- Seascape Ecology Lab, Department of Oceanography and Coastal Sciences, Louisiana State University, Baton Rouge, LA, United States
While coral larval exchange among reef patches is crucial to the persistence of coral metapopulations, larval retention within patches is critical for local population maintenance. In isolated systems such as the Flower Garden Banks (FGB) of the northwest Gulf of Mexico (NW GoM), local retention is thought to play an important role in maintaining high levels of coral cover. Numerous mesoscale cyclonic and anticyclonic features (eddies) are known to spin off from the GoM’s Loop Current, many of which pass over the FGB. We developed a biophysical model of coral larval dispersal (2004–2018) to investigate the extent to which eddies may facilitate coral larval exchange between and within the east and west FGB. Virtual larvae of the broadcast spawning Orbicella faveolata and the brooding Porites astreoides were released and tracked with species-specific reproductive and larval behaviors to investigate differences in retention and connectivity in corals with contrasting life histories. Eddies were detected and tracked using sea surface altimetry and compared with larval trajectories to assess the retentive characteristics of these features. Results suggest consistently high, but species-specific, levels of local retention and cross-bank connectivity in both coral species. High local retention is possible early in the dispersal of P. astreoides, and both species routinely experience retention due to recirculation in eddy features as late as 30 days after planulation or spawning. Eddies passing over the FGB were associated with pulses of between- and within-bank retention, indicating that larvae are capable of dispersing from and returning to coral reefs in the NW GoM. Although opportunities for retention are inherently ephemeral and stochastic due to the nature of Loop Current Eddy (LCE) shedding, eddy propagation should serve as a reliable reseeding mechanism for FGB coral populations. In particular, peaks in late summer eddy propagation correspond with mass coral spawning and may enhance larval retention. These findings support the assertions that healthy FGB reefs may be largely self-sustaining, and that persistent, self-sustaining populations at the FGB may supply downstream reefs with larvae and behave as a remote climate change refugium.
Introduction
The Flower Garden Banks (FGB) is an isolated reef system located in the northwest Gulf of Mexico (NW GoM) with two primary submerged coral reefs between 16 and 150 m in depth (Johnston et al., 2019). Other than a localized mortality event in 2016 (Johnston et al., 2019), these reefs have sustained high levels of coral cover (>50%) over the last several decades (Schmahl et al., 2008; Johnston et al., 2016). Researchers attribute their health to their depth, which provides thermal refuge (Muir et al., 2017), and their distance from shore, which provides a buffer from a variety of coastal, anthropogenic stressors (McLaughlin et al., 2003; Smith et al., 2008). Despite facing low levels of disturbance, the FGB require sufficient levels of recruitment to remain persistent. With the nearest reefs located over 600 km away along the Yucatan peninsula, it is thought that the FGB are largely self-sustaining (Gittings et al., 1992; Brazeau et al., 2005; Sammarco et al., 2012). Coral larvae produced at the FGB are subject to highly variable flow, that is thought to be largely dispersive to early life-stage propagules (e.g., Lugo-Fernández et al., 2001). Here we investigate the biophysical conditions that facilitate local retention at the FGB in two coral species.
Eddies—mesoscale cyclonic or anticyclonic features—regularly spin off from the GoM’s Loop Current (Vukovich, 2012; Lindo-Atichati et al., 2013). The Loop Current is a prominent GoM current, forced by warm water entering the GoM between the Yucatan Peninsula and Cuba (Oey et al., 2005) (Figure 1). It can intrude as far north as the Mississippi River Delta and as far east as the Florida continental shelf before feeding into the Florida Current (Alvera-Azcárate et al., 2008). FGB coral larvae may be entrained in Loop Current Eddies (LCEs) when they pass over or near the FGB, which may enhance local settlement. Here we utilize a biophysical model of coral larval dispersal to investigate FGB coral larval retention in relation to the spatiotemporal propagation and persistence of eddies in the NW GoM. We also examine the influence of contrasting life histories and reproductive seasonality on local retention and between-bank connectivity across 15 years of hydrodynamic forcing (2004–2018).
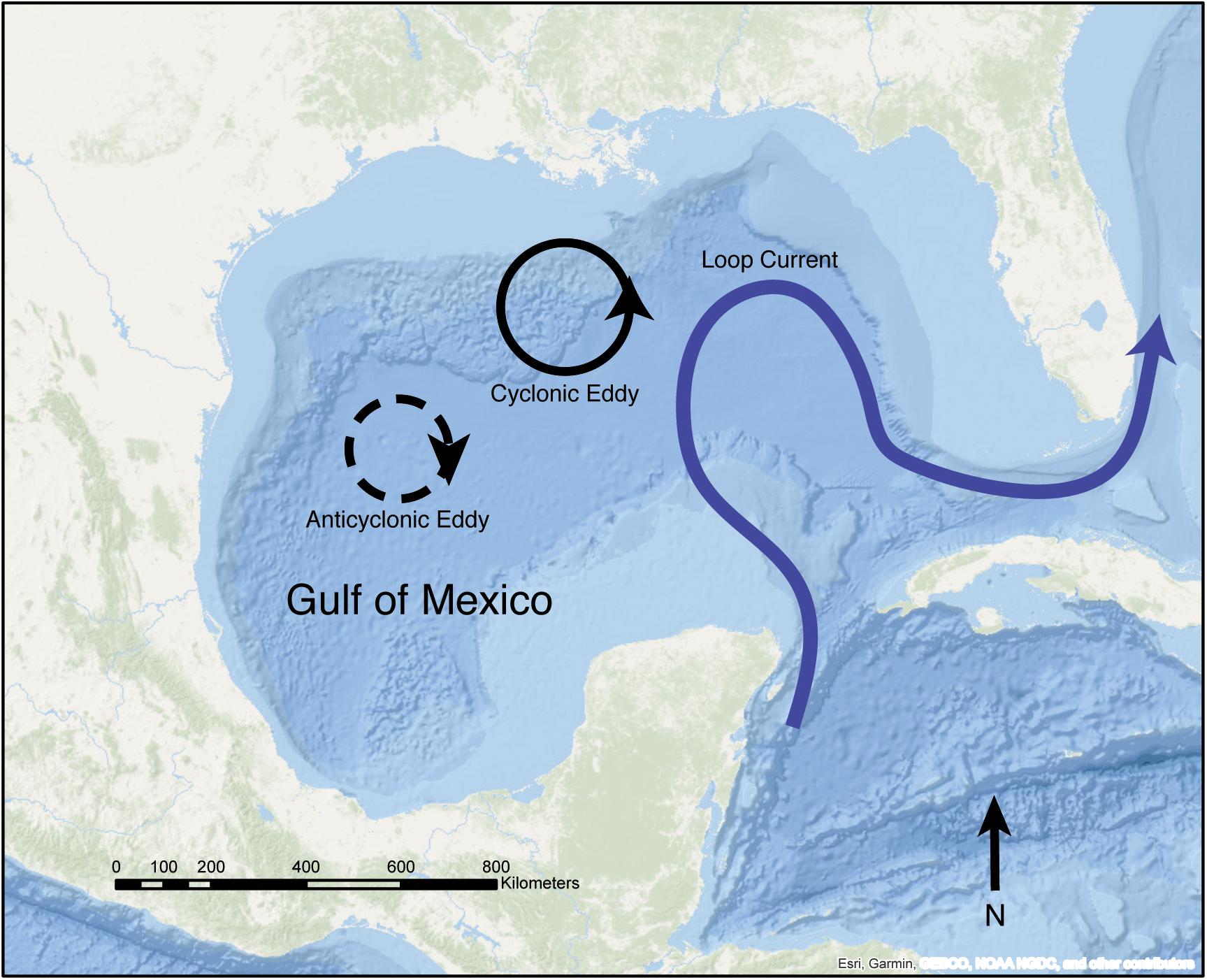
Figure 1. Schematic of the GoM showing the Loop Current (blue) the FGB, and cyclonic and anticyclonic LCEs. The FGB are located on the Texas-Louisiana continental shelf in the NW GoM (Esri, 2019).
The reef caps at the FGB are some of the few Caribbean reef systems still dominated by large, boulder and brain coral colonies (Schmahl et al., 2008; Clark et al., 2014). These include slow-growing, broadcast spawning species such as Montastraea cavernosa, Pseudodiploria strigosa, and the Orbicella species complex. The FGB National Marine Sanctuary (FGBNMS) monitors the mass spawning events at these reefs, which occur annually in the weeks following late summer full moons (Vize et al., 2005, 2008; Schmahl et al., 2008). Broadcast spawning corals will simultaneously release gametes, which fertilize in the water column or near the sea surface. Most larvae of broadcast spawning species are competent to settle in 3–6 days, and some species’ larvae are viable for as long as 4 months (Harrison and Wallace, 1990; Graham et al., 2008; Porto-Hannes et al., 2015). Estimations for the maximum pelagic larval duration (PLD) for orbicellids ranges from 10 to 120 days (Porto-Hannes et al., 2015; Davies et al., 2017). The FGB also support populations of brooding corals including Porites spp. and Agaricia spp. Brooders tend to have an extended reproductive season, and Porites astreoides populations release planula larvae throughout late spring and summer (April–September), with peaks centered on new moons (Chornesky and Peters, 1987; McGuire, 1998; Edmunds et al., 2001). These weedy, opportunistic corals experience higher recruitment rates throughout the Caribbean than broadcasting species (Green et al., 2008), possibly due to early larval competency and shorter distance dispersal. P. astreoides larvae may settle in as little as 3 h, but the maximum PLD for this species remains unclear (Edmunds et al., 2001; Harii et al., 2002; Olsen et al., 2016). PLD is a crucial parameter influencing the dispersal potential of coral and other planktonic larvae (Shanks, 2009). Typically, longer PLDs are associated with greater dispersal potential, but the increased distance of larval transport may be associated with higher larval mortality and displacement from suitable habitat (Connolly and Baird, 2010; Buston et al., 2012). In this study, we selected P. astreoides as a model for brooding corals, and Orbicella faveolata as a model for broadcasting orbicellids.
Biophysical modeling and satellite drifter studies in the GoM suggest that a long dispersal window is necessary for export from the FGB to distant reefs (Davies et al., 2017; Olascoaga et al., 2018), but retention potential is approximately equal with a short or long PLD (Davies et al., 2017). Recent studies also show M. cavernosa populations are genetically well-mixed between east FGB (EFGB) and west FGB (WFGB) and with the nearby McGrail, Geyer, and Bright Bank populations. These coupled studies utilized an evolutionary genetics framework (Studivan and Voss, 2018a) and local hydrographic conditions over ecological time scales to infer population connectivity (Garavelli et al., 2018). Broadcast coral populations at the FGB may experience recruitment from populations throughout the wider Caribbean, as the FGB O. faveolata population genetic signature shows a relatively equal proportion of genets from extraneous Caribbean populations (Rippe et al., 2017). These studies suggest that there is an apparent lack of barriers to long distance gene flow from distant coral populations to the FGB. Though this body of work has collectively increased our insight into connectivity among the FGB and throughout the GoM, demographic population maintenance is likely driven by local retention. We have, however, little understanding of the biophysical mechanisms that would support this retention, nor of the temporal scale over which they operate.
Due to the isolation and relative health of coral reefs at the FGB, their potential as a climate change refugium is of particular interest (Hickerson et al., 2012; Davies et al., 2017). Western Atlantic reefs are experiencing severe declines due to disease (Precht et al., 2016; Wilson et al., 2016), elevated sea surface temperatures (SST) (LaJeunesse et al., 2015), and ocean acidification (Hughes et al., 2017; Langdon et al., 2018). The availability of larval recruits is thought to limit population recovery at many reefs (Hughes and Tanner, 2000; Williams et al., 2008; Ritson-Williams et al., 2009). FGB reef persistence and potential as a reef refugium depend heavily on the degree to which the FGB are self-sustaining through larval retention. While eddies have been proposed to aid in local retention in the FGB (Lugo-Fernández et al., 2001; Johnson et al., 2017), a systematic understanding of how coral reproductive and larval biology interact with the physical environment to enhance settlement in the FGB remains limited.
This study is the first to investigate the influence of LCEs on FGB coral larval local retention. Our biophysical models show that for two species of reef-building corals, pulses of reproductive output contemporaneous with mesoscale features can recirculate coral larvae over the FGB early in the dispersal window. Smaller pulses of larvae may return to the reefs between 2 weeks to 1 month into the dispersal window. Our models suggest that although inherently stochastic, over sufficient timescales eddies are a consistent and reliable mechanism for coral larval reseeding at the FGB.
Materials and Methods
Study Site
Dispersal was simulated from EFGB and WFGB coral populations. The EFGB and WFGB have been under sanctuary designation since January 1992 (Schmahl et al., 2008). These coral communities are the most prominent and well-studied in the NW GoM region. While Stetson Bank is also included under the protection of the FGBNMS, it has considerably lower coral cover (DeBose et al., 2013) and low abundances of our target species, and thus was not used in the study. The reef caps on EFGB and WFGB support coral from ∼15 to 60 m (Hickerson et al., 2008).
Hydrodynamics
Fifteen years of hydrodynamic forcing were utilized from the HYbrid Coordinate Ocean Model (HYCOM, Bleck, 2002) GoM regional analysis (Chassignet et al., 2007). This hydrodynamic model has a resolution of 1/25° per grid cell (∼3.5 km). The first 5 years of hydrodynamics (2004–2008) and January–April 2009 were obtained from the HYCOM GOMl0.04 experiment 20.1. Hydrodynamics for the remainder of 2009 through April 2014 were obtained from HYCOM GOMl0.04 experiment 30.1, and the remainder of 2014 through 2018 were obtained from HYCOM GOMl0.04 experiment 32.5. Hydrodynamic fields were downloaded as daily NetCDF files each containing: eastward, northward, and vertical velocities; sea surface height (SSH or altimetry); temperature; salinity; and density. Velocities and density fields were utilized in biophysical simulations of the dispersal of P. astreoides and O. faveolata. SSH was used to identify daily eddy fields in the GoM, using “EddyScan,” an automated algorithm for the detection of eddies (Faghmous et al., 2012) (described below).
Larval Dispersal Model
Larvae were tracked within the model domain using the Connectivity Modeling System (CMS), an open-source individual-based Lagrangian particle tracking software (Paris et al., 2013). Virtual larvae (particles) of each species were simulated and tracked in three dimensions throughout their PLD. Larval position was calculated every 10 min and recorded every 3 h. Horizontal and vertical diffusivity coefficients (2.45 and 0.01 m2/s2, respectively) were used to estimate sub-grid dynamics, following the methods of Okubo (1971). Because the reef caps of each bank were contained within a single (separate) grid cell of the hydrodynamic model, one release location on each bank was used for all simulations.
When available, the timing of virtual spawning for O. faveolata was based on FGBNMS field observations (Schmahl et al., 2008; Flower Garden Banks National Marine Sanctuary-Spawning Observations, 2018). For years without field observations, spawning was simulated after the full moon in August and following full moons in late July or early September, when predicted by sanctuary researchers (Supplementary Table S1). Throughout the 15 modeled years (2004–2018), 25 O. faveolata spawning events were simulated. Each simulated spawn occurred over 3 days, 7–9 days after the full moon; 5000 virtual larvae were released per spawning day per bank, for a total of 750,000 tracked virtual O. faveolata larvae throughout the model duration.
Simulated P. astreoides larvae were released every day throughout its reproductive season from April to September. The number of particles released each day from each bank was in proportion to an adjusted moon fraction:
Moon Fraction refers to the percentage of the moon visible to the observer, and values were obtained from the US Naval Observatory (U.S. Naval Observatory, 2017). Peaks in larval releases occurred during new moons, and troughs occurred during full moons (Figure 6A). Approximately 90,000 particles were released from each bank per year, with over 2,700,000 simulated P. astreoides larvae in total.
The buoyancy module of the CMS was implemented to simulate biological processes affecting the depth of larvae throughout dispersal. During dispersal, larvae may change in size, specific gravity, or in vertical swimming behaviors, which can all affect buoyant velocity and vertical position (Szmant and Meadows, 2006). Ontogenetic changes were approximated by simulating species-specific changes in particle size and specific gravity as larvae dispersed. Orbicellids undergo size and specific gravity changes when spawned egg bundles break apart, fertilize, and develop into planulae (Wellington and Fitt, 2003; Szmant and Meadows, 2006). P. astreoides release fully developed larvae (planulae; Chornesky and Peters, 1987), and thus, changes in larval size and specific gravity were modeled during dispersal to simulate the depletion of larval lipid stores and downward swimming behaviors. A sensitivity analysis was performed to determine the appropriate ranges for specific gravity and particle size, and to determine the intervals over which these parameters should change to produce a realistic series of depth-changes throughout the dispersal window (Supplementary Figures S6, S7). Buoyancy tables were modified from Holstein et al. (2015) (Supplementary Table S2).
Virtual O. faveolata larvae were given a pre-competency period of 3 days (Porto-Hannes et al., 2015), but a maximum PLD was set to 120 days to allow the model to represent dispersal for additional broadcast coral species (Graham et al., 2008; Davies et al., 2017). P. astreoides larvae were given a pre-competency period of 3 h (Harrison and Wallace, 1990) and a maximum PLD of 30 days (Edmunds et al., 2001; Harii et al., 2002).
Eddy Tracking
EddyScan (Faghmous et al., 2012) was implemented to obtain tracks and contours for all eddies in the GoM detected from 2004 to 2018. The MATLAB algorithm implemented an iterative-thresholding approach to identify connected component features based on SSH. The features were then filtered based on a minimum feature size of four pixels. EddyScan generated a series of pixels corresponding to the contours of all eddies for each day of the year, as well as tracks for eddy centroids and the direction of spin (cyclonic or anticyclonic). The current software implements a parameter-free approach, preventing eddies from being unnecessarily discarded, an advantage over previous detection schemes. Some limitations include a higher misidentification rate for spin direction in eddies with shorter lifetimes, as well as an overestimation of eddy size (Faghmous et al., 2015). Based on the resolution of the hydrodynamic models, the minimum size of eddies detected is on the order of tens of kilometers, daily. The short timescale over which larval settlement occurs demands this daily, fine-scale approach. To investigate potential interactions between the larval trajectories and the eddies, the latitude and longitude of the eddy contours were extracted. This allowed for a record of monthly variation in the number of eddies passing over or near the FGB. For each month in each year, we calculated “Eddy Days,” a metric we define as the cumulative number of eddies intersecting a 25 km buffer of the FGBNMS boundaries per day in a given month. For example, a single eddy interacting with the FGB for 3 days would contribute 3 Eddy Days, as would three separate eddies over 1 day. The number of cyclonic and anticyclonic Eddy Days experienced by the FGB were further summarized using Poisson general additive models (GAMs) with cyclic cubic regression splines to describe long-term interactions of LCEs with the FGB (Figure 2).
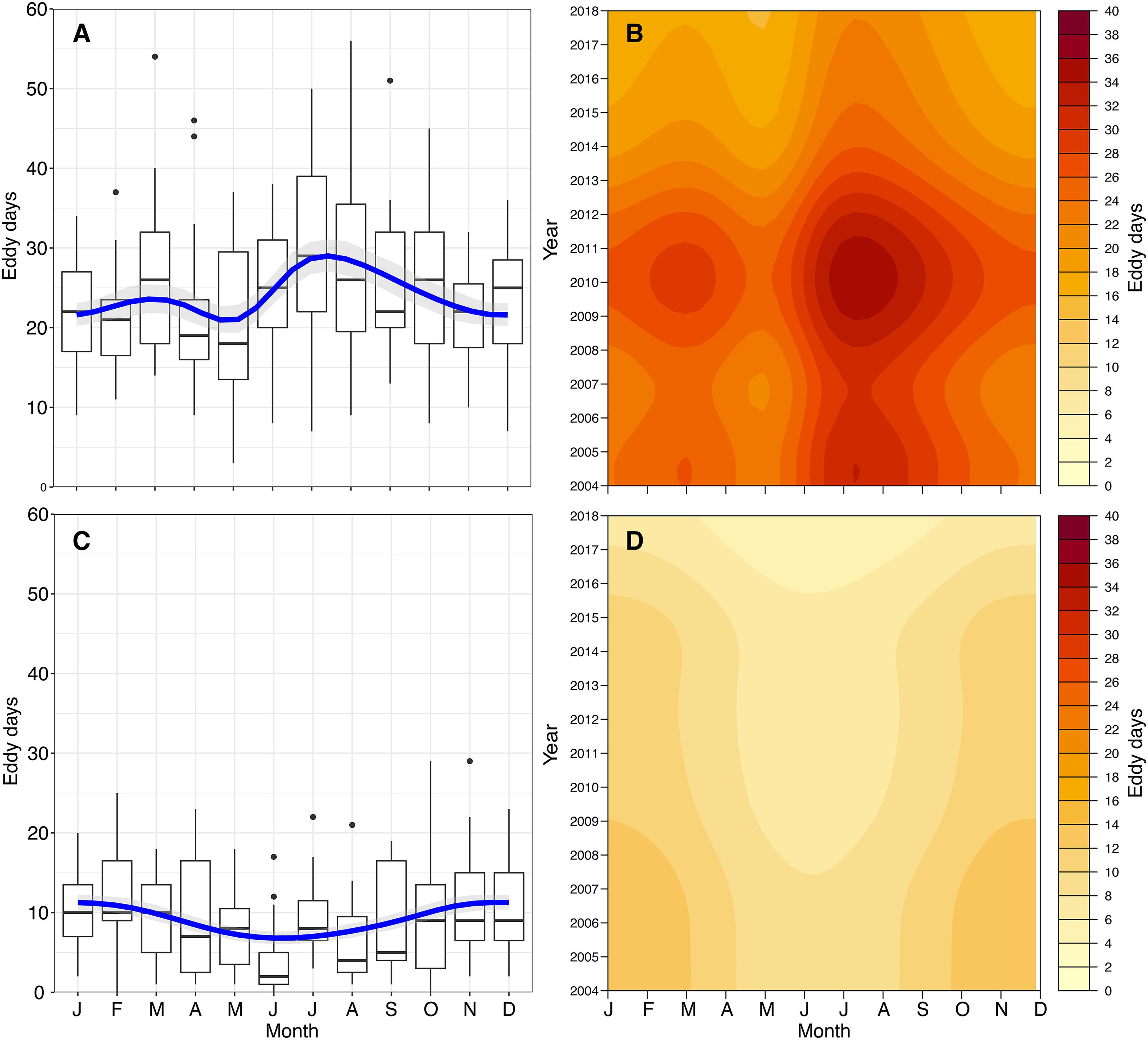
Figure 2. Interactions of mesoscale features with the FGB. Interactions are described as Eddy Days, and shown by eddy spin direction (cyclonic, A,B; anticyclonic, C,D). Monthly summaries are shown in boxplots (A,C) with Poisson GAM predictions with cyclic cubic regression splines. GAM predictions across year and month are shown as heat maps (B,D) to demonstrate broad trends in the physical environment of the FGB.
Biophysical and Retention Analysis
In order to relate this simulation study to empirical studies of larval–mesoscale structure interactions (e.g., Lindo-Atichati et al., 2012) and to understand the interactions of simulated larval dispersal with temporally and spatially dynamic mesoscale oceanographic features, the number of simulated larvae entrained within any cyclonic or anticyclonic eddy within the model domain was assessed over every day of dispersal throughout the 15-year simulation. Note that virtual larval dispersal may have been influenced by an eddy without being in the eddy core.
A binary matrix was used to determine the presence or absence of every particle within the sanctuary boundaries of both banks every 3 h. Five modes of retention were then assessed: between bank retention (or connectivity) (1) from EFGB to WFGB, and (2) from WFGB to EFGB; within-bank local retention (3) at EFGB and (4) at WFGB; and (5) total FGB retention, which sums all modes of retention (1–4) (Table 1). Every intersection within the sanctuary boundary was considered to be an opportunity for potential settlement, but a larva’s trajectory did not end after making a potential connection. Thus, the potential for multiple settlement opportunities and settlement opportunities late in dispersal were assessed. The total number of connections for each mode was determined throughout the dispersal window, as was the percentage of particles with at least one (or multiple) connection(s) (Table 2). Because the potential for retention and connectivity were being assessed, larval mortality was not considered.
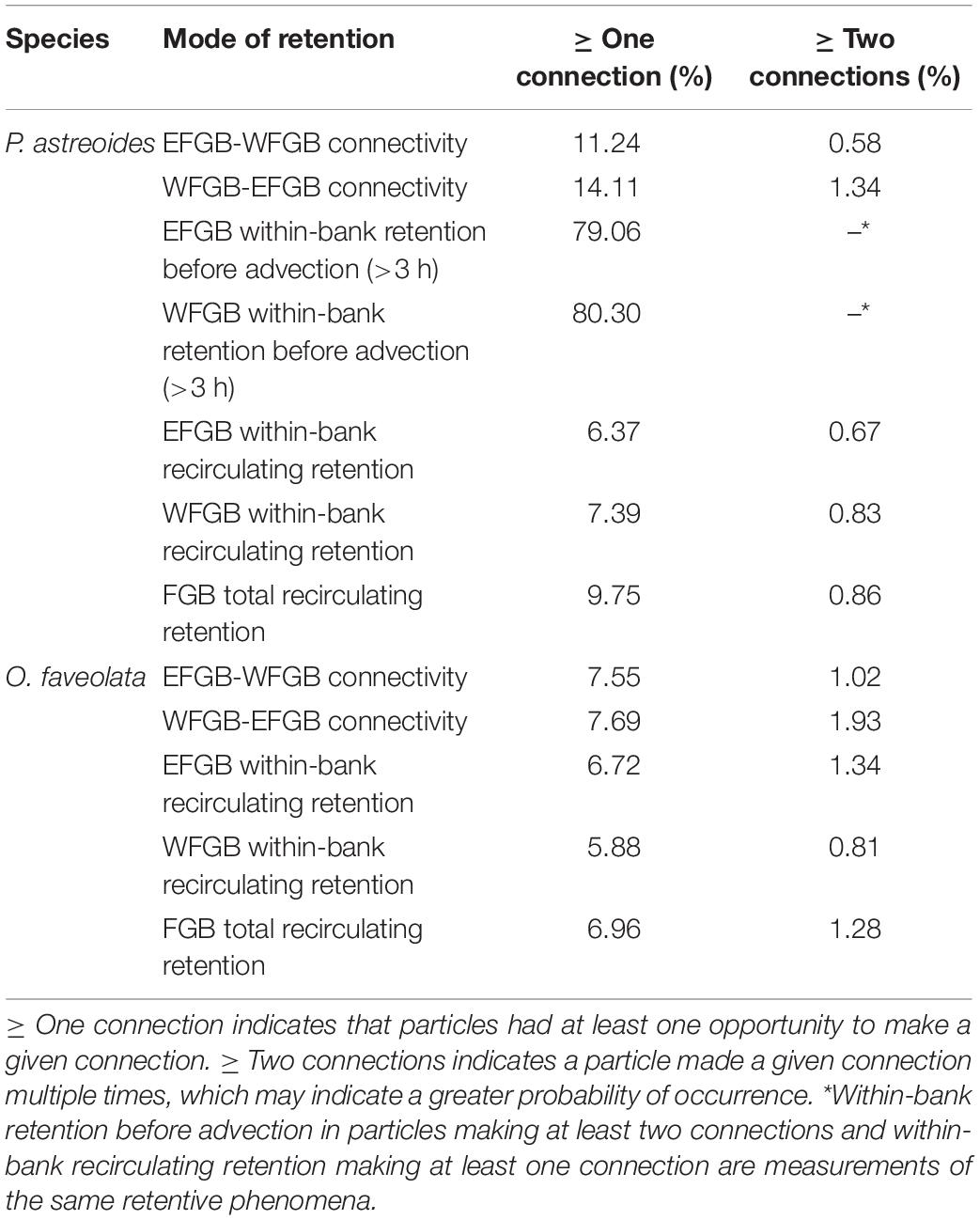
Table 2. Percentage of all larvae released making at least one or multiple connections in different modes and directions.
Results
Virtual larvae of both species rapidly dispersed away from the FGB, and the trajectories they followed varied greatly both intra- and inter-annually. Particles were often entrained within the Loop Current to the east, and, in the case of O. faveolata, were at times advected through the Florida Strait and entrained further into the Gulf Stream. However, variable directional flow and stochastic eddy features resulted in advection of virtual larvae to the north, west, and south of FGB, both over open ocean and over the continental shelf of the northern and western GoM.
Eddies and Larval Trajectories
Between 80 and 120 eddies were detected per day throughout the GoM, many of which occurred near the FGB during the 15 modeled years. Over this time span, the number of FGB cyclonic Eddy Days was significantly elevated during the late summer months (Poisson GAM with cyclic cubic regression spline, deviance explained = 22.9%, p < 0.0001; Figures 2A,B). Anticyclonic Eddy Days showed the opposite trend, with fewer late summer anticyclonic eddy–FGB interactions (Poisson GAM with cyclic cubic regression spline, deviance explained = 14%, p < 0.0001; Figures 2C,D). In both cases, there was a significant effect of year (p < 0.0001), indicating considerable interannual variation in mesoscale features over the FGB. It is important to note that the GAM models smooth intra-annual variation (Figures 2B,D), which was high (Supplementary Figures S1, S2). For example, the GAM predicts highest cyclonic Eddy Days in late summer of 2010; while there were peaks in July and September of 2010, August had considerably fewer cyclonic Eddy Days (Supplementary Figure S1).
When summarized for all years, the percentage of P. astreoides virtual larvae entrained within cyclonic eddies remained relatively consistent, with daily medians consistently ranging between 2 and 15% (Figure 3). In late summer, particularly late August and September, a significantly greater percentage of P. astreoides larvae were entrained in cyclonic eddies than in other months (Kruskal–Wallis rank sum test, p < 0.0001; and pairwise Wilcoxen rank sum tests with Benjamini and Hochberg adjusted p-values). These trends were not seen in virtual P. astreoides larvae entrained in anticyclonic eddies (Supplementary Figure S3). The percentage of O. faveolata virtual larvae entrained in cyclonic eddies also significantly peaked in late summer (Figure 4), with daily medians ranging as high as 13% (Kruskal–Wallis rank sum test, p < 0.0001; and pairwise Wilcoxen rank sum tests with Benjamini and Hochberg adjusted p-values). This trend was not observed for anticyclonic eddies (Supplementary Figure S4).
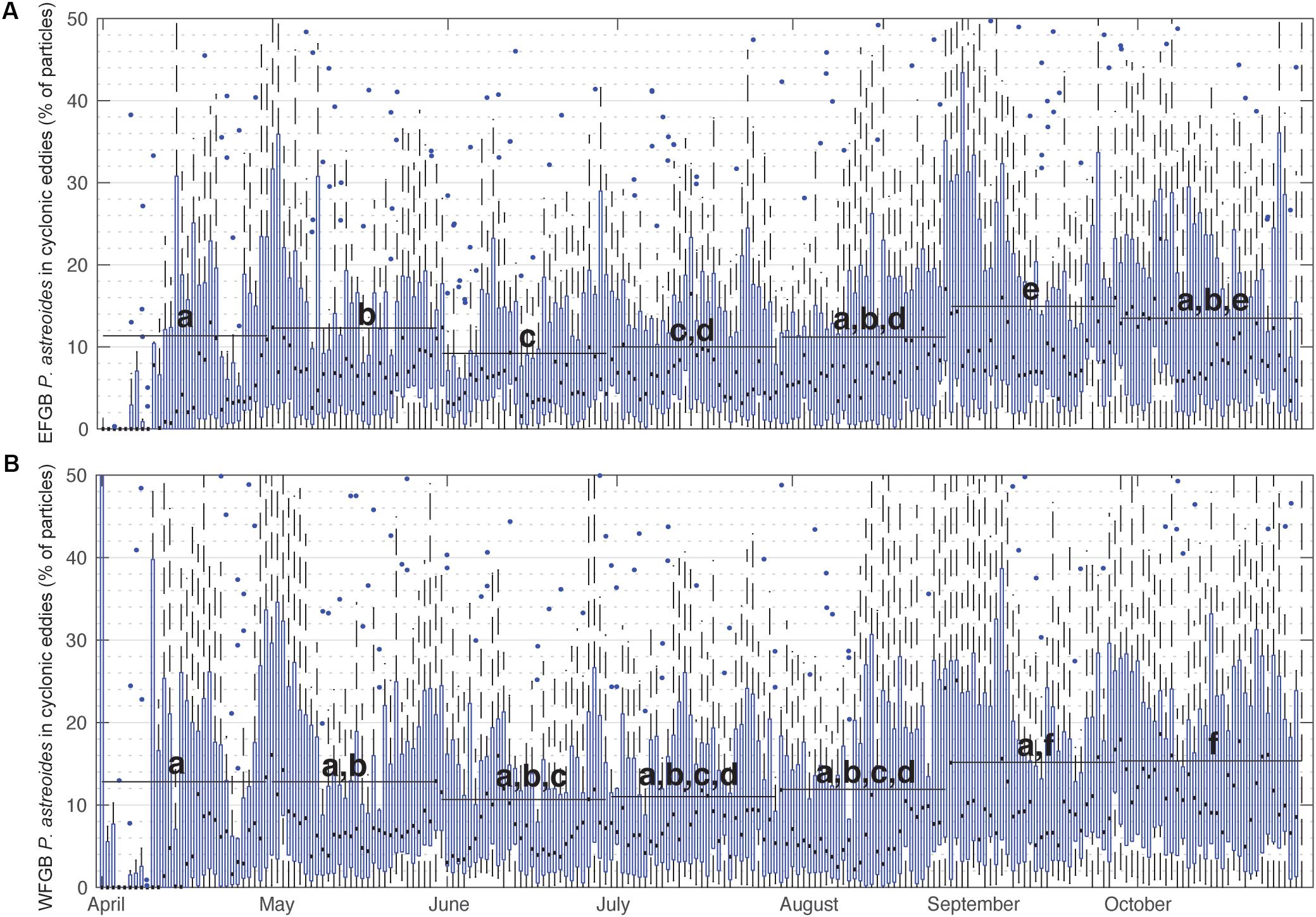
Figure 3. Percentage of P. astreoides virtual larvae entrained in cyclonic eddies over time. Box plots show annual distributions across the 15 years of seasonal planulation (2004–2018) of the percentage of (A) EFGB and (B) WFGB P. astreoides larvae entrained in any GoM cyclonic eddy feature on that day of the year. Boxes are interquartile ranges, black lines are median values, blue circles are outliers, and whiskers represent the first and fourth quartiles. Black bars represent means taken over 30-day windows and letters correspond to significantly different pairwise comparisons (all significant differences p < 0.05, pairwise Wilcoxen tests with Benjamini and Hochberg adjusted p-values).
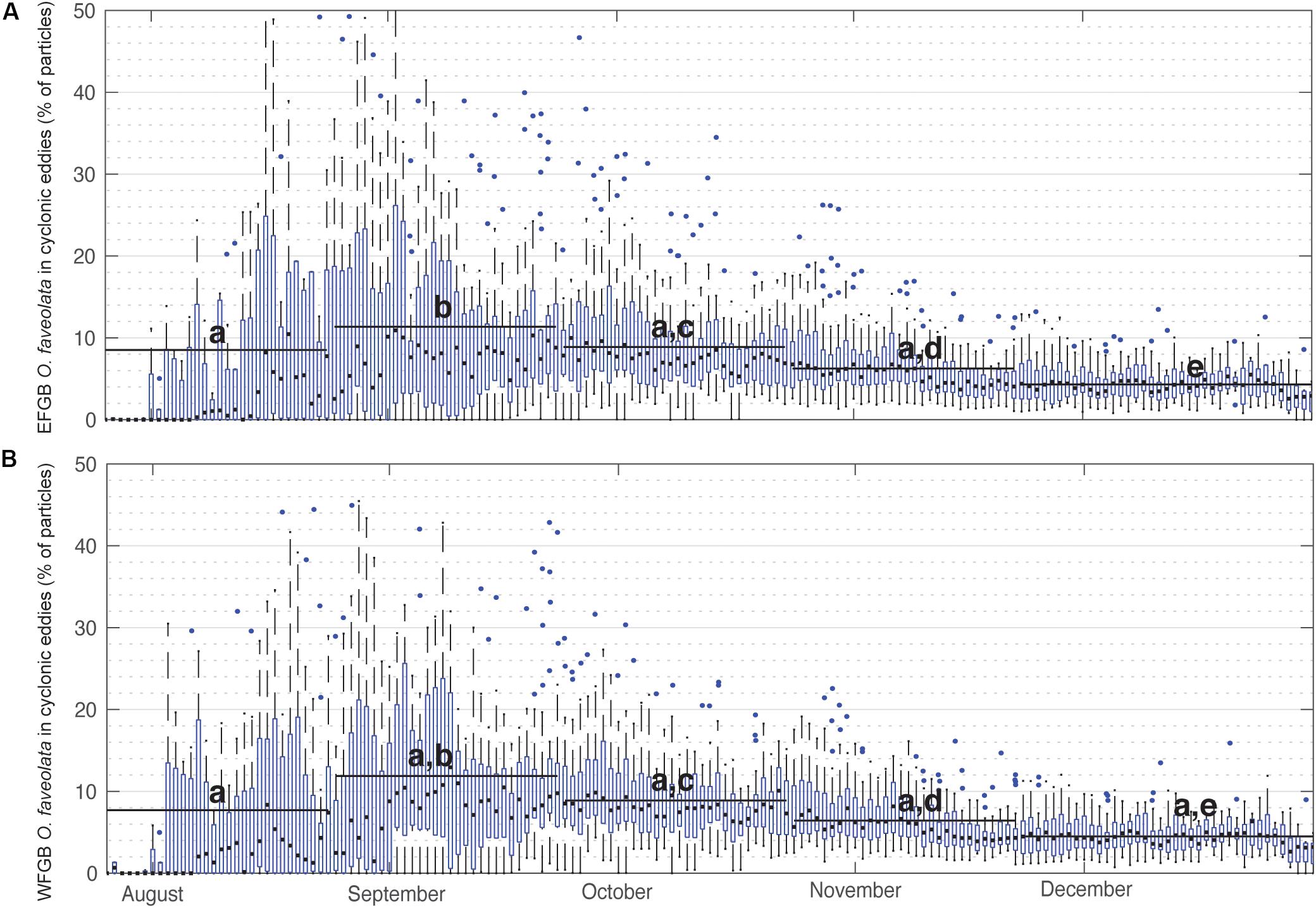
Figure 4. Percentage of O. faveolata virtual larvae entrained in cyclonic eddies over time. Box plots show annual distributions across the 25 modeled spawn events (2004–2018) of the percentage of (A) EFGB and (B) WFGB O. faveolata larvae entrained in any GoM cyclonic eddy feature on that day of the year. Boxes are interquartile ranges, black lines are median values, blue circles are outliers, and whiskers represent the first and fourth quartiles. Black bars represent means taken over 30-day windows and letters correspond to significantly different pairwise comparisons (all significant differences p < 0.05, pairwise Wilcoxen tests with Benjamini and Hochberg adjusted p-values).
Patterns in Simulated Larval Retention at FGB
Porites astreoides
The dispersal of P. astreoides virtual larvae was highly variable throughout its extended reproductive seasons. In extremely rare events (0.004% of planulae), entrainment in the Loop Current advected particles as far as the Florida Keys. High local retention occurred immediately following planulation and prior to larvae advecting from the sanctuary boundaries over the course of the first ∼3 days of dispersal (pre-advection retention) (Figure 5A); 55–95% of all virtual larvae in a given year were competent to settle before leaving the sanctuary boundaries across all reproductive events. Larvae also recirculated over the FGB frequently, potentially due to entrainment within, as well as advection influenced by LCEs; 9.75% of all virtual P. astreoides larvae left and returned to the FGB at least once (Table 2). Nearly 80% of this recirculating retention occurred after 3 days of dispersal, while ∼20% was due to very brief advection and rapid reintroduction to the sanctuary boundaries due to advective processes, some related to LCEs. Between-bank connectivity peaked 1 day into the dispersal window with an annual median of ∼2.0% and range of 0.5–4.0% of all particles released making between-bank connections in a given year (Figure 5B). Every year, between-bank exchange occurred in under 2.5 days, but connectivity declined precipitously after 1 day of dispersal. Eastward transport was slightly higher as WFGB to EFGB connections made up 56% of all P. astreoides between-bank connections (Table 2). Total retention after 3 days gradually declined over the length of the PLD, falling to a median of <0.05% beyond 2 weeks of dispersal (Figure 5C).
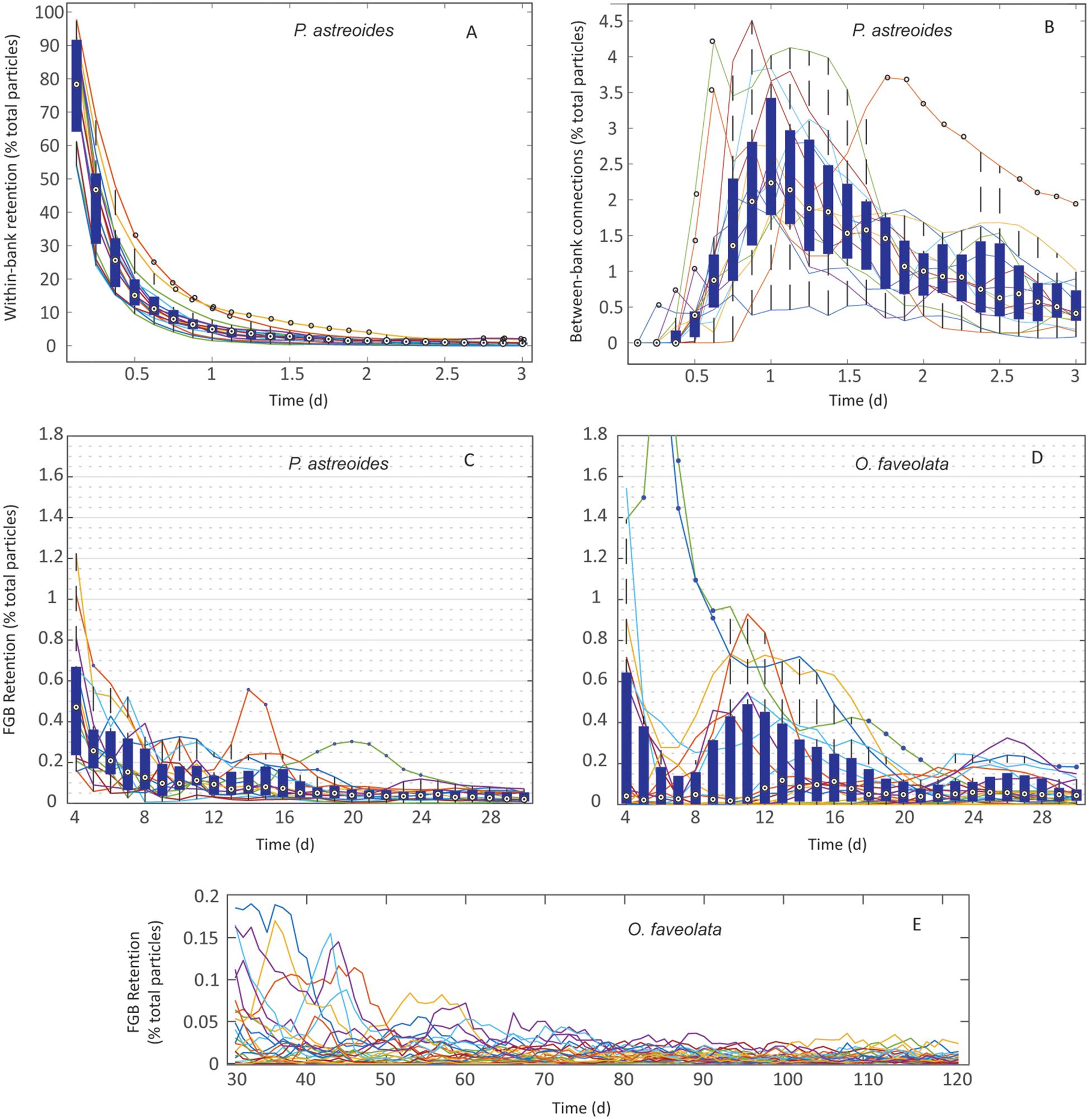
Figure 5. Retention in P. astreoides and O. faveolata at FGB: (A) P. astreoides: 3 h–3 days of within-bank local retention. (B) P. astreoides: 3 h–3 days of between-bank retention. (C) P. astreoides: 3–30 days of dispersal – total FGB retention. (D) O. faveolata: 3–30 days of dispersal – total FGB retention. (E) O. faveolata: 30–120 days of dispersal – total FGB retention. Values represent retention per day as a percentage of total particles released per year. Boxes are interquartile ranges, targets are median values, blue circles are outliers, and whiskers represent the first and fourth quartiles. Lines represent individual years for P. astreoides and individual spawning events for O. faveolata. Note different y-axes scales.
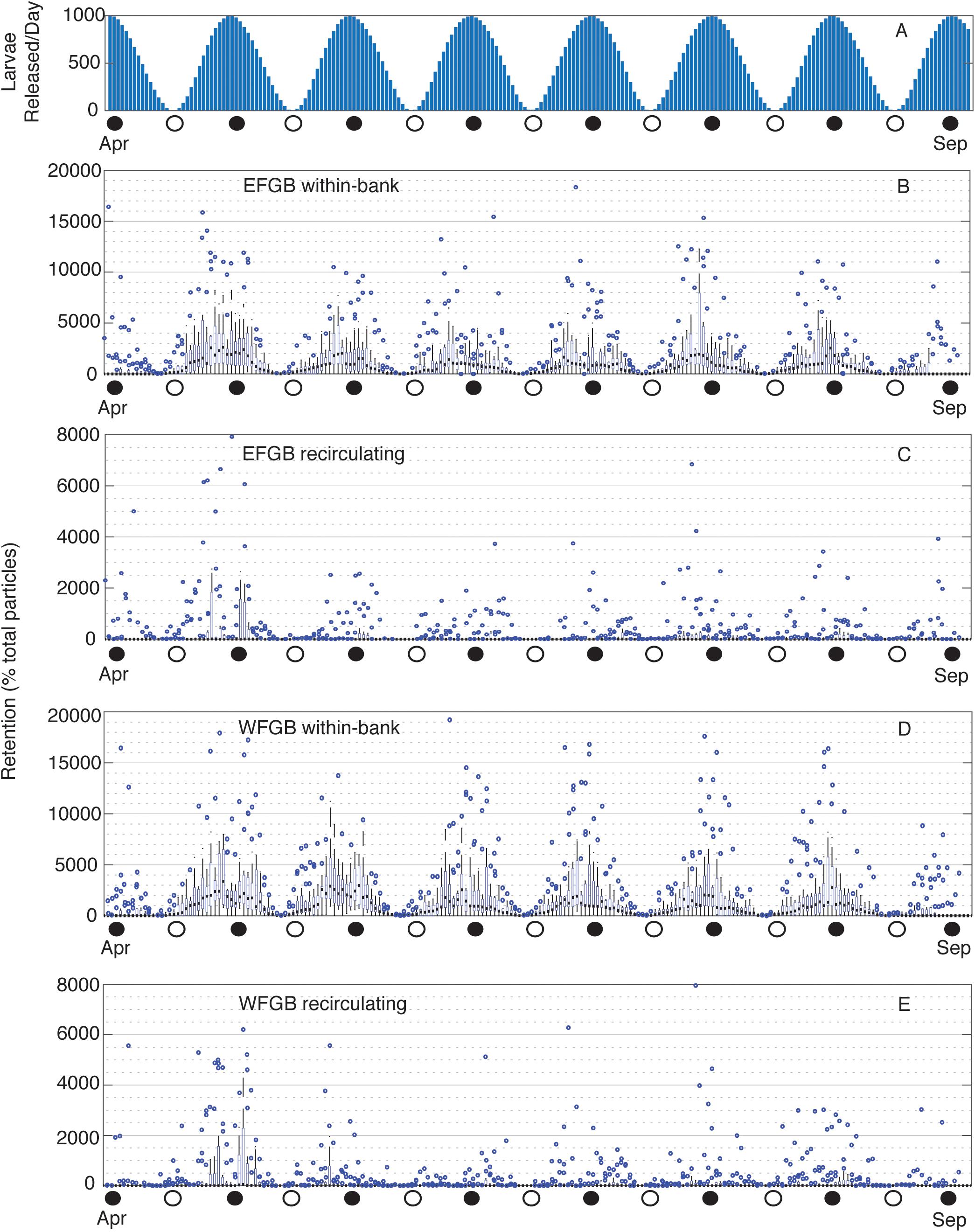
Figure 6. Particles released per lunar day and daily EFGB and WFGB P. astreoides local retention. (A) Number of particles released per lunar day from April to September. (B) EFGB total within-bank retention, and (C) total recirculating retention, followed by (D) WFGB total within-bank retention, and (E) total recirculating retention. Box plots show the annual distribution of the number of particles locally retained at WFGB per lunar day across 15 years of seasonal planulation (2004–2018). Boxes represent interquartile ranges, black squares are median values, blue circles are outliers, and whiskers represent the first and fourth quartiles. Note different y-axis scales.
Within-bank P. astreoides retention was consistently highest during new moons, following peaks in planulation (Figures 6A,B,D). Most of this was due to early retention, whereas recirculating local retention did not consistently follow this same trend. However, outlier years did follow this trend, and high reproductive output was associated with pulses of recirculating local retention when physical conditions were favorable (Figures 6C,E). P. astreoides between-bank exchange was also loosely associated with peaks in planulation (Supplementary Figure S5), particularly so in outlier years.
Larval distributions became more diffuse with time, and these distributions varied considerably between years (Figure 7). The effects of LCEs passing over the FGB in shaping the distribution of larval positions, and affecting recirculating retention, can be seen early in the competency window (Figure 7G), and as late as 4 weeks after planulation (Figure 7C).
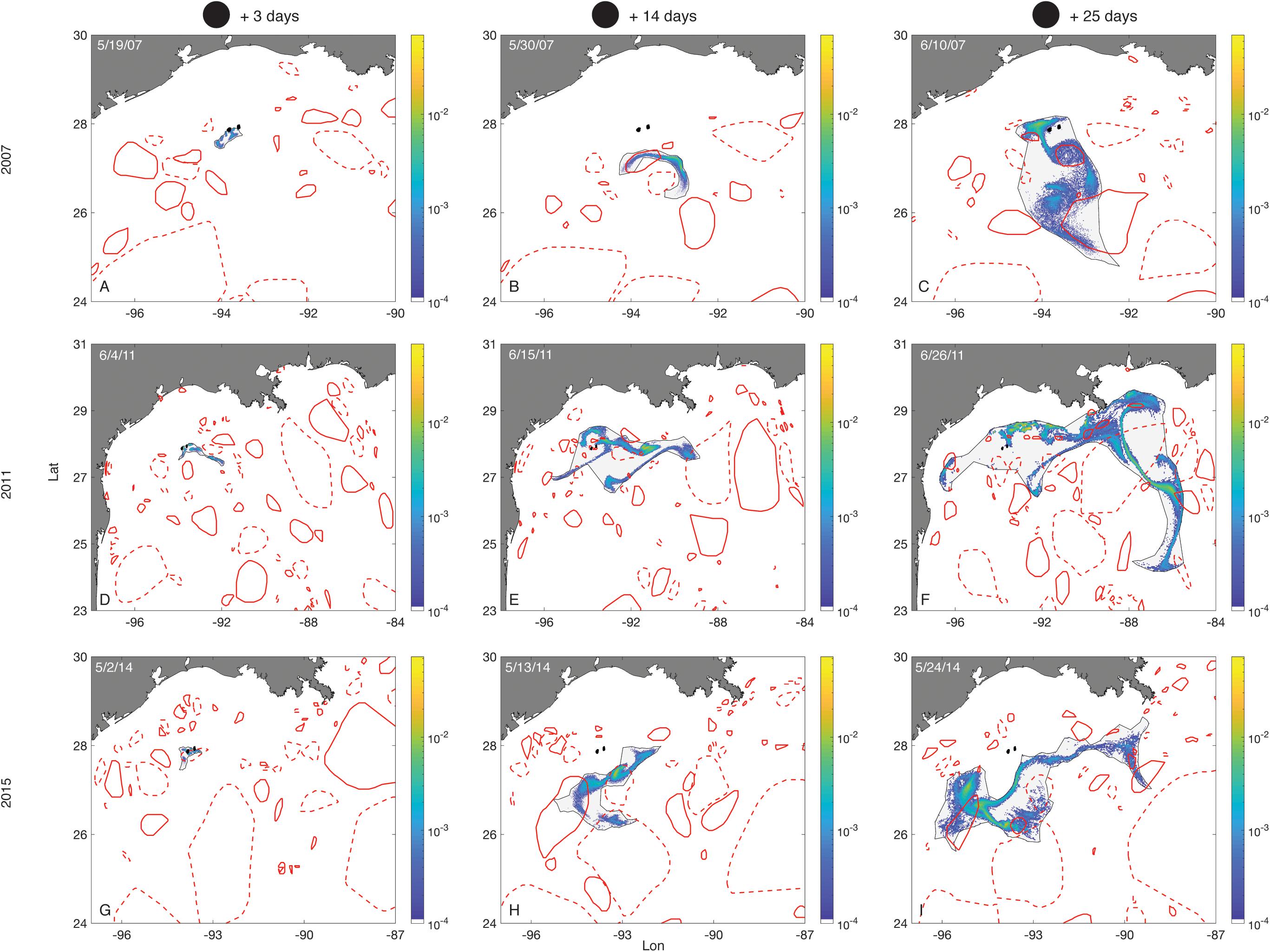
Figure 7. Larval distributions–Porites astreoides. Plots show larval distributions as probability density surfaces bounded by non-convex hulls following seven days of planulation in each of three different months (rows) (larvae released from 3 days before to 3 days after the new moon of that month). The first column shows the distribution of larvae on the third day after the new moon. The next two columns show larval distributions 14 and 25 days after the new moon, respectively. Density is scaled to the maximum single cell value within a planulation event. The FGB sanctuary boundaries are shown as black filled polygons. Eddies are represented by red empty polygons. Solid lines are cyclonic eddies and dashed lines are anticyclonic eddies.
Orbicella faveolata
Flower Garden Banks O. faveolata virtual larvae entrained in the Western Boundary Current and Loop Current had the potential to reach the Florida Keys and advect further to the northeastern boundary of the hydrodynamic model, near the mid-Atlantic Bight. Other dispersal pathways included westward transport along the Texas shelf and variable dispersal associated both with entrainment within LCEs and advection otherwise influenced by LCEs. Particles interacting with LCEs had the potential to return to the sanctuary boundaries, and 6.96% of all O. faveolata virtual larvae released throughout the simulation left and then returned to the FGB at least once (inclusive of between-bank exchange) (Table 2).
Orbicella faveolata retention peaked in magnitude at 3 days of dispersal, just after larvae became competent, and peaked again just after the first week of dispersal, which suggests recirculating interactions with LCEs (Figure 5D). Retention across years was highest later in the competency window at 1.5–2 weeks, reaching a median of >0.1%. While retention occurred in every year, it was highly variable throughout the PLD. The highest peaks in O. faveolata retention occurred stochastically, following only six of the 25 modeled spawning events from 2004 to 2018 (Figure 5D). Smaller magnitude, more frequent, and longer duration peaks in retention occurred as late as 26 days into the PLD (Figure 5D). Beyond 30 days of dispersal, retention became unlikely, and little to no retention occurred after 60–70 days of dispersal (Figure 5E).
Examples of how LCEs affected O. faveolata larval distributions over time can be seen in Figure 8. In some spawning events (August, 2006), substantial retention occurred throughout an entire month of dispersal (Figures 8A–C). On other occasions, advective forces dominated at the onset of competence (Figures 8D,G) and later in the dispersal window (Figures 8E,F,H), leading to negligible retention.
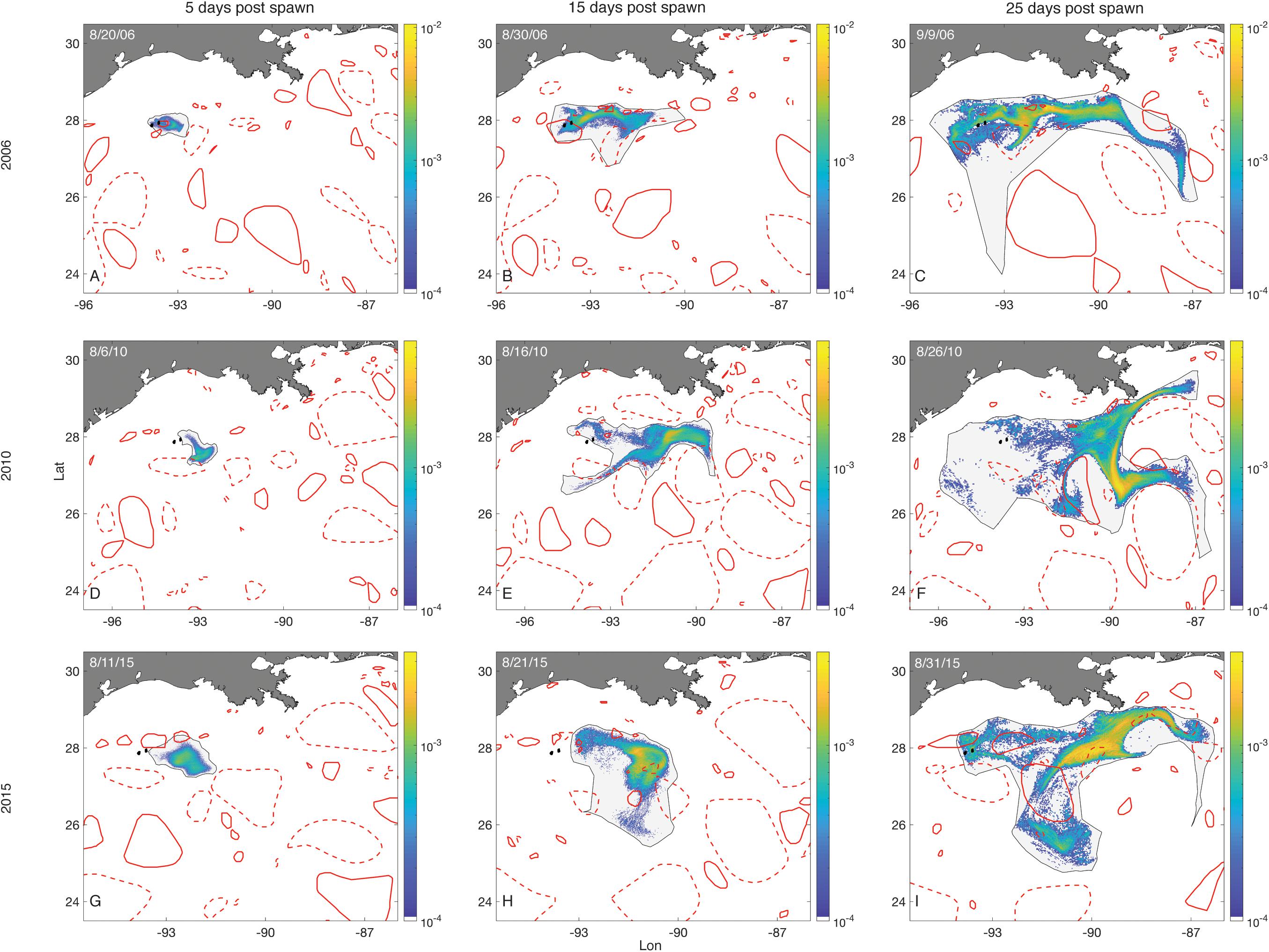
Figure 8. Larval distributions–O. faveolata. Plots show larval distributions as probability density surfaces bounded by non-convex hulls following three 3-day spawning events (rows). The first column shows the distribution of larvae on the fifth day after the start of spawning, representative of the first full day in which all larvae were competent. The next two columns show distributions of those larvae 15 and 25 days into their dispersal windows. Density is scaled to the maximum single cell value within a spawning event. The FGB sanctuary boundaries are shown as black filled polygons. Eddies are represented by red empty polygons. Solid lines are cyclonic eddies and dashed lines are anticyclonic eddies.
Eddies, Larval Trajectories, and Peaks in Retention
In general, LCEs were associated in retention that occurred later in larval PLDs. Thus, LCEs were more associated with O. faveolata retention, whereas pre-advection retention was the dominant mechanism for P. astreoides. Most individual P. astreoides planulation events did not result in recirculating retention, but advective processes associated with LCEs passing near the FGB resulted in stochastic pulses of recirculating retention.
Over the thousands of planulation and spawning events simulated, most did not involve significant recirculating retention associated with LCEs. However, these events were consistent throughout the 15 model years, resulting in heavily skewed distributions of annual retention (Figure 5). Although relatively rare, obvious entrainment within—or advection associated with LCEs and subsequent redelivery to the FGB—was observed in the dispersal of both species. For example, numerous LCEs passed over or near the FGB during and following the September 2018 O. faveolata spawning event (Supplementary Figure S8). Larval trajectories were influenced by the outer circulation of cyclonic eddies passing near or over the FGB throughout the first 5 days of their PLD. Nearly 2% of particles released from the EFGB during this spawning event passed over the WFGB during their eighth day of dispersal.
In another example, between 40 and 75% of virtual P. astreoides larvae planulated on August 24 2006 and August 25 2006 were predicted to return to the FGB between 5 and 8 days into their PLD (Supplementary Figure S9); each of the four modes of retention had a distinguishable peak in the PLD. 100% of EFGB larvae dispersed through WFGB’s boundaries during the 5–8 day window. In addition, 100% of WFGB larvae recirculated after advection, and returned to WFGB by day 10. These larval trajectories were heavily influenced by an LCE passing over the FGB (Supplementary Figures S9, S10).
Discussion
The biophysical models of larval dispersal developed for P. astreoides and O. faveolata emphasize that multiple biophysical mechanisms enhance local retention of larvae in isolated coral populations in the NW GoM. Differential life-histories in the modeled species—notably species-specific reproductive timing and larval traits—determined the relative influence of these mechanisms, including the role of LCEs in recirculating larvae over natal habitat or between submerged banks. The extended duration of this biophysical model, utilizing 15 years of regional hydrodynamics, has highlighted potential ecological trends in otherwise complex and stochastic biophysical phenomena.
Biophysical models of larval dispersal, and assessments of larval retention, are limited by the resolutions (spatial, temporal, etc.) of their hydrodynamic datasets (Briton et al., 2018). Sub-grid dynamics, here approximated by diffusion, certainly affect the movement, retention, and dispersal of larvae as they move over or near highly rugose coral reefs and complex coastlines (Koehl and Hadfield, 2010; Nickols et al., 2012; Hata et al., 2017). Additionally, eddy features that influence retention on the FGB may be smaller than the minimum detection size in this study. For these reasons, we have likely underestimated the potential for larval retention at the FGB, particularly in the first several days of dispersal.
In general, the five modes of potential FGB coral larval circulation discussed by Lugo-Fernández et al. (2001) and further investigated by Davies et al. (2017) and Garavelli et al. (2018) were well-represented in our simulations. At times virtual coral larvae dispersed (1) cyclonically to the west, (2) continually to the east where they would be influenced by the Mississippi River plume, (3) across the GoM basin, or were entrained in eddies either (4) oceanically or (5) over the continental shelf. Larval retention at FGB is theoretically possible in each of these modes of circulation, depending on the interplay between larval traits and the physical environment.
Time to larval competency following planulation or spawning strongly determined within-bank retention, and whether larvae would have the chance to settle on natal reefs before advection. Thus, for brooders like P. astreoides, larval retention could occur in nearly any velocity field and within-bank retention would be expected to be far greater than for spawners. Broadcast spawner larvae, which are more likely to advect from the FGB before becoming competent, may be more reliant on interactions with LCEs and recirculation to settle over natal reefs.
Brooders often display high settlement success (Szmant, 1986), and high seascape genetic structure (Underwood et al., 2009), which suggests that local retention and recruitment of larvae is a feature of their life-histories. However, in the FGB, both brooders (Brazeau et al., 2005) and broadcast spawners (Goodbody-Gringley et al., 2012; Studivan and Voss, 2018a, b) demonstrated reduced genetic structure, implying substantial gene flow among well-mixed populations. With high levels of between-bank exchange, especially within the first several days of dispersal, our model corroborates this hypothesis, suggesting that these populations may be well mixed. This early between-bank exchange was driven by directional currents and by short-distance interactions with LCEs, and was predominantly in the west-east direction, with frequent reversals. FGB coral populations likely experience recruitment from localized retentive events and exchanges across banks and subpopulations.
With a longer reproductive season, P. astreoides larvae are exposed to a wider variety of hydrodynamic conditions than are the gametes and larvae of O. faveolata. It would be natural to assume that this would result in greater opportunities for retention due to favorable oceanography. Interestingly, while retention at FGB was more consistent inter-annually in P. astreoides, the exposure to a wider variety of hydrodynamic conditions did not necessarily lead to greater magnitudes of retention across the PLD as compared to O. faveolata. One explanation is that retentive LCEs move over the FGB seasonally, and are more contemporaneous with late summer orbicellid spawning than with the extended P. astreoides reproductive season. Our findings corroborate studies which suggest that the Loop Current sheds the greatest number of eddies in late summer and winter due to the interactions of seasonal winds in the GoM and Caribbean (Chang and Oey, 2012), with the greatest peak in eddy formation occurring in September. In our analysis, peaks in eddy formation, eddy interactions with the FGB, and orbicellid retention are all contemporaneous in late summer. Despite September being the end of its reproductive season, P. astreoides larvae eddy entrainment also peaked in September.
Van Woesik (2010) asserts that mass coral spawning synchrony and timing is associated with calm periods in local wind fields, and that the tightest spawning synchrony occurs when there are brief and pronounced summer doldrums. These doldrums, it is thought, should enhance fertilization success and local retention, due to reduced water movement. Interestingly, at the FGB, these summer wind doldrums are contemporaneous with maximum LCE shedding in late summer (Chang and Oey, 2012), which suggests an additional physical mechanism of retention associated with the timing of coral spawning. While we have not investigated the occurrence of summer doldrums in the years simulated, this would be an interesting area for further study of coral spawning and larval retention in the GoM. Coral mass spawning is tightly synchronous and consistent at the FGB (Vize et al., 2005). Thus, it appears that late summer may be the biophysical optimum for the retention of coral larvae over the FGB, and the positive feedback of favorable biological and physical conditions may help in explaining the health and persistence of these isolated reefs.
Lugo-Fernández et al. (2001) predicted that cyclonic motion and eddy features could lead to larval retention within 24–30 days of coral spawning at the FGB. We found that after 30 days of dispersal, retention in both modeled species became vanishingly unlikely. However, we also found that an extended PLD lent itself to intra-annually rare, but inter-annually consistent late-duration larval retention. Small fractions of O. faveolata virtual larvae returned to the FGB throughout the 120 days of their PLD. Given a large reproductive event in a favorable year, this model predicts that Orbicella faveolata larvae—or larvae of other broadcast spawning species with extended maximum PLDs—could feasibly return to the FGB months after spawning. However, these long larval pathways back to FGB are less plausible due to increased mortality and involve many difficult-to-predict transitions between regional currents and mesoscale features. Longer PLDs are generally associated with longer distance dispersal in fishes (with notable exceptions) (Lester and Ruttenberg, 2005; Treml et al., 2012), but here we show that long PLDs can provide opportunities for recirculating retention late in the pelagic duration in corals, and that individual cohorts of larvae may experience multiple “chances” to settle on natal reefs.
Interactions with LCEs—both entrainment in the eddy core, and advection around its edges—have important implications for multiple chances at local retention for dispersing coral larvae. Because larvae are largely passive and at the mercy of hydrodynamics, the fact that LCEs may deliver the same larvae over the same reef multiple times may compound the probability of larval success. This is a particularly interesting phenomenon given the isolation and depth of the FGB. While shallow benthic and coastal features may influence local hydrodynamics and encourage reef-scale retention (Cetina-Heredia and Connolly, 2011), LCEs are regional mesoscale features. Mesoscale eddies have been associated with larval fish recruitment pulses and the entrainment of larvae near coastal habitats (Paris et al., 1997; Sponaugle et al., 2005; Lindo-Atichati et al., 2016; Shulzitski et al., 2016; Vaz et al., 2016), but the “second chances” potentially afforded by LCEs at the FGB may be unique due to their isolation. Because they are relatively deep and distant from shore, FGB reefs do not experience the complex coastal or barrier reef flows most typical coral reefs experience as a result of the interactions of waves, alongshore currents, and complex shallow bathymetry. As high-resolution hydrodynamics improve and become more widely available, the role of mesoscale and sub-mesoscale features in marine larval retention in a variety of habitats is becoming increasingly recognized and studied (Briton et al., 2018).
Coral larvae are expected to experience reduced fitness and high mortality as they disperse, and second chances at retention may be rarer than assumed here. We have not modeled the dynamics of recruitment or post-settlement, which may be affected, positively or negatively, by prolonged dispersal and changing seasonal environmental conditions. The overwhelming bulk of dispersal in this model, including interactions with LCEs, resulted in advection of virtual coral larvae away from the FGB and into deep or inhospitable waters. This corroborates previous modeling of corals (Davies et al., 2017; Garavelli et al., 2018) and fish (Johnson et al., 2017) in the region. Advection, rather than retention, is likely the defining feature of larval dispersal from FGB (Johnson et al., 2017).
Due to their relative geographic isolation and greater health compared to other western Atlantic reefs, the FGB have been proposed as a potential climate change refuge habitat (Hickerson et al., 2012; Davies et al., 2017; Studivan and Voss, 2018b). Climate refuges are thought to provide ecological or even evolutionary protection for a species or community during times of environmental change or instability. In general, in order for a coral reef to be an effective refuge, it should: (1) have relatively greater resistance or resilience to stress; (2) be capable of providing larvae to downstream habitats; and (3) be self-sustaining (Bongaerts et al., 2010; Davies et al., 2017; Kavousi and Keppel, 2018; Bongaerts and Smith, 2019). The stability of the coral reef community and isolation of the FGB satisfies the first criterion (Johnston et al., 2016), and several studies have suggested that the FGB provide coral recruits to downstream submerged banks (Garavelli et al., 2018; Studivan and Voss, 2018a, b), to Pulley Ridge (Olascoaga et al., 2018), to the Dry Tortugas (Studivan and Voss, 2018b), and to the Florida Keys (Lugo-Fernández et al., 2001; Davies et al., 2017), which would satisfy the second criterion. Here we have demonstrated several biophysical mechanisms thought to enhance retention of coral larvae at the FGB for species with considerably different life history traits. Due to their isolation, this local retention of larvae at the FGB may be a predominant source of coral recruitment, and these reefs may be largely self-sustaining. Assuming that conditions remain favorable for coral reefs at the FGB, the current study supports the hypothesis that these reefs could function as a refuge for Western Atlantic coral metapopulations experiencing disturbance. Our finding that LCEs play an important role in the retention of coral larvae at the FGB suggest that understanding how these ephemeral mesoscale features interact with micro- or habitat-scale oceanography and bathymetry is essential to understanding the persistence and resilience of these unique coral reefs.
Data Availability Statement
The datasets generated for this study are available at https://github.com/seascapelab/FGB-eddies and by reasonable request to the corresponding author.
Author Contributions
BL and DH conceived of and designed the experiments and analysis. BL performed all simulations and data analysis. All authors contributed to the interpretation of the results, writing the manuscript, and the manuscript revision, and read and approved the submitted version.
Funding
The Louisiana Board of Regents has provided the funding to support this research in their stipend allowance.
Conflict of Interest
The authors declare that the research was conducted in the absence of any commercial or financial relationships that could be construed as a potential conflict of interest.
Acknowledgments
This manuscript is part of a Special Topic, developed as an outcome of the Gulf of Mexico Reef Symposium, organized by A. Correa, R. Martindale, and A. Weiss. We would like to thank the sponsors of that meeting: the Rice University Creative Ventures Conference and Workshop Development Fund, the Paleontological Society, and The University of Texas, Austin, Jackson School of Geosciences. We would like to acknowledge S. Fife for providing assistance in data processing. Portions of this research were conducted with high performance computing resources provided by Louisiana State University (http://www.hpc.lsu.edu).
Supplementary Material
The Supplementary Material for this article can be found online at: https://www.frontiersin.org/articles/10.3389/fmars.2020.00372/full#supplementary-material
References
Alvera-Azcárate, A., Barth, A., and Weisberg, R. H. (2008). The surface circulation of the Caribbean sea and the Gulf of Mexico as inferred from satellite altimetry. J. Phys. Oceanogr. 39, 640–657. doi: 10.1175/2008jpo3765.1
Bleck, R. (2002). An oceanic general circulation model framed in hybrid isopycnic-Cartesian coordinates. Ocean Model. 4, 55–88. doi: 10.1016/S1463-5003(01)00012-9
Bongaerts, P., Ridgway, T., Sampayo, E. M., and Hoegh-Guldberg, O. (2010). Assessing the “deep reef refugia” hypothesis: focus on Caribbean reefs. Coral Reefs 29, 1–19. doi: 10.1007/s00338-009-0581-x
Bongaerts, P., and Smith, T. B. (2019). “Beyond the “Deep Reef Refuge” hypothesis: a conceptual framework to characterize persistence at depth,” in Mesophotic Coral Ecosystems Cham: Springer, 881–895.
Brazeau, D. A., Sammarco, P. W., and Gleason, D. F. (2005). A multi-locus genetic assignment technique to assess sources of Agaricia agaricites larvae on coral reefs. Mar. Biol. 147, 1141–1148. doi: 10.1007/s00227-005-0022-5
Briton, F., Cortese, D., Duhaut, T., and Guizien, K. (2018). High-resolution modelling of ocean circulation can reveal retention spots important for biodiversity conservation. Aquat. Conserv. Mar. Freshw. Ecosyst. 28, 882–893. doi: 10.1002/aqc.2901
Buston, P. M., Jones, G. P., Planes, S., and Thorrold, S. R. (2012). Probability of successful larval dispersal declines fivefold over 1 km in a coral reef fish. Proc. R. Soc. B Biol. Sci. 279, 1883–1888. doi: 10.1098/rspb.2011.2041
Cetina-Heredia, P., and Connolly, S. R. (2011). A simple approximation for larval retention around reefs. Coral Reefs 30, 593–605. doi: 10.1007/s00338-011-0749-z
Chang, Y. L., and Oey, L. Y. (2012). Why does the loop current tend to shed more eddies in summer and winter? Geophys. Res. Lett. 39, 1–7. doi: 10.1029/2011GL050773
Chassignet, E. P., Hurlburt, H. E., Smedstad, O. M., Halliwell, G. R., Hogan, P. J., Wallcraft, A. J., et al. (2007). The HYCOM (HYbrid coordinate ocean model) data assimilative system. J. Mar. Syst. 65, 60–83. doi: 10.1016/j.jmarsys.2005.09.016
Chornesky, E. A., and Peters, E. C. (1987). Sexual reproduction and colony growth in the scleractinian coral porites astreoides. Biol. Bull. 172, 161–177.
Clark, R., Kracker, L. M., Taylor, J. C., and Buckel, C. A. (2014). Fish and Benthic Communities of the Flower Garden Banks National Marine Sanctuary: Science to Support Sanctuary Management. NOAA Technical Memoranda, Vol. 179. Silver Spring, MD: NOS NCCOS, 317.
Connolly, S. R., and Baird, A. H. (2010). Estimating dispersal potential for marine larvae?: dynamic models applied to scleractinian corals. Ecology 91, 3572–3583. doi: 10.2307/29779541
Davies, S. W., Strader, M. E., Kool, J. T., Kenkel, C. D., and Matz, M. V. (2017). Modeled differences of coral life-history traits influence the refugium potential of a remote Caribbean reef. Coral Reefs 36, 913–925. doi: 10.1007/s00338-017-1583-8
DeBose, J. L., Nuttall, M. F., Hickerson, E. L., and Schmahl, G. P. (2013). A high-latitude coral community with an uncertain future: stetson bank, northwestern Gulf of Mexico. Coral Reefs 32, 255–267. doi: 10.1007/s00338-012-0971-3
Edmunds, P., Gates, R., and Gleason, D. (2001). The biology of larvae from the reef coral Porites astreoides, and their response to temperature disturbances. Mar. Biol. 139, 981–989. doi: 10.1007/s002270100634
Esri (2019). “Ocean [basemap].” Scale Not Given. “Ocean Basemap”. Available online at: https://www.arcgis.com/home/item.html?id=5ae9e138a17842688b0b79283a4353f6 (accessed July 11, 2019).
Faghmous, J. H., Frenger, I., Yao, Y., Warmka, R., Lindell, A., and Kumar, V. (2015). A daily global mesoscale ocean eddy dataset from satellite altimetry. Sci. Data 2:150028. doi: 10.1038/sdata.2015.28
Faghmous, J. H., Styles, L., Mithal, V., Boriah, S., Liess, S., Kumar, V., et al. (2012). “EddyScan: a physically consistent ocean eddy monitoring application,” in Proceedings of the 2012 Conference on Intelligent Data Understanding, (Boulder, CO: IEEE), 96–103. doi: 10.1109/CIDU.2012.6382189
Flower Garden Banks National Marine Sanctuary (2018). Spawning Observations. Gulf of Mexico: Flower Garden Banks National Marine Sanctuary
Garavelli, L., Studivan, M. S., Voss, J. D., Kuba, A., Figueiredo, J., and Chérubin, L. M. (2018). Assessment of mesophotic coral ecosystem connectivity for proposed expansion of a marine sanctuary in the Northwest Gulf of Mexico: larval dynamics. Front. Mar. Sci. 5:174. doi: 10.3389/fmars.2018.00174
Gittings, S. R., Boland, G. S., Deslarzes, K. J. P., Combs, C. L., Holland, B. S., and Bright, T. J. (1992). Mass spawning and reproductive viability of reef corals at the East Flower Garden Bank, northwest Gulf of Mexico. Bull. Mar. Sci. 51, 420–428.
Goodbody-Gringley, G., Woollacott, R. M., and Giribet, G. (2012). Population structure and connectivity in the Atlantic scleractinian coral Montastraea cavernosa (Linnaeus, 1767). Mar. Ecol. 33, 32–48. doi: 10.1111/j.1439-0485.2011.00452.x
Graham, E. M., Baird, A. H., and Connolly, S. R. (2008). Survival dynamics of scleractinian coral larvae and implications for dispersal. Coral Reefs 27, 529–539. doi: 10.1007/s00338-008-0361-z
Green, D. H., Edmunds, P. J., and Carpenter, R. C. (2008). Increasing relative abundance of Porites astreoides on Caribbean reefs mediated by an overall decline in coral cover. Mar. Ecol. Prog. Ser. 359, 1–10. doi: 10.3354/meps07454
Harii, S., Kayanne, H., Takigawa, H., Hayashibara, T., and Yamamoto, M. (2002). Larval survivorship, competency periods and settlement of two brooding corals, Heliopora coerulea and Pocillopora damicornis. Mar. Biol. 141, 39–46. doi: 10.1007/s00227-002-0812-y
Harrison, P. L., and Wallace, C. W. (1990). “Reproduction, dispersal and recruitment of scleractinian corals,” in Ecosystems of the World: Coral Reefs, ed. Z. Dubinsky (Amsterdam: Elsevier), 133–207.
Hata, T., Madin, J. S., Cumbo, V. R., Denny, M., Figueiredo, J., Harii, S., et al. (2017). Coral larvae are poor swimmers and require fine-scale reef structure to settle. Sci. Rep. 7:2249. doi: 10.1038/s41598-017-02402-y
Hickerson, E. L., Schmahl, G. P., Johnston, M. A., Nuttall, M. F., Embesi, J. A., and Eckert, R. J. (2012). “Flower garden banks–a refuge in the Gulf of Mexico?” in Proceedings of the 12th International Coral Reef Symposium, Vol. 1(Cairns, Australia), 9–13.
Hickerson, E. L., Schmahl, G. P., Robbart, M., Precht, W. F., and Caldow, C. (2008). The state of coral reef ecosystems of the Flower Garden Banks, Stetson Bank, and other banks in the northwestern Gulf of Mexico. The state of coral reef ecosystems of the United States and Pacific Freely Associated States, 189–217.
Holstein, D. M., Paris, C. B., Vaz, A. C., and Smith, T. B. (2015). Modeling vertical coral connectivity and mesophotic refugia. Coral Reefs 35, 23–37. doi: 10.1007/s00338-015-1339-2
Hughes, T. P., Barnes, M. L., Bellwood, D. R., Cinner, J. E., Cumming, G. S., Jackson, J. B. C., et al. (2017). Coral reefs in the Anthropocene. Nature 546, 82–90. doi: 10.1038/nature22901
Hughes, T. P., and Tanner, J. E. (2000). Recruitment failure, life histories, and long-term decline of Caribbean corals. Ecology 81, 2250–2263.
Johnson, D. R., Perry, H., Sanchez-Rubio, G., and Grace, M. A. (2017). Loop current spin-off eddies, slope currents and dispersal of reef fish larvae from the flower gardens national marine sanctuary and the florida middle grounds. Gulf Caribb. Res. 28, 29–39. doi: 10.18785/gcr.2801.10
Johnston, M. A., Eckert, R. J., Hickerson, E. L., Nuttall, M. F., Embesi, J. A., Sterne, T. K., et al. (2019). Localized coral reef mortality event at East Flower Garden Bank, Gulf of Mexico. Bull. Mar. Sci. 95, 239–250. doi: 10.5343/bms.2018.0057
Johnston, M. A., Embesi, J. A., Eckert, R. J., Nuttall, M. F., Hickerson, E. L., and Schmahl, G. P. (2016). Persistence of coral assemblages at East and West Flower Garden Banks, Gulf of Mexico. Coral Reefs 35, 821–826. doi: 10.1007/s00338-016-1452-x
Kavousi, J., and Keppel, G. (2018). Clarifying the concept of climate change refugia for coral reefs. ICES J. Mar. Sci. 75, 43–49. doi: 10.1093/icesjms/fsx124
Koehl, M. A. R., and Hadfield, M. G. (2010). Hydrodynamics of larval settlement from a larva’s point of view. Integr. Comp. Biol. 50, 539–551. doi: 10.1093/icb/icq101
LaJeunesse, T. C., Lee, S. Y., Gil-Agudelo, D. L., Knowlton, N., and Jeong, H. J. (2015). Symbiodinium necroappetens sp. nov. (Dinophyceae): an opportunist ‘zooxanthella’ found in bleached and diseased tissues of Caribbean reef corals. Eur. J. Phycol. 50, 223–238. doi: 10.1080/09670262.2015.1025857
Langdon, C., Albright, R., Baker, A. C., and Jones, P. (2018). Two threatened Caribbean coral species have contrasting responses to combined temperature and acidification stress. Limnol. Oceanogr. 63, 2450–2464. doi: 10.1002/lno.10952
Lester, S. E., and Ruttenberg, B. I. (2005). The relationship between pelagic larval duration and range size in tropical reef fishes: a synthetic analysis. Proc. R. Soc. B Biol. Sci. 272, 585–591. doi: 10.1098/rspb.2004.2985
Lindo-Atichati, D., Bringas, F., and Goni, G. (2013). Loop current excursions and ring detachments during 1993-2009. Int. J. Remote Sens. 34, 5042–5053. doi: 10.1080/01431161.2013.787504
Lindo-Atichati, D., Bringas, F., Goni, G., Muhling, B., Muller-Karger, F. E., and Habtes, S. (2012). Varying mesoscale structures influence larval fish distribution in the northern Gulf of Mexico. Mar. Ecol. Prog. Ser. 463, 245–257. doi: 10.3354/meps09860
Lindo-Atichati, D., Curcic, M., Paris, C. B., and Buston, P. M. (2016). Coastal ocean modelling description of surface transport in the region of the Belizean Barrier Reef based on observations and alternative high-resolution models. Ocean Model. 106, 74–89. doi: 10.1016/j.ocemod.2016.09.010
Lugo-Fernández, A., Deslarzes, K. J. P., Price, J. M., Boland, G. S., and Morin, M. V. (2001). Inferring probable dispersal of Flower Garden Banks coral larvae (Gulf of Mexico) using observed and simulated drifter trajectories. Cont. Shelf Res. 21, 47–67. doi: 10.1016/S0278-4343(00)00072-8
McGuire, M. P. (1998). Timing of larval release by Porites astreoides in the northern Florida Keys. Coral Reefs 17, 369–375.
McLaughlin, C. J., Bartley, J. D., Maxwell, B. A., Smith, C. A., and Buddemeier, R. W. (2003). Rivers, runoff, and reefs. Glob. Planet. Change 39, 191–199. doi: 10.1016/s0921-8181(03)00024-9
Muir, P. R., Marshall, P. A., Abdulla, A., and Aguirre, J. D. (2017). Species identity and depth predict bleaching severity in reef-building corals: shall the deep inherit the reef? Proc. R. Soc. B Biol. Sci. 284:20171551. doi: 10.1098/rspb.2017.1551
Nickols, K. J., Gaylord, B., and Largier, J. L. (2012). The coastal boundary layer: predictable current structure decreases alongshore transport and alters scales of dispersal. Mar. Ecol. Prog. Ser. 464, 17–35.
Oey, L., Ezer, T., and Lee, H. (2005). “Loop current, rings and related circulation in the Gulf of Mexico: a review of numerical models and future challenges,” in Circulation in the Gulf of Mexico: Observations and Model: Geophysical Monograph Series, Vol. 161, eds W. Sturges and A. Lugo-Fernandez (Washington, DC: American Geophysical Union), 31–56.
Okubo, A. (1971). Oceanic diffusion diagrams. Deep. Res. Oceanogr. Abstr. 18, 789–802. doi: 10.1016/0011-7471(71)90046-5
Olascoaga, M. J., Miron, P., Paris, C., Pérez-Brunius, P., Pérez-Portela, R., Smith, R. H., et al. (2018). Connectivity of pulley ridge with remote locations as inferred from satellite-tracked drifter trajectories. J. Geophys. Res. Ocean 123, 5742–5750. doi: 10.1029/2018JC014057
Olsen, K., Sneed, J. M., and Paul, V. J. (2016). Differential larval settlement responses of Porites astreoides and Acropora palmata in the presence of the green alga Halimeda opuntia. Coral Reefs 35, 521–525. doi: 10.1007/s00338-015-1394-8
Paris, C. B., Graber, H. C., Jones, D. L., Röpke, A. W., and Richards, W. J. (1997). Translocation of larval coral reef fishes via sub-mesoscale spin-off eddies from the Florida current. Bull. Mar. Sci. 60, 966–983.
Paris, C. B., Helgers, J., van Sebille, E., and Srinivasan, A. (2013). Connectivity modeling system: a probabilistic modeling tool for the multi-scale tracking of biotic and abiotic variability in the ocean. Environ. Model. Softw. 42, 47–54. doi: 10.1016/j.envsoft.2012.12.006
Porto-Hannes, I., Zubillaga, A. L., Shearer, T. L., Bastidas, C., Salazar, C., Coffroth, M. A., et al. (2015). Population structure of the corals Orbicella faveolata and Acropora palmata in the Mesoamerican Barrier Reef System with comparisons over Caribbean basin-wide spatial scale. Mar. Biol. 162, 81–98. doi: 10.1007/s00227-014-2560-1
Precht, W. F., Gintert, B. E., Robbart, M. L., Fura, R., and Van Woesik, R. (2016). Unprecedented disease-related coral mortality in Southeastern Florida. Sci. Rep. 6:31374. doi: 10.1038/srep31374
Rippe, J. P., Matz, M. V., Green, E. A., Medina, M., Khawaja, N. Z., Pongwarin, T., et al. (2017). Population structure and connectivity of the mountainous star coral, Orbicella faveolata, throughout the wider Caribbean region. Ecol. Evol. 7, 9234–9246. doi: 10.1002/ece3.3448
Ritson-Williams, R., Arnold, S., Paul, V. J., Fogarty, N., Vermeij, M., and Steneck, R. S. (2009). New perspectives on ecological mechanisms affecting coral recruitment on reefs. Smithson. Contrib. Mar. Sci. 38, 437–457. doi: 10.5479/si.01960768.38.437
Sammarco, P. W., Atchison, A. D., Boland, G. S., Sinclair, J., and Lirette, A. (2012). Geographic expansion of hermatypic and ahermatypic corals in the Gulf of Mexico, and implications for dispersal and recruitment. J. Exp. Mar. Bio. Ecol. 436–437, 36–49. doi: 10.1016/j.jembe.2012.08.009
Schmahl, G. P., Hickerson, E. L., and Precht, W. F. (2008). “Biology and ecology of coral reefs and coral communities in the Flower Garden Banks region, northwestern Gulf of Mexico,” in Coral Reefs of the USA, eds B. M. Riegl and R. E. Dodge (Dordrecht: Springer), 221–261.
Shanks, A. L. (2009). Pelagic larval duration and dispersal distance revisited. Biol. Bull. 216, 373–385. doi: 10.1086/BBLv216n3p373
Shulzitski, K., Sponaugle, S., Hauff, M., Walter, K. D., and Cowen, R. K. (2016). Encounter with mesoscale eddies enhances survival to settlement in larval coral reef fishes. Proc. Natl. Acad. Sci. U.S.A. 113, 6928–6933. doi: 10.1073/pnas.1601606113
Smith, T. B., Nemeth, R. S., Blondeau, J., Calnan, J. M., Kadison, E., and Herzlieb, S. (2008). Assessing coral reef health across onshore to offshore stress gradients in the US Virgin Islands. Mar. Pollut. Bull. 56, 1983–1991. doi: 10.1016/j.marpolbul.2008.08.015
Sponaugle, S., Lee, T., Kourafalou, V., Pinkard, D., and Sponauglel, S. (2005). Florida current frontal eddies and the settlement of coral reef fishes Florida Current. Limnol. Oceanogr. 50, 1033–1048.
Studivan, M. S., and Voss, J. D. (2018a). Assessment of mesophotic coral ecosystem connectivity for proposed expansion of a marine sanctuary in the Northwest Gulf of Mexico: population genetics. Front. Mar. Sci. 5:152.
Studivan, M. S., and Voss, J. D. (2018b). Population connectivity among shallow and mesophotic Montastraea cavernosa corals in the Gulf of Mexico identifies potential for refugia. Coral Reefs 37, 1183–1196. doi: 10.1007/s00338-018-1733-7
Szmant, A. M. (1986). Reproductive ecology of Caribbean reef corals. Coral Reefs 5, 43–53. doi: 10.1007/BF00302170
Szmant, A. M., and Meadows, M. G. (2006). Developmental changes in coral larval buoyancy and vertical swimming behavior: implications for dispersal and connectivity. Proc. 10th Int. Coral Reef Symp. 1, 431–437.
Treml, E. A., Roberts, J. J., Chao, Y., Halpin, P. N., Possingham, H. P., and Riginos, C. (2012). Reproductive output and duration of the pelagic larval stage determine seascape-wide connectivity of marine populations. Integr. Comp. Biol. 52, 525–537. doi: 10.1093/icb/ics101
Underwood, J. N., Smith, L. D., Van Oppen, M. J. H., and Gilmour, J. P. (2009). Ecologically relevant dispersal of corals on isolated reefs: implications for managing resilience stable. Ecol. Appl. 19, 18–29. doi: 10.1890/07-1461.1
U.S. Naval Observatory (2017). Fraction of the Moon Illuminated. Available online at: https://www.usno.navy.mil/USNO/astronomical-applications/data-services/data-services (accessed May 1, 2019).
Van Woesik, R. (2010). Calm before the spawn: global coral spawning patterns are explained by regional wind fields. Proc. R. Soc. B Biol. Sci. 277, 715–722. doi: 10.1098/rspb.2009.1524
Vaz, A. C., Paris, C. B., Olascoaga, M. J., Kourafalou, V. H., Kang, H., and Reed, J. K. (2016). The perfect storm: Match-mismatch of bio-physical events drives larval reef fish connectivity between Pulley Ridge mesophotic reef and the Florida Keys. Cont. Shelf Res. 125, 136–146. doi: 10.1016/j.csr.2016.06.012
Vize, P. D., Embesi, J. A., Nickell, M., Brown, D. P., and Hagman, D. K. (2005). Tight temporal consistency of coral mass spawning at the Flower Garden Banks, Gulf of Mexico, from 1997-2003. Gulf Mex. Sci. 23, 107–114. doi: 10.18785/goms.2301.08
Vize, P. D., Hilton, J. D., Brady, A. K., and Davies, S. W. (2008). Light sensing and the coordination of coral broadcast spawning behavior. Proc. 11th Int. Coral Reef Symp. 1, 385–388.
Vukovich, F. M. (2012). Changes in the loop current’s eddy shedding in the period 2001–2010. Int. J. Oceanogr. 2012, 1–18. doi: 10.1155/2012/439042
Wellington, G. M., and Fitt, W. K. (2003). Influence of UV radiation on the survival of larvae from broadcast-spawning reef corals. Mar. Biol. 143, 1185–1192. doi: 10.1007/s00227-003-1150-4
Williams, D. E., Miller, M. W., and Kramer, K. L. (2008). Recruitment failure in Florida keys Acropora palmata, a threatened Caribbean coral. Coral Reefs 27, 697–705. doi: 10.1007/s00338-008-0386-3
Keywords: coral reefs, eddies, local retention, biophysical models, larval ecology
Citation: Limer BD, Bloomberg J and Holstein DM (2020) The Influence of Eddies on Coral Larval Retention in the Flower Garden Banks. Front. Mar. Sci. 7:372. doi: 10.3389/fmars.2020.00372
Received: 16 July 2019; Accepted: 30 April 2020;
Published: 29 May 2020.
Edited by:
Hajime Kayanne, The University of Tokyo, JapanReviewed by:
Aldo Cróquer, Simón Bolívar University, VenezuelaMichael S. Studivan, University of Miami, United States
Copyright © 2020 Limer, Bloomberg and Holstein. This is an open-access article distributed under the terms of the Creative Commons Attribution License (CC BY). The use, distribution or reproduction in other forums is permitted, provided the original author(s) and the copyright owner(s) are credited and that the original publication in this journal is cited, in accordance with accepted academic practice. No use, distribution or reproduction is permitted which does not comply with these terms.
*Correspondence: Benjamin D. Limer, blimer1@lsu.edu