- 1Human Informatics and Predictive Performance Optimization (HIPPO) Lab, Department of Electrical and Computer Engineering, University of Florida, Gainesville, FL, United States
- 2Independent Researcher, Gainesville, FL, United States
- 3Department of Physiological Sciences, University of Florida, Gainesville, FL, United States
Breathing relies on unrestricted movement of the chest wall to maintain O2 and CO2 balance. Understanding the effects of chest and abdominal restrictions on respiratory function is critical for studying conditions such as respiratory diseases, extreme environments, and load-induced impairments. However, existing methods to simulate these restrictions are limited, lacking the ability to provide both static and dynamic conditions or precise load control. To address these gaps, we developed a novel chest wall and abdomen restriction device capable of independently applying and measuring static and dynamic loads with adjustable and reproducible force levels. Separate bands for the chest and abdomen enable targeted restrictions. In static conditions, the bands are immobilized, preventing any movement of the chest and abdomen. In dynamic conditions, constant force springs provide resistance, allowing movement when sufficient force is applied. Integrated sensors quantify applied loads and respiratory mechanics. To validate the device, healthy participants underwent pulmonary function testing under baseline, static, and dynamic restriction conditions. Significant reductions in forced expiratory volume (FEV1) and forced vital capacity (FVC) were observed under restrictions compared to baseline. Other respiratory metrics also differed significantly, highlighting distinct effects of static and dynamic restrictions. Pressure variability tests confirmed reproducibility and adjustability of loads, while displacement data from linear variable differential transducers (LVDTs) validated the device’s ability to distinguish static and dynamic effects. This device addresses prior limitations by enabling precise, reproducible loading and independent control of chest and abdominal restrictions, supporting research into respiratory diseases, extreme environments, and respiratory mechanics. Our results demonstrate its potential to advance respiratory function research and expand clinical and experimental applications.
1 Introduction
Breathing is an essential activity performed by the human body, serving as the cornerstone for all other activities. When one is unable to breathe, it disrupts the balance between oxygen (O2) and carbon dioxide (CO2) in the blood (Fähling and Persson, 2012). Therefore, the human body initiates a cascade of effects, leading to a lack of attention, confusion, loss of consciousness, morbidity, and mortality (Herzog, 1979; Bloomfield et al., 2022; Brannan et al., 2001). The chest wall plays a crucial role in the breathing process, serving as the housing for the lungs and dynamically expanding and contracting to facilitate air exchange (Eldridge and Davis, 1959). Thus, any restriction in the chest wall disrupts the breathing mechanism (Adler and Janssens, 2019; Altose, 2007; Eldridge and Davis, 1959). These restrictions may originate from physiological factors affecting thoracic compliance and muscle function, such as scoliosis, muscular dystrophy, and diaphragmatic paralysis (Kesten et al., 1991; Neijens et al., 1990; Šakić et al., 1992; Andersen et al., 1992), or from environmental factors, such as high g-forces, water pressure during deep-sea diving, compression from tight clothing like corsets or body armor, or heavy backpacks and safety harnesses used in activities like hiking, rock climbing, or skydiving (Brown and McConnell, 2012; Armstrong and Gay, 2016; Ribeiro Rodrigues et al., 2024a; Legg, 1988). However, studying these conditions is challenging due to the limited availability of patients, the logistical complexity of recreating environmental factors, and the need for controlled, repeatable experiments to ensure reliable data. To overcome these issues, we have developed a novel device designed to mimic chest and abdominal restrictions, enabling the exploration of both static and dynamic load-based restrictions. This novel device provides a controlled environment to study the effects of chest and abdominal restriction on breathing.
1.1 Prior work
We can categorize chest wall restrictions into two distinct types: 1) static restrictions and 2) dynamic load-based restrictions. In the static condition, the chest wall is immobile regardless of the force applied (Cline et al., 1999). Previous attempts to restrict the chest wall have mostly focused on static restriction. One of the earliest designs was a simple chest clamp used to limit the movement of the rib cage, as seen in Figure 1. The device consisted of two plywood boards—one placed on the posterior surface and the other on the anterior surface of the thorax (Zechman and Wiley, 1977). This approach, however, fails to match the contour of the body, restricting the rib cage only along the sternum and the spine. A more effective alternative that addresses this limitation is chest wall strapping (CWS). This procedure involves restricting the thorax and abdomen using fabric, cinch straps, and/or a chest cast (Sybrecht et al., 1975; Torchio et al., 2006; Eberlein et al., 2014; Miller et al., 2002; Chapman et al., 2012). While CWS is used today, it lacks the precision to quantify the load being added to the chest wall. Furthermore, none of these methods have a metric to allow for reproducible load levels or differentiate between chest and abdominal restriction.
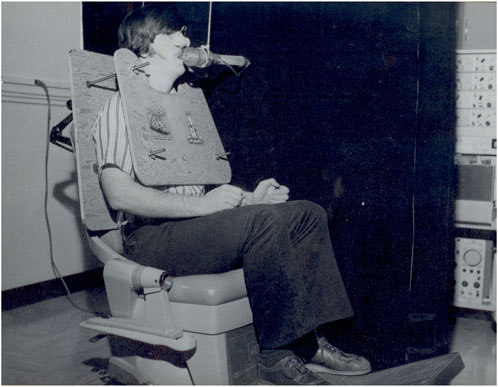
Figure 1. One of the first attempts to restrict chest wall movement using a static restriction (Zechman and Wiley, 1977).
In the dynamic condition, a consistent load is applied to the chest wall, allowing movement only when the participant generates sufficient force to overcome the resistance (Cline et al., 1999; Roethlisberger et al., 2018; Selickman and Marini, 2022; Chottidao, 2009). Previous efforts to design a dynamic restriction device that maintains the load while permitting moderate movement include a hard-shell, full-body apparatus. This device altered the pressure exerted on the chest in proportion to the inspired volume (Younes et al., 1990). However, it required participants to remain in a supine position and lacked a mechanism to quantify the applied load. Another attempt involved a fiberglass chest cast equipped with inflatable air pockets that allowed for controlled load adjustments (Cline et al., 1999). Despite this innovation, the device failed to restrict the abdomen and did not provide a way to measure the participant chest and abdomen movement. Finally, none of these devices offer the capability to provide both static and dynamic restrictions within a single design.
These gaps left by the previous designs lead to the following research questions:
RQ1 Can a single device be engineered to integrate both static and dynamic restriction capabilities, enabling comprehensive investigation of their distinct effects on respiratory mechanics?
RQ2 Can such a device be designed to deliver reproducible and precisely adjustable loads to both the chest and abdomen, facilitating targeted and reliable experimentation?
1.2 Challenges
Designing a chest wall restriction device capable of simulating a wide range of restrictions presents significant challenges. While existing methods in the literature often focus on replicating specific conditions, such as simulating respiratory diseases like cystic fibrosis or external loads from equipment like bulletproof vests, they are typically limited in scope. Previous methods lacked the precise control needed to adjust the load or the ability to transition between static and dynamic restrictions, making it difficult to tackle the diverse range of physiological and environmental conditions. Developing a more versatile device requires not only precise control over these variables but also the ability to accurately replicate both static and dynamic restrictions to better mimic real-world scenarios. Furthermore, prior methods lacked the ability to accurately quantify the applied load, as they did not have sensors or mechanisms to measure the precise force exerted on the chest wall. Consequently, researchers could not replicate specific conditions or measure the impact of varying load levels with accuracy.
Additionally, these methods did not differentiate between restrictions applied to the chest and those applied to the abdomen. These tools were often built as single-piece or full-coverage restraints, designed for general chest compression rather than targeted restriction, limiting the ability to isolate effects between the chest and abdomen. Finally, the inability to differentiate between chest and abdominal restrictions, combined with the use of single-piece designs, made measurement of chest and abdominal movement during restriction unfeasible. This limitation prevented detailed analysis of how restricted movement influences respiratory dynamics, making it difficult to capture or quantify subtle changes in breathing patterns.
1.3 Insights
To address these challenges, we developed a novel restriction device that uses two separate bands, one for the chest wall and one for the abdomen, allowing us to restrict each area independently. In the static condition, both bands are firmly held in place, preventing any movement. This setup simulates scenarios where the chest and abdomen are entirely restricted, similar to real-life situations requiring external braces or devices used to immobilize the torso. For the dynamic condition, the bands that restrict the chest and abdomen are held in place by a constant force spring, allowing the participant to move their chest and abdomen if they apply enough force to overcome the spring resistance. This design enables us to study the effects of dynamic loads where movement is possible but resisted, simulating conditions like respiratory diseases (e.g., COPD) or high G-force environments encountered by jet pilots or race car drivers (Giuriato et al., 2020). Additionally, two Linear Variable Differential Transducers (LVDTs) measure the horizontal displacement of the chest wall and abdomen during breathing under dynamic load, enabling us to detect and quantify subtle changes in breathing patterns.
The device’s vinyl-like fabric bands conform to the body, ensuring even load distribution across each targeted area. The inclusion of constant force springs enables the precise application of a known load to the chest and abdomen. Two pressure sensors monitor the pressure exerted by the bands, ensuring that the applied load is not only accurately set but also consistently maintained across different participants and trials. A calibration process ensures the device provides reproducible and adjustable loading conditions for a variety of experimental setups. By combining controlled loading, precise displacement measurement, and pressure monitoring, our device not only enables the study of static and dynamic chest wall restrictions on breathing but also provides a versatile platform to investigate their effects on cognitive function, blood circulation, and other physiological responses in a controlled environment (Napoli et al., 2020; Stephens et al., 2017).
1.4 Contributions
Our chest wall and abdomen restriction device is a powerful tool for studying respiratory challenges and dysfunction. Combining static and dynamic restrictions in one design allows us to simulate full movement restriction or use adjustable dynamics loads, creating a controlled environment to study how breathing adapts under different conditions. With separate bands for the chest and abdomen, we can isolate how each region contributes to breathing patterns, and built-in sensors like LVDTs allow us to monitor even the smallest changes in movement. The device is also highly reliable, using constant force springs and pressure sensors to ensure precise and repeatable restrictions. This makes it ideal for exploring respiratory diseases, the cognitive effects of breathing restrictions, and how the body responds to extreme conditions, opening up new possibilities for research and treatment.
2 Methods and materials
2.1 Structure design
This section provides a detailed description of the different parts of the device. For a comprehensive list of all components used, including detailed dimensions, refer to the schematics in Supplementary Appendices A–R. All parts were sourced from McMaster-Carr, except for the LVDTs, which were supplied by Omega.
2.1.1 Structure and frame
The chest wall and abdomen restriction device is a wooden structure designed for controlled chest and abdomen compression. The device measures 48 inches in height and 33 inches in width. The structure consists of three side-by-side wooden boards, forming a rectangular shape from the top and side views (see Figures 2, 3).
The device’s frame is built from a combination of precisely sized Compression Surface (front board), Rear Support Board (backboard), Chest Restriction Board and Abdominal Restriction Board (middle boards), and the Structural Base, creating a strong and stable structure. This immobility is pivotal allowing the participate to be accurately compressed against the backboard.
The Compression Surface (front board) and Rear Support Board (back board) are identical, each measuring 24 inches wide, 22 inches high, and 2 inches thick. These dimensions were chosen to provide a sturdy backbone capable of supporting the device’s components and ensuring durability during use. The 24-inch width offers ample space for mounting other parts of the device, while the 22-inch height accommodates the average torso size of participants, ensuring the device can comfortably fit a range of body types.
The Compression Surface features a foam pad measuring approximately 22 by 22 inches and 0.5 inches thick. This padding was carefully sized to match the height of the Compression Surface and Rear Support Board, ensuring full coverage and comfort for the participant’s back. The 0.5-inch thickness and appropriate softness provide sufficient cushioning to distribute pressure evenly, reducing discomfort and preventing pressure points during use. Importantly, the material is not too soft, ensuring that the restriction applied by the device remains effective and is not compromised by the padding.
Between the Compression Surface and Rear Support Board are two middle boards: the Chest Restriction Board and Abdominal Restriction Board. These boards slide along four rods, which connect the Compression Surface and Rear Support Board. Each middle board is 22 inches wide, 8 inches high, and 2 inches thick, with a 3.3-inch vertical gap separating them. The 22-inch width aligns with the width of the Compression Surface and Rear Support Board, maintaining structural consistency. The 8-inch height provides enough surface area to cover the chest wall and abdomen of participants, as each board is responsible for holding the mechanism that restricts both the chest and abdomen separately.
The Structural Base of the device serves as the foundation, ensuring overall stability and balance. It consists of four identical legs, each 1.5 inches by 2 inches wide and standing 16.5 inches tall. These dimensions provide a sturdy base capable of supporting the device’s height and weight without tipping. The legs are connected by two horizontal wooden beams, each measuring 1.5 inches by 1.5 inches in width and 24 inches long. The horizontal beams distribute the load evenly across all four legs, preventing wobbling and enhancing the device’s durability during prolonged use.
2.1.2 Rod placement and hooks
The rods connect the exterior wooden boards, creating a space of approximately 18 inches that allows the two middle boards to move freely. This spacing was chosen to provide enough room for placing the LVDTs (each 6.5 inches long) and for extending the springs to about 1.25 times their diameter (around 3 inches). More space might be needed depending on the LVDT being used and the diameter of the chosen springs. To support the springs, four metal hooks are positioned between the rods, as shown in Figures 3, 7. Each hook is spaced 6 inches apart, with an additional 6-inch gap between the rods and the hooks.
2.1.3 Strapping system
The blue vinyl strapping material, shown in Figure 4, is the device’s main component for applying restriction to the participant’s chest and abdomen, whether static or dynamic. Made from non-stretchable fabric, it prevents any unintended movement during use. Each strap is 3 feet long and 8 inches wide, providing enough material to fit individuals of various sizes. Once the participant is strapped in, the right side of the band overlaps the left side and is secured with Velcro. Then, the left band, connected to a metal handle and a ratchet gear mechanism, is tightened, pulling the right band along with it. The ratchet gear mechanism is shown in detail in Figure 5.
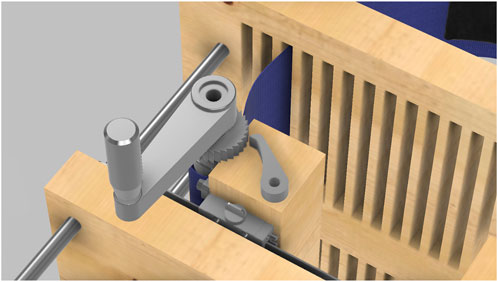
Figure 5. Close-up view of the ratchet gear mechanism used to adjust and secure the strapping system. This mechanism allows fine-tuning of the tension applied to the participant’s chest, ensuring precise control over compression.
2.1.4 Restriction modes overview
The same structural configuration is used for both dynamic and static restriction conditions. The key difference lies in how the middle boards are constrained, as illustrated in Figure 6. In the static condition, the boards are held in place and locked using shaft collars, preventing any movement regardless of the effort exerted by the participant. This creates a non-yielding restriction, where the participant breathes without any mechanical give. In the dynamic condition, the shaft collars are removed, and constant force springs are attached to the middle boards. This places the participant under a constant resistive load while still allowing movement of the boards if sufficient force is generated during breathing.
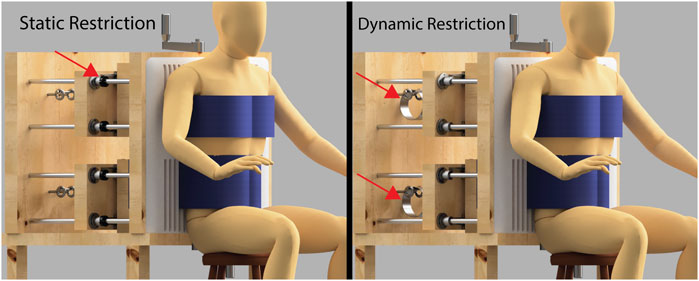
Figure 6. Side-by-side comparison of the static and dynamic chest restriction conditions. Notice that the overall setup remains exactly the same in both conditions. The only components that differ are the mechanisms shown by the red arrows. In the static restriction condition (left), the arrows point to shaft collars tightened onto the guide rods, which lock the middle boards in place and prevent any movement. This results in a fixed restriction that remains immobile regardless of the force exerted by the participant. In the dynamic restriction condition (right), the arrows highlight constant force springs that attach to the middle boards. These springs apply a constant resistive force that pulls the boards back, but still allow movement if sufficient force is generated by the participant’s chest and abdominal expansion.
2.1.5 Dynamic restriction and constant force springs
The adjustable force springs that provide a constant force are essential to the device’s functionality. They are made from a flat strip of spring material coiled into a cylinder. Unlike traditional compression springs, these springs provide a steady force throughout their range of motion, reaching full strength after being stretched to 1.25 times their diameter. Once tensioned, they maintain a consistent force, allowing precise adjustment of the restriction applied to the participant’s chest and abdomen. The springs are mounted on the device’s backboard and connect to the tie-down rings via carabiners (not pictured). This setup, shown in Figure 7, enables the device to apply a consistent, controllable dynamic restriction as the participant breathes, allowing the middle boards to move slightly while maintaining a steady resistive force.
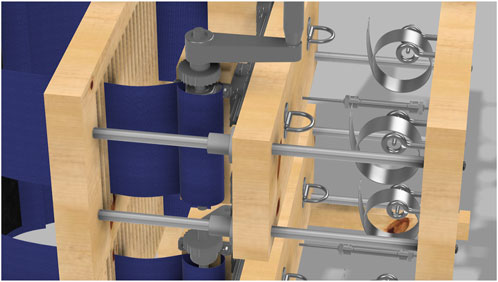
Figure 7. Close-up view of the dynamic load mechanism showing the constant force springs. Each spring is connected to a tie-down ring via a carabiner (not pictured).
2.1.6 Static restriction mechanism
For static restriction, the device uses four shaft collars, one on each rod. When these collars are positioned and tightened, the interior wooden walls are fixed in place, preventing any forward movement, as seen in Figure 8. This creates a static compression environment, allowing for studies where a constant chest or abdominal restriction is required. In this mode, the middle boards remain fixed and immobile, preventing any expansion beyond the preset restriction level during breathing.
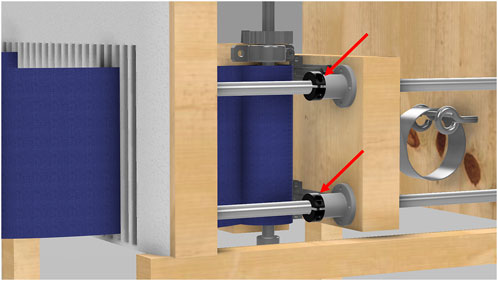
Figure 8. Close-up view of the static load mechanism. This image details the shaft collars positioned on the rods, which lock the interior wooden walls into place, creating a static restriction.
2.1.7 Adjustable width system
To ensure that the bands described in Section 3 fit snugly against the participant’s body, a mechanism was built into the middle boards to adjust the horizontal distance between the left and right bands for both the chest and abdomen separately.
Four wooden blocks (two for each middle board) move horizontally along metal rails mounted on the boards. These blocks, measuring 9 × 3.5 × 3.5 inches, slide on 19-inch rails attached to the interior walls (see Figure 9 for details). Slits cut into the front board, each 0.5 inches wide and spaced 1 inch apart, allow the bands to pass through and securely fit the participants’ body sizes.
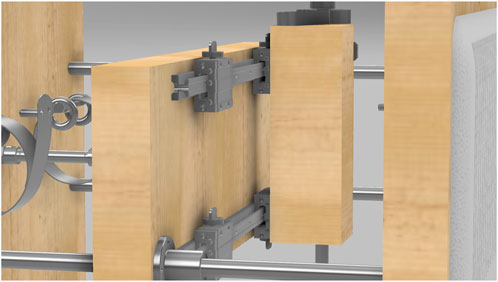
Figure 9. Close-up view of the adjustable width mechanism. The figure shows how the wooden boards slide along the metal rails, allowing controlled movement to adjust the width of the bands for a better fit.
2.1.8 Pressure sensors
The pressure sensors integrated into the strapping system are designed to accurately measure the pressure applied to the chest and abdomen, ensuring reproducibility and consistency in experimental conditions. Each sensor (shown in black in Figure 10) consists of a Millar Mikro-Cath pressure catheter inserted into an air bladder placed inside a pocket on the left band. The bladders measure 3.75 inches by 23 inches and are filled with exactly 60 mL of air using a calibrated syringe before each trial to standardize internal volume. The pressure catheters are calibrated before use according to the manufacturer’s guidelines to ensure signal accuracy.
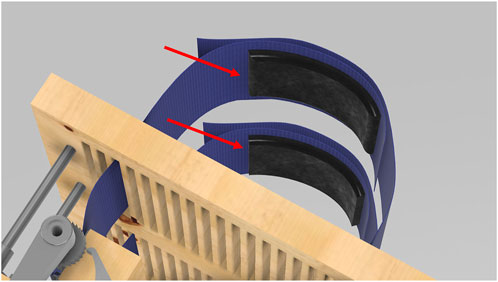
Figure 10. Close-up view of the integrated pressure sensors within the strapping system. These sensors are used to measure the pressure applied to the chest and abdomen, ensuring accurate and consistent experimental conditions.
2.2 Device setup
2.2.1 Participant positioning
The first step in setting up the participant in our procedure involves measuring the participant’s height and torso width. The participant then sits on an adjustable stool, initially measuring from the floor to the seat 16 inches and extending to a total height of 23 inches. We adjust the stool based on their chest and abdomen. The participant sits with their back against the front of the device, pressing onto the padded front wooden wall, and we use a metal gear handle to unwind enough vinyl strapping (loosen the straps) for a customized circumferential fit. We confirm the participant’s width and adjust the width of the bands to match using the adjustable width system (described in Section 7) and pass the bands through the corresponding slits on the front board. The goal is to align the straps parallel to the participant’s body.
2.2.2 Strapping and alignment
Once the participant is properly seated, we use the vinyl straps as a preliminary check for chest and abdominal strapping, ensuring proper alignment before tightening. The lower vinyl strap fully encircles the abdomen, and the upper strap covers the chest once the proportions are confirmed. The participant sits upright, and we adjust the straps accordingly, securing the left vinyl strap onto the chest and the abdomen. The right strap is wrapped on top of the left strap and securely attached using Velcro.
2.2.3 Restriction mode configuration
For the dynamic condition, the chosen springs are mounted on the device’s backboard and connected to the tie-down rings using carabiners. For a static restriction, the springs are not used, and the two middle boards, which have rods running through them, are pulled back to their maximum position as restricted by the LVDT. Shaft collars are then attached to secure the wooden boards in place, establishing the static simulation setup.
2.2.4 Load calibration
Once fully strapped and positioned on the device, tension is applied using the metal gear. Adjusting the gear to different levels allows for varying pressure to be applied to the participant’s abdomen and chest. To ensure consistent pressure across participants, a calibration process is conducted. Participants are restricted by the bands and instructed to perform normal breathing for about a minute. Using a live feed of data from the pressure sensors, the minimum pressure values, typically occurring at the final phase of expiration, are analyzed and used as the baseline pressure. If the baseline pressure is lower than the target pressure, the process is repeated: the restriction is tightened further, the participant performs normal breathing for another minute, and the baseline pressure is reassessed. This cycle continues until the baseline pressure matches the target pressure.
2.3 Experimental design
2.3.1 RQ1: breathing restriction - static and dynamic design validation
To validate the capability of the device to enable both static and dynamic restriction within the same design, an experiment was conducted to evaluate how the device affects breathing under these conditions. Six healthy, non-smoking individuals (four males, two females) volunteered to participate and completed the entire study. The mean age of participants was 24.0
The study protocol included three conditions: baseline (without restriction), static restriction, and dynamic restriction (with a load of 45 kg). Participants performed the tasks in a fixed order: baseline condition first, followed by the static restriction and the dynamic restriction. For each condition, pulmonary function testing was conducted to measure forced expiratory volume in one second (FEV1) and forced vital capacity (FVC). At least three tests were performed per condition, ensuring they met the acceptability criteria of the American Thoracic Society and European Respiratory Society, with the highest values recorded. Additionally, after each pulmonary function test, participants were asked to breathe normally for 5 min. Respiratory data, including airflow and mouth pressure, were recorded using a pneumotach connected to a mouthpiece and a two-way non-rebreathing valve (Hans Rudolph).
These measurements provided quantitative metrics to confirm differences between static and dynamic conditions, as indicated by LVDT displacement and respiratory changes. The data were tested for equal variance and normality, revealing non-normal distribution. Consequently, the Kruskal-Wallis test, a non-parametric alternative to ANOVA, was used to analyze differences between conditions, ensuring the validity of the statistical evaluation.
2.3.2 RQ2: reproducible and adjustable loads - pressure variability validation
To evaluate the reproducibility and adjustability of the loads applied to the chest and abdomen, a second experiment was conducted focusing on pressure variability. The built-in pressure sensors of the device were connected to a data acquisition system, enabling real-time monitoring and recording of pressure. One participant was strapped in and instructed to breathe for 1 min, then fully released. This process was repeated three times for each condition (static and dynamic), with pressure data from both the abdomen and chest recorded during each trial.
The pressure signals were analyzed using MATLAB, where the trough of each respiratory oscillation was selected as the fiducial point to measure pressure, as shown in Figure 11. These troughs were identified as the local minima in the pressure signal and used to define a baseline pressure for each breath. The baseline pressure
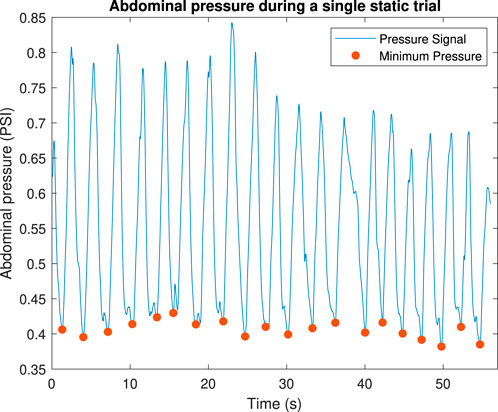
Figure 11. Example of how abdominal pressure varies within a single trial. Dots show the baseline pressure.
This experiment demonstrated the ability of the device to apply consistent and adjustable loads across trials, confirming its reproducibility and flexibility. The use of fiducial points ensures precise calibration and measurement, validating the device’s performance in achieving adjustable and reproducible load conditions.
2.4 Statistical analysis
For all experiments, data were tested for normality using the Shapiro-Wilk test. If data were found to be non-normal, the Kruskal-Wallis test was used to compare differences between conditions. Post hoc pairwise comparisons were performed using Dunn’s test with Bonferroni correction to adjust for multiple comparisons. Statistical significance was set at a p-value of 0.05.
3 Results
3.1 RQ1: static and dynamic restriction validation
The ability of the device to achieve both static and dynamic restriction was validated through respiratory metrics and displacement measurements. Figure 12 summarizes the results for various respiratory metrics across the baseline, static, and dynamic conditions.
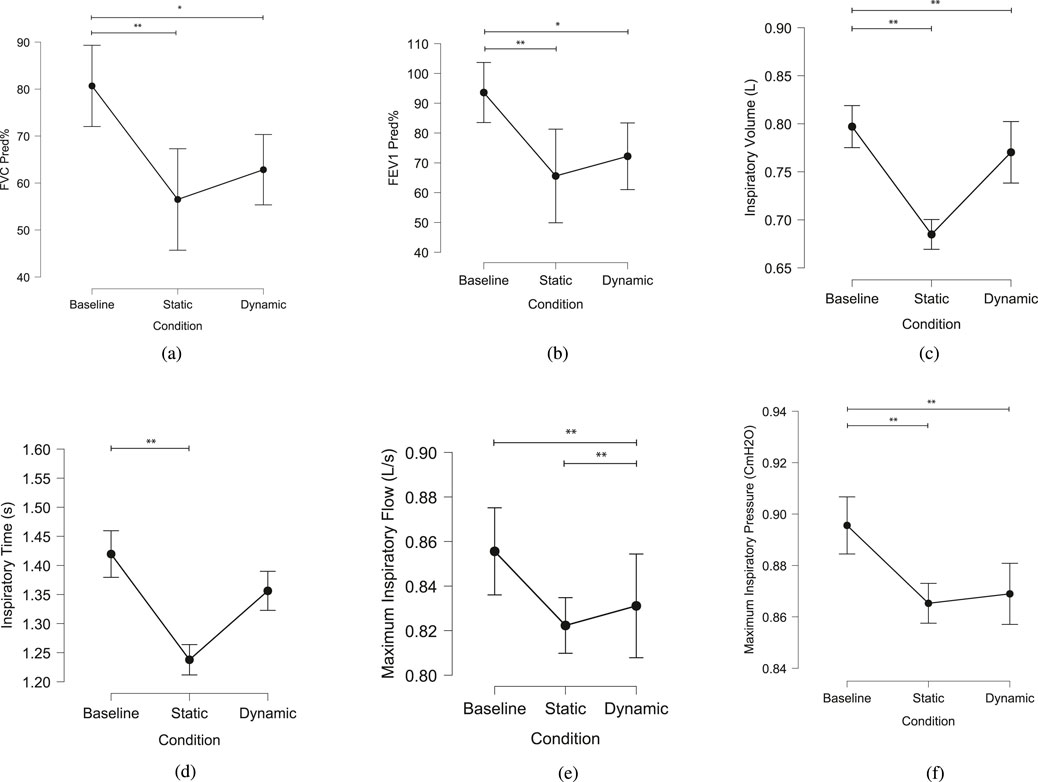
Figure 12. Summary of various respiratory metrics across different conditions: baseline, static, and dynamic. Significant differences between conditions are indicated by asterisks: *p < 0.05, **p < 0.01. (a) FVC Predicted Percent (FVC Pred%) (b) FEV1 Predicted Percent (FEV1 Pred%) (c) Inspiratory Volume (d) Inspiratory Time (e) Average peak inspiratory airflow (f) Average peak inspiratory mouth pressure.
The Kruskal-Wallis test revealed statistically significant differences in FEV1 Predicted % (Figure 12a) among the three conditions (Baseline, Static, and Dynamic), with a p-value of 0.011. Dunn’s Post Hoc Comparisons highlighted significant differences between Baseline and Static (p = 0.004) and between Baseline and Dynamic (p = 0.026), with no significant difference between Static and Dynamic conditions (p = 0.524). Similarly, for FVC Predicted % (Figure 12b), significant differences were observed between Baseline and both Static (p = 0.002) and Dynamic (p = 0.017) conditions, while Static and Dynamic did not differ significantly (p = 0.448).
Additional metrics, including inspiratory volume, inspiratory time, peak inspiratory airflow, and peak inspiratory pressure, also demonstrated significant differences across conditions. Inspiratory volume (Figure 12c) in the static (p < 0.001) and dynamic (p < 0.001) conditions was significantly lower during baseline. No significant difference in inspiratory volume was found between static and dynamic conditions (p = 0.747). Inspiratory time (Figure 12d) in the baseline condition was significantly higher compared to the static condition (p < 0.001). No significant difference was found between the baseline and dynamic conditions (p = 0.453). Peak inspiratory airflow (Figure 12e) did not differ significantly between the baseline and static conditions (p = 0.946). However, maximum inspiratory airflow was significantly higher in the dynamic condition compared to both the baseline (p < 0.001) and static (p < 0.001) conditions. Peak inspiratory pressure (Figure 12f) was significantly higher in the static condition compared to baseline (p = 0.001) and in the dynamic condition compared to baseline (p < 0.001). No significant difference was found between the static and dynamic conditions (p = 0.148).
Figure 13 highlights the displacement of the LVDTs during 1 min for a single participant. During the static condition, minimal movement was observed, with displacements close to zero (approximately
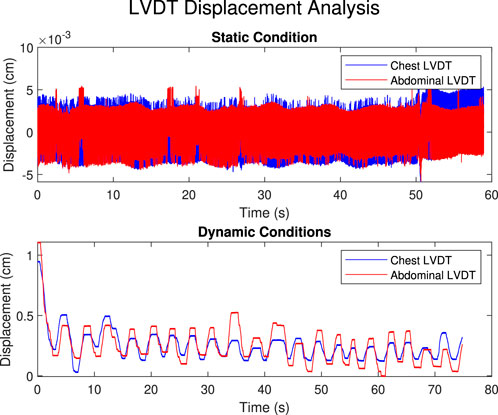
Figure 13. Displacement of the LVDT across static and dynamic conditions for a single participant over a 1-min period. During the static condition, minimal movement is observed, with displacements close to zero (approximately
3.2 RQ2: reproducibility and adjustability of load
The reproducibility and adjustability of the device were validated by analyzing pressure variability across trials for both static and dynamic conditions. Pressure data were recorded for the chest and abdomen, and the results are summarized in Tables 1, 2.
In the static condition (Table 1), chest pressure showed consistent mean values across trials: 3.25 kPa (SD = 0.17), 3.18 kPa (SD = 0.19), and 3.41 kPa (SD = 0.22). Similarly, minimum abdominal pressures were consistent, with means of 2.96 kPa (SD = 0.08), 2.8 kPa (SD = 0.08), and 2.7 kPa (SD = 0.09). In the dynamic condition (Table 2), the minimum chest pressure exhibited a decrease across trials, with mean values of 3.04 kPa (SD = 0.12) in the first trial, 2.76 kPa (SD = 0.39) in the second, and 2.66 kPa (SD = 0.26) in the third. The minimum abdominal pressure also showed slight variations, with mean pressures of 2.69 kPa (SD = 0.08), 2.79 kPa (SD = 0.52), and 2.71 kPa (SD = 0.26).
These results demonstrate the device’s capability to apply reproducible and adjustable loads, supported by consistent pressure measurements across trials for both static and dynamic conditions.
4 Discussion
4.1 RQ1: static and dynamic restriction validation
The findings demonstrate that our CWR device effectively modulates respiratory function, influencing both lung function measures and respiratory mechanics across static and dynamic conditions. The significant differences in FEV1 Predicted% and FVC Predicted% between baseline and the restricted conditions (static and dynamic) indicate that the device successfully induces a restriction (Gonzalez et al., 1999).
To further contextualize these results, we compared baseline pulmonary function values with normative ranges reported in the literature. Prior studies show that FVC values in healthy adults typically range from 4.7 to 5.8 L, and FEV1 ranges from 3.8 to 4.44 L (Miller et al., 2002; Torchio et al., 2006; Cline et al., 1999). In our study, baseline FVC and FEV1 averaged 3.80 L and 3.79 L, respectively—lower than the reported averages but within physiological limits for our sample. Published values under restricted breathing report FVC between 3.66 and 4.2 L and FEV1 between 2.64 and 3.55 L. Our results showed that FVC dropped to 3.01 L (dynamic) and 2.73 L (static) and FEV1 to 2.97 L and 2.72 L, respectively. In terms of percent change under restriction, FVC declined by 20.8% during dynamic loading and 28.2% during static loading, while FEV1 decreased by 21.6% and 28.2%, respectively. These changes are consistent with the average reductions reported in prior work (FVC: 25.1%, FEV1: 24.9%) (Miller et al., 2002; Torchio et al., 2006; Cline et al., 1999). This confirms that the imposed loads replicate physiologically realistic constraints. It is important to notice that our approach uniquely combines chest wall and abdominal restriction and incorporates both static and dynamic loading conditions. Prior studies applied restriction using different methods and typically focused on either a single body region or a single mode of restriction. While this makes direct one-to-one comparisons difficult, the consistency in the direction and magnitude of changes observed across conditions supports the external validity of our device’s effects.
Inspiratory volume, inspiratory time, and maximum airflow showed statistically significant differences between baseline and each restricted condition, further validating the effectiveness of the device. These findings align with previous research on the work of breathing under load and compensatory mechanisms (Zechman et al., 1957; Cain and Otis, 1949; Napoli et al., 2021). The equation that describes work of breathing using airflow, first defined by (Otis et al., 1950) and later updated by (Napoli et al., 2021),
shows that the work of breathing is influenced by tidal volume
The data from the LVDTs further confirm the effectiveness of the static restriction, with virtually zero movement recorded during the static trials, validating the ability of the device to enforce a rigid, non-movable condition. In contrast, the dynamic condition allowed for measurable displacement of both the chest and abdomen, highlighting the device’s ability to simulate dynamic breathing scenarios. These results demonstrate that the CWR device achieves distinct and quantifiable static and dynamic restrictions.
4.2 RQ2: reproducibility and adjustability of load
The experiment designed to measure the variability of pressure exerted on the chest and abdomen showed that in the static condition, both chest and abdominal pressures were highly consistent across repeated trials. This consistency validates the reproducibility of the device’s load application. The data also confirm that the pressure levels can be adjusted and maintained precisely, supporting the adjustability of the device. In the dynamic condition, the pressure and displacement data indicate the device’s ability to allow separate movements of the chest and abdomen, offering a flexible platform to study respiratory mechanics difference between chest and abdominal breathing (Kaneko and Horie, 2012; Hughes, 1979). Further, the combined data from the LVDTs and pressure sensors enable future research on the quantification of breathing patterns, muscle group activation and respiratory effort.
4.3 Future directions
Although this study was conducted in a relatively young adult sample, the physiological effects of chest wall and abdominal restriction may be amplified in older individuals due to age-related changes in the respiratory system. Aging is associated with both reduced chest wall compliance and increased lung compliance, resulting in lower overall respiratory system compliance. Calcification of the costal cartilages, kyphosis, and vertebral deformities contribute to stiffening of the chest wall (Janssens, 2005; Sharma and Goodwin, 2006), while simultaneous degeneration of elastic fibers in the lung parenchyma reduces elastic recoil and increases lung compliance (Arif and Pisani, 2020; Schneider et al., 2021). This combination leads to higher functional residual capacity and air trapping, potentially making external restriction more limiting in older individuals. In addition, inspiratory muscle strength declines with age due to muscle atrophy and neuromuscular changes, with studies reporting 13%–25% reductions in diaphragmatic pressure compared to younger adults (Janssens, 2005; Sharma and Goodwin, 2006). These factors reduce respiratory reserve and increase the work of breathing under load. Blunted ventilatory responses to hypoxia and hypercapnia in older populations further diminish adaptive capacity (Sharma and Goodwin, 2006; Levitzky, 1984). Together, these age-related changes suggest that the physiological effects of the restriction device may be more pronounced in older adults. Future studies should include a broader age range to assess generalizability and potential age-specific responses.
Future research should address the effectiveness of the device across various body types. Although the design includes features to accommodate different body shapes and sizes, extreme body types may challenge full conformity to the device. Enhancing customization options for the device remains critical to improving its adaptability and effectiveness across a broader user base. Additional studies could refine the device to better address the needs of individuals with extreme body sizes.
Further research should also explore the use of the device in populations with respiratory diseases such as asthma. Special attention must be given to avoid triggering bronchoconstriction, and load levels should be carefully adjusted for such populations. Investigating device modifications tailored for individuals with compromised pulmonary function, such as adjustable spring resistance or softer band materials, could expand the device’s applications and improve its safety and efficacy.
Finally, the potential applications of this device extend beyond respiratory function. Future studies could evaluate its utility in assessing cognitive function, muscle fatigue, or blood circulation under restricted conditions, broadening the impact of this novel technology.
5 Conclusion
Our CWR device offers a novel design to study the impact of chest wall impairment on breathing and cognition. It is an improvement on earlier devices, offering improved precision and versatility while allowing for accurate quantification of the load applied to the chest wall and abdomen. Thus, it provides researchers with controlled conditions for exploring the impacts of static and dynamic load-based restrictions on physiological and cognitive processes and modeling breathing dynamics under load (Napoli et al., 2021). Our validation studies have demonstrated significant experimental manipulation in the breathing process. Additional use cases for this device include respiratory training, assisting patients with respiratory conditions such as neuromuscular diseases (Watson et al., 2022; Rodrigues et al., 2022), and reducing the effort required to breathe (Neumannova et al., 2019; Ribeiro Rodrigues et al., 2024b).
Data availability statement
The raw data supporting the conclusions of this article will be made available by the authors, without undue reservation.
Ethics statement
The studies involving humans were approved by University of Florida Institutional Review Board. The studies were conducted in accordance with the local legislation and institutional requirements. The participants provided their written informed consent to participate in this study. Written informed consent was obtained from the individual(s) for the publication of any potentially identifiable images or data included in this article.
Author contributions
VR: Conceptualization, Data curation, Formal Analysis, Investigation, Methodology, Visualization, Writing – original draft, Writing – review and editing. LM: Investigation, Writing – original draft. RZ: Conceptualization, Investigation, Writing – review and editing. PD: Conceptualization, Investigation, Methodology, Resources, Supervision, Writing – review and editing. NN: Conceptualization, Investigation, Methodology, Project administration, Resources, Supervision, Validation, Writing – review and editing.
Funding
The author(s) declare that financial support was received for the research and/or publication of this article. This work was supported by the Office of Naval Research (grant number N00014-22-1-2653).
Conflict of interest
The authors declare that the research was conducted in the absence of any commercial or financial relationships that could be construed as a potential conflict of interest.
Generative AI statement
The author(s) declare that no Generative AI was used in the creation of this manuscript.
Publisher’s note
All claims expressed in this article are solely those of the authors and do not necessarily represent those of their affiliated organizations, or those of the publisher, the editors and the reviewers. Any product that may be evaluated in this article, or claim that may be made by its manufacturer, is not guaranteed or endorsed by the publisher.
Supplementary material
The Supplementary Material for this article can be found online at: https://www.frontiersin.org/articles/10.3389/fmede.2025.1560136/full#supplementary-material
References
Adler, D., and Janssens, J.-P. (2019). The pathophysiology of respiratory failure: control of breathing, respiratory load, and muscle capacity. Respiration 97 (2), 93–104. doi:10.1159/000494063
Altose, M. (2007). Respiratory sensation and control of breathing. Curr. Respir. Med. Rev. 3, 3–6. doi:10.2174/157339807779941730
Andersen, S. J., Arvidsson, U., Fransson, L., Nemcek, K., and Svensson, S. (1992). The relationship between the transfer factor obtained at rest, and arterial oxygen tension during exercise, in patients with miscellaneous pulmonary diseases. J. Intern. Med. 232, 415–419. doi:10.1111/j.1365-2796.1992.tb00607.x
Arif, S., and Pisani, M. A. (2020). Aging and respiratory diseases. U. S. Respir. and Pulm. Dis. 1. doi:10.17925/USPRD.2020.5.1.33
Armstrong, N. C., and Gay, L. A. (2016). The effect of flexible body armour on pulmonary function. Ergonomics 59, 692–696. doi:10.1080/00140139.2015.1084052
Bloomfield, P. M., Green, H., Fisher, J. P., and Gant, N. (2022). Complex cognitive functions and neurovascular coupling are protected by carbon dioxide during hypoxia. FASEB J. 36, fasebj–R2479. doi:10.1096/fasebj.2022.36.s1.r2479
Brannan, S., Liotti, M., Egan, G., Shade, R., Madden, L., Robillard, R., et al. (2001). Neuroimaging of cerebral activations and deactivations associated with hypercapnia and hunger for air. Proc. Natl. Acad. Sci. 98, 2029–2034. doi:10.1073/pnas.98.4.2029
Brown, P. I., and McConnell, A. K. (2012). Respiratory-related limitations in physically demanding occupations. Aviat. Space, Environ. Med. 83, 424–430. doi:10.3357/asem.3163.2012
Cain, C. C., and Otis, A. B. (1949). Some physiological effects resulting from added resistance to respiration. J. Aviat. Med. 20 (3), 149–160.
Chapman, D. G., Berend, N., Horlyck, K. R., King, G. G., and Salome, C. M. (2012). Does increased baseline ventilation heterogeneity following chest wall strapping predispose to airway hyperresponsiveness? J. Appl. Physiology 113, 25–30. doi:10.1152/japplphysiol.01582.2011
Chottidao, M. (2009). Effects of elastic chest wall restriction on lung functionafter aerobic exercise training. Chulalongkorn Med. J. 53, 39–49. doi:10.58837/chula.cmj.53.1.4
Cline, C. C., Coast, J. R., and Arnall, D. A. (1999). A chest wall restrictor to study effects on pulmonary function and exercise: 1. Development and validation. Respiration 66 (2), 182–187. doi:10.1159/000029366
Eberlein, M., Schmidt, G. A., and Brower, R. G. (2014). Chest wall strapping. An old physiology experiment with new relevance to small airways diseases. Ann. Am. Thorac. Soc. 11, 1258–1266. doi:10.1513/annalsats.201312-465oi
Eldridge, F., and Davis, J. M. (1959). Effect of mechanical factors on respiratory work and ventilatory responses to CO2. J. Appl. Physiology 14, 721–726. doi:10.1152/jappl.1959.14.5.721
Fähling, M., and Persson, P. B. (2012). Oxygen sensing, uptake, delivery, consumption and related disorders. Acta Physiol. 205, 191–193. doi:10.1111/j.1748-1716.2012.02432.x
Giuriato, G., Gundersen, A., Verma, S., Pelletier, E., Bakewell, B., and Ives, S. J. (2020). The effects of chest wall loading on perceptions of fatigue, exercise performance, pulmonary function, and muscle perfusion. Sports 8, 3. doi:10.3390/sports8010003
Gonzalez, J., Coast, J. R., Lawler, J. M., and Welch, H. G. (1999). A chest wall restrictor to study effects on pulmonary function and exercise: 2. The energetics of restrictive breathing. Respiration 66 (2), 188–194. doi:10.1159/000029367
Hughes, R. L. (1979). Does abdominal breathing affect regional gas exchange? Chest 76, 288–293. doi:10.1378/chest.76.3.288
Janssens, J.-P. (2005). Aging of the respiratory system: impact on pulmonary function tests and adaptation to exertion. Clin. Chest Med. 26, 469–484. doi:10.1016/j.ccm.2005.05.004
Kaneko, H., and Horie, J. (2012). Breathing movements of the chest and abdominal wall in healthy subjects. Respir. Care 57, 1442–1451. doi:10.4187/respcare.01655
Kesten, S., Garfinkel, S. K., Wright, T., and Rebuck, A. S. (1991). Impaired exercise capacity in adults with moderate scoliosis. Chest 99, 663–666. doi:10.1378/chest.99.3.663
Legg, S. J. (1988). Influence of body armour on pulmonary function. Ergonomics 31, 349–353. doi:10.1080/00140138808966679
Miller, J. D., Beck, K. C., Joyner, M. J., Brice, A. G., and Johnson, B. D. (2002). Cardiorespiratory effects of inelastic chest wall restriction. J. Appl. Physiology 92, 2419–2428. doi:10.1152/japplphysiol.00394.2001
Napoli, N. J., Demas, M., Stephens, C. L., Kennedy, K. D., Harrivel, A. R., Barnes, L. E., et al. (2020). Activation complexity: a cognitive impairment tool for characterizing neuro-isolation. Sci. Rep. 10 (1), 3909. doi:10.1038/s41598-020-60354-2
Napoli, N. J., Rodrigues, V. R., and Davenport, P. W. (2021). Characterizing and modeling breathing dynamics: flow rate, rhythm, period, and frequency. Front. Physiology 12, 772295. doi:10.3389/fphys.2021.772295
Neijens, H. J., Sinaasappel, M., De Groot, R., De Jongste, J. C., and Overbeek, S. E. (1990). Cystic fibrosis, pathophysiological and clinical aspects. Eur. J. Pediatr. 149, 742–751. doi:10.1007/bf01957271
Neumannova, K., Kuzilkova, V., Zurková, M., Hubackova, L., Michalcikova, T., Jakubec, P., et al. (2019). “Respiratory muscle training improves the work of breathing and decreases inspiratory muscle fatigue in patients after lung transplantation,” in Physiotherapists, Lausanne, Switzerland: European respiratory society (Sept).
Otis, A. B., Fenn, W. O., and Rahn, H. (1950). Mechanics of breathing in man. J. Appl. Physiology 2, 592–607. doi:10.1152/jappl.1950.2.11.592
Ribeiro Rodrigues, V., Beres, S. L., Davenport, P. W., and Napoli, N. J. (2024b). “Modeling and analysis of mechanical work of breathing,” in Handbook of AI and data sciences for sleep disorders. Editors R. B. Berry, P. M. Pardalos, and X. Xian (Springer).
Ribeiro Rodrigues, V., Pratt, R. A., Stephens, C. L., Alexander, D. J., and Napoli, N. J. (2024a). Work of breathing for aviators: a missing link in human performance. Life 14, 1388. doi:10.3390/life14111388
Rodrigues, V. R., Olsen, W. L., Sajjadi, E., Smith, B. K., and Napoli, N. J. (2022). Exploring inspiratory occlusion metrics to assess respiratory drive in patients under acute intermittent hypoxia. Respir. Physiology and Neurobiol. 304, 103922. doi:10.1016/j.resp.2022.103922
Roethlisberger, K., Nyilas, S., Riedel, T., Wolfensberger, J., Singer, F., and Latzin, P. (2018). Short-term effects of elastic chest wall restriction on pulmonary function in children with cystic fibrosis. Respiration 96, 535–542. doi:10.1159/000491094
Šakić, K., Pećina, M., and Pavičić, F. (1992). Cardiorespiratory function in surgically treated thoracic scoliosis with respect to degree and apex of scoliotic curve. Respiration 59 (6), 327–331. doi:10.1159/000196082
Schneider, J. L., Rowe, J. H., Garcia-de-Alba, C., Kim, C. F., Sharpe, A. H., and Haigis, M. C. (2021). The aging lung: physiology, disease, and immunity. Cell 184, 1990–2019. doi:10.1016/j.cell.2021.03.005
Selickman, J., and Marini, J. J. (2022). Chest wall loading in the ICU: pushes, weights, and positions. Ann. Intensive Care 12, 103. doi:10.1186/s13613-022-01076-8
Sharma, G., and Goodwin, J. (2006). Effect of aging on respiratory system physiology and immunology. Clin. Interventions Aging 1, 253–260. doi:10.2147/ciia.2006.1.3.253
Stephens, C., Kennedy, K., Napoli, N., Demas, M., Barnes, L., Crook, B., et al. (2017). “Effects on task performance and psychophysiological measures of performance during normobaric hypoxia exposure,” in 19th international symposium on aviation psychology, 202.
Sybrecht, G. W., Garrett, L., and Anthonisen, N. R. (1975). Effect of chest strapping on regional lung function. J. Appl. Physiology 39, 707–713. doi:10.1152/jappl.1975.39.5.707
Torchio, R., Gulotta, C., Ciacco, C., Perboni, A., Guglielmo, M., Crosa, F., et al. (2006). Effects of chest wall strapping on mechanical response to methacholine in humans. J. Appl. Physiology 101, 430–438. doi:10.1152/japplphysiol.00379.2005
Watson, K., Egerton, T., Sheers, N., Retica, S., McGaw, R., Clohessy, T., et al. (2022). Respiratory muscle training in neuromuscular disease: a systematic review and meta-analysis. Eur. Respir. Rev. 31, 220065. doi:10.1183/16000617.0065-2022
Younes, M., Jung, D., Puddy, A., Giesbrecht, G., and Sanii, R. (1990). Role of the chest wall in detection of added elastic loads. J. Appl. Physiology 68, 2241–2245. doi:10.1152/jappl.1990.68.5.2241
Zechman, F., Hall, F. G., and Hull, W. E. (1957). Effects of graded resistance to tracheal air flow in man. J. Appl. Physiology 10, 356–362. doi:10.1152/jappl.1957.10.3.356
Keywords: load-based chest wall restriction, static chest wall restriction, chest wall strapping, breathing impairment, abdominal restriction
Citation: Ribeiro Rodrigues V, Mejia L, Zucchi RG, Davenport PW and Napoli NJ (2025) Chest wall restriction device for modeling respiratory challenges and dysfunction. Front. Med. Eng. 3:1560136. doi: 10.3389/fmede.2025.1560136
Received: 13 January 2025; Accepted: 23 April 2025;
Published: 20 May 2025.
Edited by:
Lorenzo Vannozzi, Sant’Anna School of Advanced Studies, ItalyReviewed by:
Chiara Tonda-Turo, Polytechnic University of Turin, ItalyAndrea Cafarelli, Sant’Anna School of Advanced Studies, Italy
Copyright © 2025 Ribeiro Rodrigues, Mejia, Zucchi, Davenport and Napoli. This is an open-access article distributed under the terms of the Creative Commons Attribution License (CC BY). The use, distribution or reproduction in other forums is permitted, provided the original author(s) and the copyright owner(s) are credited and that the original publication in this journal is cited, in accordance with accepted academic practice. No use, distribution or reproduction is permitted which does not comply with these terms.
*Correspondence: Nicholas J. Napoli, bi5uYXBvbGlAdWZsLmVkdQ==