CRISPR/Cas genome editing improves abiotic and biotic stress tolerance of crops
- 1College of Plant Protection, Shandong Agricultural University, Tai’an, China
- 2Hunan Tobacco Research Institute, Changsha, China
Abiotic stress such as cold, drought, saline-alkali stress and biotic stress including disease and insect pest are the main factors that affect plant growth and limit agricultural productivity. In recent years, with the rapid development of molecular biology, genome editing techniques have been widely used in botany and agronomy due to their characteristics of high efficiency, controllable and directional editing. Genome editing techniques have great application potential in breeding resistant varieties. These techniques have achieved remarkable results in resistance breeding of important cereal crops (such as maize, rice, wheat, etc.), vegetable and fruit crops. Among them, CRISPR/Cas (clustered regularly interspaced short palindromic repeats/CRISPR-associated) provides a guarantee for the stability of crop yield worldwide. In this paper, the development of CRISRR/Cas and its application in different resistance breeding of important crops are reviewed, the advantages and importance of CRISRR/Cas technology in breeding are emphasized, and the possible problems are pointed out.
Introduction
Genome editing, which involves precise modifications at specific sites in the genome to make desired changes to the DNA sequence. The key breakthrough of genome editing techniques come from the development of site-broken DNA technology. In recent years, with the development of synthetic sequence-specific nuclease (SSN), genome editing techniques have entered a period of rapid development. At present, three types of genome editing tools are widely used by researchers, including zinc finger nuclease (ZFN) (Kim et al., 1996), transcription activator-like effector nuclease (TALEN) (Boch et al., 2009; Christian et al., 2010), and clustered regularly interspaced short palindromic repeats/CRISPR associated (CRISPR/Cas) (Jinek et al., 2012; Cong et al., 2013). ZFN and TALEN have not been widely used due to complicated operation and high failure rate.
The CRISPR/Cas realizes the recognition process through the base complementation between guide RNA and target sequence, which is simple and flexible, and the target site selection only needs to conform to the requirements of protospacer-adjacent motif (PAM) of different systems. Compared to the previous two generations of genome editing techniques, the CRISPR/Cas system is simple, flexible, stable, efficient and easy to transform. These features enabled CRISPR/Cas to quickly replace ZFN and TALEN as the mainstream genome editing techniques. CRISPR/Cas is a defense system, protecting bacteria and archaea from being invaded by mobile genetic elements and bacteriophages (Hille et al., 2018). It is composed of a single-guide RNA (sgRNA), which is a simplified combination of crRNA and tracrRNA, and RNA-guided Cas endonuclease (Hu et al., 2018). During the process of genome editing, Cas endonuclease was recruited by sgRNA to a specific site of the genome to catalyze a DNA double-stranded break (DSB) which can be repaired by diverse DNA repair mechanisms, non-homologous end joining (NHEJ), microhomology-mediated end joining (MMEJ), and homology-directed repairs (HDR), resulting in gene knockout, DNA fragment insertion, deletion, and replacement as specifically required (Hua et al., 2019; Lu et al., 2020). Recently, many efforts have been focused on improving the CRISPR/Cas system to expand the genome-targeting scope of this tools. For example, SpCas9-VRQR, xCas9, and Cas9-NG variants could recognize non-canonical NGA and NG PAM sites in plant (Nishimasu et al., 2018; Ming et al., 2020). SpCas9 orthologues have been identified from Streptococcus canis (ScCas9), Staphylococcus aureus (SaCas9), Streptococcus thermophiles (St1Cas9), and Brevibacillus laterosporus (BlatCas9) and have been demonstrated to edit plant genomic loci bearing PAM sequence of NNG, NNGRRT, NNAG AAW, and NNNCND, respectively (Cong and Zhang, 2015; Tan et al., 2020). In addition, the type V Cas12a and Cas12b that isolated from diverse bacterial have been characterized with AT-rich PAM specificity, which were utilized successfully in genome editing of targeted plant (Tang et al., 2017; Wang et al., 2020b).
Since 2013, the CRISPR/Cas has successfully implemented efficient genome editing and regulation in multiple species (Mali et al., 2013; Ran et al., 2015; Xie et al., 2015; Chen et al., 2017). Although CRISPR/Cas has only recently become the preferred tool for genetic manipulation in plants, it has shown great application value in genetic improvement of crops (Wolt et al., 2016). Nowadays, the CRISPR/Cas has been widely used in improving crop yield (Zhou et al., 2019; Cai et al., 2021) and quality (Xing et al., 2020; Xu et al., 2021), enhancing abiotic stress resistance (Nieves-Cordones et al., 2017; Bouzroud et al., 2020) and biotic stress resistance (Ji et al., 2018; Oliva et al., 2019), giving crops herbicide resistance (Zhang et al., 2019b; Liu et al., 2021) and de novo crop domestication (Li et al., 2018; Zsögön et al., 2018). Wang et al. (2020a) used CRISPR/Cas9 to edit 25 amino acid sequences conserved at the C-terminal of rice cytokinin-activation enzyme-like gene LONELY GUY (OsLOGL5), and obtained edited lines that significantly increased grain yield under multiple geographical conditions (Wang et al., 2020a). 2-acetyl-1-pyrroline (2AP) is a major source of aroma, and its level can be significantly increased by impaired or deficient function of BETAINE ALDEHYDE DEHYDROGEN-ASE 2 (BADH2), which increases aroma. Using CRISPR/Cas9 to disrupt BADH2 function, the scientists created new rice (Oryza sativa), maize (Zea mays) and sorghum (Sorghum bicolor) germplasm with aroma (Wang et al., 2021a; Tang et al., 2021; Zhang et al., 2022). Alfatih et al. (2020) obtained three independent PARAQUAT TOLERANCE 3 (PQT3) functional deficiency mutants of rice using CRISPR/Cas9, and the germination rate and growth status of the mutants were significantly better than those of the wild type under oxidative stress and salt stress (Alfatih et al., 2020). 5-enolpyruvylshikimate-3-phosphate synthase (EPSPS) is a key enzyme involves in the synthesis pathway of aromatic amino acids, and is the target of glyphosate, a broad-spectrum and highly effective pesticide. Wang et al. (2021a) used a CRISPR/Cas9-mediated ho-mology directed repair (HDR) strategy to successfully replace endogenous EPSPS with EPSPSmTIPS and EPSPSmLFGAAGMCRL in rapeseed, and obtained a new line with stable inheritance and glyphosate-resistant rapeseed (Wang et al., 2021b). Yu et al. (2021) accelerated de novo domestication of wild rice by using CRISPR/Cas9 multi-gene editing targeting genes for important agronomic traits (Yu et al., 2021).
In recent years, with the development of industry and frequent occurrence of extreme climate, the natural environment has gradually developed towards the unsuitable direction for the growth of crops. Under the influence of abiotic stresses such as low temperature (Shi et al., 2018), high temperature (Tang et al., 2020), drought (Gupta et al., 2020), saline-alkali (Ismail and Horie, 2017), heavy metal (Chauhan et al., 2020), and biotic stresses including fungal, bacterial, viral diseases (Li et al., 2019) and insect pests (Liu et al., 2016), the yield and quality of crops are reduced. Traditional crossbreeding, mutagenesis breeding and other breeding methods can not meet the requirements of resistance breeding (Zhang et al., 2017). CRISPR/Cas can be used for directional improvement of crops and greatly shorten the breeding life, which has become the mainstream technology of resistance breeding at present. This paper reviews the application of CRISPR/Cas in crop re-sistance gene improvement, and puts forward the possible problems and challenges.
CRISPR/Cas gene editing improves abiotic stress tolerance of crops
Abiotic stresses such as salinity, drought, extreme temperature and heavy metals are important factors affecting plant growth and development, which can lead to 50% crop yield reduction (Liu et al., 2022). It is essential to generate crop types with greater adaptability for growth under a variety of environmental conditions in such circumstances. Though traditional breeding increases production to a large extent, it has the drawback of losing genetic variety and fitness (Wolter et al., 2019). In addition to being time-consuming, its reliance on natural allelic variants makes it difficult to create the desired characteristic and ensure the sustainability of production (Gao, 2018). Herein, we reviewed CRISPR/Cas-mediated crop plant editing to address the remarkable problem of various abiotic stressors in this paper (Table 1).
It has been reported that 7% of the Earth’s land and 20% of arable land were affected by salinization, and the situation was only going to deteriorate (Al Murad et al., 2020). Salt stress induces osmotic stress, ion stress and secondary stress in plants (Yang and Guo, 2018), which reduces yield and quality of crops (Siddiqui et al., 2017). In tomato plants, the exact deletion of SlHyPRP1 negative-response domain(s) significantly enhanced the salinity tolerance at both of the germination and vegetative stages (Tran et al., 2021). Alam et al. (2022) used CRISPR/Cas9 system to knock out OsbHLH024 gene in rice and enhance the expression of ion transporter gene OsHKT1;3, OsHAK7, and OsSOS1, enhancing salt tolerance of rice (Alam et al., 2022). Mutation of OsRR22 gene induced by CRISPR/Cas9 enhanced salt tolerance of rice without changing other agronomic traits (Zhang et al., 2019a; Han et al., 2022). OsRAV2 was successfully mutated using CRISPR/Cas9, and the mutant had higher survival viability under salt stress (Liu et al., 2020b). In addition, using CRISPR/Cas9 technology to knock out rice OsDST (Santosh Kumar et al., 2020), OsNAC041 (Wang et al., 2016b) and OsmiR535 (Yue et al., 2020)], barley HvITPK1 (Vlčko and Ohnoutková, 2020) and tomato SlARF4 (Bouzroud et al., 2020) can also effectively improve the ability of crops to resist salt stress.
Drought stress is the main cause of serious loss of yield and productivity of major crops and poses the greatest threat to global food security (Joshi et al., 2020). Using CRISPR/Cas system, the natural ARGOS8 promoter sequence of maize was replaced by GOS2 promoter to improve the yield of maize under drought stress in field (Shi et al., 2017). CRISPR/Cas9-mediated mutagenesis of OsERA1 resulted in great drought stress tolerance in rice (Ogata et al., 2020). Drought resistance of wheat was improved by CRISPR/Cas editing of wheat TaDREB2 and TaERF3 (Kim et al., 2018). Santosh Kumar et al. (2020) used CRISPR/Cas9 to edit OsDST gene to obtain the mutant of indica mega rice cultivar MTU1010, with wider leaves, lower stomatal density and enhanced leaf water retention ability under drought stress (Santosh Kumar et al., 2020). Usman et al. (2020) found that the ospyl9 mutant created by CRISPR/Cas9 could improve drought tolerance and yield of rice (Usman et al., 2020). CRISPR/Cas9 induced SRL1 and SRL2 gene mutations in rice to achieve the curled leaves phenotype and drought tolerance by changing expression patterns of protein and scavenging of reactive oxygen species (Liao et al., 2019). Tomato plants with high leaf water content were obtained under drought conditions using CRISPR/Cas9 to modify GID1, and tomato drought resistance was effectively increased (Illouz-Eliaz et al., 2020). In addition, CRISPR/Cas9-mediated SlLBD40 gene mutation also significantly enhanced drought resistance of tomato (Liu et al., 2020a).
Cold stress, which includes chilling (<20°C) and freezing (<0°C) temperatures, inhibited growth and development of plants, and seriously restricts plant spatial distribution and agricultural productivity (Ding et al., 2020). Low temperature directly inhibits plant metabolic response and induces osmotic stress, oxidative stress and other stress. Zeng et al. showed that the ospin5b mutant, gs3 mutant and osmyb30 mutant created by CRISPR/Cas9 increased spike length, grain size and cold tolerance (Zeng et al., 2019). High temperature affects the whole growth cycle of crops, especially in the heat sensitive period such as early establishment, flowering and gametophytogenesis (Jagadish et al., 2021). Compared with the wild type, the CRISPR/Cas9-mediated slmapk3 mutant maintained reactive oxygen species homeostasis by regulating the expression of antioxidant enzymes and HSPs/HSFs genes, enhancing the high temperature tolerance of tomato plants (Yu et al., 2019). CRISPR/Cas9 editing was used to make pyl1/4/6 triple knockout rice. The mutant showed a greater yield, higher temperature tolerance, and less germination before harvest than the wild variety (Miao et al., 2018). Heavy metal toxicity is one of the most destructive abiotic stress.
Heavy metals cause serious damage to plant growth and yield and are the main problem of sustainable agricultural development. It has adverse effects on plant physiology and biochemistry through osmotic stress, ion imbalance, oxidative stress, membrane tissue disorder, cytotoxicity and metabolic homeostasis (Hoque et al., 2021). Heavy metals accumulated in plants will cause serious harm to human health after ingestion (Kaur et al., 2021). Knockdown of OsNramp5 and OsLCT1 by CRISPR/Cas9 reduces cadmium (Cd) accumulation in rice (Songmei et al., 2019). OsNRAMP1 was knocked out using CRISPR/Cas9, which resulted in lower Cd and plumbum (Pb) levels in rice grains (Chang et al., 2020; Chu et al., 2022). At the same time, the function of OsNRAMP5 and OsNRAMP1 to reduce Cd accumulation is not redundant. Wang et al. (2017) inhibited the absorption and transport of arsenic in rice by eliminating an R2R3 MYB transcription factor OsARM1 by CRISPR/Cas9 (Wang et al., 2017). To create low Caesium (Cs) rice plants, Nieves-Cordones et al. (2017) used the CRISPR/Cas to inactivate the K+ transporter OsHAK1 (Nieves-Cordones et al., 2017).
CRISPR/Cas gene editing improves biotic stress tolerance of crops
Biotic stresses, such as viral, fungal, and bacterial infections, account for 20–40% of global agricultural output losses (Walker, 1984). In order to address the food crisis, conferring pathogen resistance to host plants can lessen the impact of disease on crop productivity (Borrelli et al., 2018). So far, scientists have obtained plants that are highly resistant to fungal, bacterial and viral diseases, as well as insects, through CRISPR/Cas9 knockout (Chen et al., 2019) (Table 2).
Fungus disease is a kind of devastating disease in crops, among which powdery mildew seriously affects crop productivity. CRISPR/Cas9 was used to knock out all three TaMLO alleles in wheat, and wheat plants with enhanced powdery mildew resistance were obtained (Wang et al., 2014). Similarly, CRISPR/Cas9-mediated knockdown of SlMLO and VvMOL3 made tomato (Nekrasov et al., 2017) and grape (Wan et al., 2020) resistant to powdery mildew. In addition, CRISPR/Cas9-mediated SlPMR4 mutation also significantly increased tomato powdery mildew resistance, but could not completely immune (Santillán Martínez et al., 2020). Rice blast is a destructive fungal disease. CRISPR/Cas9 was used to enhance resistance to rice blast disease by interrupting the OsERF922 and OsSEC3A genes in rice (Wang et al., 2016a; Ma et al., 2018). Among them, other agronomic traits of oserf922 mutant did not change (Wang et al., 2016a), while SA content in ossec3a increased, resulting in dwarfing (Ma et al., 2018). CRISPR/Cas9-induced rice Bsr-d1 and Pi21 mutations could also cause partial resistance to rice blast, but the effect was not as strong as oserf922 (Nawaz et al., 2020; Zhou et al., 2022). Tomato late blight is a serious tomato fungal disease caused by Phytophthora infestans, which mainly affects tomato yield. miRNAs can enhance plant resistance by inhibiting their target genes. miR482b and miR482c were simultaneously knocked out by multiple editing systems, and double mutants were found to have higher resistance than single mutants, revealing a new mechanism by which miRNAs regulate fungal resistance (Hong et al., 2021). Furthermore, Silva et al. (2021) discovered that when the PL was knocked out by CRISPR/Cas9, the incidence of gray mold infection in tomato fruits was significantly reduced (Silva et al., 2021).
Of all the bacterial species on earth, hundreds can cause disease in plants, often exposing multiple disease symptoms (Schloss and Handelsman, 2004). Plant pathogenic bacteria are difficult to control due to the difficulty of detecting disease before it appears and the lack of effective pesticides. CRISPR/Cas9 modification of plant genomes has been found to improve crop resistance to bacterial diseases. For example, OsSWEET13 is a susceptibility (S) gene that codes for a sucrose transporter that plays an important role in the interaction between plant and pathogen. PthXo2, an effector protein produced by X. oryzae, causes OsSWEET13 expression in the host and, as a result, susceptibility. In rice plants, knocking down the promoter OsSWEET13 resulted in bacterial blight resistance (Zhou et al., 2015). Citrus bacterial canker (CBC) is the most widespread bacterial disease in citrus, which was caused by Xanthomonas citri subspecies citri. Jia et al. (2016) generated CBC-resistant mutants by editing the promoter sequence of the CsLOB1 gene in Duncan grapefruit (Jia et al., 2016). Meanwhile, Peng et al. (2017) also reported that CRISPR/Cas9 targeted modification of citrus susceptible gene CsLOB1 promoter EBEPthA4 combined with the original to improve the resistance of Wanjincheng orange (Citrus sinensis Osbeck) to citrus canker disease (Peng et al., 2017). Pseudomonas Syringae is the cause of bacterial leaf spot disease. It induces stomatal opening of plants by releasing coronatine, which is conducive to bacterial infection. The Jasmonate-ZIM domain protein is a COR coreceptor, and SlJAZ2 is edited by CRISPR/Cas9 to lack JAZ domain, resulting in resistance to bacterial leaf spot disease (Ortigosa et al., 2019). In addition, the CRISPR/Cas9 technology provides a strategy for the creation of multiple resistant materials that induce mutations in the acetylegenase-encoding genes ACER1a and ACET1b that show increased resistance to fungal and bacterial pathogens (Jeon et al., 2020). CRISPR/Cas9-induced mutations in tomato susceptibility gene SlDMR6-1 confer resistance to different types of pathogens, including bacteria, oomycetes and fungi (Thomazella et al., 2021). CRISPR/Cas9-mediated osnramp1 mutants increased hydrogen peroxide (H2O2) content and superoxide dismutase (SOD) activity, but decreased catalase (CAT) activity, showing broad-spectrum resistance to bacteria and fungi (Chu et al., 2022).
A number of economically important staples and specialty crops are threatened by plant viruses. According to the nature of their genomes, they are divided into six major groups: single-stranded DNA (ssDNA), double-stranded DNA (dsDNA)viruses with no plant viruses in this group, double-stranded RNA (dsRNA), reverse-transcribing viruses, positive sense single-stranded RNA (ssRNA+) viruses and negative sense single-stranded RNA (ssRNA) (Roossinck et al., 2015). Studies using CRISPR-edited plants for virus resistance have focused on ssDNA geminivirus genomes (Ali et al., 2015; Baltes et al., 2015; Ji et al., 2015). It includes many kinds of plant viruses that causes worldwide crop losses, affecting many important families, including Euphorbiaceae, Cucurbitaceae, Malvaceae, Solanaceae, and Fabaceae (Zaidi et al., 2016). By rolling-circle amplification or recombination-mediated replication, the virus genome replicates itself via a dsDNA replicative form (Hanley-Bowdoin et al., 2013). In economic terms, Begomovirus is the most important genus of geminiviruses. Begomovirus infect dicotyledonous plants primarily through the sweet tobacco/potato/silverleaf whitefly (Bemisia tabaci) and are found attached to phloem of plants (Gilbertson et al., 2015). The genome is composed of one (A, monopartite) or two (A and B, bipartite) components, which contain a common 220-bp region (Fondong, 2013). At first, Baltes et al. (2015) and Ji et al. (2015) reported on resistance to geminiviruses, beet severe curly top virus (BSCTV) and bean yellow dwarf virus (BeYDV) in model plants Nicotiana benthamiana and Arabidopsis Baltes et al., 2015; Ji et al., 2015). Ji et al. (2015) identified 43 possible sgRNA/Cas9 targets within the coding and non-coding domains of the BSCTV genome (Ji et al., 2015). Each sgRNA/Cas9 construct reduced virus content to varying degrees in inoculated leaves. And, the plants with the highest expressing levels of Cas9 and sgRNAs seem to be more resistance to virus infection. Similarly, Baltes et al. (2015) utilized 11 sgRNAs targeting Rep motifs, Rep-binding sites, hairpins, and the nonanucleotide sequence of BeYDV to achieve similar results (Baltes et al., 2015). A CRISPR/Cas9 approach was also used for enhancing resistance to begomovirus in two recent studies (Ali et al., 2015; Ali et al., 2016). The CRISPR/Cas9 systems were expressed in the host cell nucleus, and the viral genome was targeted and cleaved during replication in both studies. The sgRNA molecules developed by Ali et al. (2015) were delivered into N. benthamiana plants overexpressing the Cas9 endonuclease via a tobacco rattle virus (TRV) vector (Ali et al., 2015). SgRNAs target different coding and non-coding sequences of the tomato yellow leaf curl virus (TYLCV), including the RCRII motif of the replication protein (Rep), the capsid protein (CP) and the intergenic region (IR). The sgRNAs that target stem-loop invariant sequences in the IR caused a significant reduction in viral replication and accumulation but did not interfere with TYLCV genome sequences. Bipartite Merremia mosaic virus (MeMV) and the monopartite beet curly top virus (BCTV) have same stem-loop sequence in the IR. Therefore, CRISPR/Cas9 system was designed to target this sequence simultaneously. The results demonstrated that a sgRNA specific for conserved sequences from multiple viral strains can be used to realize mixed infection immunity. Furthermore, Different CRISPR/Cas9 tools were designed to target different coding and non-coding sequences of MeMV, cotton leaf curl Kokhran virus (CLCuKoV), and different severe and mild strains of TYLCV (Ali et al., 2016). Researchers found that when the viral coding regions were edited by sgRNA/Cas9 complex, virus variants that were able to replicate and escape CRISPR/Cas9 were generated. In contrast, no new variants were found in plants carrying sgRNAs targeting the IR sequences in N. benthamiana plants. A second technique for achieving viral disease resistance entails altering plant genes that provide virus resistance qualities, segregating the CRISPR/Cas9 tool, and releasing non-transgenic mutants into the field (Chandrasekaran et al., 2016; Pyott et al., 2016; Macovei et al., 2018). Plant host factors, such as the eukaryotic translation initiation factors eIF4E, eIF(iso)4E, and eIF4G, are required by RNA viruses to maintain their life cycle (Sanfaçon, 2015). By modifying two different sites of the host susceptibility gene eIF4E with CRISPR/Cas9, Chandrasekaran et al. (2016) were able to develop cucumber plants that were resistant to potyviruses. Homozygous eif4e mutants demonstrated protection to viruses from the Potyviridae family, such as zucchini yellow mosaic virus (ZYMV), cucumber vein yellowing virus (CVYV), and papaya ring spot mosaic virus-W (PRSV-W). However, heterozygous knockout plants and nonmutant plants showed no resistance to these viruses. Macovei et al. (2018) used mutagenesis of eIF4G alleles in rice plants to establish novel sources of resistance to rice tungro spherical virus (RTSV) (Macovei et al., 2018). Furthermore, after inoculation with RTSV, agronomic parameters, e.g., plant height and grain production of the edited rice plants were improved compared to that the wild-type. In tomato plant, CRISPR/Cas9 was used to edit SlDCL2b with the highest expression in four DCL2 subfamilies (SLDCL2a-SLDCL2d), which significantly enhanced tomato resistance to tomato mosaic virus (ToMV) (Wang et al., 2018a). Wang et al. (2018a) discovered that editing SlDCL2a and SlDCL2b at the same time increased tomato resistance to potato virus X (PVX) and tobacco mosaic virus (TMV) (Wang et al., 2018b).
Pests is thought to be responsible for 20–40% of global crop output loss (Douglas, 2018). Pests adversely affect crop yield and quality through direct damage and transmission of plant diseases (Oerke, 2006). In recent years, agricultural losses caused by pests have increased as the climate has warmed (Deutsch et al., 2018), but widespread use of pesticides can have a negative impact on the environment. Therefore, there is an urgent need for a safe and effective method to control the occurrence of insect pests on crops. The level of salicylic acid levels was raised when the serotonin biosynthesis was prevented by disrupting OsCYP71A1, which results in greater resistance to plant hoppers and stem borers in rice (Lu et al., 2018). Li et al. (2022) showed that the gmcdpk38 mutant with Hap3 knockout using CRISPR/Cas9 showed high resistance to common cutworm (Li et al., 2022).
Conclusion and future prospects
Traditional plant breeding, such as conventional intergeneric crosses and chemical/physical mutagenesis are non-specific. Moreover, introgression of beneficial traits into an elite variety is often accompanied by introgression of non-target traits because of linkage drag. Therefore, it is a long-term effort for the development of new cultivars using traditional breeding methods, especially it is time-consuming of backcross to segregate the unwanted changes in their offspring (Hartung and Schiemann, 2014). In contrast, CRISPR/Cas9 can accelerate plant breeding by modify genomes rapidly in a precise and predictable manner. Because of its efficiency, simplicity, and versatility, CRISPR/Cas9 has recently become a popular tool for genome editing and has been widely used in crop resistance breeding (Wolter et al., 2019). CRISPR-Cas9 system can be used for gene knockout, gene insertion and gene replacement, resulting in loss-of-function, knock down or activation mutants, which can lead to generation of abiotic/biotic stress-tolerant crop plants (Figure 1). Meanwhile, the availability of the genome sequence of crops allows scientists to precisely design its genome, which facilitates the application of CRISPR/Cas9 in resistance breeding (Jackson et al., 2011). Firstly, the major genes controlling important traits of crops have not been identified, which limits the application of CRISPR/Cas in plant genetic engineering breeding (Jin et al., 2019). Secondly, pathogens keep to modify its genome though evolution to break the already available resistance obtained by CRISPR/Cas gene editing. Therefore, it is required to design new variants every few years and insert them into the plants(Ahmad et al., 2020). Thirdly, many genes are represented by multi-gene families with functional redundancy, making it difficult to produce resistance phenotype by knocking-out a single gene (Li et al., 2013). It is required to develop powerful CRISPR-Cas tools to realize multiplex genome editing.
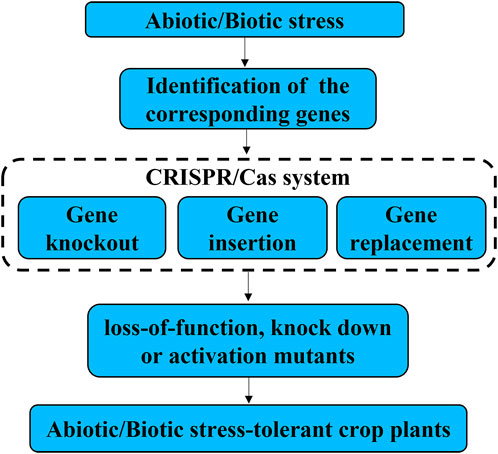
FIGURE 1. Review of the applications of CRISPR/Cas systems in improving biotic and abiotic stress tolerance of crop. CRISPR-Cas9 system can be used for gene knockout, gene insertion and gene replacement, resulting in loss-of-function, knock down or activation mutants, which can lead to generation of abiotic/biotic stress-tolerant crop plants.
Off-target effect is another major limitation of CRISPR-Cas system. Although much work has been done to optimize CRISPR/Cas system and improve its specificity, there is still no way to completely avoid editing individuals off-target (Pattanayak et al., 2013; Fu et al., 2014; Kleinstiver et al., 2016; Tang et al., 2019). Therefore, the potential off-target risk cannot be ignored when using CRISPR/Cas for genome editing. For gene function studies, in order to exclude misjudgment of results caused by off-target phenomenon, association analysis between genotype and phenotype should be carried out in multiple independently edited individuals to determine whether phenotypic changes are caused by mutations in target genes. In addition, one big challenge in crop breeding is efficient delivery of CRISPR/Cas components into reproductive cells. In the case of plants that can be transformed, foreign genes can be introduced into their reproductive cells by genetic transformation methods in a quite effective way. However, the associated tissue culture and regeneration steps are time consuming and complex. Furthermore, many crops are recalcitrant or extremely difficult to transform. For gene editing to be applied to all plants, we need technology that can deliver gene editing reagents independent of tissue culture and plant regeneration.
Despite the overwhelming benefits of CRISPR/Cas system for crop improvement, regulatory policies that classify gene-edited goods as GMOs may prevent their use in some nations (Callaway, 2018). However, from a scientific point of view, the mutants obtained by CRISPR/Cas are exactly the same as those obtained by natural mutation or conventional mutagenesis after removing the transgenic label. We think more publicity should be given in this regard to dispel the prejudice of most people. On January 24, 2022, the Ministry of Agriculture and Rural Affairs of The People’s Republic of China issued the 《Guidelines for Safety Evaluation of Gene-edited Plants for Agricultural Use (Trial)》 (http://www.moa.gov.cn/ztzl/zjyqwgz/sbzn/202201/t20220124_6387561.htm). This guideline mainly applies for safety evaluation of gene-edited plants without introducing exogenous genes according to different risk levels to apply for production and application safety certificates. The release of this guideline provides a basis for the standardized development of gene-edited crops and a reference for further deregulation of gene-edited crops in the future.
CRISPR and other gene editing technologies have already made significant gains in crop breeding, and we expect that this is just the beginning, with many more exciting developments to follow. With the development of second-generation sequencing, gene editing technology and target analysis technology based on high-throughput sequencing method have a solid technical foundation, and the acquisition of high-throughput big data has become more common, convenient and affordable, which will greatly promote the application of CRISPR/Cas in crop genetic improvement.
Author contributions
QZ and YZ designed the article. YL and XW drafted the manuscript and arranged the references. QZ and YZ incorporated all necessary modifications. All authors have read and agreed to the published version of the manuscript.
Funding
Hunan Tobacco Company Science and Technology Project (HN2022KJ01).
Conflict of interest
The authors declare that the research was conducted in the absence of any commercial or financial relationships that could be construed as a potential conflict of interest.
Publisher’s note
All claims expressed in this article are solely those of the authors and do not necessarily represent those of their affiliated organizations, or those of the publisher, the editors and the reviewers. Any product that may be evaluated in this article, or claim that may be made by its manufacturer, is not guaranteed or endorsed by the publisher.
References
Ahmad, S., Wei, X., Sheng, Z., Hu, P., and Tang, S. (2020). CRISPR/Cas9 for development of disease resistance in plants: Recent progress, limitations and future prospects. Brief. Funct. Genomics 19 (1), 26–39. doi:10.1093/bfgp/elz041
Al Murad, M., Khan, A. L., and Muneer, S. (2020). Silicon in horticultural crops: Cross-talk, signaling, and tolerance mechanism under salinity stress. Plants (Basel) 9 (4), E460. doi:10.3390/plants9040460
Alam, M. S., Kong, J., Tao, R., Ahmed, T., Alamin, M., Alotaibi, S. S., et al. (2022). CRISPR/Cas9 mediated knockout of the OsbHLH024 transcription factor improves salt stress resistance in rice (Oryza sativa L.). Plants (Basel) 11 (9), 1184. doi:10.3390/plants11091184
Alfatih, A., Wu, J., Jan, S. U., Zhang, Z. S., Xia, J. Q., and Xiang, C. B. (2020). Loss of rice PARAQUAT TOLERANCE 3 confers enhanced resistance to abiotic stresses and increases grain yield in field. Plant Cell Environ. 43 (11), 2743–2754. doi:10.1111/pce.13856
Ali, Z., Abulfaraj, A., Idris, A., Ali, S., Tashkandi, M., and Mahfouz, M. M. (2015). CRISPR/Cas9-mediated viral interference in plants. Genome Biol. 16, 238. doi:10.1186/s13059-015-0799-6
Ali, Z., Ali, S., Tashkandi, M., Zaidi, S. S., and Mahfouz, M. M. (2016). CRISPR/Cas9-mediated immunity to geminiviruses: Differential interference and evasion. Sci. Rep. 6, 26912. doi:10.1038/srep26912
Baltes, N. J., Hummel, A. W., Konecna, E., Cegan, R., Bruns, A. N., Bisaro, D. M., et al. (2015). Conferring resistance to geminiviruses with the CRISPR-Cas prokaryotic immune system. Nat. Plants 1 (10), 15145. doi:10.1038/nplants.2015.145
Boch, J., Scholze, H., Schornack, S., Landgraf, A., Hahn, S., Kay, S., et al. (2009). Breaking the code of DNA binding specificity of TAL-type III effectors. Science 326 (5959), 1509–1512. doi:10.1126/science.1178811
Borrelli, V. M. G., Brambilla, V., Rogowsky, P., Marocco, A., and Lanubile, A. (2018). The enhancement of plant disease resistance using CRISPR/Cas9 technology. Front. Plant Sci. 9, 1245. doi:10.3389/fpls.2018.01245
Bouzroud, S., Gasparini, K., Hu, G., Barbosa, M. A. M., Rosa, B. L., Fahr, M., et al. (2020). Down regulation and loss of auxin response factor 4 function using CRISPR/Cas9 alters plant growth, stomatal function and improves tomato tolerance to salinity and osmotic stress. Genes (Basel) 11 (3), E272. doi:10.3390/genes11030272
Cai, Z., Xian, P., Cheng, Y., Ma, Q., Lian, T., Nian, H., et al. (2021). CRISPR/Cas9-mediated gene editing of GmJAGGED1 increased yield in the low-latitude soybean variety Huachun 6. Plant Biotechnol. J. 19 (10), 1898–1900. doi:10.1111/pbi.13673
Callaway, E. (2018). CRISPR plants now subject to tough GM laws in European Union. Nature 560 (7716), 16. doi:10.1038/d41586-018-05814-6
Chandrasekaran, J., Brumin, M., Wolf, D., Leibman, D., Klap, C., Pearlsman, M., et al. (2016). Development of broad virus resistance in non-transgenic cucumber using CRISPR/Cas9 technology. Mol. Plant Pathol. 17 (7), 1140–1153. doi:10.1111/mpp.12375
Chang, J. D., Huang, S., Yamaji, N., Zhang, W., Ma, J. F., and Zhao, F. J. (2020). OsNRAMP1 transporter contributes to cadmium and manganese uptake in rice. Plant Cell Environ. 43 (10), 2476–2491. doi:10.1111/pce.13843
Chauhan, R., Awasthi, S., Indoliya, Y., Chauhan, A. S., Mishra, S., Agrawal, L., et al. (2020). Transcriptome and proteome analyses reveal selenium mediated amelioration of arsenic toxicity in rice (Oryza sativa L.). J. Hazard. Mat. 390, 122122. doi:10.1016/j.jhazmat.2020.122122
Chen, J. S., Dagdas, Y. S., Kleinstiver, B. P., Welch, M. M., Sousa, A. A., Harrington, L. B., et al. (2017). Enhanced proofreading governs CRISPR-Cas9 targeting accuracy. Nature 550 (7676), 407–410. doi:10.1038/nature24268
Chen, K., Wang, Y., Zhang, R., Zhang, H., and Gao, C. (2019). CRISPR/Cas genome editing and precision plant breeding in agriculture. Annu. Rev. Plant Biol. 70, 667–697. doi:10.1146/annurev-arplant-050718-100049
Christian, M., Cermak, T., Doyle, E. L., Schmidt, C., Zhang, F., Hummel, A., et al. (2010). Targeting DNA double-strand breaks with TAL effector nucleases. Genetics 186 (2), 757–761. doi:10.1534/genetics.110.120717
Chu, C., Huang, R., Liu, L., Tang, G., Xiao, J., Yoo, H., et al. (2022). The rice heavy-metal transporter OsNRAMP1 regulates disease resistance by modulating ROS homoeostasis. Plant Cell Environ. 45 (4), 1109–1126. doi:10.1111/pce.14263
Cong, L., and Zhang, F. (2015). Genome engineering using CRISPR-Cas9 system. Methods Mol. Biol. 1239, 197–217. doi:10.1007/978-1-4939-1862-1_10
Cong, L., Ran, F. A., Cox, D., Lin, S., Barretto, R., Habib, N., et al. (2013). Multiplex genome engineering using CRISPR/Cas systems. Science 339 (6121), 819–823. doi:10.1126/science.1231143
Deutsch, C. A., Tewksbury, J. J., Tigchelaar, M., Battisti, D. S., Merrill, S. C., Huey, R. B., et al. (2018). Increase in crop losses to insect pests in a warming climate. Science 361 (6405), 916–919. doi:10.1126/science.aat3466
Ding, Y., Shi, Y., and Yang, S. (2020). Molecular regulation of plant responses to environmental temperatures. Mol. Plant 13 (4), 544–564. doi:10.1016/j.molp.2020.02.004
Douglas, A. E. (2018). Strategies for enhanced crop resistance to insect pests. Annu. Rev. Plant Biol. 69, 637–660. doi:10.1146/annurev-arplant-042817-040248
Fondong, V. N. (2013). Geminivirus protein structure and function. Mol. Plant Pathol. 14 (6), 635–649. doi:10.1111/mpp.12032
Fu, Y., Sander, J. D., Reyon, D., Cascio, V. M., and Joung, J. K. (2014). Improving CRISPR-Cas nuclease specificity using truncated guide RNAs. Nat. Biotechnol. 32 (3), 279–284. doi:10.1038/nbt.2808
Gao, C. (2018). The future of CRISPR technologies in agriculture. Nat. Rev. Mol. Cell Biol. 19 (5), 275–276. doi:10.1038/nrm.2018.2
Gilbertson, R. L., Batuman, O., Webster, C. G., and Adkins, S. (2015). Role of the insect supervectors bemisia tabaci and frankliniella occidentalis in the emergence and global spread of plant viruses. Annu. Rev. Virol. 2 (1), 67–93. doi:10.1146/annurev-virology-031413-085410
Gupta, A., Rico-Medina, A., and Caño-Delgado, A. I. (2020). The physiology of plant responses to drought. Science 368 (6488), 266–269. doi:10.1126/science.aaz7614
Han, X., Chen, Z., Li, P., Xu, H., Liu, K., Zha, W., et al. (2022). Development of novel rice germplasm for salt-tolerance at seedling stage using CRISPR-Cas9. Sustainability 14, 2621. doi:10.3390/su14052621
Hanley-Bowdoin, L., Bejarano, E. R., Robertson, D., and Mansoor, S. (2013). Geminiviruses: Masters at redirecting and reprogramming plant processes. Nat. Rev. Microbiol. 11 (11), 777–788. doi:10.1038/nrmicro3117
Hartung, F., and Schiemann, J. (2014). Precise plant breeding using new genome editing techniques: Opportunities, safety and regulation in the EU. Plant J. 78 (5), 742–752. doi:10.1111/tpj.12413
Hille, F., Richter, H., Wong, S. P., Bratovič, M., Ressel, S., and Charpentier, E. (2018). The biology of CRISPR-cas: Backward and forward. Cell 172 (6), 1239–1259. doi:10.1016/j.cell.2017.11.032
Hong, Y., Meng, J., He, X., Zhang, Y., Liu, Y., Zhang, C., et al. (2021). Editing miR482b and miR482c simultaneously by CRISPR/Cas9 enhanced tomato resistance to phytophthora infestans. Phytopathology 111 (6), 1008–1016. doi:10.1094/phyto-08-20-0360-r
Hoque, M. N., Tahjib-Ul-Arif, M., Hannan, A., Sultana, N., Akhter, S., Hasanuzzaman, M., et al. (2021). Melatonin modulates plant tolerance to heavy metal stress: Morphological responses to molecular mechanisms. Int. J. Mol. Sci. 22 (21), 11445. doi:10.3390/ijms222111445
Hu, J. H., Miller, S. M., Geurts, M. H., Tang, W., Chen, L., Sun, N., et al. (2018). Evolved Cas9 variants with broad PAM compatibility and high DNA specificity. Nature 556 (7699), 57–63. doi:10.1038/nature26155
Hua, K., Tao, X., and Zhu, J. K. (2019). Expanding the base editing scope in rice by using Cas9 variants. Plant Biotechnol. J. 17 (2), 499–504. doi:10.1111/pbi.12993
Illouz-Eliaz, N., Nissan, I., Nir, I., Ramon, U., Shohat, H., and Weiss, D. (2020). Mutations in the tomato gibberellin receptors suppress xylem proliferation and reduce water loss under water-deficit conditions. J. Exp. Bot. 71 (12), 3603–3612. doi:10.1093/jxb/eraa137
Ismail, A. M., and Horie, T. (2017). Genomics, Physiology, and molecular breeding approaches for improving salt tolerance. Annu. Rev. Plant Biol. 68, 405–434. doi:10.1146/annurev-arplant-042916-040936
Jackson, S. A., Iwata, A., Lee, S.-H., Schmutz, J., and Shoemaker, R. (2011). Sequencing crop genomes: Approaches and applications. New Phytol. 191 (4), 915–925. doi:10.1111/j.1469-8137.2011.03804.x
Jagadish, S. V. K., Way, D. A., and Sharkey, T. D. (2021). Plant heat stress: Concepts directing future research. Plant Cell Environ. 44 (7), 1992–2005. doi:10.1111/pce.14050
Jeon, J. E., Kim, J. G., Fischer, C. R., Mehta, N., Dufour-Schroif, C., Wemmer, K., et al. (2020). A Pathogen-responsive gene cluster for highly modified fatty acids in tomato. Cell 180 (1), 176–187. e119. doi:10.1016/j.cell.2019.11.037
Ji, X., Zhang, H., Zhang, Y., Wang, Y., and Gao, C. (2015). Establishing a CRISPR-Cas-like immune system conferring DNA virus resistance in plants. Nat. Plants 1, 15144. doi:10.1038/nplants.2015.144
Ji, X., Si, X., Zhang, Y., Zhang, H., Zhang, F., and Gao, C. (2018). Conferring DNA virus resistance with high specificity in plants using virus-inducible genome-editing system. Genome Biol. 19 (1), 197. doi:10.1186/s13059-018-1580-4
Jia, H., Orbovic, V., Jones, J. B., and Wang, N. (2016). Modification of the PthA4 effector binding elements in Type I CsLOB1 promoter using Cas9/sgRNA to produce transgenic Duncan grapefruit alleviating XccΔpthA4:dCsLOB1.3 infection. Plant Biotechnol. J. 14 (5), 1291–1301. doi:10.1111/pbi.12495
Jin, S., Zong, Y., Gao, Q., Zhu, Z., Wang, Y., Qin, P., et al. (2019). Cytosine, but not adenine, base editors induce genome-wide off-target mutations in rice. Science 364 (6437), 292–295. doi:10.1126/science.aaw7166
Jinek, M., Chylinski, K., Fonfara, I., Hauer, M., Doudna, J. A., and Charpentier, E. (2012). A programmable dual-RNA-guided DNA endonuclease in adaptive bacterial immunity. Science 337 (6096), 816–821. doi:10.1126/science.1225829
Joshi, R. K., Bharat, S. S., and Mishra, R. (2020). Engineering drought tolerance in plants through CRISPR/Cas genome editing. 3 Biotech. 10 (9), 400. doi:10.1007/s13205-020-02390-3
Kaur, R., Das, S., Bansal, S., Singh, G., Sardar, S., Dhar, H., et al. (2021). Heavy metal stress in rice: Uptake, transport, signaling, and tolerance mechanisms. Physiol. Plant. 173 (1), 430–448. doi:10.1111/ppl.13491
Kim, Y. G., Cha, J., and Chandrasegaran, S. (1996). Hybrid restriction enzymes: Zinc finger fusions to fok I cleavage domain. Proc. Natl. Acad. Sci. U. S. A. 93 (3), 1156–1160. doi:10.1073/pnas.93.3.1156
Kim, D., Alptekin, B., and Budak, H. (2018). CRISPR/Cas9 genome editing in wheat. Funct. Integr. Genomics 18 (1), 31–41. doi:10.1007/s10142-017-0572-x
Kleinstiver, B. P., Pattanayak, V., Prew, M. S., Tsai, S. Q., Nguyen, N. T., Zheng, Z., et al. (2016). High-fidelity CRISPR-Cas9 nucleases with no detectable genome-wide off-target effects. Nature 529 (7587), 490–495. doi:10.1038/nature16526
Li, J.-F., Norville, J. E., Aach, J., McCormack, M., Zhang, D., Bush, J., et al. (2013). Multiplex and homologous recombination–mediated genome editing in Arabidopsis and Nicotiana benthamiana using guide RNA and Cas9. Nat. Biotechnol. 31 (8), 688–691. doi:10.1038/nbt.2654
Li, T., Yang, X., Yu, Y., Si, X., Zhai, X., Zhang, H., et al. (2018). Domestication of wild tomato is accelerated by genome editing. Nat. Biotechnol. 36, 1160–1163. doi:10.1038/nbt.4273
Li, S., Shen, L., Hu, P., Liu, Q., Zhu, X., Qian, Q., et al. (2019). Developing disease-resistant thermosensitive male sterile rice by multiplex gene editing. J. Integr. Plant Biol. 61 (12), 1201–1205. doi:10.1111/jipb.12774
Li, X., Hu, D., Cai, L., Wang, H., Liu, X., Du, H., et al. (2022). CALCIUM-DEPENDENT PROTEIN KINASE38 regulates flowering time and common cutworm resistance in soybean. Plant Physiol., kiac260. doi:10.1093/plphys/kiac260
Liao, S., Qin, X., Luo, L., Han, Y., Wang, X., Usman, B., et al. (2019). CRISPR/Cas9-Induced mutagenesis of semi-rolled leaf1, 2 confers curled leaf phenotype and drought tolerance by influencing protein expression patterns and ROS scavenging in rice (Oryza sativa L.). Agronomy 9 (11), 728. doi:10.3390/agronomy9110728
Liu, T., Chen, W., Wu, W., Sun, C., Guo, W., and Zhu, X. (2016). Detection of aphids in wheat fields using a computer vision technique. Biosyst. Eng. 141, 82–93. doi:10.1016/j.biosystemseng.2015.11.005
Liu, L., Zhang, J., Xu, J., Li, Y., Guo, L., Wang, Z., et al. (2020a). CRISPR/Cas9 targeted mutagenesis of SlLBD40, a lateral organ boundaries domain transcription factor, enhances drought tolerance in tomato. Plant Sci. 301, 110683. doi:10.1016/j.plantsci.2020.110683
Liu, X., Wu, D., Shan, T., Xu, S., Qin, R., Li, H., et al. (2020b). The trihelix transcription factor OsGTγ-2 is involved adaption to salt stress in rice. Plant Mol. Biol. 103 (4-5), 545–560. doi:10.1007/s11103-020-01010-1
Liu, L., Kuang, Y., Yan, F., Li, S., Ren, B., Gosavi, G., et al. (2021). Developing a novel artificial rice germplasm for dinitroaniline herbicide resistance by base editing of OsTubA2. Plant Biotechnol. J. 19 (1), 5–7. doi:10.1111/pbi.13430
Liu, Z., Ma, C., Hou, L., Wu, X., Wang, D., Zhang, L., et al. (2022). Exogenous SA affects rice seed germination under salt stress by regulating Na(+)/K(+) balance and endogenous GAs and ABA homeostasis. Int. J. Mol. Sci. 23 (6), 3293. doi:10.3390/ijms23063293
Lu, H. P., Luo, T., Fu, H. W., Wang, L., Tan, Y. Y., Huang, J. Z., et al. (2018). Resistance of rice to insect pests mediated by suppression of serotonin biosynthesis. Nat. Plants 4 (6), 338–344. doi:10.1038/s41477-018-0152-7
Lu, Y., Tian, Y., Shen, R., Yao, Q., Wang, M., Chen, M., et al. (2020). Targeted, efficient sequence insertion and replacement in rice. Nat. Biotechnol. 38 (12), 1402–1407. doi:10.1038/s41587-020-0581-5
Ma, J., Chen, J., Wang, M., Ren, Y., Wang, S., Lei, C., et al. (2018). Disruption of OsSEC3A increases the content of salicylic acid and induces plant defense responses in rice. J. Exp. Bot. 69 (5), 1051–1064. doi:10.1093/jxb/erx458
Macovei, A., Sevilla, N. R., Cantos, C., Jonson, G. B., Slamet-Loedin, I., Čermák, T., et al. (2018). Novel alleles of rice eIF4G generated by CRISPR/Cas9-targeted mutagenesis confer resistance to Rice tungro spherical virus. Plant Biotechnol. J. 16 (11), 1918–1927. doi:10.1111/pbi.12927
Mali, P., Yang, L., Esvelt, K. M., Aach, J., Guell, M., DiCarlo, J. E., et al. (2013). RNA-guided human genome engineering via Cas9. Science 339 (6121), 823–826. doi:10.1126/science.1232033
Miao, C., Xiao, L., Hua, K., Zou, C., Zhao, Y., Bressan, R. A., et al. (2018). Mutations in a subfamily of abscisic acid receptor genes promote rice growth and productivity. Proc. Natl. Acad. Sci. U. S. A. 115 (23), 6058–6063. doi:10.1073/pnas.1804774115
Ming, M., Ren, Q., Pan, C., He, Y., Zhang, Y., Liu, S., et al. (2020). CRISPR-Cas12b enables efficient plant genome engineering. Nat. Plants 6 (3), 202–208. doi:10.1038/s41477-020-0614-6
Nawaz, G., Usman, B., Peng, H., Zhao, N., Yuan, R., Liu, Y., et al. (2020). Knockout of Pi21 by CRISPR/Cas9 and iTRAQ-Based proteomic analysis of mutants revealed new insights into M. oryzae resistance in elite rice line. Genes (Basel) 11 (7), E735. doi:10.3390/genes11070735
Nekrasov, V., Wang, C., Win, J., Lanz, C., Weigel, D., and Kamoun, S. (2017). Rapid generation of a transgene-free powdery mildew resistant tomato by genome deletion. Sci. Rep. 7 (1), 482. doi:10.1038/s41598-017-00578-x
Nieves-Cordones, M., Mohamed, S., Tanoi, K., Kobayashi, N. I., Takagi, K., Vernet, A., et al. (2017). Production of low-Cs(+) rice plants by inactivation of the K(+) transporter OsHAK1 with the CRISPR-Cas system. Plant J. 92 (1), 43–56. doi:10.1111/tpj.13632
Nishimasu, H., Shi, X., Ishiguro, S., Gao, L., Hirano, S., Okazaki, S., et al. (2018). Engineered CRISPR-Cas9 nuclease with expanded targeting space. Science 361 (6408), 1259–1262. doi:10.1126/science.aas9129
Oerke, E. C. (2006). Crop losses to pests. J. Agric. Sci. 144 (1), 31–43. doi:10.1017/s0021859605005708
Ogata, T., Ishizaki, T., Fujita, M., and Fujita, Y. (2020). CRISPR/Cas9-targeted mutagenesis of OsERA1 confers enhanced responses to abscisic acid and drought stress and increased primary root growth under nonstressed conditions in rice. PLoS One 15 (12), e0243376. doi:10.1371/journal.pone.0243376
Oliva, R., Ji, C., Atienza-Grande, G., Huguet-Tapia, J. C., Perez-Quintero, A., Li, T., et al. (2019). Broad-spectrum resistance to bacterial blight in rice using genome editing. Nat. Biotechnol. 37 (11), 1344–1350. doi:10.1038/s41587-019-0267-z
Ortigosa, A., Gimenez-Ibanez, S., Leonhardt, N., and Solano, R. (2019). Design of a bacterial speck resistant tomato by CRISPR/Cas9-mediated editing of SlJAZ2. Plant Biotechnol. J. 17 (3), 665–673. doi:10.1111/pbi.13006
Pattanayak, V., Lin, S., Guilinger, J. P., Ma, E., Doudna, J. A., and Liu, D. R. (2013). High-throughput profiling of off-target DNA cleavage reveals RNA-programmed Cas9 nuclease specificity. Nat. Biotechnol. 31 (9), 839–843. doi:10.1038/nbt.2673
Peng, A., Chen, S., Lei, T., Xu, L., He, Y., Wu, L., et al. (2017). Engineering canker-resistant plants through CRISPR/Cas9-targeted editing of the susceptibility gene CsLOB1 promoter in citrus. Plant Biotechnol. J. 15 (12), 1509–1519. doi:10.1111/pbi.12733
Pyott, D. E., Sheehan, E., and Molnar, A. (2016). Engineering of CRISPR/Cas9-mediated potyvirus resistance in transgene-free Arabidopsis plants. Mol. Plant Pathol. 17 (8), 1276–1288. doi:10.1111/mpp.12417
Ran, F. A., Cong, L., Yan, W. X., Scott, D. A., Gootenberg, J. S., Kriz, A. J., et al. (2015). In vivo genome editing using Staphylococcus aureus Cas9. Nature 520 (7546), 186–191. doi:10.1038/nature14299
Roossinck, M. J., Martin, D. P., and Roumagnac, P. (2015). Plant virus metagenomics: Advances in virus discovery. Phytopathology 105 (6), 716–727. doi:10.1094/phyto-12-14-0356-rvw
Sanfaçon, H. (2015). Plant translation factors and virus resistance. Viruses 7 (7), 3392–3419. doi:10.3390/v7072778
Santillán Martínez, M. I., Bracuto, V., Koseoglou, E., Appiano, M., Jacobsen, E., Visser, R. G. F., et al. (2020). CRISPR/Cas9-targeted mutagenesis of the tomato susceptibility gene PMR4 for resistance against powdery mildew. BMC Plant Biol. 20 (1), 284. doi:10.1186/s12870-020-02497-y
Santosh Kumar, V. V., Verma, R. K., Yadav, S. K., Yadav, P., Watts, A., Rao, M. V., et al. (2020). CRISPR-Cas9 mediated genome editing of drought and salt tolerance (OsDST) gene in indica mega rice cultivar MTU1010. Physiol. Mol. Biol. Plants 26 (6), 1099–1110. doi:10.1007/s12298-020-00819-w
Schloss, P. D., and Handelsman, J. (2004). Status of the microbial census. Microbiol. Mol. Biol. Rev. 68 (4), 686–691. doi:10.1128/mmbr.68.4.686-691.2004
Shi, J., Gao, H., Wang, H., Lafitte, H. R., Archibald, R. L., Yang, M., et al. (2017). ARGOS8 variants generated by CRISPR-Cas9 improve maize grain yield under field drought stress conditions. Plant Biotechnol. J. 15 (2), 207–216. doi:10.1111/pbi.12603
Shi, Y., Ding, Y., and Yang, S. (2018). Molecular regulation of CBF signaling in cold acclimation. Trends Plant Sci. 23 (7), 623–637. doi:10.1016/j.tplants.2018.04.002
Siddiqui, M. N., Mostofa, M. G., Akter, M. M., Srivastava, A. K., Sayed, M. A., Hasan, M. S., et al. (2017). Impact of salt-induced toxicity on growth and yield-potential of local wheat cultivars: Oxidative stress and ion toxicity are among the major determinants of salt-tolerant capacity. Chemosphere 187, 385–394. doi:10.1016/j.chemosphere.2017.08.078
Silva, C. J., van den Abeele, C., Ortega-Salazar, I., Papin, V., Adaskaveg, J. A., Wang, D., et al. (2021). Host susceptibility factors render ripe tomato fruit vulnerable to fungal disease despite active immune responses. J. Exp. Bot. 72 (7), 2696–2709. doi:10.1093/jxb/eraa601
Songmei, L., Jie, J., Yang, L., Jun, M., Shouling, X., Yuanyuan, T., et al. (2019). Characterization and evaluation of OsLCT1 and OsNramp5 mutants generated through CRISPR/Cas9-mediated mutagenesis for breeding low Cd rice. Rice Sci. 26, 88–97. doi:10.1016/j.rsci.2019.01.002
Tan, J., Zhao, Y., Wang, B., Hao, Y., Wang, Y., Li, Y., et al. (2020). Efficient CRISPR/Cas9-based plant genomic fragment deletions by microhomology-mediated end joining. Plant Biotechnol. J. 18 (11), 2161–2163. doi:10.1111/pbi.13390
Tang, X., Lowder, L. G., Zhang, T., Malzahn, A. A., Zheng, X., Voytas, D. F., et al. (2017). A CRISPR-Cpf1 system for efficient genome editing and transcriptional repression in plants. Nat. Plants 3, 17103. doi:10.1038/nplants.2017.103
Tang, J., Chen, L., and Liu, Y. G. (2019). Off-target effects and the solution. Nat. Plants 5 (4), 341–342. doi:10.1038/s41477-019-0406-z
Tang, Y., Gao, C. C., Gao, Y., Yang, Y., Shi, B., Yu, J. L., et al. (2020). OsNSUN2-Mediated 5-methylcytosine mRNA modification enhances rice adaptation to high temperature. Dev. Cell 53 (3), 272–286. e277. doi:10.1016/j.devcel.2020.03.009
Tang, Y., Abdelrahman, M., Li, J., Wang, F., Ji, Z., Qi, H., et al. (2021). CRISPR/Cas9 induces exon skipping that facilitates development of fragrant rice. Plant Biotechnol. J. 19 (4), 642–644. doi:10.1111/pbi.13514
Thomazella, D. P. T., Seong, K., Mackelprang, R., Dahlbeck, D., Geng, Y., Gill, U. S., et al. (2021). Loss of function of a DMR6 ortholog in tomato confers broad-spectrum disease resistance. Proc. Natl. Acad. Sci. U. S. A. 118 (27), e2026152118. doi:10.1073/pnas.2026152118
Tran, M. T., Doan, D. T. H., Kim, J., Song, Y. J., Sung, Y. W., Das, S., et al. (2021). CRISPR/Cas9-based precise excision of SlHyPRP1 domain(s) to obtain salt stress-tolerant tomato. Plant Cell Rep. 40 (6), 999–1011. doi:10.1007/s00299-020-02622-z
Usman, B., Nawaz, G., Zhao, N., Liao, S., Liu, Y., and Li, R. (2020). Precise editing of the OsPYL9 gene by RNA-guided Cas9 nuclease confers enhanced drought tolerance and grain yield in rice (Oryza sativa L.) by regulating circadian rhythm and abiotic stress responsive proteins. Int. J. Mol. Sci. 21 (21), E7854. doi:10.3390/ijms21217854
Vlčko, T., and Ohnoutková, L. (2020). Allelic Variants of CRISPR/Cas9 induced mutation in an inositol trisphosphate 5/6 kinase gene manifest different phenotypes in Barley. Plants (Basel) 9 (2), E195. doi:10.3390/plants9020195
Walker, P. T. (1984). “Quantification and economic assessment of crop losses due to pests, diseases and weeds”, in: Advancing Agricultural Production in Africa: Cabs First Scientific Conference, Arusha, Tanzania, 12–18 February.
Wan, D. Y., Guo, Y., Cheng, Y., Hu, Y., Xiao, S., Wang, Y., et al. (2020). CRISPR/Cas9-mediated mutagenesis of VvMLO3 results in enhanced resistance to powdery mildew in grapevine (Vitis vinifera). Hortic. Res. 7, 116. doi:10.1038/s41438-020-0339-8
Wang, Y., Cheng, X., Shan, Q., Zhang, Y., Liu, J., Gao, C., et al. (2014). Simultaneous editing of three homoeoalleles in hexaploid bread wheat confers heritable resistance to powdery mildew. Nat. Biotechnol. 32 (9), 947–951. doi:10.1038/nbt.2969
Wang, F., Wang, C., Liu, P., Lei, C., Hao, W., Gao, Y., et al. (2016a). Enhanced rice blast resistance by CRISPR/Cas9-targeted mutagenesis of the ERF transcription factor gene OsERF922. PLoS One 11 (4), e0154027. doi:10.1371/journal.pone.0154027
Wang, H., La Russa, M., and Qi, L. S. (2016b). CRISPR/Cas9 in genome editing and beyond. Annu. Rev. Biochem. 85, 227–264. doi:10.1146/annurev-biochem-060815-014607
Wang, F. Z., Chen, M. X., Yu, L. J., Xie, L. J., Yuan, L. B., Qi, H., et al. (2017). OsARM1, an R2R3 MYB transcription factor, is involved in regulation of the response to arsenic stress in rice. Front. Plant Sci. 8, 1868. doi:10.3389/fpls.2017.01868
Wang, T., Deng, Z., Zhang, X., Wang, H., Wang, Y., Liu, X., et al. (2018a). Tomato DCL2b is required for the biosynthesis of 22-nt small RNAs, the resulting secondary siRNAs, and the host defense against ToMV. Hortic. Res. 5, 62. doi:10.1038/s41438-018-0073-7
Wang, Z., Hardcastle, T. J., Canto Pastor, A., Yip, W. H., Tang, S., and Baulcombe, D. C. (2018b). A novel DCL2-dependent miRNA pathway in tomato affects susceptibility to RNA viruses. Genes Dev. 32 (17-18), 1155–1160. doi:10.1101/gad.313601.118
Wang, C., Wang, G., Gao, Y., Lu, G., Habben, J. E., Mao, G., et al. (2020a). A cytokinin-activation enzyme-like gene improves grain yield under various field conditions in rice. Plant Mol. Biol. 102 (4-5), 373–388. doi:10.1007/s11103-019-00952-5
Wang, M., Xu, Z., Gosavi, G., Ren, B., Cao, Y., Kuang, Y., et al. (2020b). Targeted base editing in rice with CRISPR/ScCas9 system. Plant Biotechnol. J. 18 (8), 1645–1647. doi:10.1111/pbi.13330
Wang, Y., Liu, X., Zheng, X., Wang, W., Yin, X., Liu, H., et al. (2021a). Creation of aromatic maize by CRISPR/Cas. J. Integr. Plant Biol. 63 (9), 1664–1670. doi:10.1111/jipb.13105
Wang, Z., Wan, L., Xin, Q., Zhang, X., Song, Y., Wang, P., et al. (2021b). Optimizing glyphosate tolerance in rapeseed by CRISPR/Cas9-based geminiviral donor DNA replicon system with Csy4-based single-guide RNA processing. J. Exp. Bot. 72 (13), 4796–4808. doi:10.1093/jxb/erab167
Wolt, J. D., Wang, K., and Yang, B. (2016). The regulatory status of genome-edited crops. Plant Biotechnol. J. 14 (2), 510–518. doi:10.1111/pbi.12444
Wolter, F., Schindele, P., and Puchta, H. (2019). Plant breeding at the speed of light: The power of CRISPR/Cas to generate directed genetic diversity at multiple sites. BMC Plant Biol. 19 (1), 176. doi:10.1186/s12870-019-1775-1
Xie, K., Minkenberg, B., and Yang, Y. (2015). Boosting CRISPR/Cas9 multiplex editing capability with the endogenous tRNA-processing system. Proc. Natl. Acad. Sci. U. S. A. 112 (11), 3570–3575. doi:10.1073/pnas.1420294112
Xing, S., Chen, K., Zhu, H., Zhang, R., Zhang, H., Li, B., et al. (2020). Fine-tuning sugar content in strawberry. Genome Biol. 21 (1), 230. doi:10.1186/s13059-020-02146-5
Xu, Y., Lin, Q., Li, X., Wang, F., Chen, Z., Wang, J., et al. (2021). Fine-tuning the amylose content of rice by precise base editing of the Wx gene. Plant Biotechnol. J. 19 (1), 11–13. doi:10.1111/pbi.13433
Yang, Y., and Guo, Y. (2018). Elucidating the molecular mechanisms mediating plant salt-stress responses. New Phytol. 217 (2), 523–539. doi:10.1111/nph.14920
Yu, W., Wang, L., Zhao, R., Sheng, J., Zhang, S., Li, R., et al. (2019). Knockout of SlMAPK3 enhances tolerance to heat stress involving ROS homeostasis in tomato plants. BMC Plant Biol. 19 (1), 354. doi:10.1186/s12870-019-1939-z
Yu, H., Lin, T., Meng, X., Du, H., Zhang, J., Liu, G., et al. (2021). A route to de novo domestication of wild allotetraploid rice. Cell 184 (5), 1156–1170.e14. e1114. doi:10.1016/j.cell.2021.01.013
Yue, E., Cao, H., and Liu, B. (2020). OsmiR535, a potential genetic editing target for drought and salinity stress tolerance in Oryza sativa. Plants (Basel) 9 (10), E1337. doi:10.3390/plants9101337
Zaidi, S. S., Tashkandi, M., Mansoor, S., and Mahfouz, M. M. (2016). Engineering plant immunity: Using CRISPR/Cas9 to generate virus resistance. Front. Plant Sci. 7, 1673. doi:10.3389/fpls.2016.01673
Zeng, Y., Wen, J., Zhao, W., Wang, Q., and Huang, W. (2019). Rational Improvement of rice yield and cold tolerance by editing the three genes OsPIN5b, GS3, and OsMYB30 with the CRISPR-Cas9 System. Front. Plant Sci. 10, 1663. doi:10.3389/fpls.2019.01663
Zhang, H., Zhang, J., Lang, Z., Botella, J., and Zhu, J.-K. (2017). Genome editing—Principles and applications for functional genomics research and crop improvement. Crit. Rev. Plant Sci. 36, 291–309. doi:10.1080/07352689.2017.1402989
Zhang, A., Liu, Y., Wang, F., Li, T., Chen, Z., Kong, D., et al. (2019a). Enhanced rice salinity tolerance via CRISPR/Cas9-targeted mutagenesis of the OsRR22 gene. Mol. Breed. 39, 47. doi:10.1007/s11032-019-0954-y
Zhang, R., Liu, J., Chai, Z., Chen, S., Bai, Y., Zong, Y., et al. (2019b). Generation of herbicide tolerance traits and a new selectable marker in wheat using base editing. Nat. Plants 5 (5), 480–485. doi:10.1038/s41477-019-0405-0
Zhang, D., Tang, S., Xie, P., Yang, D., Wu, Y., Cheng, S., et al. (2022). Creation of fragrant sorghum by CRISPR/Cas9. J. Integr. Plant Biol. 64 (5), 961–964. doi:10.1111/jipb.13232
Zhou, J., Peng, Z., Long, J., Sosso, D., Liu, B., Eom, J. S., et al. (2015). Gene targeting by the TAL effector PthXo2 reveals cryptic resistance gene for bacterial blight of rice. Plant J. 82 (4), 632–643. doi:10.1111/tpj.12838
Zhou, J., Xin, X., He, Y., Chen, H., Li, Q., Tang, X., et al. (2019). Multiplex QTL editing of grain-related genes improves yield in elite rice varieties. Plant Cell Rep. 38 (4), 475–485. doi:10.1007/s00299-018-2340-3
Zhou, Y., Xu, S., Jiang, N., Zhao, X., Bai, Z., Liu, J., et al. (2022). Engineering of rice varieties with enhanced resistances to both blast and bacterial blight diseases via CRISPR/Cas9. Plant Biotechnol. J. 20 (5), 876–885. doi:10.1111/pbi.13766
Keywords: gene editing, CRISRR/cas, crop breeding, abiotic stress, biotic stress
Citation: Li Y, Wu X, Zhang Y and Zhang Q (2022) CRISPR/Cas genome editing improves abiotic and biotic stress tolerance of crops. Front. Genome Ed. 4:987817. doi: 10.3389/fgeed.2022.987817
Received: 06 July 2022; Accepted: 16 August 2022;
Published: 07 September 2022.
Edited by:
Hakim Manghwar, Lushan Botanical Garden (CAS), ChinaReviewed by:
Channakeshavaiah Chikkaputtaiah, North East Institute of Science and Technology (CSIR), IndiaAbira Chaudhuri, National Institute of Plant Genome Research (NIPGR), India
Copyright © 2022 Li, Wu, Zhang and Zhang. This is an open-access article distributed under the terms of the Creative Commons Attribution License (CC BY). The use, distribution or reproduction in other forums is permitted, provided the original author(s) and the copyright owner(s) are credited and that the original publication in this journal is cited, in accordance with accepted academic practice. No use, distribution or reproduction is permitted which does not comply with these terms.
*Correspondence: Qiang Zhang, zhangqiang1858@163.com; Yan Zhang, zhangyan6032@163.com
†These authors have contributed equally to this work