPNs, co-spray–dried with different excipients (Leu 100%, Tre 100%, Dex 100%, Leu:Tre (40:60, w/w), Leu:Dex (40:60, w/w), and Leu/(Tre/Dex) [40:60 w/w (10:90 w/w)]), on day 0 (left column) and 6 months after storage at 25°C/3% RH (middle left column), 25°C/58% RH (middle right column), and 40°C/3% RH (right column). The scale bars represent 10 µm (microscopic magnification 4,000 × ). The scale bar for the DPI formulation containing Tre 100% and stored at 25°C/58% RH (middle right column) is 250 µm.
The solid state of the DPI formulations after 6 months of storage was investigated using XRPD (Figure 7). DPI formulations spray-dried with Leu 100% were not stable under high temperature and high moisture conditions, that is, 40°C and 58% RH, and displayed additional crystalline peaks [6.00, 12.25, 15.24, 19.04, 22.28, 24.41, 31.04, and 33.64 2(θ)] after 6 months of storage. Tre 100% and Leu/Tre formulations were also in a crystalline state after 6 months of storage at 25°C/3% RH. However, Tre 100% and Leu/Tre formulations were stable at high temperature, that is, 40°C/3% RH after 6 months of storage. The DPI formulations prepared with Tre 100% under 25°C/58% RH recrystallized after 3 days and displayed an almost fully crystalline state with structural rearrangement after 2 weeks of storage (Supplementary Figure S4). In contrast, the solid state of the DPI formulations containing Dex remained unchanged under both high humidity and high temperature conditions. The XRPD diffractograms for DPI formulations prepared with Dex 100% including Dex 100%, Leu/Dex (40:60 w/w), and Leu/(Tre/Dex)) [40:60 w/w (10:90 w/w)], stored for 6 months under three different conditions, were similar to those obtained at day 0.
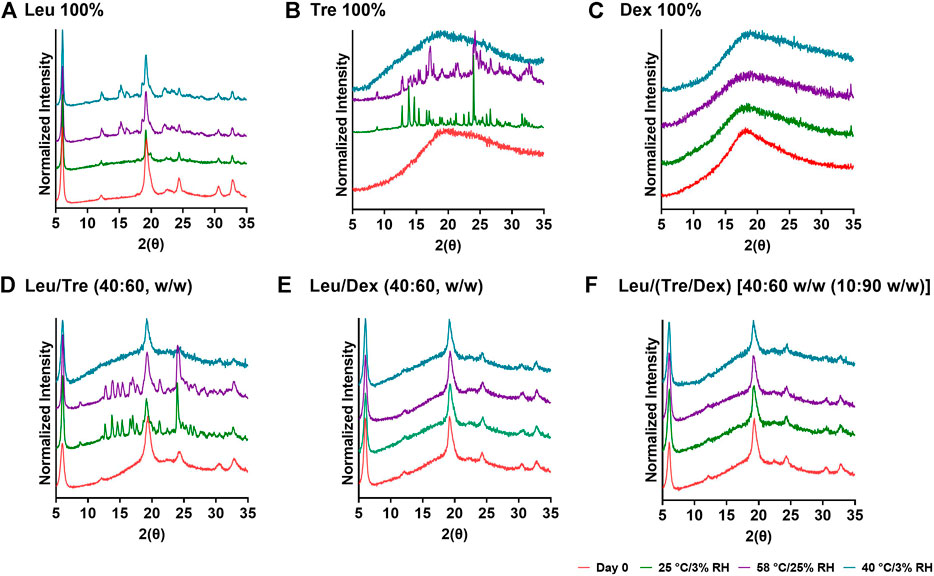
FIGURE 7. Representative diffractograms of DPI formulations of siRNA-loaded LPNs, which were co-spray–dried with Leu 100% (A) Tre 100% (B) or Dex 100% (C) or their combinations Leu/Tre (40:60, w/w), Leu/Dex (40:60, w/w), and Leu/(Tre/Dex) [40:60 w/w (10:90 w/w)] (D–F), at day 0 (red) and after 6 months of storage at 25°C/3% RH (green), 25°C/58% RH (purple), and 40°C/3% RH (blue), respectively.
The combination of Leu with saccharides affected the moisture uptake of the DPI formulations during long-term storage (Supplementary Table S6). Leu-containing formulations displayed strikingly low moisture content (approximately 0%), which can be attributed to the crystalline state of Leu. In the absence of Leu, DPI formulations containing Tre 100% (8.33%) and Dex 100% (8.58%) both had a high moisture content after 6 months of storage at 25°C/3% RH. Leu/Tre (40:60, w/w) and Leu/Dex (40:60, w/w) had a moisture content of approximately 5%, while Leu/(Tre/Dex)) [40:60 w/w (10:90 w/w)] had a lower moisture content of 3.28%. In the absence of Leu, formulations containing Tre 100% (8.61%) and Dex 100% (7.96%) had a high moisture content after 6 months of storage at 25°C/58% RH. The combinations of 40% Leu, that is, Leu/Tre (40:60, w/w), Leu/Dex (40:60, w/w), and Leu/(Tre/Dex)) [40:60 w/w (10:90 w/w)] displayed a relatively low moisture content at 25°C/58% RH, which was similar to that measured after storage at 25°C/3% RH.
Combining Leu With Saccharides Results in Preserved Aerosol Performance
The aerosolization performance of the DPI formulations was assessed at day 0 and after 1, 3, and 6 months of storage at 25°C/3% RH, 25°C/58% RH, and 40°C/3% RH, respectively. The DPI formulations containing Leu 100% displayed the highest
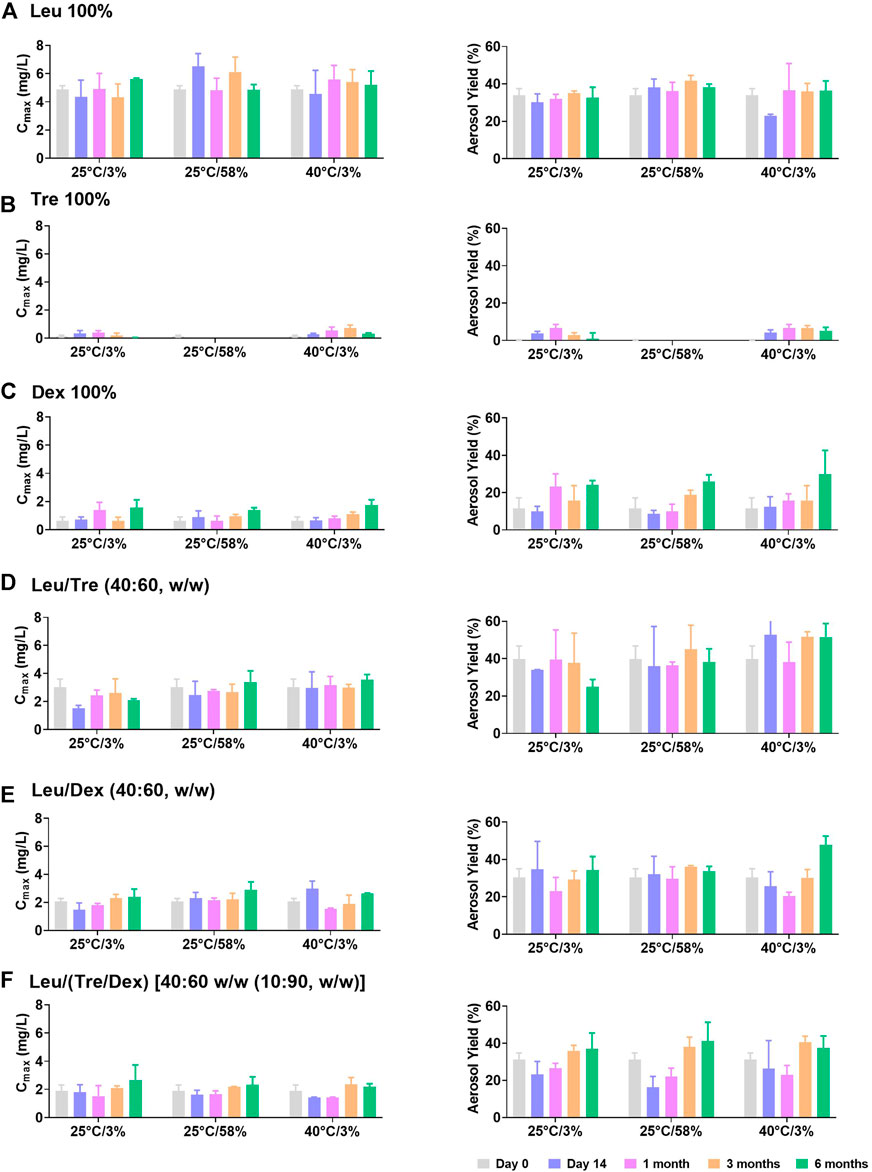
FIGURE 8. Maximum concentration in Cmax and aerosol yield of DPI formulations of siRNA-loaded LPNs, which were co-spray–dried with Leu 100% (A), Tre 100% (B), or Dex 100% (C) or their combinations Leu/Tre (40:60, w/w), Leu/Dex (40:60, w/w), and Leu/(Tre/Dex) [40:60 w/w (10:90), w/w] (D–F), at day 0 and after 6 months of storage at 25°C/3% RH, 25°C/58% RH, and 40°C/3% RH, respectively. Color code: DPI formulations on day 0 (gray), day 14 (blue), 1 month (purple), 3 months (yellow), and 6 months (green). Data represent mean values ± SD (n = 3).
Discussion
The aim of this study was to investigate the effect of the anti-adhesive excipient Leu on the aerosol performance and long-term storage stability of DPI formulations of siRNA-loaded LPNs co-spray–dried with Leu and either the polysaccharide Dex or the disaccharide Tre, or their combination (Leu/Tre/Dex), as the stabilizing excipients. The main findings are as follows: 1) DPI formulations of siRNA-loaded LPNs, which were co-spray–dried with Leu/Dex and Leu/Tre, display different physiochemical properties after spray drying, for example, moisture content, aerosol performance, and long-term storage stability; 2) combining Dex with Tre can efficiently prevent recrystallization of Tre in a high moisture environment; and 3) the combined use of Leu and Dex is important for maintaining the long-term stability of DPI formulations of siRNA-loaded LPNs designed for inhalation.
Dex and Tre influence the physicochemical properties of re-dispersed siRNA-loaded LPNs to different extents. The z-average of reconstituted LPNs increased after spray drying when using Dex and Leu/Dex, which suggests incomplete stabilization during the spray drying process. In contrast, formulations containing Tre and Leu/Tre have relatively smaller z-averages after spray drying, which might be related to better stabilization as a result of higher coverage of the surface by the disaccharide Tre, than by the polysaccharide Dex, which prevents aggregation of LPNs (Tonnis et al., 2015). Tre might provide structural and conformational stability through direct hydrogen bond formation to the LPNs, or by vitrifying the LPNs in a glassy state, thereby restricting the mobility, eventually preventing LPN aggregation (Ingvarsson et al., 2011).
In addition, the combination of Leu with saccharides affects the solid state. When Leu is combined with saccharides, the mobility of the crystallized components is reduced due to the increased viscosity of the solutions at higher concentrations (Galmarini et al., 2011). Since the saturation of Leu is low in the mixture with the LPNs, the saccharides will reach the true density (tt) before the formation of Leu nucleates (tc), hence inhibiting crystallization of Leu. Therefore, formulations containing a high saccharide content/low Leu content form a highly amorphous form of powder (Chew et al., 2005). Based on XRPD analysis, Leu/Tre was almost fully amorphous at 20% Leu (w/w), while Leu/Dex displayed a partially crystalline state of Leu at 20% (w/w). Accordingly, 20% (w/w) Leu is the critical concentration for Leu/Dex formulation to begin shell-formation around the microparticles, whereas this is inhibited by Tre. XPS measurements of two different N environments of Leu/Tre formulation suggest that the difference in the solid state between Leu/Tre and Leu/Dex is caused by a possible interaction between Leu and Tre. For DPI formulations containing binary Leu/Tre mixtures, the Leu component may not form a continuous surface shell coverage on the surface of the microparticles, but the surface might rather consist of a mixture of Leu and Tre. The
A high aerosol performance is essential when developing DPI formulations. However, prediction of the aerosol performance is challenging, because it is influenced by a number of different powder properties, that is, the microparticle morphology, the residual moisture content, the MMAD, and the surface composition. In this study, the aerosol performance (Cmax and Mcas) of the DPI formulations was improved when increasing the Leu content for both Leu/Tre and Leu/Dex DPI formulations. This is due to the fact that the combination of Leu with saccharides results in 1) decreased cohesion of the microparticles by changing the morphology, 2) reduced moisture content by decreasing the amorphous content, and 3) lower MMAD. Particles with a corrugated surface morphology have been shown to display a higher aerosol performance, as compared to smooth particles, because particle agglomeration is prevented (Chew et al., 2005). The morphology, for example, a corrugated dry powder surface, is the result of various interacting physical and chemical mechanisms during the particle formation process, which can be explained by the related Peclet number. Compared with Tre solutions, Dex solutions at equal mass concentrations have a higher viscosity and, in turn, a lower diffusion coefficient and a higher Peclet number (Vehring et al., 2007). For a large Peclet number (Leu crystals and Dex), the component is expected to be relatively immobile and hence accumulates close to the droplet surface. When the solvent evaporates, the surface tends to shrink into a corrugated surface. In addition, Dex has a higher surface tension in water than Tre, which plays an important role during spray drying for the resulting particle morphology (Kadota et al., 2019). Since both excipients tend to affect the morphology of a corrugated surface, the addition of Leu did not significantly influence the particle morphology of Dex-containing DPI formulations. Therefore, although the
Furthermore, with the increased Leu content and reduced saccharide content, the moisture content decreased due to the decreased amorphous content and density, which also improved the aerosolization behavior of the DPI formulations. The DPI formulations containing binary Leu/Dex mixtures displayed a higher moisture content than DPI formulations containing Leu/Tre mixtures, eventually resulting in lower flowability of the DPI formulations containing Leu/Dex mixtures. More specifically, the DPI formulations of siRNA-loaded LPNs co-spray–dried with binary mixtures of Leu/Dex (40:60, w/w) and Leu/Tre (40:60, w/w) had moisture contents of 2.8% and 1.2%, respectively. The ability to prevent moisture adsorption is important for 1) long-term stability of DPI formulations and 2) the ability to overcome the barriers related to lung geometry and the physiological conditions (approximately 90% RH) in the lungs (Xu et al., 2021). Both DPI formulations Leu/Dex (40:60, w/w) and Leu/Tre (40:60, w/w) displayed similar water adsorption during long-term storage.
The physical stability of DPI formulations is critical to ensure the delivery of a reproducible drug dose to the airways. DPI formulations containing Tre 100% and Dex 100% are in an amorphous state after spray drying, which is hygroscopic and has a tendency to adsorb moisture [5]. The weight gain (%) of DPI formulations containing Tre 100% and Dex 100% increased after storage, either at 3% or 58% RH, which eventually resulted in microparticle agglomeration and a subsequent decrease in the aerosol performance (Shetty et al., 2020). Leu adsorbs on the surface of the droplets and forms a hydrophobic layer, which hinders diffusion of water (Mangal et al., 2015). Amorphous samples/parts are thermodynamically unstable with a high risk of recrystallization when exposed to moisture (Chang et al., 2017). According to the DVS measurements and the long-term storage stability data, Leu significantly improved the long-term storage stability of DPI formulations containing Leu/Tre (40:60, w/w), but the addition of 40% (w/w) Leu was not sufficient to prevent recrystallization of Tre during storage. This recrystallization of DPI formulations containing Leu/Tre (40:60, w/w) can be explained by the discontinuous surface coverage of the microparticles with Leu, which results in moisture uptake, eventually leading to the formation of solid bridges or hard cakes. The powders adsorbed moisture resulting in a mass increase, and subsequent recrystallization caused a decrease in mass, because the phase transition from an amorphous phase to a crystalline phase results in the exclusion of water from the crystal lattice (Chang et al., 2019), as shown using DVS. DPI formulations are highly hygroscopic, and hence they require a particular storage environment to avoid moisture adsorption. In addition, although Leu is in a crystalline state after spray drying, the crystal structure of DPI formulations containing 100% Leu is not stable during storage at 25°C/58% RH and 40°C/3% RH, because significant structural rearrangement occurs after 6 months of storage. In contrast, DPI formulations containing Leu/Dex (40:60, w/w) showed long-term storage stability at both high temperature and high moisture conditions. Data on Leu/(Tre/Dex) formulation showed that Tre does not recrystallize, even at 6% (w/w) Dex concentration [(40:60 w/w (10:90) w/w], which indicates that Dex prevents Tre and Leu from recrystallization. Therefore, the combined use of the disaccharide Tre and the polysaccharide Dex is important for maintaining the long-term stability of DPI formulations containing Leu. In addition, the microparticle morphology, the residual moisture content, the MMAD, and the aerosol performance of the DPI formulations after 6 months of storage under exaggerated conditions were comparable to those measured on day 0. Therefore, we expect that these formulations display similar in vivo biodistribution, which was investigated in our previous study (Xu et al., 2022). Since the integrity of siRNA after storage was also preserved after 6 months of storage, we also expect preserved bioactivity.
Conclusion
Understanding the solid-state properties of DPI formulations and how they affect aerosol performance and long-term stability is fundamental to the success of pulmonary drug delivery. Improvement in the flowability, aerosol yield, and long-term stability of DPI formulations of siRNA-loaded LPNs was achieved using excipient mixtures of Leu and saccharide as protectants during the spray drying process. In the absence of Leu, DPI formulations containing Tre 100% and Dex 100% displayed a high moisture content after storage, which influences the morphology and long-term stability of the DPI formulations. Increasing the Leu mass fraction in the DPI formulation leads to the reduced particle size and moisture content, as a consequence of reduced cohesiveness. A minimum Leu threshold must be exceeded to reach these advantages, for example, an amount of 40% Leu (w/w) is needed to achieve a high aerosol performance when combining Leu with saccharides. Mixing Leu with the disaccharide Tre is not sufficient to preserve the long-term storage stability of LPNs in the solid state, because additional crystalline structures appeared after 6 months of storage, which may ultimately lead to instability of the DPI formulations. For hygroscopic amorphous DPI formulations, for example, Leu/Tre, a critical storage environment is necessary. However, the combination of polysaccharide (Dex) and Leu ensures long-term physical stability of DPI formulations and chemical stability of siRNA, which is favorable for nanocarrier-based DPI formulations. This study shows that the combined use of polysaccharide and Leu is promising for the design of thermostable DPI formulations.
Data Availability Statement
The original contributions presented in the study are included in the article/Supplementary Material; further inquiries can be directed to the corresponding author.
Author Contributions
YX, ET, AT, and CF drafted the project and designed the experiments with contributions from all authors. YX and ET performed the experiments. ZS contributed to the solid state characterization of the DPI formulations. HF synthesized, purified, and characterized L5N12. ZS and AT contributed to evaluating the aerosol performance of DPI formulations. YX, ET, AT, and CF contributed to data analysis and interpretation. YX and ET drafted the manuscript with subsequent contributions from all authors.
Funding
We gratefully acknowledge the support from the Innovative Medicines Initiative Joint Undertaking under grant agreement No. 115363 resources which are composed of financial contribution from the European Union’s Seventh Framework Programme (FP7/2007-2013) and EFPIA companies’ in kind contribution. This project has received funding from the European Union’s Seventh Framework Programme for research, technological development and demonstration under grant agreement No. 600207. We acknowledge the Drug Research Academy Graduate Programme in Pharmaceutical Sciences, University of Copenhagen, for funding the Small-Scale Powder Disperser. We are also grateful to the Lundbeck Foundation—Denmark (R219-2016-908), the Novo Nordisk Foundation—Denmark (Grant No. NNF17OC0026526 to CF), Independent Research Fund Denmark (Grant Nos 4184-00,422 and 9041-00198B to CF), and the Hørslev-Fonden—Denmark for financial support. We acknowledge the China Scholarship Council (CSC) for the scholarship to YX (201906210064).
Conflict of Interest
The authors declare that the research was conducted in the absence of any commercial or financial relationships that could be construed as a potential conflict of interest.
Publisher’s Note
All claims expressed in this article are solely those of the authors and do not necessarily represent those of their affiliated organizations, or those of the publisher, the editors, and the reviewers. Any product that may be evaluated in this article, or claim that may be made by its manufacturer, is not guaranteed or endorsed by the publisher.
Acknowledgments
We are grateful to Per Gerde, Ewa Selg, and Carl-Olof Sjöberg for excellent technical support and analysis of data generated using the PreciseInhale® system. We thank Abhijeet Girish Lokras and Akash Chakravarty for synthesizing L5N12. Novo Nordisk, Denmark, has kindly supplied the Aerodynamic Particle Sizer Spectrometer 3321 and the Next Generation Pharmaceutical Impactor.
Supplementary Material
The Supplementary Material for this article can be found online at: https://www.frontiersin.org/articles/10.3389/fddev.2022.945459/full#supplementary-material
References
Akinc, A., Zumbuehl, A., Goldberg, M., Leshchiner, E. S., Busini, V., Hossain, N., et al. (2008). A Combinatorial Library of Lipid-like Materials for Delivery of RNAi Therapeutics. Nat. Biotechnol. 26 (5), 561–569. doi:10.1038/nbt1402
Bosquillon, C., Lombry, C., Préat, V., and Vanbever, R. (2001). Influence of Formulation Excipients and Physical Characteristics of Inhalation Dry Powders on Their Aerosolization Performance. J. Control. Release 70 (3), 329–339. doi:10.1016/s0168-3659(00)00362-x
Chang, R. Y. K., Wallin, M., Kutter, E., Morales, S., Britton, W., Li, J., et al. (2019). Storage Stability of Inhalable Phage Powders Containing Lactose at Ambient Conditions. Int. J. Pharm. 560, 11–18. doi:10.1016/j.ijpharm.2019.01.050
Chang, R. Y., Wong, J., Mathai, A., Morales, S., Kutter, E., Britton, W., et al. (2017). Production of Highly Stable Spray Dried Phage Formulations for Treatment of Pseudomonas aeruginosa Lung Infection. Eur. J. Pharm. Biopharm. 121, 1–13. doi:10.1016/j.ejpb.2017.09.002
Chew, N. Y. K., Shekunov, B. Y., Tong, H. H. Y., Chow, A. H. L., Savage, C., Wu, J., et al. (2005). Effect of Amino Acids on the Dispersion of Disodium Cromoglycate Powders. J. Pharm. Sci. 94 (10), 2289–2300. doi:10.1002/jps.20426
Dormenval, C., Lokras, A., Cano-Garcia, G., Wadhwa, A., Thanki, K., Rose, F., et al. (2019). Identification of Factors of Importance for Spray Drying of Small Interfering RNA-Loaded Lipidoid-Polymer Hybrid Nanoparticles for Inhalation. Pharm. Res. 36 (10), 142. doi:10.1007/s11095-019-2663-y
Fioni, A., Selg, E., Cenacchi, V., Acevedo, F., Brogin, G., Gerde, P., et al. (2018). Investigation of Lung Pharmacokinetic of the Novel PDE4 Inhibitor CHF6001 in Preclinical Models: Evaluation of the PreciseInhale Technology. J. Aerosol Med. Pulm. Drug Deliv. 31 (1), 61–70. doi:10.1089/jamp.2017.1369
Galmarini, M. V., Baeza, R., Sanchez, V., Zamora, M. C., and Chirife, J. (2011). Comparison of the Viscosity of Trehalose and Sucrose Solutions at Various Temperatures: Effect of Guar Gum Addition. LWT - Food Sci. Technol. 44 (1), 186–190. doi:10.1016/j.lwt.2010.04.021
Ingvarsson, P. T., Yang, M., Nielsen, H. M., Rantanen, J., and Foged, C. (2011). Stabilization of Liposomes during Drying. Expert Opin. Drug Deliv. 8 (3), 375–388. doi:10.1517/17425247.2011.553219
Jansen, M. A. A., Klausen, L. H., Thanki, K., Lyngsø, J., Skov Pedersen, J., Franzyk, H., et al. (2019). Lipidoid-polymer Hybrid Nanoparticles Loaded with TNF siRNA Suppress Inflammation after Intra-articular Administration in a Murine Experimental Arthritis Model. Eur. J. Pharm. Biopharm. 142, 38–48. doi:10.1016/j.ejpb.2019.06.009
Jensen, D. K., Jensen, L. B., Koocheki, S., Bengtson, L., Cun, D., Nielsen, H. M., et al. (2012). Design of an Inhalable Dry Powder Formulation of DOTAP-Modified PLGA Nanoparticles Loaded with siRNA. J. Control. Release 157 (1), 141–148. doi:10.1016/j.jconrel.2011.08.011
Kadota, K., Yanagawa, Y., Tachikawa, T., Deki, Y., Uchiyama, H., Shirakawa, Y., et al. (2019). Development of Porous Particles Using Dextran as an Excipient for Enhanced Deep Lung Delivery of Rifampicin. Int. J. Pharm. 555, 280–290. doi:10.1016/j.ijpharm.2018.11.055
Lechanteur, A., and Evrard, B. (2020). Influence of Composition and Spray-Drying Process Parameters on Carrier-free DPI Properties and Behaviors in the Lung: A Review. Pharmaceutics 12 (1), 55. doi:10.3390/pharmaceutics12010055
Lokras, A., Thakur, A., Wadhwa, A., Thanki, K., Franzyk, H., and Foged, C. (2020). Optimizing the Intracellular Delivery of Therapeutic Anti-inflammatory TNF-α siRNA to Activated Macrophages Using Lipidoid-Polymer Hybrid Nanoparticles. Front. Bioeng. Biotechnol. 8, 601155. doi:10.3389/fbioe.2020.601155
Mah, P. T., O'Connell, P., Focaroli, S., Lundy, R., O'Mahony, T. F., Hastedt, J. E., et al. (2019). The Use of Hydrophobic Amino Acids in Protecting Spray Dried Trehalose Formulations against Moisture-Induced Changes. Eur. J. Pharm. Biopharm. 144, 139–153. doi:10.1016/j.ejpb.2019.09.014
Mangal, S., Meiser, F., Tan, G., Gengenbach, T., Denman, J., Rowles, M. R., et al. (2015). Relationship between Surface Concentration of L-Leucine and Bulk Powder Properties in Spray Dried Formulations. Eur. J. Pharm. Biopharm. 94, 160–169. doi:10.1016/j.ejpb.2015.04.035
Selg, E., Ewing, P., Acevedo, F., Sjöberg, C.-O., Ryrfeldt, Å., and Gerde, P. (2013). Dry Powder Inhalation Exposures of the Endotracheally Intubated Rat Lung, Ex Vivo and In Vivo: the Pulmonary Pharmacokinetics of Fluticasone Furoate. J. Aerosol Med. Pulm. Drug Deliv. 26 (4), 181–189. doi:10.1089/jamp.2012.0971
Shaffer, C. (2020). Mist Begins to Clear for Lung Delivery of RNA. Nat. Biotechnol. 38 (10), 1110–1112. doi:10.1038/s41587-020-0692-z
Shetty, N., Cipolla, D., Park, H., and Zhou, Q. T. (2020). Physical Stability of Dry Powder Inhaler Formulations. Expert Opin. Drug Deliv. 17 (1), 77–96. doi:10.1080/17425247.2020.1702643
Tarara, T. E., Miller, D. P., Weers, A. E., Muliadi, A., Tso, J., Eliahu, A., et al. (2022). Formulation of Dry Powders for Inhalation Comprising High Doses of a Poorly Soluble Hydrophobic Drug. Front. Drug. Deliv. 2. 862336. doi:10.3389/fddev.2022.862336
Thanki, K., Zeng, X., and Foged, C. (2019). Preparation, Characterization, and In Vitro Evaluation of Lipidoid-Polymer Hybrid Nanoparticles for siRNA Delivery to the Cytosol. Methods Mol. Biol., 1943, 141–152. doi:10.1007/978-1-4939-9092-4_9
Thanki, K., Zeng, X., Justesen, S., Tejlmann, S., Falkenberg, E., Van Driessche, E., et al. (2017). Engineering of Small Interfering RNA-Loaded Lipidoid-Poly( DL -Lactic-Co-Glycolic Acid) Hybrid Nanoparticles for Highly Efficient and Safe Gene Silencing: A Quality by Design-Based Approach. Eur. J. Pharm. Biopharm. 120, 22–33. doi:10.1016/j.ejpb.2017.07.014
Tonnis, W. F., Mensink, M. A., de Jager, A., van der Voort Maarschalk, K., Frijlink, H. W., and Hinrichs, W. L. J. (2015). Size and Molecular Flexibility of Sugars Determine the Storage Stability of Freeze-Dried Proteins. Mol. Pharm. 12 (3), 684–694. doi:10.1021/mp500423z
Vehring, R., Foss, W. R., and Lechuga-Ballesteros, D. (2007). Particle Formation in Spray Drying. J. Aerosol Sci. 38 (7), 728–746. doi:10.1016/j.jaerosci.2007.04.005
Xu, Y., Harinck, L., Lokras, A. G., Gerde, P., Selg, E., Sjöberg, C.-O., et al. (2022). Leucine Improves the Aerosol Performance of Dry Powder Inhaler Formulations of siRNA-Loaded Nanoparticles. Int. J. Pharm. 621, 121758. doi:10.1016/j.ijpharm.2022.121758
Keywords: inhalation, pulmonary delivery, aerosol performance, siRNA, stability
Citation: Xu Y, Turan ET, Shi Z, Franzyk H, Thakur A and Foged C (2022) Inhalable Composite Microparticles Containing siRNA-Loaded Lipid-Polymer Hybrid Nanoparticles: Saccharides and Leucine Preserve Aerosol Performance and Long-Term Physical Stability. Front. Drug. Deliv. 2:945459. doi: 10.3389/fddev.2022.945459
Received: 16 May 2022; Accepted: 20 June 2022;
Published: 11 July 2022.
Edited by:
Philip Chi Lip Kwok, The University of Sydney, AustraliaReviewed by:
Jenny Lam, The University of Hong Kong, Hong Kong, SAR ChinaMohammad Momin, Virginia Commonwealth University, United States
Hideyuki Sato, University of Shizuoka, Japan
Copyright © 2022 Xu, Turan, Shi, Franzyk, Thakur and Foged. This is an open-access article distributed under the terms of the Creative Commons Attribution License (CC BY). The use, distribution or reproduction in other forums is permitted, provided the original author(s) and the copyright owner(s) are credited and that the original publication in this journal is cited, in accordance with accepted academic practice. No use, distribution or reproduction is permitted which does not comply with these terms.
*Correspondence: Camilla Foged, Y2FtaWxsYS5mb2dlZEBzdW5kLmt1LmRr