- 1Bigelow Laboratory for Ocean Sciences, East Boothbay, ME, United States
- 2Halmos College of Natural Sciences and Oceanography, Nova Southeastern University, Fort Lauderdale, FL, United States
- 3School of Marine Science and Policy, University of Delaware, Newark, DE, United States
- 4Colby College, Waterville, ME, United States
- 5Dartmouth College, Hanover, NH, United States
Among planktonic communities haloperoxidase enzymes may play a role in the control of intracellular and extracellular reactive oxygen species, in the generation of halogenated organic compounds and in chemical interactions between microbes. We introduce a sensitive fluorometric assay with a large dynamic range that is based on the dearylation of aminophenyl fluorescein (APF) to fluorescein by highly reactive oxygen species. Bromoperoxidase and chloroperoxidase enzymes catalyze the reaction between hydrogen peroxide and halides to generate highly reactive hypohalite intermediates able to dearylate APF. The fundamentals and standardization of the approach are illustrated using a partially purified, vanadium-dependent bromoperoxidase from the red seaweed Corallina officinalis. Laboratory cultures of two polar diatoms, Porosira glacialis and Fragilariopsis cylindrus, are used to illustrate the sensitivity and potential applications of the approach for in vitro, in vivo and in situ measurements of bromoperoxidase activity. These two diatoms differ in biovolume-specific bromoperoxidase activity by 2-orders of magnitude, from 5.4 to 0.044 fmol fluorescein μm-3 h-1, respectively. The approach is also used to investigate the partition of haloperoxidase activity between different size fractions of summer coastal planktonic communities, illustrating that generally more than 50% of the haloperoxidase activity occurred in a >10 μm size fraction that was dominated by diatoms. The assay has the potential to be of value in many aspects of haloperoxidase research, including developing an improved understanding of the roles of haloperoxidase enzymes in microbial planktonic communities.
Introduction
Haloperoxidase enzymes (HPO) catalyze the oxidation of halides by hydrogen peroxide (H2O2) to form a hypohalite (ClO-, BrO-, IO-) intermediate (1) that can react rapidly with organic substrates to produce halogenated compounds (2) or react with excess H2O2 to generate singlet oxygen (1O2) (Hewson and Hager, 1980; Everett et al., 1990). HPO can be classified according to the most electronegative halide they oxidize: chloroperoxidases (ClPO) oxidize chloride, bromide, and iodide; bromoperoxidases (BrPO) oxidize bromide and iodide; and iodoperoxidases (IPO) oxidize iodide. Haloperoxidases are generally metalloenzymes with either heme or vanadium co-factors, although enzymes not requiring a metal co-factor occur in some bacteria (Littlechild, 1999).
Vanadium-bromoperoxidases (V-BrPO) appear to be the most common form of haloperoxidase in the marine environment (Leblanc et al., 2015). Among planktonic microbes, V-BrPO activity has been demonstrated to occur in a variety of diatom species (Moore et al., 1996; Murphy et al., 2000; Hill and Manley, 2009) and a functional V-BrPO has been characterized in several strains of the globally distributed marine cyanobacterium Synechococcus sp. (Johnson et al., 2011). In contrast, a putative heme-containing IPO was found in the microalgal rhodophyte Porphyridium purpureum (CCAP 1380/3), and a vanadium-containing IPO (V-IPO) has recently been characterized and sequenced in the marine flavobacterium Zobellia galactanivorans (Fournier et al., 2014). Information on ClPOs among planktonic microbes is limited, although ClPOs have been characterized from sediment-associated bacteria (Winter et al., 2007; Bernhardt et al., 2011).
Marine HPO enzymes are implicated in a variety of intracellular and extracellular physiological roles, may have an influence on the redox chemistry of seawater and are the source of a suite of halogenated compounds (Butler and Walker, 1993; La Barre et al., 2010; Wever and van der Horst, 2013). Through halide-assisted disproportionation of H2O2 (1), HPO enzymes are likely to be a component of the intricate antioxidant systems used by many cells, including both phototrophic and heterotrophic planktonic microbes, to maintain balanced intracellular levels of reactive oxygen species (ROS) (Mittler, 2017). Planktonic microbes make a large contribution to the production and turnover of ROS, including H2O2, in the surface ocean (Vermilyea et al., 2010; Morris et al., 2016; Roe et al., 2016). As broadly captured in reaction (2), in which varied levels of halide selectivity and organic substrate selectivity may be involved, HPO activity is responsible for the production of multiple halogenated compounds (Butler and Carter-Franklin, 2004). Among a variety of ecophysiological roles, halogenated secondary metabolites act as signaling molecules to reduce epiphyte colonization in some seaweeds and microalgae (Nylund et al., 2008; Syrpas et al., 2014), in allelopathic interactions between benthic diatoms (Vanelslander et al., 2012) and as grazing deterrents in seaweeds (Enge et al., 2012). HPO-derived volatile halogenated organic compounds (VHOC) can be emitted from the ocean and play a crucial role in atmospheric chemistry by reducing concentrations of O3, thereby increasing transmission of UV, reducing the greenhouse effect and influencing the lifetimes of other climate active components (Carpenter et al., 2012). There is a clear need to better understand which components of the plankton possess HPO activity, what functions the enzyme activity has and how that affects biogeochemical cycles of bromine and iodine, in particular.
One of the limitations to understanding the role of HPO activity in the oceans has been the availability of sensitive, easily applied assays. A variety of colorimetric approaches to determine HPO activity have been developed, some of which have previously been used to investigate HPO activity in microalgae. The classic approach to determining ClPO and BrPO activity involves monitoring the decrease in absorbance of monochlorodimenone (MCD) at 290 nm as it is halogenated to bromochlorodimenone (Br-MCD) (Hewson and Hager, 1980). This approach was used in the first study to demonstrate BrPO activity in microalgae using partially purified enzyme from the diatom Nitzschia sp. CCMP580 (Moore et al., 1996). An alternative spectrophotometric assay involving the conversion of phenol red (phenolsulfonphthalein) to bromophenol blue or iodophenol blue has been used extensively to study BrPO or IPO activity in macroalgae (De Boer et al., 1987) and was adapted to monitor “in situ” brominating activity by suspensions of 11 species of diatoms (Hill and Manley, 2009). The same “in situ” phenol red assay was used to investigate the physiological basis of brominating activity in two polar diatoms (Hughes and Sun, 2016). Verhaeghe et al. (2008) introduced an assay for IPO and BrPO activity based on the halogenation of thymol blue [thymolsulfonphthalein (TB)] and spectrophotometric measurement of the formation of TBBr2 and TBI2. This was successfully applied to determine V-BrPO rates in crude extracts of the cyanobacterium Synechococcus sp. (Johnson et al., 2011), and has the advantage of also being able to discriminate IPO activity. In the majority of cases, large samples of laboratory culture (e.g., 1 to 3 l) or long duration assays (∼24 h) have been required for these colorimetric assays and a more sensitive approach would be an advantage for studies of the physiological and biogeochemical roles of HPOs among planktonic microbes.
The present study aimed to demonstrate the efficacy of a novel assay for BrPO (and ClPO) activity, based on the fluorescent probe [6-(4′-amino)phenoxy-3H-xanthen-3-on-9-yl]benzoic acid [aminophenyl fluorescein (APF)], designed to selectively detect highly reactive oxygen species (hROS) (Setsukinai et al., 2003). The APF probe has previously been used to detect the enzymatic activity of myeloperoxidase found in neutrophils and eosinophil peroxidase, both heme-proteins that generate either HOCl or HOBr in mammalian blood (Flemmig et al., 2012). In the present study APF-based assays are used in three applications: (i) to demonstrate the generation of highly reactive hypohalite by the partially purified V-BrPO of the red seaweed Corallina officinalis and to establish the temperature response and pH optima for V-BrPO of C. officinalis; (ii) quantify the BrPO activity in two different species of diatom, Porosira glacialis and Fragilariopsis cylindrus; and (iii) measure BrPO activity in planktonic communities of coastal waters and investigate the size-distribution and temporal change of enzyme rates.
Materials and Methods
Materials
Partially purified (∼10%) bromoperoxidase from Corallina officinalis was obtained as a lyophilized powder from Millepore Sigma, Burlington, NJ, United States. The fluorescent probe APF was obtained as a 5 mmol l-1 solution in dimethylformamide from ThermoFisher, Waltham, MA, United States. The non-axenic diatom isolates Porosira glacialis CCMP651 and Fragilariopsis cylindrus CCMP3323 were sourced from the National Center for Marine Algae and Microbiota, Bigelow Laboratory for Ocean Sciences, East Boothbay, ME, United States.
Demonstrating the APF Response to V-BrPO From C. officinalis
To investigate whether APF can be used as a quantitative probe for BrPO activity, the V-BrPO of C. officinalis was used as a representative standard. This standard was chosen as it is readily available [Millipore-Sigma (B2170)], previous studies indicate that V-BPOs are present in some microalgae, particularly diatoms (Moore et al., 1996; Hill and Manley, 2009), and among the protein structures of V-HPOs that have been characterized, those from red seaweeds including C. officinalis V-BrPO, are closely aligned with those of cyanobacteria (Leblanc et al., 2015). The enzyme was reconstituted in 100 mmol l-1 2-(N-morpholino)ethanesulfonic acid (MES) buffer adjusted to pH 7, and prior to experimentation 2 mmol l-1 sodium orthovanadate was added to the enzyme to ensure full loading of the active sites with vanadate (Yu and Whittaker, 1989). MES buffer was used because of its non-coordinating properties and the potential for phosphate-based buffers to inhibit enzyme activity by competing with the vanadate cofactor (De Boer et al., 1986; Everett et al., 1990).
To demonstrate a calibration of the assay the reaction were carried out in 50 mmol l-1 MES buffer containing 100 mmol l-1 KBr and 1.25 μmol l-1 APF to which varied amounts of reconstituted V-BrPO were added. The reaction was carried out at pH 6.4 which was the optimum pH previously determined in phosphate buffer (Sheffield et al., 1992). The assays were generally conducted over a 300 s period. To initiate the reaction H2O2 was added to generate a final concentration of 50 μmol l-1 after ∼50 s. Fluorescence was measured using a Photon Technology International (PTI) QuantaMaster fluorometer. Excitation wavelength was set to 490 nm and emission recorded at 515 nm by parallel photomultiplier detection systems; lamp output was 74 W. Fluorescence generation was monitored continuously in a 3 ml quartz cuvette with stirring at 25°C.
To confirm that BrPO, rather than IPO or ClPO activity is detected by the APF assay, the assay mixture was altered to contain either 100 mmol l-1 KI or KCl instead of KBr. To verify that the observed fluorescence generation was enzymatically derived, sodium azide (NaN3) was added to the incubation assay at a final concentration of 1 mmol l-1.
Temperature and pH Response of V-BrPO From C. officinalis
The temperature response of C. officinalis V-BrPO was determined by altering the assay temperature between 0 and 30°C in the quartz cuvettte using a Northwest Quantum Temperature Controller (TC-125) integrated with the fluorometer. An enzyme concentration of 0.5 mU ml-1 was used for the temperature tests. The pH sensitivity of C. officinalis V-BrPO activity was determined by altering the pH of the 50 mmol l-1 MES buffer containing 0.5 mU ml-1 of V-BrPO to between pH 5.8 and 7.8 at 25°C. For both temperature and pH tests the assays were performed in triplicate for each treatment level.
BrPO Activity in Microalgal Cultures
Two species of polar diatoms were chosen to test the suitability of the APF assay to determine BrPO activity in microalgae. The polar diatom P. glacialis CCMP651 has previously been shown to generate volatile halogenated compounds (Moore et al., 1996) and to possess high BrPO activity relative to other diatom species that have been tested (Hill and Manley, 2009). To our knowledge, the haloperoxidase activity of the sea ice diatom F. cylindrus CCMP3323 has not been reported previously. Both diatom strains were grown in L1 medium (Guillard and Hargraves, 1993), at 4°C under a 14h:10h light:dark cycle and light intensity of 60 μmol photons m-2 s-1.
Three approaches that provide contrasting measurements of BrPO rates in microalgae were compared using samples taken at the same time from each diatom culture. In order to determine an in vitro rate, 1 ml of culture was gravity filtered on to a 2.0 μm polycarbonate filter [(1) Figure 1]. Cells were then lysed by bead beating in the presence of a non-ionic surfactant. The polycarbonate filter was placed in a bead beating tube (Qiagen, Germantown, MA, United States) containing 1 ml of 50 mmol l-1 MES buffer and 0.1% of Triton X-100. Approximately 0.5 g zirconia beads were added to the tube and bead beating was carried out using a Retsch Mixer Mill MM 400 set to run at 30 beats s-1 for 3 min. A series of tests were carried out to optimize the bead beating process for the specific instrument used in this study. It is not clear whether the BrPO of either diatom strain was contained within the cells, associated with membranes or located on the exterior of the cells, therefore, no attempt was made to separate dissolved and particulate material following bead beating for the BrPO activity assay. A 50 μl portion of the crude extract was added to 3 ml of 50 mmol l-1 MES buffer at pH 6.4 in a quartz cuvette. When bead beating was followed by centrifugation and measurements carried out on the supernatant, only ∼50 % of the BrPO activity was obtained.
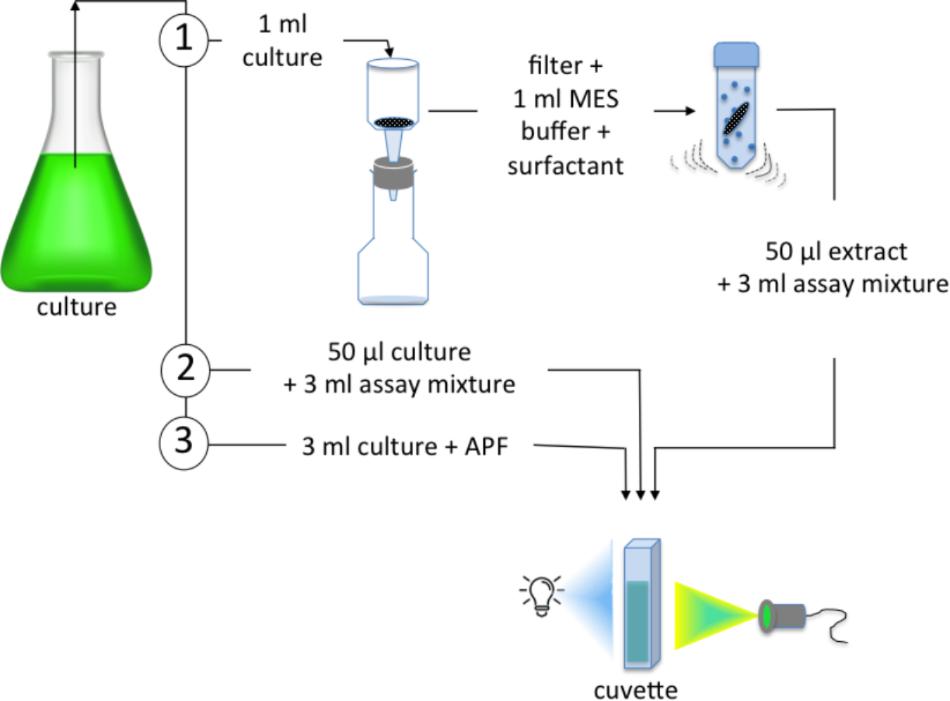
Figure 1. The three assays tested using laboratory cultures of diatoms to determine: (1) an in vitro rate involving bead beating of a filtered sample to generate a crude extract that is added to an assay mixture of MES buffer, KBr and APF; (2) an in vivo rate in which intact cells were added directly to the assay mixture; (3) an in situ rate in which APF was added to the algal culture. The addition of H2O2 initiated the reaction in (1) and (2), while in (3) an “ambient in situ” rate was determined with no H2O2 addition and a “maximal in situ” rate obtained by adding H2O2.
An alternative in vivo measure of BrPO activity was determined by the addition of 50 μl of algal culture directly to the 50 mmol l-1 MES buffer at pH 6.4 in a quartz cuvette [(2) Figure 1]. In each case, fluorescence was measured for ∼50 s in order to obtain a baseline rate of fluorescence increase, prior to the addition of 50 μmol l-1 final concentration of H2O2. In the present study, the in vivo preparation using intact cells in an assay buffer is a comparable measurements to the “in situ” measurement carried out by Hill and Manley (2009) and Hughes and Sun (2016).
An in situ assay involved adding APF directly to the diatom cultures [(3) Figure 1]. An “ambient in situ rate” of fluorescence increase was assumed to be due to ambient levels of H2O2; a “maximal in situ rate” was recorded following the addition of 50 μmol l-1 H2O2 final concentration. Each assay was carried out in triplicate using three subsamples of each diatom culture.
It should be noted that both diatom cultures included bacterial populations that may have contributed to BrPO activity. As haloperoxidases may play a role in algal-bacterial interactions (Vanelslander et al., 2012; Syrpas et al., 2014), we chose to use non-axenic cultures in the present study. Unattached bacteria are expected to have passed through the 2.0 μm filter used in the in vitro preparation and therefore, to not have been included in the assay. Tests carried out by Hill and Manley (2009) on similar diatom cultures, found little evidence of bacterial contributions to bromination rates. Whether diatoms respond to being cultured in an axenic environment by altering their haloperoxidase activity, remains to be established.
In the APF assay, the hypohalite that generates fluorescein will potentially also react with other organic compounds if they are present, including molecules susceptible to electrophilic attack and halogenation. Where a purified enzyme is being assayed, competing organics are expected to have a minimal influence on the reaction. In the case of the microalgal and plankton analyses, there is the potential for sufficiently high concentrations of competing compounds to reduce the rate of fluorescein generation. To address this issue, a saturating concentration of APF was added to the assays to reduce the influence of competing compounds and obtain a maximal rate of enzyme activity. The concentration of APF required to effectively saturate the reaction was established empirically with each of the sample types.
BrPO Activity in Natural Plankton Communities
A temporal study carried out using water samples collected from the deep-water dock at Bigelow Laboratory (43° 51′ N, 69° 35′ W) in the coastal Gulf of Maine, aimed to demonstrate the potential of the assay for investigating BrPO activity in natural planktonic systems. On each date, three seawater samples were collected within ± 1 h of high tide. A polypropylene pipe with tap was used to obtain a 2 m depth-integrated ∼500 ml sample. The samples were stored at ambient temperature and in the dark until processed, usually within < 1 h. For BrPO analysis 100 ml from each seawater sample was first passed through a 200 μm mesh and then sequentially size-fractionated through a filter tower cascade of 25 mm 10, 2, and 0.2 μm polycarbonate filters. The 10 and 2 μm filtration occurred under gravity but it was necessary to use gentle vacuum (∼5inches Hg) to filter the 0.2 μm fraction in a reasonable time. Filters were immediately frozen in liquid nitrogen until analyzed that day. An in vitro rate was determined as illustrated in (1) (Figure 1) except that the whole 1 ml of crude extract was added to 2 ml of assay mixture in the quartz cuvette including the polycarbonate filter. In separate tests the presence of the polycarbonate filter was shown to have a minimal (∼2%) impact on measured rates of the activity of the C. officinalis V-BrPO.
Phytoplankton Composition and Biomass
Chlorophyll a (Chl a) was measured as a proxy of phytoplankton biomass. To obtain total and >10 μm Chl a concentrations, 100 ml was gravity filtered through a 25 mm 10 μm polycarbonate filter and a separate 100 ml gravity filtered through a 47 mm GF/F filter. Filters were immediately frozen in liquid nitrogen and stored at -80°C until analyzed. Chl a concentration was determined on a PTI fluorometer following extraction in 90% acetone with emission set at 680 nm and excitation at 430 nm. Chl a concentration was calculated from the difference between non-acidified and acidified measurements (Yentsch and Menzel, 1963). For the analysis by microscopy of phytoplankton composition, 100 ml of seawater was preserved using acid-lugols fixative and stored in the dark at room temperature. Cells were enumerated in 50 ml samples using Utermöhl settling chambers. The dimensions of cells of the most abundant diatom taxa were measured and cell volume estimates made based on standard geometric shapes in order to determine a total diatom biovolume (Hillebrand et al., 1999).
Results and Discussion
This section aims to demonstrate that the APF fluorescent probe for hROS designed by Setsukinai et al. (2003), provides a very sensitive and specific probe for BrPO activity with a high dynamic range that offers considerable potential to investigate haloperoxidase activity and function in microbial planktonic communities and in other situations.
Bromoperoxidase-Mediated Conversion of APF to Fluorescein
The conversion of non-fluorescent APF to fluorescein through the production of HOBr (or Br2, , Enz-Br) by V-BrPO of C. officinalis is shown by increases in fluorescence following the addition of H2O2 to the enzyme assay mixture at approximately 50 s after initiation of data collection (Figure 2A). Rates of increase in fluorescence were calculated during the linear portion of the response from 60 to 120 s (Figure 2C). This rate of increase in fluorescence is directly related to enzyme concentration for concentrations ranging over more than 3-orders of magnitude from 0.008 U l-1 to 2.500 U l-1 (Figure 2C). A non-limiting concentration of APF was established for the reaction assay involving C. officinalis BrPO of 1.25 μM up to a fluorescein production rate of >3.5 nmol l-1 s-1 (Figure 2). The standard assay showed a high level of reproducibility with coefficients of variation from triplicate analyses of 14 % at the lowest enzyme concentration of 0.008 U l-1 to < 5 % at 2.500 U l-1. Increased sensitivity and improved precision at the lowest activities could be achieved by running the assay for longer than the 300 s that was used.
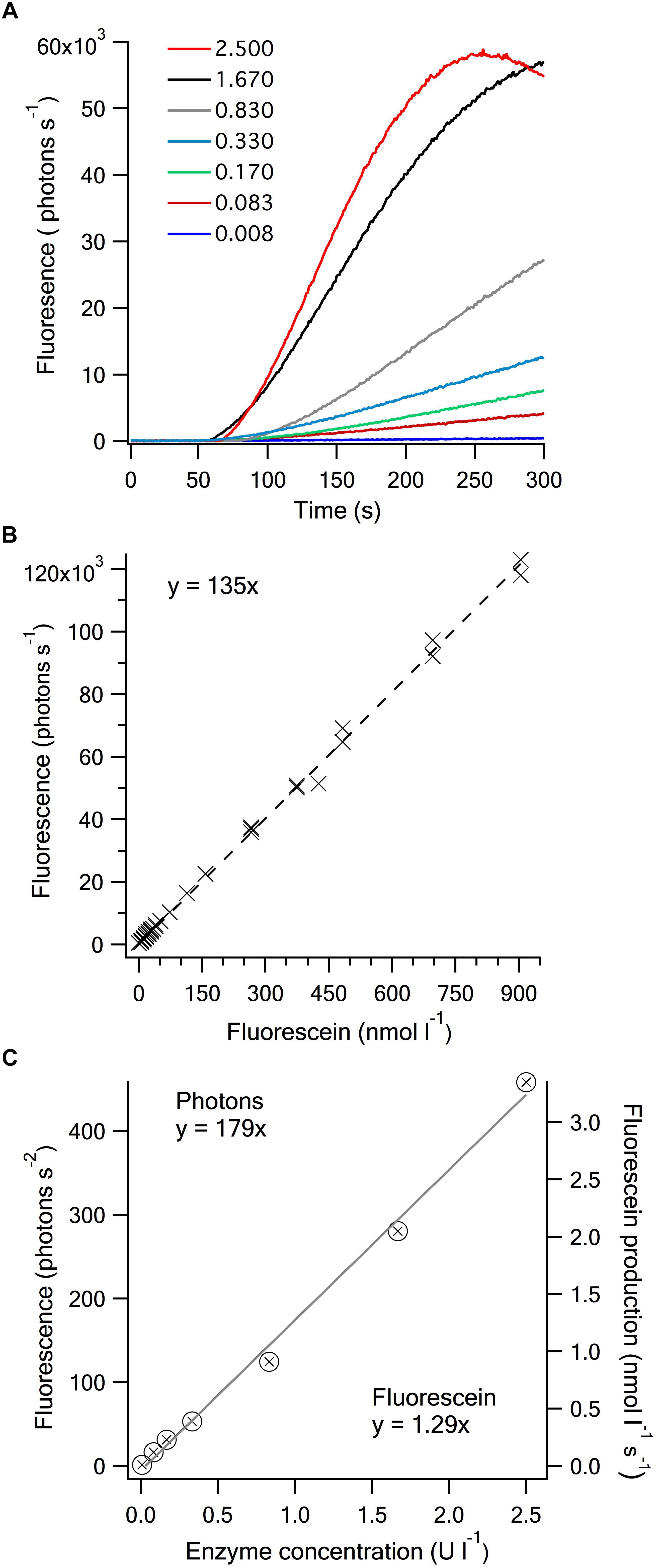
Figure 2. Bromoperoxidase concentration dependence of the dearylation of APF to fluorescein. (A) Time-dependent fluorescence increase on addition of H2O2 to assays containing varied amounts of Corallina V-BrPO (U l-1). The enzyme was added to an assay mixture comprised of 50 mmol l-1 MES buffer, 100 mmol l-1 KBr and 1.25 μmol l-1 APF at pH 6.4, with the addition of 50 μmol l-1 H2O2 at approximately 50 s to start the reaction. (B) Relationship between fluorescein concentration and fluorescence. The relationship was compiled from measurements on three separate dates (slope ( ± SD): 135 ( ± 0.8 × 103) ×103 photons s-1 nmol l-1). (C) The rate of fluorescence increase as a function of V-BrPO concentration from the assays shown in Figure 2A. The rate of fluorescence increase was determined over 60 s of the linear portion of the response curve, starting ∼20 s after the addition of the H2O2 (slope ( ± SD): 1.79 ( ± 6)photons s-2 U l-1). The fluorescein production rate increase (slope ( ± SD): 1.29 ( ± 0.04) nmol s-1 U-1) was calculated from the empirically determined relationship between fluorescence (photons s-1) and fluorescein concentration (nmol l-1) shown in Figure 2B.
Based on an established relationship between fluorescein and fluorescence (Figure 2B) the fluorescence increase can be directly related to the rate of fluorescein production and APF cleaved. In the standard assay conditions shown in Figure 2 in which 1.25 μmol l-1 APF was used, this relates to 1.29 nmol fluorescein s-1 U-1 V-BrPO, or 77 nmol min-1 U-1. Yamada et al. (1985) defined the standard activity of 1 U of BrPO enzyme as the amount that would catalyze the formation of 1 μmol min-1 of monobromomonochlorodimenone (Br-MCD) from monochlorodimenone (MCD) in a standard assay buffer that contained 60 μmol l-1 MCD. The bromination of TB to TBBr2 showed similar rates of reactivity to MCD when the two compounds were mixed in the presence of V-BrPO (Verhaeghe et al., 2008), indicating that the sensitivity of the TB assay is similar to the MCD assay. We have not investigated the APF concentration dependence of the enzyme-specific fluorescein production using the purified V-BrPO of C. officinalis but expect comparable enzyme-specific rates (μmol min-1) to those of MCD or TB bromination if comparable APF concentrations were used in the assay (i.e., ∼50-fold higher). This suggests that the dearylation of APF by hROS, in this case HOBr, to release fluorescein, requires similar activation energy to the bromination of MCD and TB. Where the APF assay has an advantage in terms of sensitivity, is in the considerably more specific and sensitive measurement of fluorescence compared to absorbance.
When KI was substituted for KBr in the assay buffer, leading to the production of HOI (or I2, , Enz-I) by C. officinalis V-BrPO, no dearylation of APF to fluorescein occurred, confirming the specifity of the APF probe for highly ROS (Table 1). The substitution of KBr with KCl confirmed the inability of the V-BrPO to oxidize Cl- to produce HOCl (Table 1), as APF is susceptible to dearylation by the hypochlorite anion (Setsukinai et al., 2003), and would display chloroperoxidase activity if present. The lack of fluorescence when KI is present indicates that the APF assay could not be used to detect IPO activity. The addition of 1 mmol l-1 NaN3 caused a 97% decrease in fluorescein production of the V-BrPO of C. officinalis (Table 1). Previously, NaN3 has been shown to result in the complete loss of brominating activity in in situ assays of P. glacialis (Hill and Manley, 2009) and to partially inhibit the bromination of MCD by purified V-BrPOs of Corallina pilulifera (Itoh et al., 1986) and Ascophyllum nodosum (Everett et al., 1990). The addition of NaN3 inactivates horse radish peroxidase following the oxidation of (NaN3) to an azidyl radical () that binds to the heme prosthetic group (Ortiz de Montellano et al., 1988). As the vanadate prosthetic group of V-BrPO lacks similar carbon bonds, the mechanism by which the activity of V-BrPO is inhibited or inactivated by NaN3 warrants further investigation.

Table 1. The effect of different halide substrates and enzyme inhibitor sodium azide on Corallina V-BrPO activity.
The APF assay is amenable to pH and temperature variation permitting investigations of the physiological basis of BrPO activity. The response to temperature of V-BrPO activity was typical of many enzyme reactions with a 100% increase in activity per 10°C temperature range between 5 and 30°C (Figure 3A). A characteristic of HPO enzymes is their robustness in the face of pH and temperature change. The C. officinalis V-BrPO has been shown to maintain high activity up to 60°C (Itoh et al., 1986). When pH was adjusted in the 50 mmol l-1 MES buffer containing 0.5 mU ml-1 of V-BrPO, fluorescein production varied >2-fold from 0.89 nmol l-1 s-1 at pH 6.2 to 2.03 nmol l-1 s-1 at between pH 7.0 and 7.2 (Figure 3B). The apparent pH optimum of pH 7.0 is higher than previously reported for the V-BrPO of C. officinalis of pH ∼6.0 (Yamada et al., 1985; Itoh et al., 1986; Carter et al., 2002). In both cases where the lower pH optimum was established a phosphate buffer was used in treatments that spanned the range pH 5.0 to 8.0. In contrast, MES is one of the Good’s buffers and has a working range of approximately ± 1 pH unit of the pKa of 6.1 at 25°C. Enzyme pH is known to vary between different types of buffer but further studies are required to confirm this is the case for the V-BrPO of C. officinalis.
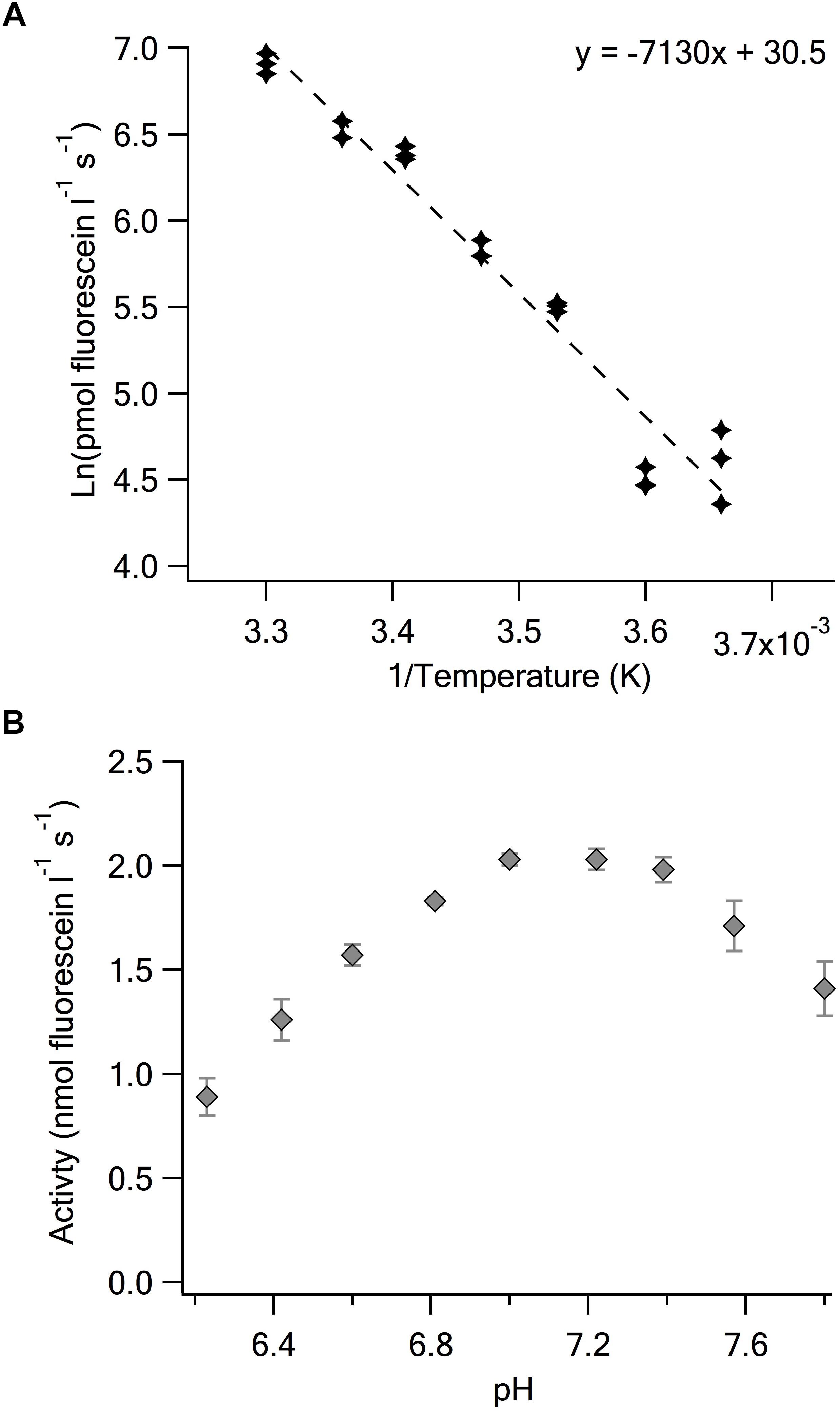
Figure 3. Determination of the temperature and pH dependence of Corallina V-BrPO. (A) Arrehnius plot determined using the standard assay of 50 mmol l-1 MES buffer, 100 mmol l-1 KBr, 1.25 μmol l-1 APF, 50 μmol l-1 H2O2 and V-BrPO 0.5 mU ml-1 at pH 6.4. Replicate measurements at each temperature are shown [slope ( ± SD): –7130 ( ± 380) + 30.5 ( ± 1.3)]. (B) Enzyme activity determined in the same assay mixture at 25°C over a range of pH. Error bars are the SD of triplicate assays at each pH.
BrPO Activity in Diatoms Cultures
Quantitative Interpretation of the APF Assay
An APF saturating approach was tested for the in vitro and in vivo assays of the bead-beaten crude cell extracts from the diatom cultures and showed similar saturation levels of ≤20 μmol l-1 for both P. glacialis and F. cylindrus sample preparations (Figure 4A). The same issue of competition exists for the other HPO assays that monitor a secondary product of the enzyme reaction particularly those that depend on observing the bromination of a substrate in “in situ” assays or in sample extracts in which other compounds are included that may react with the enzyme-derived hypohalite.
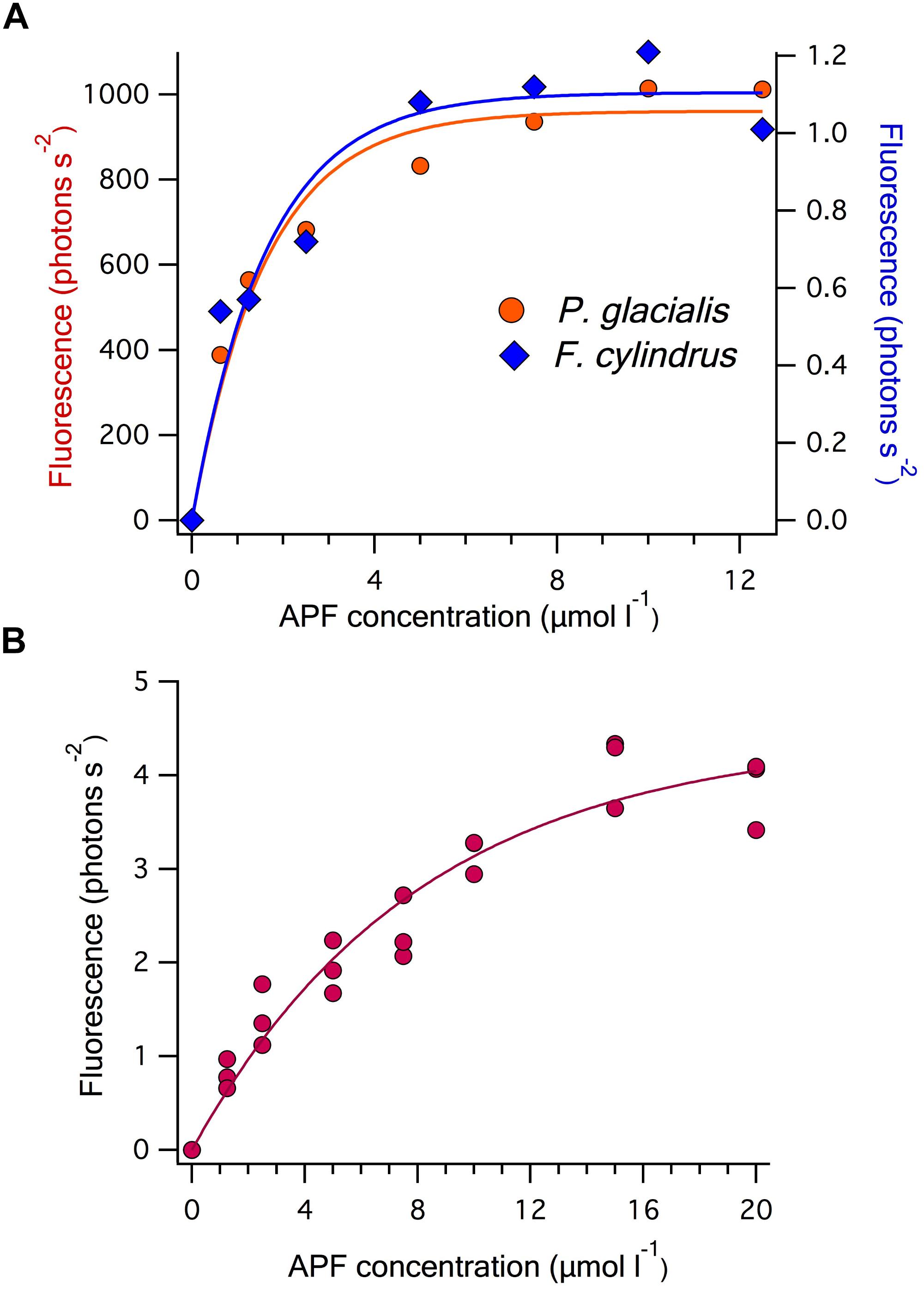
Figure 4. APF concentration dependence of the rate of fluorescence increase in: (A) the in vitro assays used to determine BrPO activity of 1 ml of culture of the two diatom strains Porosira glacialis and Fragilariopsis cylindrus. The curves are Michaelis-Menten fits of each dataset giving values of Vmax and KM of 1053 photons s-2 and 1.08 μmol l-1 for P. glacialis and 1.1 photons s-2 and 0.8 μmol l-1 for F. cylindrus; and (B) the in vitro assay of a 100 ml natural seawater sample filtered onto a >10 μm polycarbonate filter. Vmax and KM constants for the Michaelis-Menten fit are 4.9 photons s-2 and 6.7 μmol l-1, respectively.
Interpretation of the Different Assay Preparations
The APF-based measurements confirm considerable variation in BrPO activity among species of diatoms (Table 2 and Moore et al., 1996; Hill and Manley, 2009). The in vitro assay involved the lysis of cells followed by the measurement of all released enzyme activity. This theoretically provides an estimate of the maximum potential BrPO activity of the organism. The fluorescence-based rate measurement can be used to calculate a fluorescein production rate and on the basis of the activity determined for the C. officinalis BrPO, the cell-specific equivalent enzyme units (Table 2). Cell-specific BrPO activity varied by more than 4-orders of magnitude between P. glacialis and F. cylindrus. Even when cell biovolume is considered, which ranged between 4440 μm3 cell-1 for P. glacialis and 31 μm3 cell-1 for F. cylindrus, there is 2-orders of magnitude difference in biovolume-specific BrPO activity (Table 2). High interspecific variation in BrPO activity has been previously observed among diatoms species using an in situ assay in which cells were pre-concentrated by centrifugation and then re-suspended in a buffer containing phenol red (Hill and Manley, 2009). In that study, P. glacialis CCMP 651 showed a brominating activity that when normalized to cell surface area, was >10-fold higher than the ten other diatom species tested. The phylogenetic relationship of haloperoxidase activity and the physiological consequences of intraspecific and interspecific differences in activity among diatoms remain to be fully resolved.
Cell-specific BrPO activity was ∼50% lower for P. glacialis in the in vivo assay compared to the in vitro assay, suggesting some of the enzyme may be located within the cells and released by the bead beating (Table 2). In contrast, no significant difference was apparent between the in vitro and in vivo sample preparations for F. cylindrus, in part because the higher relative level of uncertainty at the lower rate hampers statistical discrimination (Table 2). Limitation of the enzyme reaction and APF oxidation in the in vivo assay may be due to restricted transport rates of substrates or products in or out of the cells. Determining whether HPO are located within the cell or extracellularly may help explain their physiological function and kinetic properties. It is possible that the addition of H2O2 to the culture enhanced intracellular H2O2 concentrations in P. glacialis and that the observed increased fluorescein production stemmed from intracellular BrPO activity. APF may be taken up and converted to fluorescein in cells. For instance, intracellular loading of APF was used to demonstrate formation of hypohalous acids by eosinophil and neutrophil peroxidases using fluorescence microscopy (Setsukinai et al., 2003) and flow cytometry (Flemmig et al., 2012) and hence, APF may be taken up within P. glacialis cells. Alternatively, HOBr may be released from the cells and react with the APF in the seawater media. In longer term assays, HOBr produced by P. glacialis BrPO activity was shown to diffuse from the cells across a dialysis membrane and subsequently react with phenol red (Hill and Manley, 2009). H2O2 is generally not freely diffusible across biological membranes and transport into cells may have been regulated by aquaporin water channels (Dynowski et al., 2008).
The cellular location of HPO may be reflected in the pH optima of the enzymes, something that was not tested in the present study. For instance, pH optima differs by >1 pH unit between the two BrPO found in Ascophyllum nodosum, reflecting their intra-thallus and surface distribution in the seaweed (Krenn et al., 1989).
The in situ assays highlight the possibility that microalgal BrPOs may influence seawater H2O2 concentrations, and that the level of influence on H2O2 concentrations may be a product of diatom species composition. When APF was added directly to the P. glacialis culture, a constant rate of fluorescence increase was observed, indicating an “ambient” rate of HOBr formation and APF oxidation. This suggests that ambient concentrations of H2O2 were being produced in the culture and were reacting with the BrPO of P. glacialis. Whether this occurred within the cells following uptake of APF or occurred extracellularly is unclear. The addition of H2O2 to the APF-containing P. glacialis culture resulted in enhanced fluorescein production (Table 2). In contrast, if ambient BrPO-generated fluorescence production occurred in the F. cylindrus culture when APF was added, the rates were not discernable using this particular combination of substrate concentrations and analysis time. This was also the case following the addition of H2O2 (Table 2). Depending on which species are present, diatoms may play an important contribution to the control of H2O2 concentrations in natural seawater. Note, no attempt was made to control levels of pH in the in situ assays and hence, the HPO activity that was measured occurred at the in situ pH of the media at the time of sampling or possibly, of the intracellular environment in which the enzyme (s) was located. Moreover, the considerably lower cell-specific rates observed in the in situ assays compared to the in vitro and in vivo rates (Table 2) may have been partly a result of higher concentrations of other compounds competing with APF for the reaction with HOBr.
BrPO in Coastal Waters
The dependence of fluorescein production rate on APF concentration was also tested for >10 μm 100 ml filtered seawater samples in order to establish a saturating level for use in the assays (Figure 4B). In consequence, 20 μmol l-1 APF, similar to the value established for the in vitro sample preparations for the diatom cultures, was added to the assay buffer mixture following bead-beating. This APF-saturated, in vitro assay provides a measurement of the maximum potential BrPO activity in the natural plankton community.
During mid-summer, from 15th June to 12th July, environmental variables at high tide, when samples were collected, were relatively constant. At that time, water temperatures gradually increased from 14.5 to 16.0°C while salinity varied between 31.5 and 32.5 PSU. Total Chl a concentrations peaked at 8.9 μg l-1 on 22nd June and generally declined to 3.3 μg l-1 on 12th July. During this period the diatom population transitioned from a centric to a pennate diatom dominated population and declined in total biovolume, in alignment with the >10 μm Chl a size fraction (Figure 5A). The chain-forming centric diatom Leptocylindrus minimus made-up the bulk of diatom biovolume initially but declined in the following weeks while pennate diatoms belonging to Pleurosigma and Fragilariopsis families remained relatively constant (Figure 5B).
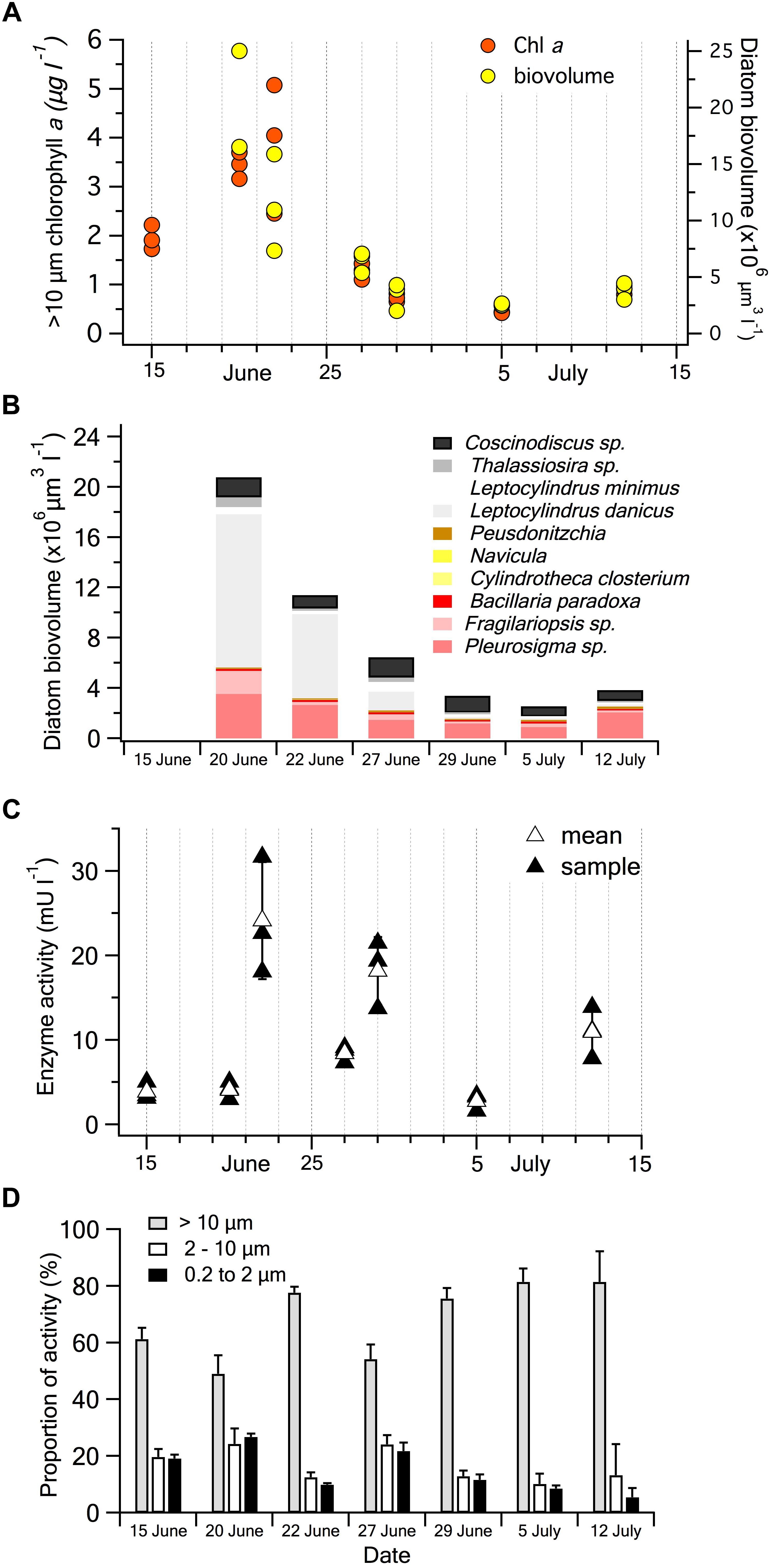
Figure 5. BrPO activity and phytoplankton in seawater samples collected in Gulf of Maine coastal waters in June-July 2018. (A) Temporal change in Chl a concentration in the >10 μm size fraction and the total diatom biovolume determined by microscopy in each seawater sample. (B) Average biovolume and taxonomic composition of diatoms in each of the seawater samples, note no sample was analyzed on 15th June. (C) Temporal change in BrPO activity of the >10 μm size fraction measured using an in vitro APF-saturated assay. Values for each individual seawater sample and the mean of the triplicate samples are shown. The error bars are the SD of the mean. (D) Temporal changes of the relative proportions of BrPO activity in the three plankton size fractions.
Over the course of the month, BrPO activity in the >10 μm fraction varied by more than 10-fold between 3.0 and 32.7 mU l-1 for the individual seawater samples (Figure 5C). For these size-fractionated natural samples, the coefficients of variation ranged from 11 to 37% among the triplicate water samples. This high variability may be a product of real differences between samples as Chl a analyses also showed relatively high coefficients of variation among samples, ranging from 3 to 34% and the levels of variability on individual dates appears to show similar trends to BrPO activity (Figures 5A,C). For triplicate 100 ml subsamples from the same water sample, the coefficient of variation was ∼10%.
Based on measurements of laboratory cultures (Moore et al., 1996; Murphy et al., 2000; Hill and Manley, 2009) diatoms were expected to dominate the BrPO activity in the coastal water samples. Over the course of the month, no significant relationships were observed between the >10 μm BrPO activity vs. >10 μm Chl a concentrations, between the >10 μm BrPO activity and diatom biovolume, or between total BrPO activity and diatom biovolume. Temporal patterns of the biovolume of individual taxonomic groups of diatoms also showed no significant correlations to >10 μm BrPO activity. However, total BrPO activity was consistently dominated by the >10 μm size fraction on each sampling day (Figure 5D). A highly significant linear relationship occurred between the >10 μm Chl a concentration and diatom biovolume across all samples [y = 3.72x + 1.10, P = 1.5 × 10-5, r2 = 0.72; where y = biovolume (× 106 μm3 l-1) and x = Chl a (μg l-1)]. The close relationship between the >10 μm Chl a and diatom biovolume, apparent in their similar temporal trends (Figure 5A), supports the suggestion that diatoms may have been responsible for the dominant BrPO activity of the >10 μm size fraction. If this was the case, it means that there is not a straightforward relationship between diatom biovolume and BrPO activity, possibly as a result of changes in diversity that we did not observe or due to physiological responses. Cell-specific approaches using APF as a probe, such as epifluorescence microscopy or flow cytometry, may be useful in identifying the components of the plankton that are responsible for HPO activity.
Between 19 and 51% of the BrPO activity in the seawater samples occurred in the <10 μm size fractions and was generally evenly split between the 10.0 – 2.0 μm and 2.0 – 0.2 μm fractions (Figure 5D). There is limited evidence that the non-diatom microalgae that generally contribute to the 10.0 – 2.0 μm size fraction, possess haloperoxidase activity. Peroxidase activity capable of oxidizing iodide has been observed in a red microalga Porphyridium purpureum but no haloperoxidase activity was observed in the marine chlorophyte Dunaliella tertiolecta (Murphy et al., 2000) or in the haptophyte, Isochrysis sp. (T.ISO) (van Bergeijk et al., 2013). Although diatoms small enough to pass through the 10 μm filter, may have contributed the < 10 μm activity, clearly, a more comprehensive investigation of the distribution of haloperoxidases among microalgal taxa is required.
Both cyanobacteria and heterotrophic bacteria may have contributed to the observed BrPO activity in the smallest size fractions. Synechococcus sp. are present throughout the summer in coastal waters of the Gulf of Maine (Shapiro and Haugen, 1988). Homologous genes to the V-BrPO found in Synechococcus sp. were also observed in several other marine and estuarine cyanobacteria (Johnson et al., 2011) and more recently, a functional V-BrPO was characterized from the symbiotic Acaryochloris marina (Frank et al., 2016) emphasizing the potential widespread occurrence of BrPO in cyanobacteria. It is also possible that heterotrophic bacteria contributed to the observed BrPO activity, with ClPOs and IPOs having been characterized from marine bacteria (Bernhardt et al., 2011; Fournier et al., 2014), although not necessarily among planktonic taxa. Free-living bacteria are most likely to have contributed to the 2.0 – 0.2 μm size fraction but particle-associated cells may also have contributed to BrPO activity in the larger size fractions.
Conclusion
We have demonstrated that the conversion of APF to fluorescein by hROS is the basis of a sensitive and quantitative fluorescent assay for the study of BrPO and potentially, ClPO enzyme activity. Current colorimetric approaches will need to be used for the detection and quantification of IPO activity until a suitable fluorescent assay is developed. Depending on the particular application, several aspects of the APF assay will require further refinement and optimization and will need to strike the balance between obtaining enzyme rates that are informative of processes under natural environmental conditions and physiological states versus information on specific kinetic properties of the enzymes themselves in more purified extracts. The fluorescent basis of the assay potentially allows the application of both fluorescent microscopy and flow cytometric approaches to better understand the occurrence and roles of haloperoxidases among microorganisms. This assay has the potential to be of value in many aspects of haloperoxidase research, including developing an improved understanding of the roles of haloperoxidase enzymes in microbial planktonic communities. Among the key questions to be explored are the physiological role (s) of haloperoxidases in controlling intracellular and extracellular H2O2 concentrations in the ocean and in generating halogenated compounds including climate-active volatile halogenated compounds.
Author Contributions
The assay approach was designed by DW with modifications by SA and KP. KP, AH, AL, and SA developed the assay using standards and laboratory cultures. Natural sample collection and processing was carried out by JD, EC, and KP. Manuscript writing was carried out by SA with input from all other authors.
Funding
JD and AH were supported by Bigelow Laboratory’s REU program funded by the National Science Foundation (#1460861). SA acknowledges funding from the Davis Family Foundation.
Conflict of Interest Statement
The authors declare that the research was conducted in the absence of any commercial or financial relationships that could be construed as a potential conflict of interest.
Abbreviations
APF, aminophenyl fluorescein; Br-MCD, bromochlorodimenone; BrPO, bromoperoxidase enzyme; Chl a, chlorophyll a; ClPO, chloroperoxidase enzyme; HPO, haloperoxidase enzyme; hROS, highly reactive oxygen species; IPO, iodoperoxidase enzyme; MCD, monochlorodimenone; MES, 2-(N-morpholino) ethanesulfonic acid; TB, thymolsulfonphthalein; V-BrPO, vanadium-bromoperoxidase; V-IPO, vanadium-iodoperoxidase.
References
Bernhardt, P., Okino, T., Winter, J. M., Miyanaga, A., and Moore, B. S. (2011). A stereoselective vanadium-dependent chloroperoxidase in bacterial antibiotic biosynthesis. J. Am. Chem. Soc. 133, 4268–4270. doi: 10.1021/ja201088k
Butler, A., and Carter-Franklin, J. N. (2004). The role of vanadium bromoperoxidase in the biosynthesis of halogenated marine natural products. Nat. Prod. Rep. 21, 180–188. doi: 10.1039/b302337k
Butler, A., and Walker, J. V. (1993). Marine haloperoxidases. Chem. Rev. 93, 1937–1944. doi: 10.1021/cr00021a014
Carpenter, L. J., Archer, S. D., and Beale, R. (2012). Ocean-atmosphere trace gas exchange. Chem. Soc. Rev. 41, 6473–6506. doi: 10.1039/c2cs35121h
Carter, J. N., Beatty, K. E., Simpson, M. T., and Butler, A. (2002). Reactivity of recombinant and mutant vanadium bromoperoxidase from the red alga Corallina officinalis. J. Inorg. Biochem. 91, 59–69. doi: 10.1016/S0162-0134(02)00400-2
De Boer, E., Plat, H., Tromp, M. G. M., Wever, R., Franssen, M. C. R., Van der Plas, H. C., et al. (1987). Vanadium containing bromoperoxidase: an example of an oxidoreductase with high operational stability in aqueous and organic media. Biotechnol. Bioeng. 30, 607–610. doi: 10.1002/bit.260300504
De Boer, E., Van Kooyk, Y., Tromp, M. G. M., Plat, H., and Wever, R. (1986). Bromoperoxidase from Ascophyllum nodosum: a novel class of enzymes containing vanadium as a prosthetic group? Biochim. Biophys. Acta BBA 869, 48–53. doi: 10.1016/0167-4838(86)90308-0
Dynowski, M., Schaaf, G., Loque, D., Moran, O., and Ludewig, U. (2008). Plant plasma membrane water channels conduct the signalling molecule H2O2. Biochem. J. 414, 53–61. doi: 10.1042/BJ20080287
Enge, S., Nylund, G. M., Harder, T., and Pavia, H. (2012). An exotic chemical weapon explains low herbivore damage in an invasive alga. Ecology. 93, 2736–2745. doi: 10.1890/12-0143.1
Everett, R. R., Kanofsky, J. R., and Butler, A. (1990). Mechanistic investigations of the novel non-heme vanadium bromoperoxidases. Evidence for singlet oxygen production. J. Biol. Chem. 265, 4908–4914.
Flemmig, J., Zschaler, J., Remmler, J., and Arnhold, J. (2012). The fluorescein-derived dye aminophenyl fluorescein is a suitable tool to detect hypobromous acid (HOBr)-producing activity in eosinophils. J. Biol. Chem. 287, 27913–27923. doi: 10.1074/jbc.M112.364299
Fournier, J.-B., Rebuffet, E., Delage, L., Grijol, R., Meslet-Cladière, L., Rzonca, J., et al. (2014). The bacterial vanadium iodoperoxidase from the marine flavobacteriaceae Zobellia galactanivorans reveals novel molecular and evolutionary features of halide specificity in this enzyme family. Appl. Environ. Microbiol. 80, 7561–7573. doi: 10.1128/AEM.02430-14
Frank, A., Seel, C. J., Groll, M., and Gulder, T. (2016). Characterization of a cyanobacterial haloperoxidase and evaluation of its biocatalytic halogenation potential. Chem. BioChem. 17, 2028–2032. doi: 10.1002/cbic.201600417
Guillard, R. R. L., and Hargraves, P. E. (1993). Stichochrysis immobilis is a diatom, not a chrysophyte. Phycologia 32, 234–236. doi: 10.2216/i0031-8884-32-3-234.1
Hewson, W. D., and Hager, L. P. (1980). Bromoperoxidases and halogenated lipids in marine algae. J. Phycol. 16, 340–345. doi: 10.1111/j.1529-8817.1980.tb03043.x
Hill, V. L., and Manley, S. L. (2009). Release of reactive bromine and iodine from diatoms and its possible role in halogen transfer in polar and tropical oceans. Limnol. Oceanogr. 54, 812–822. doi: 10.4319/lo.2009.54.3.0812
Hillebrand, H., Dürselen, C.-D., Kirschtel, D., Pollingher, U., and Zohary, T. (1999). Biovolume calculation for pelagic and benthic microalgae. J. Phycol. 35, 403–424. doi: 10.1046/j.1529-8817.1999.3520403.x
Hughes, C., and Sun, S. (2016). Light and brominating activity in two species of marine diatom. Mar. Chem. 181, 1–9. doi: 10.1016/j.marchem.2016.02.003
Itoh, N., Izumi, Y., and Yamada, H. (1986). Characterization of nonheme type bromoperoxidase in Corallina pilulifera. J. Biol. Chem. 261, 5194–5200.
Johnson, T. L., Palenik, B., and Brahamsha, B. (2011). Characterization of a functional vanadium-dependent bromoperoxidase in the marine cyanobacterium Synechococcus sp. CC9311 1. J. Phycol. 47, 792–801. doi: 10.1111/j.1529-8817.2011.01007.x
Krenn, B. E., Tromp, M. G., and Wever, R. (1989). The brown alga Ascophyllum nodosum contains two different vanadium bromoperoxidases. J. Biol. Chem. 264, 19287–19292.
La Barre, S., Potin, P., Leblanc, C., and Delage, L. (2010). The halogenated metabolism of brown algae (Phaeophyta), its biological importance and its environmental significance. Mar. Drugs 8, 988–1010. doi: 10.3390/md8040988
Leblanc, C., Vilter, H., Fournier, J.-B., Delage, L., Potin, P., Rebuffet, E., et al. (2015). Vanadium haloperoxidases: from the discovery 30 years ago to X-ray crystallographic and V K-edge absorption spectroscopic studies. Coord. Chem. Rev. 301, 134–146. doi: 10.1016/j.ccr.2015.02.013
Littlechild, J. (1999). Haloperoxidases and their role in biotransformation reactions. Curr. Opin. Chem. Biol. 3, 28–34. doi: 10.1016/S1367-5931(99)80006-4
Moore, R. M., Webb, M., Tokarczyk, R., and Wever, R. (1996). Bromoperoxidase and iodoperoxidase enzymes and production of halogenated methanes in marine diatom cultures. J. Geophys. Res. Oceans 101, 20899–20908. doi: 10.1029/96JC01248
Morris, J. J., Johnson, Z. I., Wilhelm, S. W., and Zinser, E. R. (2016). Diel regulation of hydrogen peroxide defenses by open ocean microbial communities. J. Plankton Res. 38, 1103–1114. doi: 10.1093/plankt/fbw016
Murphy, C. D., Moore, R. M., and White, R. L. (2000). Peroxidases from marine microalgae. J. Appl. Phycol. 12, 507–513. doi: 10.1023/A:1008154231462
Nylund, G. M., Cervin, G., Persson, F., Hermansson, M., Steinberg, P. D., and Pavia, H. (2008). Seaweed defence against bacteria: a poly-brominated 2-heptanone from the red alga Bonnemaisonia hamifera inhibits bacterial colonisation. Mar. Ecol. Prog. Ser. 369, 39–50. doi: 10.3354/meps07577
Ortiz de Montellano, P. R., David, S. K., Ator, M. A., and Tew, D. (1988). Mechanism-based inactivation of horseradish peroxidase by sodium azide. Formation of meso-azidoprotoporphyrin IX. Biochemistry. 27, 5470–5476. doi: 10.1021/bi00415a013
Roe, K. L., Schneider, R. J., Hansel, C. M., and Voelker, B. M. (2016). Measurement of dark, particle-generated superoxide and hydrogen peroxide production and decay in the subtropical and temperate North Pacific Ocean. Deep Sea Res. Pt. I 107, 59–69. doi: 10.1016/j.dsr.2015.10.012
Setsukinai, K., Urano, Y., Kakinuma, K., Majima, H. J., and Nagano, T. (2003). Development of novel fluorescence probes that can reliably detect reactive oxygen species and distinguish specific species. J. Biol. Chem. 278, 3170–3175. doi: 10.1074/jbc.M209264200
Shapiro, L. P., and Haugen, E. M. (1988). Seasonal distribution and temperature tolerance of Synechococcus in Boothbay Harbor, Maine. Estuar. Coast. Shelf Sci. 26, 517–525. doi: 10.1016/0272-7714(88)90004-2
Sheffield, D. J., Harry, T., Smith, A. J., and Rogers, L. J. (1992). Purification and characterization of the vanadium bromoperoxidase from the macroalga Corallina officinalis. Phytochemistry 32, 21–26. doi: 10.1016/0031-9422(92)80099-Z
Syrpas, M., Ruysbergh, E., Blommaert, L., Vanelslander, B., Sabbe, K., Vyverman, W., et al. (2014). Haloperoxidase mediated quorum quenching by Nitzschia cf pellucida: study of the metabolization of N-acyl homoserine lactones by a benthic diatom. Mar. Drugs 12, 352–367. doi: 10.3390/md12010352
van Bergeijk, S. A., Hernández Javier, L., Heyland, A., Manchado, M., and Pedro Cañavate, J. (2013). Uptake of iodide in the marine haptophyte Isochrysis sp.(T. ISO) driven by iodide oxidation. J. Phycol. 49, 640–647. doi: 10.1111/jpy.12073
Vanelslander, B., Paul, C., Grueneberg, J., Prince, E. K., Gillard, J., Sabbe, K., et al. (2012). Daily bursts of biogenic cyanogen bromide (BrCN) control biofilm formation around a marine benthic diatom. Proc. Natl. Acad. Sci. 109, 2412–2417. doi: 10.1073/pnas.1108062109
Verhaeghe, E., Buisson, D., Zekri, E., Leblanc, C., Potin, P., and Ambroise, Y. (2008). A colorimetric assay for steady-state analyses of iodo-and bromoperoxidase activities. Anal. Biochem. 379, 60–65. doi: 10.1016/j.ab.2008.04.041
Vermilyea, A. W., Paul Hansard, S., and Voelker, B. M. (2010). Dark production of hydrogen peroxide in the Gulf of Alaska. Limnol. Oceanogr. 55, 580–588. doi: 10.4319/lo.2010.55.2.0580
Wever, R., and van der Horst, M. A. (2013). The role of vanadium haloperoxidases in the formation of volatile brominated compounds and their impact on the environment. Dalton Trans. 42, 11778–11786. doi: 10.1039/c3dt50525a
Winter, J. M., Moffitt, M. C., Zazopoulos, E., McAlpine, J. B., Dorrestein, P. C., and Moore, B. S. (2007). Molecular basis for chloronium-mediated meroterpene cyclization: cloning, sequencing, and heterologous expression of the napyradiomycin biosynthetic gene cluster. J. Biol. Chem. 282, 16362–16368. doi: 10.1074/jbc.M611046200
Yamada, H., Itoh, N., Murakami, S., and Izumi, Y. (1985). New bromoperoxidase from coralline algae that brominates phenol compounds. Agric. Biol. Chem. 49, 2961–2967.
Yentsch, C. S., and Menzel, D. W. (1963). A method for the determination of phytoplankton chlorophyll and phaeophytin by fluorescence. Deep Sea Res. Oceanogr. Abstracts 10, 221–231. doi: 10.1016/0011-7471(63)90358-9
Keywords: haloperoxidase, bromoperoxidase, microalgae, diatom, fluorescent assay, enzyme activity
Citation: Archer SD, Posman KM, DeStefano J, Harrison AO, Ladina A, Cheff EA and Witt DP (2019) Fluorescent Detection of Bromoperoxidase Activity in Microalgae and Planktonic Microbial Communities Using Aminophenyl Fluorescein. Front. Mar. Sci. 6:68. doi: 10.3389/fmars.2019.00068
Received: 10 August 2018; Accepted: 06 February 2019;
Published: 20 February 2019.
Edited by:
Fabiano Thompson, Instituto Alberto Luiz Coimbra de Pós-Graduação e Pesquisa de Engenharia (COPPE), BrazilReviewed by:
Arthur W. Silva Lima, Universidade Federal do Rio de Janeiro, BrazilCristiane Carneiro Thompson, Universidade Federal do Rio de Janeiro, Brazil
Copyright © 2019 Archer, Posman, DeStefano, Harrison, Ladina, Cheff and Witt. This is an open-access article distributed under the terms of the Creative Commons Attribution License (CC BY). The use, distribution or reproduction in other forums is permitted, provided the original author(s) and the copyright owner(s) are credited and that the original publication in this journal is cited, in accordance with accepted academic practice. No use, distribution or reproduction is permitted which does not comply with these terms.
*Correspondence: Stephen D. Archer, sarcher@bigelow.org