- Department of Clinical Biochemistry and Pharmacology, Faculty of Health Sciences, Ben-Gurion University of the Negev, Beer-Sheva, Israel
Endothelial cell adhesion molecules have long been proposed as promising targets in many pathologies. Despite promising preclinical data, several efforts to develop small molecule inhibitors or monoclonal antibodies (mAbs) against cell adhesion molecules (CAMs) ended in clinical-stage failure. In parallel, many well-validated approaches for targeting CAMs with nanomedicine (NM) were reported over the years. A wide range of potential applications has been demonstrated in various preclinical studies, from drug delivery to the tumor vasculature, imaging of the inflamed endothelium, or blocking immune cells infiltration. However, no NM drug candidate emerged further into clinical development. In this review, we will summarize the most advanced examples of CAM-targeted NMs and juxtapose them with known traditional drugs against CAMs, in an attempt to identify important translational hurdles. Most importantly, we will summarize the proposed strategies to enhance endothelial CAM targeting by NMs, in an attempt to offer a catalog of tools for further development.
Introduction
Recent failures of advanced nanomedicine (NM) drug candidates (due to lack of efficacy or dose limiting toxicity) (1) inspire a drop in the nanomedicine “hype” (2, 3). Among the reasons for modest success of clinical translation of NM are the poor accumulation of injected nanoparticles at the site of inflammation and solid tumor and the poor tissue penetration of NM (4, 5). One of the proposed strategies to overcome these limitations is to actively direct NM toward the disease-related vasculature via endothelial cell adhesion molecules (eCAMs-for the sake of simplicity we will use the abbreviation CAM) using high-affinity binding ligands. Many of these targets exhibit their function on the endothelium of blood vessels in the vicinity of inflammatory processes and are mainly absent from the non-activated endothelium (6, 7). Furthermore, targeting CAMs eliminates the need for deep tissue penetration, as these targets are directly accessible for NMs in the blood stream.
Structurally, CAMs like selectins (E-, P- and L-selectin), vascular cell adhesion molecule 1 (VCAM-1), intracellular adhesion molecule 1 (ICAM-1), and integrins are transmembrane glycoproteins, and their expression is correlated with the presence of inflammatory signaling via cytokines and chemokines in the proximity of tissue damage. Their main function is to facilitate the process of leukocyte infiltration from the blood stream into the inflamed tissues, by forming bonds with ligands on these circulating cells, allowing for rolling on the endothelium, adhesion and trans-endothelial migration (8, 9). This role makes CAMs crucial in acute response to tissue damage and acute inflammation, however in chronic inflammation excessive or prolonged infiltration of leukocytes can lead to further damage. Binding of NMs to CAMs can improve the vascular uptake and therapeutic efficacy of drug molecules entrapped within, or attached to the nanomedicine formulation. Due to their distinct expression patterns linked to the inflammatory state of the proximate tissue, CAMs are viable disease and progression markers (10, 11), hence NMs targeting CAMs depict a useful tool for vascular imaging (12). Furthermore, successful binding of CAMs can impair their function as anchors supporting leukocyte infiltration, translating to a reduction of inflammation severity. Lastly, presence of CAM ligands on CAR T-cells was shown to improve their homing to tumors or in case of leukemias, to the bone marrow (13). Glycoengineering of the CAR T-cell surface receptors could promote E-selectin binding for improved bone marrow or any inflammatory site homing (14). For these reasons, CAMs are recognized as viable targets for controlling inflammation, especially in the field of NM.
In the present manuscript, we highlight the key studies in which endothelial CAM-targeted NM formulations were explored to improve drug delivery, to enhance vascular imaging or to disrupt CAM-mediated activity in pre-clinical settings, and we discuss how these approaches can be employed to enhance clinical translation. This mini-review will focus on the CAMs that are considered as early markers of endothelial activation and dysfunction, e.g., selectins (E-, P-and L-selectin), ICAM-1 and VCAM-1. Integrins, which are expressed on endothelial cells, but they are also present in a variety of tumor cells, are discussed as a separate group of vascular adhesion molecules.
Physiological Role of CAMs
The major endothelial expressed CAMs can be divided into integrins, selectins (E- and P-selectin), and immunoglobulin superfamily members (ICAM-1, VCAM-1). L-selectin is expressed on the membranes of immune cells (7). The structure of these transmembrane glycoproteins is discussed in detail elsewhere (6, 7) and summarized in Scheme 1.
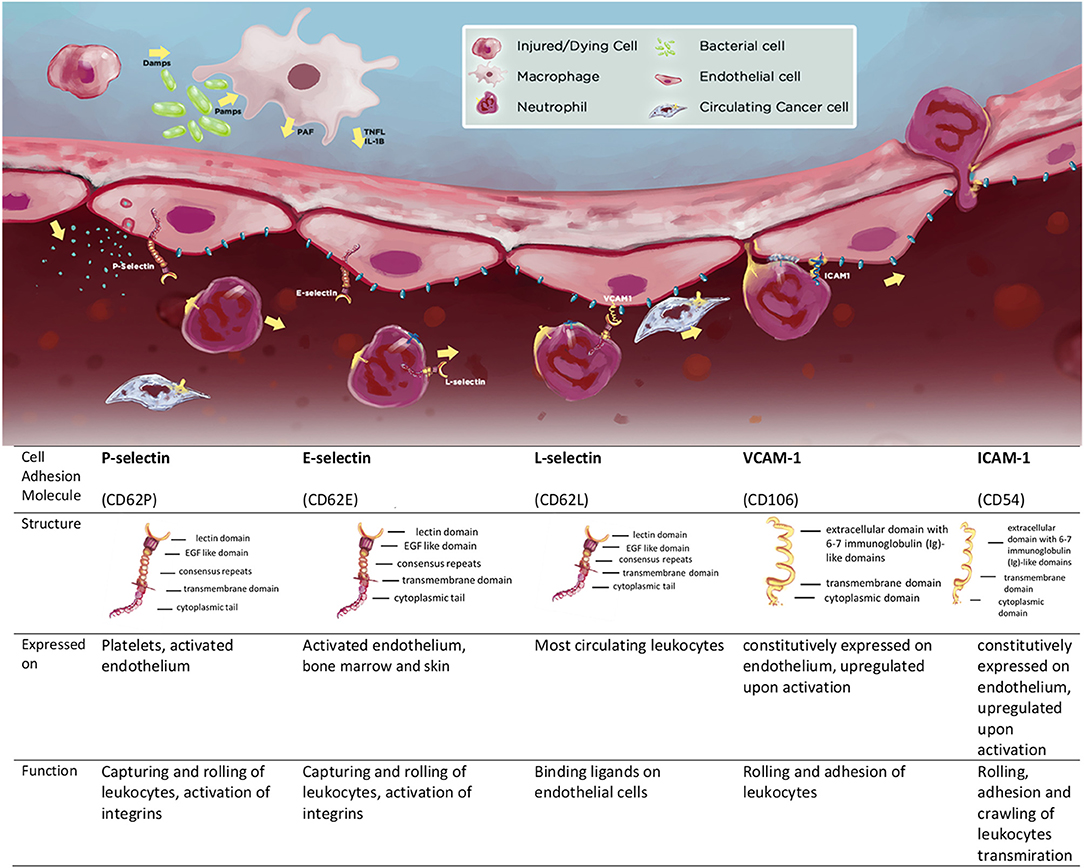
Scheme 1. Cell adhesion molecules (CAMs) P-, E-, and L-selectin, VCAM-1, ICAM-1 and the summary of their structure and function; expression patterns and function.
The main functions of CAMs become apparent following “endothelial activation”- a process of profound changes on the vascular endothelium induced by inflammatory mediators (9). Resident immune cells (such as tissue-resident macrophages) act as “first responders” by releasing inflammatory cytokines that activate endothelial cells and release chemokines to recruit circulating inflammatory cells (8, 9). Once activated, endothelial cells can also produce cytokines and chemokines, stimulating further migration and accumulation of leukocytes in the affected tissues (9). Under non-inflammatory conditions, circulating leukocytes would not adhere to the endothelium, however, upon activation endothelial cells start to express CAMs. In a matter of minutes, as a rapid response to mediators such as histamine or platelet-activating factor (PAF), pre-synthesized CAMs (e.g., P-selectin) are transported to the surface of cells. Other mediators, such as cytokines (TNFα, IL-1β) induce a more gradual activation of endothelial cells including transcriptional induction and synthesis of numerous other CAMs over hours (15). In addition, leukocytes themselves become activated and change the expression patterns of CAMs on their surface (α4β1 also known as very late antigen-4 or VLA-4, and lymphocyte function-associated antigen-1, LFA-1), making them more adhesive to endothelial cells. Together these changes facilitate a multistep process of leukocyte transendothelial migration (Scheme 1). In a defined sequence of events, leukocytes initially form transient connections with selectins on endothelial cells, causing them to slow down and start to roll on their surface. Conformational changes in leukocyte-expressed integrins induce the formation of high-affinity bonds through binding to other CAMs (VCAM-1, ICAM-1) (16). In addition, vasoactive substances from the activated endothelium increase the diameter of the blood vessels, reducing intravascular pressure and increasing blood flow, allowing higher chances for circulating leukocytes to form bonds with CAMs successfully. In the final stage, leukocytes transmigrate through the endothelial cell layer, basement membrane, and pericytes, and reach the tissue. Once there, these leukocytes collaborate with tissue-resident macrophages to exert their effector functions (17). Usually, after the trigger for the inflammation is eliminated, the inflammatory process is directed to resolution. This crucial step can be absent or altered in several chronic immune-mediated inflammatory diseases (IMID e.g., rheumatoid arthritis, psoriasis, and Crohn's disease), where CAMs play a critical role (18). They stay up-regulated in the inflamed tissues, over a prolonged period of time, while the extent of their presence is linked to the severity of the disease. The excessive recruitment of leukocytes by CAMs can propagate the inflammatory process and, in many cases, aggravate tissue injury (18).
Pathological Conditions With CAM Implications
Leukocyte adhesion mediated by CAMs is crucial in (patho)physiological events such as acute inflammation, immune response, wound repair, and hemostasis (7, 8, 15, 19). More recently, their role in chronic inflammation, promoting cancer metastases, and other pathological processes has been described in more detail (20, 21). This led researchers to emphasize the ambiguity of CAMs, for example, selectins, as having the nature of Dr. Jekyll and Mr. Hyde (11). The “good” face represents the physiological role of selectins, while the “evil” one becomes dominant whenever the leukocyte adhesion is dysregulated, as during chronic inflammation. This imbalance is well described in IMIDs such as rheumatoid arthritis, Chron's disease, and in other chronic cardiovascular or neurological diseases (18, 22).
The implications of CAMs in pathologies were extensively studied and confirmed on several levels. In some cases, CAMs show elevated plasma levels of their soluble forms in patients suffering from chronic diseases: soluble E-selectin in inflammatory bowel disease, bronchial asthma, atopic dermatitis (10) as well as in hypertension, diabetes, and hyperlipidemia (23); soluble P-selectin in cardiovascular diseases, especially in deep vein thrombosis (24). Not only are soluble VCAM-1 and ICAM-1 elevated in the plasma of breast cancer patients (25), but higher VCAM-1 serum levels in patients with epithelial ovarian cancer were also linked with disease progression and metastasis (26). There is evidence that elevated levels of E-selectin and ICAM-1 may also serve as molecular markers for atherosclerosis and the development of clinical coronary heart disease (27, 28).
Secondly, tissue up-regulation of CAMs was confirmed in clinical samples taken from patients suffering from various inflammatory diseases. Elevated levels of E-selectin were detected in liver sections of patients with alcoholic liver disease, bronchial biopsies in chronic bronchitis, and synovium in rheumatoid arthritis (10). Patients with sickle cell disease (SCD) express higher levels of P-selectin, which is thought to contribute to the adhesion of sickle red blood cells to the endothelium (29, 30). Higher VCAM-1 expression was, for example, associated with higher eosinophil infiltration into the inflamed lungs of asthmatic patients, rejection of liver allografts, the severity of RA, and cancer progression (31).
Furthermore, animal disease models are providing valuable insight into CAM involvement in pathological processes. E-selectin was identified as a target in rodent models of asthma, myocardial infarction, stroke, and inflammatory bowel disease (IBD) (10, 32). VCAM-1 was upregulated in a model of experimental allergic encephalomyelitis (33) and aortic sections in collagen-induced murine model or rheumatoid arthritis (34).
Finally, drugs that exert anti-inflammatory effects, especially on the level of the vasculature, decrease the levels of CAMs and leukocyte adhesion. Statins reduce VCAM-1 protein levels and E-selectin mRNA levels in vitro in TNFα activated HUVECs (35, 36), as confirmed in hypercholesterolemic patients (37). Therapy with methotrexate (MTX) reduced expression levels of E-selectin and VCAM-1 in synovial tissues of patients with rheumatoid arthritis (38). Similarly, some natural products, like herbal of fungal extracts, can reduce expression of CAMs on activated endothelial cells (39, 40). Also, interventions that influence vascular health and reduce endothelial inflammation, like smoking cessation (sICAM-1) (41) and physical exercise (E-selectin, ICAM-1) (42), have been shown to reduce CAM plasma levels.
Together, these findings indicate that CAMs are viable pharmacological targets in conditions where leukocyte infiltration is dysregulated.
Drug Candidates-Inhibitors of CAMs: mAbs and Small Molecules
Successful examples of clinically approved inhibitors of CAMs are limited to three monoclonal antibodies (mAbs): natalizumab and vedolizumab, which target leukocyte expressed α4β7 integrins and are approved for the treatment of IBD (43) and crizanlizumab (anti-P-selectin mAb)-approved in 2019 for vaso-occlusive crisis (VOC) in SCD (29). Table 1 summarizes some examples of CAMs inhibitors that were tested in clinical trials (CTs) and their implications in human diseases. Another mAb against P-selectin, inclacumab, is currently being developed for therapy of VOC in SCD (44). Aselizumab (anti-L-selectin mAb) was developed for controlling the infiltration of activated neutrophils into different inflamed organs. It was expected that targeting L-selectin, expressed on all leukocytes, would lead to better systemic effects than blocking regionally expressed E- or P-selectin. Clinical trial results showed a lack of efficacy, even though the saturation of leukocyte L-selectin was 89%. Possible explanations could be that the remaining 10% of L-selectin could still be sufficient to mediate adhesion or that other CAMs could compensate for L-selectin blockage (45, 46). The murine anti-ICAM-1 mAb, enlimomab, reached clinical-stage development for stroke treatment, following the rationale that ICAM-1 blockade inhibits reperfusion-induced inflammation with neuronal injury after stroke. Even though preclinical studies demonstrated a reduction in the extent of reperfusion injury, clinical results showed negative effects on patient survival and symptom-free recovery (47). Besides mAbs, another biological CAM inhibitor was tested. Recombinant P-selectin glycoprotein ligand IgG fusion protein (YSPSL) failed in CTs for protecting allograft function after transplantation, which stopped further development (48, 49).
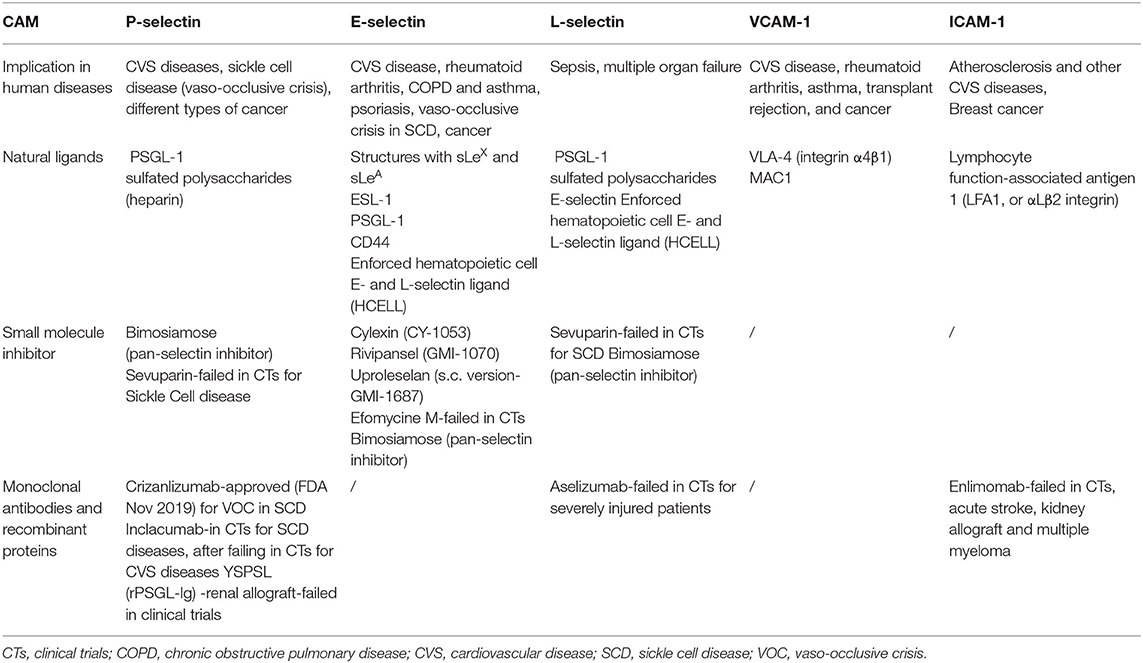
Table 1. Cell adhesion molecules (CAMs) P-, E-, and L-selectin, VCAM-1, ICAM-1 and their implications in human diseases; natural ligands, and low Mw inhibitors/monoclonal antibody drug candidates.
Apart from monoclonal Abs, many attempts were made to develop small molecule inhibitors of CAMs. An array of natural products isolated from plant material (terpenoids, lignans etc.), or from microbial (efomycine M) or marine origin, have initially demonstrated in vitro inhibition of CAMs, however, no further clinical development can be identified (50). Employing the rational design-driven approach, a series of glycomimetic inhibitors were derived from the structure of the selectin ligand sialyl Lewis X (sLeX). One of the first analogs of sLeX, pentasaccharide cylexin (CY-1053), was discontinued due to poor efficacy in CTs for reperfusion injury (51), likely due to the low metabolic stability and rapid clearance associated with the carbohydrate structure (11). “Second-generation” pan-selectin inhibitor bimosiamose (binding to E-selectin, P-selectin, and L-selectin) has minimal carbohydrate structural motives and was developed for the treatment of psoriasis and airway inflammation (52–55). Even though inhalation of bimosiamose induced a reduction of airway inflammation in chronic obstructive pulmonary disease (COPD) patients, it appears that it was discontinued (49, 56). Rivipansel (GMI-1070), another pan-selectin inhibitor (more active on E-selectin), is being developed for the treatment of SCD. In a phase III CT, rivipansel did not meet the efficacy endpoints (57), however, the post-hoc analysis of trial results revealed that patients treated with rivipansel shortly after VOC onset had benefited from the therapy. This led the FDA to grant a Rare Pediatric Disease designation for rivipansel to treat SCD in pediatric patients. Another glycomimetic, uproleselan (GMI-1271), a specific E-selectin inhibitor, is being investigated as an add-on therapy to standard chemotherapy in acute myeloid leukemia (58) and as antithrombotic treatment (59). Similarly, another anti-adhesive polysaccharide drug candidate, sevuparin (non-anticoagulant low molecular weight heparinoid), failed to improve clinical outcomes in patients with SCD (57, 60).
Interestingly, even though VCAM-1 is established as a viable target in immunological disorders and cancer (31), no human or humanized mAb or low molecular weight (Mw) inhibitor is being tested in clinics.
Research and development of these CAM inhibitors provided valuable insights on the complexity of future drug candidate development. Some of the lessons could be summarized as:
1. Inhibition of leukocyte infiltration by blocking CAMs is a viable strategy for limiting inflammation, as validated by the success of natalizumab and vedolizumab.
2. The clinical translation of several small molecule CAM inhibitors has stagnated either due to the insufficient binding affinity (glycomimetics), unfavorable pharmacokinetics (glycomimetics), lack of clinical efficacy (glycomimetics, anti ICAM-1, L-selectin, P-selectin mAbs) or potential immunogenicity (mAbs). Additionally, in the case of aselizumab it was postulated that even if the drug achieves the blockage of the majority of targeted CAMs, there is the possibility of compensatory activity of other CAMs that can diminish inhibitory effects on leukocyte infiltration.
3. Choosing the right pathological process (indication) matters. Blocking P-selectin was not successful in CTs for cardiovascular diseases, but later it proved to be a good strategy in a specific niche: VOC in SCD.
While the CAM-binding molecules alone showed insufficient efficacy, they could pose a functional part of a NM system. Using NM carriers with affinity ligands against CAMs could simultaneously provide both blocking of these leukocyte-anchors, which would potentially limit inflammation, and a possibility to deliver therapeutic cargo in a targeted manner right to the inflamed site. Attachment of multiple targeting ligands would improve the binding efficiency and the macromolecular nature of NM could improve the pharmacokinetic (PK) properties and stability, providing an answer to several issues that led to failure of mAbs and small molecule inhibitors of CAMs.
Nanomedicines Targeting CAMs
In parallel with the development of mAbs and small molecule CAM inhibitors, many research groups aimed to develop CAM targeted NMs. Compared to small molecule CAM inhibitors and traditional small molecule anti-inflammatory or chemotherapeutic drugs, the advantages of NM are: a. better PK profile - prolonging half-life time in the circulation b. solubility enhancement of poorly water-soluble drugs c. improved stability d. delivery of the drug to the target site e. controlled release of the drug at the target tissue. Compared to mAbs, NMs that block CAM function possess: a. no/reduced immunogenicity b. multivalency c. the potential of steric shielding of cells. These goals can be achieved by the properties of NMs (macromolecular size and easy chemical modification) and can be further improved in order to target selected cells in the desired tissue by constructing NMs bearing multiple targeting ligands.
Targeting CAMs with affinity NMs can pursue several therapeutic goals (Figure 1A):
a. Targeted drug delivery to the inflamed endothelium - using CAM-targeted carriers bearing a. chemotherapeutic drugs to be delivered to the vicinity of tumors, or b. anti-inflammatory drugs for suppression of inflammation.
b. Imaging - using NM imaging probes targeted toward CAMs for imaging of a. cancer vasculature or b. inflamed vasculature.
c. Functional blocking - using “drug-free” NM (without cargo) to bind CAMs and block their function, in order to: a. inhibit leukocyte trafficking into the inflamed tissues or b. Inhibit cancer cells homing to the pre-metastatic niche and metastasis formation.
An array of different nanocarriers (e.g., liposomes, polymer-based NPs, magnetic NPs, dendrimers, quantum dots, Figure 1B) have been used for designing and constructing CAM-targeted NMs (61).
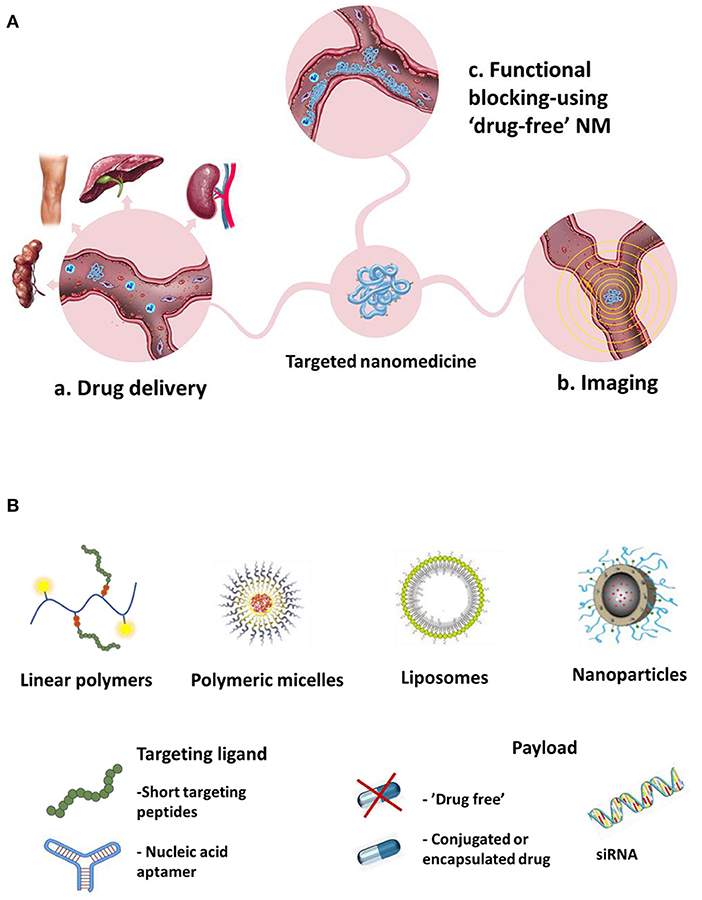
Figure 1. Different proposed goals of CAMs-targeted NM (A) Different systems used for targeting CAMs, types of targeting ligands and payloads (B).
a. Drug Delivery Systems
Localization of CAMs at the inflamed endothelium makes them attractive targets for drug delivery both to tumors and sites of inflammation of various pathologies. Accordingly, different CAM-targeted systems were developed to carry chemotherapeutic and anti-inflammatory drugs. Targeting ligands employed in these systems vary in their structural complexity, binding affinity, and potential to induce adverse effects. More details on the chemical/structural properties of these systems can be found in reviews by Muzykantov's group (61, 62).
One of the earliest approaches was to utilize oligosaccharides, the natural ligands of CAMs as ligands. Liposomes decorated with sLex, a tetrasaccharide molecule present on the terminus of selectin glycoprotein ligands, were developed for selective binding to E-selectin and delivery of therapeutic agents to activated endothelium (63–65). Apart from targeting drugs to the tumor vasculature (66–68), such systems were used for the delivery of anti-inflammatory drugs in order to control inflammation in the eye or in inflamed joints (69, 70). In addition to liposomes, other drug-delivery systems (DDS) with conjugated sLex were reported, including polyethyleneoxide-b-polybutadiene (PEO-b-PBD) block copolymers and poly(D,L-lactide) (PLA) nanoparticles (71, 72). Due to the structural complexity of sLex and its complicated synthesis, several sLex mimicking structures (3'-(1-carboxy)ethyl (3'-CE)) or quinic acid (Qa)-based mimetics were used to decorate the surface of liposomes or were conjugated to an N-(2-hydroxypropyl)methacrylamide (HPMA) copolymer, respectively, as selectin targeting ligands, and exhibited higher uptake into activated endothelial cells (73, 74). The natural product polysaccharide fucoidan has also been proposed as a targeting ligand for P-selectin and employed in NM development, both for cancer drug delivery (75) and for inflammation control (76).
Monoclonal antibodies bind CAMs with higher affinity and selectivity compared to carbohydrate-based ligands, and therefore have been investigated as potential targeting moieties on DDS. Liposomes decorated with anti-E-selectin Ab were utilized for selective delivery of dexamethasone (DEX) to the inflamed kidneys in a murine model (77). Similar systems, targeting E-selectin or VCAM-1, were used for short interfering RNA (siRNA), cytotoxic (78, 79) or anti-inflammatory drug delivery (80). Anti-ICAM-1-targeted immunoliposomes were also utilized for delivery specifically to injured tissues in a rat paw inflammation model (81) and in lung injury model (82). To overcome the disadvantages of antibodies, such as the potential immunogenicity of the Fc fragment, and their relatively high molecular weight, some research groups have used antibody fragments as affinity ligands (61), however not many examples of advanced systems can be identified.
However, high “antibody-like” binding affinity can also be achieved with short targeting peptides. As NM ligands, peptides possess several advantages, mainly owing to their small size - low immunogenicity, stability, easy manufacturing and low cost (83). For example, our group utilized a short high-affinity E-selectin binding peptide (Esbp, DITWDQLWDLMK), identified in (84)) for targeted drug delivery of a cytotoxic drug (Doxorubicin, DOX) or a pro-apoptotic peptide (D(KLAKLAK)2, KLAK) to tumor vasculature. The hydrophilic HPMA-based copolymer bearing multiple copies of Esbp showed high binding affinity (at low nanomolar range) and selectivity to activated endothelial cells (85). The polymer-DOX conjugate (P-Esbp-DOX) demonstrated selective cytotoxicity toward E-selectin-expressing vascular endothelial cells that was 150-fold higher compared to a non-targeted polymer (85). In vivo, the E-selectin targeted polymer-drug conjugates (P-Esbp-DOX and P-Esbp-KLAK) decreased the rate of tumor growth and prolonged the survival of mice bearing primary Lewis lung carcinoma, or established melanoma (B16-F10) lung metastases (86). P-Esbp-DOX was also proven to be safe and highly efficacious in treating mice with established colorectal cancer liver metastases [unpublished data]. In addition to tumor targeting, this system was employed by our group for targeted delivery of an anti-inflammatory drug (DEX) to inflamed atherosclerotic plaques, to prevent cardiac remodeling and atherosclerosis (87). Esbp-modified bovine serum albumin (BSA) nanoparticles or Esbp-hyaluronic acid-paclitaxel (Esbp-HA-PTX) micelles were used by other groups to target DEX or PTX to acute lung injury or to inhibit breast cancer metastasis, respectively (88, 89). Other sequences such as IELLQAR (shown to bind to E-selectin and with much lower affinity to P- and L-selectin) (90–92), YRNWFGRW and YRNWDGRW (93) have been proposed as well for selective targeting of E-selectin. Interestingly, a study by Fernandes et al. directly compared all of these peptides for their ability to bind E-selectin. Their results suggest that Esbp is the ideal candidate for NM development, as it binds E-selectin better than the IELLQAR sequence and with better specificity when compared to the other two sequences (94).
Other short, high-affinity peptides were incorporated into NMs for targeting VCAM-1 [sequences VHPKQHR(GGSKGC) and cyclic FLDVRK (cyclo(MePhe-Leu-Asp-Val-D-Arg-D-Lys)) reviewed in (12)], P-selectin [sequences EWVDV (95–97) and LVSVLDLEPLDAAWL (98)] and ICAM-1 (cyclo(1,12)PenITDGEATDSGC (99) (Table 2).
Similar to short peptides, short sequences of nucleotides, commonly referred to as DNA or RNA aptamers were also developed for targeting CAMs. A thioaptamer targeting E-selectin (ESTA-1) (109) demonstrated selective binding with nanomolar binding affinity, and was able to target porous silicon particles to the tumor vasculature in a breast cancer xenograft model, endothelium of bone marrow or atherosclerotic plaque (104–106). Conversely, other groups reported that they couldn't confirm ESTA-1 binding to human E-selectin in their assays (110) and identified a new high affinity E- and P-selectin binding aptamer (SDA). These discrepancies could serve as a warning of difficulties in the translation of these ligands among different systems, laboratory facilities, and types of NM.
CAM-targeting of NM can also be achieved through usage of cell-membrane fragments of cells known to engage with CAMs. Liang et al. (107) used the presence of α4 integrin on the surface of macrophages to generate membrane-coated liposomes that could bind to VCAM-1 and effectively deliver drug payload to lung metastases.
Recently, a new approach for generating targeting ligands on NMs emerged from the advances in genetical engineering. Park et al. (108) developed VCAM-1 targeted NM by coating poly(lactic-co-glycolic acid) (PLGA)-based NPs with membrane fragments of cells expressing the ligand for VCAM-1. They chose a cell line which expresses β1 integrin, and modified it to express integrin α4, which together form a complex, VLA-4, a ligand for VCAM-1. This NM was loaded with DEX and used for drug delivery to inflamed lungs and suppressing inflammation.
b. Imaging
Targeting of imaging probes to endothelial CAMs in cancerous and inflammation sites holds promise to improve management of these conditions. Nano-sized imaging probes of diverse shape, materials and physical properties have been developed over the years to improve the visualization of pathological sites by intravital microscopy (IVM), positron emission tomography (PET), magnetic resonance imaging (MRI) and ultrasound (US) modality in pre-clinical settings (111). Selected examples of CAMs-targeted NM imaging probes are listed in Table 3. In principle, imaging of vascular inflammation can be easily achieved with ligand-targeted imaging probes, since CAMs on the activated endothelium are readily accessible for circulating nanomaterials.
Indeed, a variety of NM systems were developed for diagnostic purposes. These molecular probes are designed to bind to CAMs allowing early detection of inflammatory lesions, and metastases formation, as well as disease progression and treatment monitoring. Most of such systems are directed at imaging of atherosclerotic lesions. VCAM-1 is the most exploited target, followed by E- and P-selectin (Table 3). As seen with NMs for drug delivery, first systems used mAbs and carbohydrates as targeting ligands. In more recently developed systems, researchers shifted to using short high-affinity targeting peptides or in a few cases, nucleotide aptamers, due to the already discussed favorable characteristics. The best example for this development could be the evolution of VCAM-1 targeting. Motivated by the sub-optimal performances of mAb targeted NMs for VCAM-1 imaging, Kelly et al. (120) employed a modified phage display approach, identifying a panel of binding peptides. One of the sequences with the highest binding affinity, a cyclic peptide VHSPNKK (VNP), was homologous to very late antigen-4 (VLA4), a counterpart of VCAM-1. In vitro, the peptide was shown to bind to VCAM-1 with 12-fold higher target-to-background ratios comparing to anti-VCAM-1 mAb. Magneto-fluorescent NPs modified with this peptide were tested in vivo. Interestingly, in their following work, the authors conducted further phage-display studies with in vivo atherosclerotic plaques, and identified a linear sequence VHPKQHR (VINP), with even higher binding affinity. In direct comparison of VNP and VINP, the latter was shown to bind VCAM-1 with 20 times higher affinity (123). After conjugation to magneto-fluorescent NP, this NM demonstrated excellent binding and uptake into endothelial cells and signal enhancement in a TNFα inflammation model, atherosclerotic ApoE−/− mice and human atheroma samples.
c. Functional Blockade of Immune and Cancer Cell Homing
The proinflammatory and disease promoting function of CAMs, their upregulation and hence increased impact on the course of disease have placed CAMs among viable targets for controlling pathological cell migration processes by functional receptor blockade. Accomplishments with mAbs and small molecule inhibitors are briefly summarized in this review.
However, the CAM-blocking properties of NMs targeted to CAMs have just recently entered the spotlight of scientific interest. The presence of targeting ligands on NMs can be utilized not only to direct a drug or dye cargo, but to functionally block CAM-mediated activity. These “drug-free macromolecular therapeutics” present an alternative to the discussed small molecule inhibitors and Abs recognizing CAMs and are summarized in Table 4.
The choice of carrier systems and ligands, as well as the design of ligand presentation, determine the blocking efficacy. Especially the multivalent presentation of ligands such as carbohydrates, which often possess relatively weak binding affinity, displays an advantage over small molecules or mAbs, which have only one or few binding sites (146, 147). The significantly higher binding affinity achieved by multiple copies of the selectin ligands sLeX or sLea conjugated to a linear backbone, such as polyacrylamide (PAA) or HPMA, compared to monovalent sLeX/sLea, illustrates the potential of multivalent systems to target CAM-expressing cells more efficiently than their monovalent counterparts (133–135). The same concept applies to other, non-natural ligands such as glycomimetic (134–136) or non-carbohydrate ligand analogs (Table 4) (74, 85).
Besides higher binding affinity, the use of NMs for functional blockade reveals another advantage over non-polymeric inhibitors, which is the shielding from cell-cell interactions by nanosized inhibitors. The binding of CAMs to ligands on flexible polymeric carriers sterically hinders the interaction of surrounding adhesion molecules with their ligands and therefore increases the inhibitory potential of the NM (137, 148, 149). This was extensively investigated for P- and L-selectin targeted dendritic polyglycerol sulfates (dPGS), for which increasing size, as well as increasing valency, are associated with higher inhibitory activity (137, 138). Similar dPGS system was also employed for drug delivery, through binding to P-selectin on both the activated endothelium and cancer cells expressing high levels of this marker (glioblastoma multiforme) (150).
Indeed, several of these “drug-free” CAM-targeted NMs have proven their therapeutic potential in pre-clinical research (147, 151, 152). sLeX- or other glycomimetic ligand-bearing polymer treatment reduces neutrophil and/or macrophage infiltration in rodent models of inflammation, such as peritoneal inflammation (136, 139), liver injury (140) and dermatitis (138). In a murine model of polymyositis, the infiltration of pro-inflammatory T-cells was blocked by dPGS, targeting their L-selectin mediated recruitment (141).
High-affinity peptides as targeting moieties, have been investigated for targeted drug delivery and imaging, but also possess desirable properties for the functional blockade of CAMs. In our research, we demonstrated that an HPMA-based copolymer carrying multiple copies of Esbp inhibits neutrophil capture and endothelial transmigration (142). The strong inhibition of E-selectin function, without the involvement of anti-inflammatory drugs, is an effective strategy to reduce alcohol-induced liver injury (142) or high fat diet-promoted cardiac remodeling (87) in apolipoprotein E knockout (ApoE−/−) mice. P-Esbp reduced atherosclerotic lesions in these mice, stabilized atherosclerotic plaques and reduced the total leukocyte count in the blood stream, as a response to less inflammation (87). Similarly, P-Esbp treatment caused a reduction of E-selectin expression in inflamed livers in mice (post ethanol feeding), indicating a sustainable reduction of inflammation (142). Along in the same lines stand results of neutrophil adhesion blockade achieved by three different peptides, respectively attached to a dermatan sulfate backbone, binding to E- and P-selectin, as well as, to a lesser extent, to L-selectin and ICAM-1 (90, 143). These glycopolymer-peptide conjugates reduce thrombus size in a deep vein thrombosis model by inhibiting platelet activation (143).
In our other studies, we have shown that P-Esbp effectively inhibits the cell-cell interaction with E-selectin ligand-expressing B16 melanoma cells (86). A pretreatment with P-Esbp prevented the extravasation and tissue colonization of circulating cancer cells, which resulted in a significant decrease of established lung metastasis colonies in mice (86). Accordingly, we have designed an HPMA copolymer bearing the laminin α5-derived peptide A5G27 (P-A5G27), which specifically binds CD44v3 and CD44v6, two subtypes of the selectin ligand CD44, which are expressed on invasive cancer cells (144). We confirmed that P-A5G27 reduces cell migration in vitro and inhibits cancer cell metastasis of murine 4T1 breast cancer cells in vivo.
Blocking of ICAM-1 by the short cyclic peptide cLABL, was investigated for interfering with immunological synapses between T-cells and antigen presenting dendritic cells (DCs). Binding of PLGA NPs decorated with cLABL decreased T-cells conjugation to DCs, inhibiting the activation of T-cells, much more effectively than the free cLABL peptide (145).
Already discussed anti-VCAM-1 aptamer conjugated SPIONs, apart from demonstrating favorable tumor imaging ability, were also investigated for tumor growth suppression (131). When injected along with anti-IL-4Rα aptamer-conjugated SPIONs to tumor bearing mice, these two NMs significantly decreased tumor size comparing to control or single NM-treated animals, indicating that simultaneous functional blockade of these targets potentiates antitumor activity. Similarly, ESTA-1 thioaptamer was shown to block adhesion of sLeX positive cells to endothelium (109). However, in addition to drug delivery this thioaptamer is being developed as a stand-alone treatment (153), probably owing to the improved serum stability and to our best knowledge no ESTA-1 drug-free NM was reported.
d. Other Vascular-Targeted Nanomedicines
A separate class of CAMs are integrins, a group of heterodimeric transmembrane receptors (composed of one α- and one β-subunit) with various functions in cell-cell and cell-extracellular matrix interactions. This family of receptors can be divided into several groups, based on their ligand specificity: endothelial specific receptors, leukocyte-specific receptors, laminin-binding receptors, and collagen-binding receptors (154). In contrast to the other groups of discussed CAMs, integrin expression is not limited to the endothelium, but they are present inside many other tissues, and their expression on the leukocyte surface makes them another regulator of leukocyte infiltration (155).
RGD-binding integrins were named for their affinity to tripeptide Arginine-Glycine-Aspartic acid (RGD) and are present at elevated levels on a variety of different cancer cells (156). Their blockade via RGD-based ligands has been demonstrated beneficial for cancer therapy, and a great variety of NMs with RGD-based targeting are extensively reviewed elsewhere (155, 157, 158). In order to improve binding affinity, selectivity and stability, several modifications of RGD peptides were reported, including cyclization, incorporation of D-amino acids and N-methylation (156). While many integrins are involved in the regulation of vascular functions, only few are predominantly expressed on the vascular endothelium (159, 160) therefore only a small number of systems is designed to target specifically endothelium expressed αvβ3 and αvβ5 integrins (Table 5). Integrin αvβ3 and αvβ5 are upregulated on the tumor neovasculature and critically associated with angiogenesis. In addition to discontinued mAb drug candidate, Etaracizumab (166), several targeted nanomedicine were developed for imaging and drug delivery purposes. Earliest attempts include using the anti-αvβ3 antibody LM609 conjugated to the surface of paramagnetic liposomal nanoparticles for tumor imaging (162) or utilizing small molecule integrin αvβ3 inhibitor on the surface of cationic nanoparticles for gene delivery to tumor endothelium (163). Through lead optimization of the integrin antagonist compounds, Xie et al. developed an antagonist with superior binding to the previously used one and conjugated it in a multiple manner to lipid nanoparticles. These NPs accumulated in the tumor angiogenic vessels in a murine model of squamous cell carcinoma (164).
Several drug-loaded nanoparticles decorated with cyclic iRGD (CRGDKGPD) peptides have been investigated as DDS for cancer therapy, which bind to vascular integrins and additionally to neuropilin-1 (161). iRGD-decorated indocyanine green-loaded liposomes, which target endothelial integrins αvβ3 and αvβ5, were developed for imaging and imaging-guided phototherapy (167).
Besides cancer therapy, DDS targeting αvβ5 via RGD-peptide have demonstrated therapeutic antiangiogenic efficacy for the treatment of choroidal neovascularization (168). Recent in vitro results confirm the contribution of a multivalent ligand presentation for the inhibition of angiogenesis, using drug-free antiangiogenic peptide GHSDVHK-decorated micelles (165).
Strategies and Considerations for Designing Cam-Targeted NMs
Whether or not an actively targeted NM will demonstrate high selectivity for the diseased tissue, will depend on the binding avidity of the NM toward its target and on the selective localization and the degree of up-regulation of the target itself. Certain CAMs can be present at un-desired locations (selectins in skin or bone marrow or on platelets) or are insufficiently up-regulated at the desired site (8, 11). Increasing the site-specific accumulation of NMs can sometimes be achieved by strategical use of dual or simultaneous targeting or enhancement of CAM expression.
a. Dual or Simultaneous Targeting of Multiple CAMs
If we consider the physiological functions of CAMs in leukocyte adhesion and tissue transmigration, it is intuitively apparent that binding multiple CAMs can ensure better affinity of NMs, leading to superior efficacy. The notion of potential binding synergism mainly was investigated in molecular ultrasound imaging by targeted microbubbles (MBs) (169, 170).
Weller et al. reported the development of ultrasound contrast MBs with targeting moieties against both ICAM-1 (anti-human-ICAM-1 antibody) and selectins (sLeX) with the aim to improve imaging of inflamed vasculature. These dual-targeted MBs demonstrated 20 % higher adhesion to activated endothelial cells under flow, than MBs bearing either anti-ICAM-1 Ab or sLeX alone (169). Similarly, dual-targeted MBs bearing mAb against VCAM-1 and a sLeX−decorated PAA polymer for selectin targeting were developed by Ferrante et al. In a series of flow chamber experiments, the dual-targeted MBs were compared to single targeted microbubbles. At higher shear stress conditions, the dual-targeted MBs out-performed both of the single-targeted MBs (170). Additional steps toward obtaining ‘leukocyte-like' NMs were achieved by Yan et al. who developed VCAM-1/ICAM-1/selectin targeted MBs by integrating VCAM-1 and ICAM-1 antibodies, and synthetic polymeric sLex onto the MBs surface. Triple-targeted MBs achieved 3-fold higher binding to activated endothelial cells compared to single and dual-targeted MBs. In vivo data confirmed the advantageous effects of triple-targeting in ultrasound molecular imaging of atherosclerotic plaques of ApoE-deficient mice (171).
In addition to imaging, multiple-targeting was proposed for general drug delivery to the inflamed endothelium. Using model polystyrene particles decorated with sLeX and/or anti-ICAM-1 Ab, Fromen et al. confirmed the synergy of dual targeting and demonstrated 3-7-fold increase in adherence compared to single-targeted particles (172).
Another example of multiple targeting leukocyte-mimicking particles are polymerosomes - synthetic analogs of leukocytes assembled from block copolymers and functionalized for simultaneous targeting of ICAM-1 and selectins, by anti-ICAM-1 and sLex, respectively (71). More recently, lipid NPs incorporating leukocyte plasma membrane proteins termed leukosomes were developed. These structures were shown to preferentially target inflamed murine endothelium in models of LPS-induced ear inflammation (173, 174), atherosclerotic plaques or breast cancer (175) and were able to deliver DEX to the tissues (173). Among other cell membrane proteins, ligands for ICAM-1, VCAM-1, E- and P-selectin were incorporated in the leukosome lipid bilayer and were crucial for tumor accumulation (175).
b. Enhancement of CAM Expression With Pharmacological or Physical Agents
Radiation
Radiation therapy has been proven to be an effective modality in cancer treatment, however, it is non-specific, and its effects cannot be limited to malignant cells. Radiation-induced damage to the surrounding endothelium can in fact change the elasticity and functionality of cancer vasculature (176), increase the vascular permeability (177) and activate endothelial cells causing an overexpression of various CAMs (178–181). Apart from in vitro and mouse model data, these changes were confirmed in cancer patients (182).
This change in the expression of endothelial CAMs was exploited to further enhance the targeting of cancer vasculature by NMs. First systems including anti-ICAM-1 targeted microspheres (183) or iron oxide microparticles (184) showed several folds higher binding to irradiated endothelial cells compared to control cells. Anti-E-selectin antibody-conjugated immunoliposomes were utilized for the delivery of the vascular-damaging drug combretastatin A4, to MCa-4 mouse mammary tumors (185). The basal expression of E-selectin in these tumors was shown to be low, however, it was significantly upregulated after irradiation, allowing for the preferential delivery of immunoliposomes and tumor growth delay.
Radiation-guided endothelial targeting can be achieved with other targeting ligands, besides antibodies. Quinic acid analogs as synthetic mimetic of selectin ligands, were used for decorating PLGA nanoparticles (Qa-NP), yielding NMs which bind to E- and P-selectin (186). To investigate biodistribution, mice bearing CT26 colon cancers in both hind limbs underwent treatment of 6 Gy X-irradiation in only one tumor. Confocal imaging with immunofluorescence staining confirmed the upregulation of E- and P-selectin in irradiated tumors and greater accumulation of QA-NP. Polysaccharide fucoidan, which exhibits nanomolar affinity to P-selectin, was utilized for preparing a nanoparticle carrier for a chemotherapeutic drug. When injected in mice bearing bilateral flank LLC tumors, this system exhibited 3.8-fold higher fluorescence signal in irradiated over non-irradiated tumors (187). Interestingly, an increase in P-selectin expression was also detected in non-irradiated tumors, however to a lesser extent than in irradiated ones, indicating an immune system-mediated activation of distant sites.
Apart from radiation, other physical agents, such as heat were shown to induce up-regulation of CAMs mostly in the tumor vasculature (188), without affecting non-activated endothelium (189). However, we did not identify specific NMs which exploit this approach.
Pharmacological Agents
Considering the inflammation-inducible nature of CAMs, the most obvious intervention that could enhance their presence on the blood vessel endothelium is administration of pro-inflammatory cytokines. However, systemic administration of such cytokines would lead to overall inflammation and, given their complex effects on many receptors and multiple tissues, unpredictable adverse effects. For example, TNFα, although named for its ability to shrink certain tumors in animal models, turned out to have a rather complex effect on tumor growth and a potential for supporting tumor progression (190). Furthermore, systemic administration of TNFα induced a dose limiting toxicity, a condition termed “septic shock like syndrome” (21, 191).
A more tailored approach includes using known drugs, with defined safety profiles that have been shown to up-regulate CAMs at the sites of inflammation. Imiquimod, an agonist of toll-like receptor 7 (TLR-7) was shown to induce E-selectin expression on previously negative human squamous cell carcinomas (SCC) (21, 192), which promoted T-cell infiltration. This finding could be employed for directing E-selectin targeted NMs to tumor vasculature, but given that imiquimod is a topical drug further modalities of application should be investigated in order to cover a broader spectrum of applications.
Another pharmacological intervention exploits the connection between CAM expression and angiogenesis. As it is known that angiogenic factors inhibit the expression of CAMs (ICAM-1 and VCAM-1) (21), some studies looked into effects of angiogenesis inhibitors on vascular CAM expression. Anginex, a synthetic anti-angiogenic peptide-drug candidate, significantly reduced the downregulation of ICAM-1, VCAM-1 and E-selectin on endothelial cells exposed to angiogenic factors (193). Similar results were obtained by different angiogenesis inhibitors such as SU6668-Orantinib (194) and angiostatin (195), validating this approach. This class of drugs is being developed for tackling neoangiogenesis, a hallmark of cancer, and the effect on CAM expression could be exploited for targeting NMs for achieving synergistic tumor growth inhibition.
Combination of Factors
Upregulation of ICAM-1 in cervical cancer cell lines can be achieved by irradiation alone, however combining gamma irradiation with the pharmacological agent retinoic acid leads to an additive effect, superior to either agent alone (196). Possibly, this approach of testing different rational combinations of agents can yield the most efficient strategies for enhanced NM targeting.
Limitations and Risks of Using CAMs as Targets for NMs
Although CAM-targeted NMs have shown promising therapeutic results in pre-clinical settings, there are challenges that hamper their clinical translation. Targeting success depends on several parameters, including relative expression of CAMs in diseased and normal tissues, binding affinity and avidity, and target trafficking and turnover. Moreover, the properties of the carrier molecule itself or ligand determine the stability, biodistribution, general toxicity, and safety of the vascular-targeted system, making it difficult to predict the feasibility and clinical efficacy of endothelial CAM-targeted NM formulations for the treatment of cancer or inflammatory disorders.
a. Endothelial CAM Expression Levels
Since pathological factors modulate CAM expression in the disease site, it is important to pre-determine the target-CAM's expression level, both in the diseased tissue and in normal tissues, as this ratio may play a significant role in the success of drug delivery. In case the CAM is almost exclusively expressed on the inflamed endothelium at high density (i.e., E-selectin), a system with high binding affinity may give better outcomes. Moreover, if the target is expressed at low levels on vascular endothelium under physiological conditions, and upregulated in disease (e.g., ICAM-1) - a system with “marginal” avidity might be sufficient to anchor only to cells with pathological levels of the marker. Indeed, a controlled reduction of the ligand surface density on the carrier enhanced the selectivity of targeting to inflamed pulmonary vasculature in animal models, as determined by PET imaging (197, 198).
b. Lack of Efficacy Due to Compensation
Targeting only one CAM alone may be insufficient in controlling disease as observed in a clinical trial of L-selectin neutralizing Ab (aselizumab), mentioned above. In these patients, the lack of efficacy was reported, even though very high level of L-selectin blockade on neutrophils was achieved (46). A possible explanation could be that inactivity of L-selectin was compensated by the synthesis of active E- and P-selectin ligands. The problem of CAM family member compensation can theoretically be circumvented with a pan-selectin antagonist. Bimosiamose (reported to prevent P-, E-, and L-selectin-mediated adhesion in vitro) has shown promise as potential therapeutic for human diseases, albeit the success was limited, and its development appears to be discontinued. Since bimosiamose functions primarily by blocking E-selectin-mediated adhesion, it probably did not fully block L- and P-selectin activity and the remaining selectins could still be sufficient to mediate adhesion. Alternatively, it's possible that the other CAMs (ICAM-1, VCAM-1) could compensate for selectin blockage. Thus, a Pan-selectin antagonist in combination with blocking other CAMs should be considered for effective therapies for the prevention of leukocyte infiltration in inflammatory conditions.
c. Adverse Effects Arising From the Mechanism
Given that CAMs play an essential role in leukocyte traffic into inflamed tissues, interfering with CAM functions by blocking their mediated activity may pose a risk. Neutrophils play a crucial role in the resolution of inflammation and blocking their activity might delay the healing process (18, 199). A disturbed balance of the neutrophil pool might also increase the risk of infections, and change neutrophil levels in some organs other than the affected tissue. Indeed, in a Phase II trial of traumatized patients with aselizumab, the incidence of infections was higher in the treatment group (46).
A further concern with CAM blockade and infection rates was identified in a drug blocking a counterpart of CAMs. Efalizumab, an mAb against integrin αLβ2 (LFA-1, ligand for ICAM-1), reached the market for psoriasis treatment. In consistence with the mechanism of action, this mAb induced a marked increase in peripheral WBC count, due to blocking their migration into tissues. Additionally, it precipitated T-cells hyporesponsivness, through an effect on α4β1 integrin, which correlated with reduced ability to produce an immune response (200). These finding raised a question of safety of similar drugs and their potential to promote infections. Indeed, clinical trials confirmed increased susceptibility to bacterial infections with efalizumab therapy. Longer treatments, unfortunately, revealed an association to a potentially life-threatening condition - progressive multifocal leukoencephalopathy (PML) caused by a reactivation of JC polyomavirus. This rare serious side effect led to the removal of efalizumab from the market. Similar issues have been risen with another mAb against α4β1 (VLA-4, ligand for VCAM-1) natalizumab. Soon after being approved for treatment of multiple sclerosis in 2004, it was withdrawn from the market due to cases of PML. Following further risk-benefit analysis, natalizumab was re-introduced under a special prescription program. Since then, this drug was approved for the treatment of Crohn's disease by FDA (not by EMA). Interestingly, another integrin-blocking mAb, Vedolizumab, which binds to α4β7 integrins did not show potential to induce PML (200).
Another concern regarding blocking CAMs can be predicted in tumor treatment. Blockade or attenuation of CAM-mediated activity can interfere with or decrease the metastatic spread of cancer, and this indeed prevented the seeding of circulating melanoma cells in the lungs of mice (86). Yet, the number of tumor-infiltrating cytotoxic lymphocytes (mainly CD3+CD8+ T lymphocytes), which negatively correlates with cancer severity, can also be decreased and this in turn may hamper the antitumor immune response (21, 201, 202). Irradiation and pharmacological intervention have been proposed as strategies to enhance CAM expression in tumors with low basal expression, leading to better drug accumulation. Yet, circulating cancer cells utilize CAMs to promote their own trafficking to the pre-metastatic niche and seeding in secondary organs. Thus, upregulation in CAM expression during irradiation might contribute to, rather than inhibit cancer progression, and this should be taken into account. This emphasizes the complexity and the need for precision in local irradiation, or the necessity for a personalized approach to get the best therapeutic outcome.
These examples illustrate the potential serious adverse effects of non-selective inhibition of blood leukocytes' interaction with endothelium and their activation.
The challenge for the future is to closely characterize roles of different CAMs in each pathology and further identify leukocyte subpopulations and their trafficking molecules that have a beneficial role. A CAM-targeted NM that accumulates preferentially in the inflamed tissue and reduces, but not completely blocks neutrophil infiltration might be beneficial, as demonstrated in the ability of P-Esbp to attenuate excessive inflammation in injured liver tissue (142). Such systems limit the blockade of CAMs only to the specific disease sites and could lower the risk of generalized adverse effect. This will help to prevent collateral effects (infections, tissue injury, or metastasis) and will leave protective leukocyte extravasation and immune surveillance unaffected.
d. Binding Affinity and Avidity
Features of the carrier itself, such as size, ligand valence, density, and carrier geometry, determine the binding affinity of the system to CAMs. High ligand densities on the surface of NPs, liposomes or polymer could be beneficial regarding the increase of binding affinity for the vascular endothelium, however they might be difficult to achieve. An efficient system should also exhibit high specificity to the target tissue, and high serum stability, to demonstrate a clear in vivo benefit.
Several CAM-targeted NMs demonstrated enhanced therapeutic efficiency over non-targeted counterparts in pre-clinical settings but failed to demonstrate clinical advantage (83). One of the limitations of immunoliposomes is the fast clearance by the reticuloendothelial system, especially of liposomes heavily decorated with antibody (or Ab fragment), and as a result decreased accumulation the target tissue. Small high-affinity non-antibody ligands (small glycomimetics, short peptides or aptamers) are less expensive and should demonstrate favorable clinical benefit for some of these new NMs.
e. Target Trafficking, Intracellular Fate and Toxicity
The structure of the nano-carrier and drug release features determine the sub-cellular fate, therapeutic activity, and duration of the effects of the cargoes. Following binding to CAMs, the system can be released from the target, remain surface bound, or be internalized, depending on the carrier properties. The binding of certain ligands to CAMs can cause receptor-mediated internalization. This is the desired outcome for targeting drugs to selected cells. In contrast, for blocking the interactions mediated by CAMs, or for imaging purposes, internalization is not desired. The selected carrier must be biocompatible, with minimal side effects and preferentially accumulate at its target site.
Comprehensive research needs to be done to optimize factors such as drug-release rates, internalization, subcellular fate in cell culture, as well as pharmacokinetics, biodistribution and toxicity in naive animals. Studies must be also designated to titrate the optimal dosage, time window of administration, and time intervals for repeated injections of the attached/encapsulated therapeutic molecules. All these issues apply to all new drugs or drug delivery systems, and thus, they are not unique to CAM-targeted NMs. Yet, they are of special importance and will determine the outcome of the study (as demonstrated by the effect on patients treated with rivipansel) (57).
Future Perspectives
To date, a vast number of NM targeting CAMs has been developed and tested preclinically with various theranostic goals. No such system is close to regulatory approval, even though several non-NM drugs targeting CAMs have successfully found their therapeutic niche. We highlighted potential strategies that could help to maximize the potential advantages of NM and help clinical translation (Scheme 2).
A close reading of up-to-date literature signals us that future successful CAM-targeting NMs will be systems with a biocompatible carrier, have multivalent display of low Mw high-affinity ligands (peptide or aptamer), target two or several CAMs, with or without a conventional drug payload, depending on their therapeutic purpose. All of the critical elements (size of the carrier, type of the targeting ligand and ligand density, drug loading) should be investigated in order to find the optimal conditions to assure enhanced binding avidity, with minimal off-target effects, optimal PK properties, and avoid immune clearance. Furthermore, this NM could be combined with a pharmacological or physical agent that could potentiate the expression of the target CAM at the disease site, leading to improved targeting.
Choosing the relevant indication is another level of complexity. The roles of target CAMs should be well established and close attention should be paid to the possibility of compensatory up-regulation of other non-target CAMs as a result of successful targeting. We believe that NM translation should be directed first at diseases of those organs where NMs tend to accumulate (liver, kidneys), as this increases the chance of good clinical outcomes. Alternatively, CAM targeting could be combined with the local administration of NMs.
New inspiration comes from an array of “drug-free” macromolecular therapeutics for CAM targeting as an alternative to unsuccessful low-Mw drug inhibitors. These systems lack the presence of drug payload, which eliminates the potential for inducing drug-related adverse effects.
All of the building elements for CAM-targeted NM are well studied and an array of systems confirmed their preclinical efficacy. We are looking forward to seeing their clinical translation in the following years.
Author Contributions
NM and MR were involved with drafting and writing the review manuscript, and including tables and figures. AD was involved in planning and writing this manuscript. All authors contributed to the article and approved the submitted version.
Funding
The laboratory of AD is supported by Grant No. 1115/19 from the Israel Science Foundation (ISF), Grant No. 2017200 given by the United States–Israel Binational Science Foundation (BSF), by Grant No. 3-15008 from the Israeli Ministry of Health (MOH) and Grant No. 85698 from the Israeli Ministry of Science and Technology (MOS). MR is grateful to the Minerva fellowship for financial support.
Conflict of Interest
The authors declare that the research was conducted in the absence of any commercial or financial relationships that could be construed as a potential conflict of interest.
Publisher's Note
All claims expressed in this article are solely those of the authors and do not necessarily represent those of their affiliated organizations, or those of the publisher, the editors and the reviewers. Any product that may be evaluated in this article, or claim that may be made by its manufacturer, is not guaranteed or endorsed by the publisher.
Abbreviations
ApoE−/−, apolipoprotein E knockout; BSA, bovine serum albumin; CAM, cell adhesion molecule; COPD, chronic obstructive pulmonary disease; CTs, clinical trials; CVS, cardiovascular disease; DDS, drug delivery system; DEX, dexamethasone; DOX, doxorubicin; Esbp, E-selectin binding peptide; ESTA-1, E-selectin thioaptamer 1; HPMA, N-(2-hydroxypropyl)methacrylamide; IBD, inflammatory bowel disease; ICAM-1, intracellular adhesion molecule 1; mAbs, monoclonal antibodies; MBs, microbubbles; MPIO, microparticles of iron oxide; MW, molecular weight; NM, nanomedicine; PAA, polyacrylamide; PAF, platelet-activating factor; PLGA, poly(lactic-co-glycolic acid); PTX, paxlitaxel; Qa, quinic acid; RA, rheumatoid arthritis; RGD, arginine-glycine-aspartic acid; SCD, sickle cell disease; sLea, sialyl LewisA; sLex, sialyl LewisX; SPIONs, superparamagnetic iron oxide nanoparticles; TNFα, tumor necrosis factor alpha; USPIO, ultrasmall superparamagnetic iron oxide particles; VCAM-1, vascular cell adhesion molecule 1; VOC, vaso-occlusive crisis.
References
1. Ledford H. Bankruptcy of nanomedicine firm worries drug developers. Nature. (2016) 533:304–5. doi: 10.1038/533304a
2. Lammers T, Ferrari M. The success of nanomedicine. Nano Today. (2020) 31:100853. doi: 10.1016/j.nantod.2020.100853
3. Wilhelm S, Tavares AJ Dai Q, Ohta S, Audet J, Dvorak HF, et al. Analysis of nanoparticle delivery to tumours. Nat Rev Mater. (2016) 1:1–12. doi: 10.1038/natrevmats.2016.14
4. Lammers T. Kiessling F, Hennink WE, Storm G. Drug targeting to tumors: principles, pitfalls and (pre-) clinical progress. J Controlled Release. (2012) 161:175–87. doi: 10.1016/j.jconrel.2011.09.063
5. Zhang YR, Lin R, Li HJ. He Wl, Du JZ, Wang J. Strategies to improve tumor penetration of nanomedicines through nanoparticle design. Wiley Interdiscip Rev Nanomed Nanobiotechnol. (2019) 11:e1519. doi: 10.1002/wnan.1519
6. McEver RP. Selectins: lectins that initiate cell adhesion under flow. Curr Opin Cell Biol. (2002) 14:581–6. doi: 10.1016/S0955-0674(02)00367-8
7. Smith CW. 3. Adhesion molecules and receptors. J Allergy Clin Immunol. (2008) 121:S375–9. doi: 10.1016/j.jaci.2007.07.030
8. Kreuger J, Phillipson M. Targeting vascular and leukocyte communication in angiogenesis, inflammation and fibrosis. Nat Rev Drug Discov. (2016) 15:125. doi: 10.1038/nrd.2015.2
9. Moreira MB, Garcia-Cardeña G, Saffi MAL, Libby P. Endothelium: A Coordinator of Acute and Chronic Inflammation, in Endothelium and Cardiovascular Diseases. 2018. Elsevier. pp. 485–491. doi: 10.1016/B978-0-12-812348-5.00032-5
10. Jubeli E, Moine L, Vergnaud-Gauduchon J, Barratt G. E-selectin as a target for drug delivery and molecular imaging. J Control Release. (2012) 158:194–206. doi: 10.1016/j.jconrel.2011.09.084
11. Tvaroška I, Selvaraj C, Koča J. Selectins—The two Dr. Jekyll and Mr Hyde faces of adhesion molecules—a review. Molecules. (2020) 25:2835. doi: 10.3390/molecules25122835
12. Ailuno G, Baldassari S, Zuccari G, Schlich M, Caviglioli G. Peptide-based nanosystems for vascular cell adhesion molecule-1 targeting: a real opportunity for therapeutic and diagnostic agents in inflammation associated disorders. J Drug Deliv Sci Technol. (2020) 55:101461. doi: 10.1016/j.jddst.2019.101461
13. Sackstein R. The first step in adoptive cell immunotherapeutics: assuring cell delivery via glycoengineering. Front Immunol. (2019) 9:3084. doi: 10.3389/fimmu.2018.03084
14. Mondal N, Silva M, Castano AP, Maus MV, Sackstein R. Glycoengineering of chimeric antigen receptor (CAR) T-cells to enforce E-selectin binding. J Biol Chem. (2019) 294:18465–74. doi: 10.1074/jbc.RA119.011134
15. Nourshargh S, Alon R. Leukocyte migration into inflamed tissues. Immunity. (2014) 41:694–707. doi: 10.1016/j.immuni.2014.10.008
16. Vestweber D. How leukocytes cross the vascular endothelium. Nat Rev Immunol. (2015) 15:692–704. doi: 10.1038/nri3908
17. Silva MT. When two is better than one: macrophages and neutrophils work in concert in innate immunity as complementary and cooperative partners of a myeloid phagocyte system. J Leukoc Biol. (2010) 87:93–106. doi: 10.1189/jlb.0809549
18. Hopkin SJ, Lewis JW, Krautter F, Chimen M, McGettrick HM. Triggering the resolution of immune mediated inflammatory diseases: can targeting leukocyte migration be the answer? Front Pharmacol. (2019) 10:184. doi: 10.3389/fphar.2019.00184
19. Behm B, Babilas P, Landthaler M, Schreml S. Cytokines, chemokines and growth factors in wound healing. J Eur Acad Dermatol Venereol. (2012) 26:812–20. doi: 10.1111/j.1468-3083.2011.04415.x
20. Zhao H, Wu L, Yan G, Chen Y, Zhou M, Wu Y, et al. Inflammation and tumor progression: signaling pathways and targeted intervention. Signal Transduct Targeted Ther. (2021) 6:1–46. doi: 10.1038/s41392-021-00658-5
21. Harjunpää H, Llort Asens M, Guenther C, Fagerholm SC. Cell adhesion molecules and their roles and regulation in the immune and tumor microenvironment. Front Immunol. (2019) 10:1078. doi: 10.3389/fimmu.2019.01078
22. Kuek A, Hazleman BL, Östör AJ. Immune-mediated inflammatory diseases (IMIDs) and biologic therapy: a medical revolution. Postgrad Med J. (2007) 83:251–60. doi: 10.1136/pgmj.2006.052688
23. Roldán V, Marín F, Lip G, Blann AD. Soluble E-selectin in cardiovascular disease and its risk factors. Thromb Haemost. (2003) 90:1007–20. doi: 10.1160/TH02-09-0083
24. Ramacciotti E, Blackburn S, Hawley AE, Vandy F, Ballard-Lipka N, Stabler C, et al. Evaluation of soluble P-selectin as a marker for the diagnosis of deep venous thrombosis. Clin Appl Thromb Hemost. (2011) 17:425–31. doi: 10.1177/1076029611405032
25. O'hanlon D, Fitzsimons H, Lynch J, Tormey S, Malone C, Given H. Soluble adhesion molecules (E-selectin, ICAM-1 and VCAM-1) in breast carcinoma. Eur J Cancer. (2002) 38:2252–7. doi: 10.1016/S0959-8049(02)00218-6
26. Tas F, Karabulut S, Serilmez M, Ciftci R, Duranyildiz D. Clinical significance of serum epithelial cell adhesion molecule (EPCAM) and vascular cell adhesion molecule-1 (VCAM-1) levels in patients with epithelial ovarian cancer. Tumor Biology. (2014) 35:3095–102. doi: 10.1007/s13277-013-1401-z
27. Hwang SJ, Ballantyne CM, Sharrett AR, Smith LC, Davis CE, Gotto Jr AM, et al. Circulating adhesion molecules VCAM-1, ICAM-1, and E-selectin in carotid atherosclerosis and incident coronary heart disease cases: the Atherosclerosis Risk In Communities (ARIC) study. Circulation. (1997) 96:4219–25. doi: 10.1161/01.CIR.96.12.4219
28. Steyers CM, Miller FJ. Endothelial dysfunction in chronic inflammatory diseases. Int J Mol Sci. (2014) 15:11324–49. doi: 10.3390/ijms150711324
29. Blair HA. Crizanlizumab: first approval. Drugs. (2020) 80:79–84. doi: 10.1007/s40265-019-01254-2
30. Matsui NM, Borsig L, Rosen SD, Yaghmai M, Varki A, Embury SH. P-selectin mediates the adhesion of sickle erythrocytes to the endothelium. Blood. (2001) 98:1955–62. doi: 10.1182/blood.V98.6.1955
31. Kong DH, Kim YK, Kim MR, Jang JH, Lee S. Emerging roles of vascular cell adhesion molecule-1 (VCAM-1) in immunological disorders and cancer. Int J Mol Sci. (2018) 19:1057. doi: 10.3390/ijms19041057
32. Ulbrich H, Eriksson EE, Lindbom L. Leukocyte and endothelial cell adhesion molecules as targets for therapeutic interventions in inflammatory disease. Trends Pharmacol Sci. (2003) 24:640–7. doi: 10.1016/j.tips.2003.10.004
33. Gimenez MAT, Sim JE, Russell JH. TNFR1-dependent VCAM-1 expression by astrocytes exposes the CNS to destructive inflammation. J Neuroimmunol. (2004) 151:116–25. doi: 10.1016/j.jneuroim.2004.02.012
34. Denys A, Clavel G, Lemeiter D, Schischmanoff O, Boissier MC, Semerano L. Aortic VCAM-1: an early marker of vascular inflammation in collagen-induced arthritis. J Cell Mol Med. (2016) 20:855–63. doi: 10.1111/jcmm.12790
35. Greenwood J, Mason JC. Statins and the vascular endothelial inflammatory response. Trends Immunol. (2007) 28:88–98. doi: 10.1016/j.it.2006.12.003
36. Rasmussen LM. Hansen PR, Nabipour MT, Olesen P, Kristiansen MT, Ledet T. Diverse effects of inhibition of 3-hydroxy-3-methylglutaryl-CoA reductase on the expression of VCAM-1 and E-selectin in endothelial cells. Biochem J. (2001) 360:363–70. doi: 10.1042/bj3600363
37. Koh KK, Son JW, Ahn JY, Jin DK, Kim HS, Choi YM, et al. Vascular effects of diet and statin in hypercholesterolemic patients. Int J Cardiol. (2004) 95:185–91. doi: 10.1016/j.ijcard.2003.05.018
38. Dolhain R, Tak P, Dijkmans B, De Kuiper P, Breedveld F, Miltenburg A. Methotrexate reduces inflammatory cell numbers, expression of monokines and of adhesion molecules in synovial tissue of patients with rheumatoid arthritis. Br J Rheumatol. (1998) 37:502–8. doi: 10.1093/rheumatology/37.5.502
39. Lammel C, Zwirchmayr J, Seigner J, Rollinger JM, de Martin R. Peucedanum ostruthium Inhibits E-Selectin and VCAM-1 Expression in Endothelial Cells through Interference with NF-κB Signaling. Biomolecules. (2020) 10:1215. doi: 10.3390/biom10091215
40. Madan B, Mandal B, Kumar S. Ghosh B. Canscora decussata (Roxb) Schult (Gentianaceae) inhibits LPS-induced expression of ICAM-1 and E-selectin on endothelial cells and carageenan-induced paw-edema in rats. J Ethnopharmacol. (2003) 89:211–6. doi: 10.1016/S0378-8741(03)00281-2
41. Scott DA, Stapleton JA, Wilson RF, Sutherland G, Palmer RM, Coward PY, et al. Dramatic decline in circulating intercellular adhesion molecule-1 concentration on quitting tobacco smoking. Blood Cells Mol Dis. (2000) 26:255–8. doi: 10.1006/bcmd.2000.0304
42. Saetre T, Enoksen E, Lyberg T, Stranden E, Jørgensen J, Sundhagen J, et al. Supervised exercise training reduces plasma levels of the endothelial inflammatory markers E-selectin and ICAM-1 in patients with peripheral arterial disease. Angiology. (2011) 62:301–5. doi: 10.1177/0003319710385338
43. Hindryckx P, D'Haens G. Biological Therapy of Crohn's Disease: Natalizumab, Vedolizumab, and Anti-MadCAM, in Crohn's Disease and Ulcerative Colitis. Springer (2017). p. 375–80.
44. Mayer C, Cooper DS, Redfern A, Geng X, Shi J, van Zutphen-van Geffen M, et al. Preliminary Results of a Phase 1 Study in Healthy Subjects Administered Inclacumab, a Fully Human IgG4 Anti-P-Selectin Monoclonal Antibody in Development for Treatment of Sickle Cell Disease. Blood. (2021) 138(Suppl 1):977. doi: 10.1182/blood-2021-153370
45. Seekamp A, van Griensven M, Rusu C, König J, Khan-Boluki J, Redl H. The effect of anti-L-selectin (Aselizumab) on the posttraumatic inflammatory response in multiply traumatized patients. Eur J Trauma. (2005) 31:557–67. doi: 10.1007/s00068-005-1066-4
46. Seekamp A, Van Griensven M, Dhondt E, Diefenbeck M, Demeyer I, Vundelinckx G, et al. The effect of anti-L-selectin (aselizumab) in multiple traumatized patients—Results of a phase II clinical trial. Crit Care Med. (2004) 32:2021–8. doi: 10.1097/01.CCM.0000142396.59236.F3
47. Investigators E.A.S.T., Use of anti-ICAM-1 therapy in ischemic stroke: results of the Enlimomab Acute Stroke Trial. Neurology. (2001) 57:1428–34. doi: 10.1212/WNL.57.8.1428
48. Osama Gaber A, Mulgaonkar S, Kahan BD, Steve Woodle E, Alloway R, Bajjoka I, et al. YSPSL (rPSGL-Ig) for improvement of early renal allograft function: a double-blind, placebo-controlled, multi-center Phase IIa study 1, 2, 3. Clin Transplant. (2011) 25:523–33. doi: 10.1111/j.1399-0012.2010.01295.x
49. Smith BA, Bertozzi CR. The clinical impact of glycobiology: targeting selectins, Siglecs and mammalian glycans. Nat Rev Drug Discov. (2021) 20:217–43. doi: 10.1038/s41573-020-00093-1
50. Takamatsu S. Naturally occurring cell adhesion inhibitors. J Nat Med. (2018) 72:817–35. doi: 10.1007/s11418-018-1220-z
51. Romano SJ. Selectin antagonists. Treat Respir Med. (2005) 4:85–94. doi: 10.2165/00151829-200504020-00002
52. Aydt E, Wolff G. Development of synthetic pan-selectin antagonists: a new treatment strategy for chronic inflammation in asthma. Pathobiology. (2002) 70:297–301. doi: 10.1159/000070746
53. Beeh KM, Beier J, Meyer M, Buhl R, Zahlten R, Wolff G. Bimosiamose, an inhaled small-molecule pan-selectin antagonist, attenuates late asthmatic reactions following allergen challenge in mild asthmatics: a randomized, double-blind, placebo-controlled clinical cross-over-trial. Pulm Pharmacol Ther. (2006) 19:233–41. doi: 10.1016/j.pupt.2005.07.004
54. Watz H, Bock D, Meyer M, Schierhorn K, Vollhardt K, Woischwill C, et al. Inhaled pan-selectin antagonist Bimosiamose attenuates airway inflammation in COPD. Pulm Pharmacol Ther. (2013) 26:265–70. doi: 10.1016/j.pupt.2012.12.003
55. Kirsten A, Watz H, Kretschmar G, Pedersen F, Bock D, Meyer-Sabellek W, et al. Efficacy of the pan-selectin antagonist Bimosiamose on ozone-induced airway inflammation in healthy subjects–a double blind, randomized, placebo-controlled, cross-over clinical trial. Pulm Pharmacol Ther. (2011) 24:555–8. doi: 10.1016/j.pupt.2011.04.029
56. Peres RS, Menezes GB, Teixeira MM, Cunha FQ. Pharmacological opportunities to control inflammatory diseases through inhibition of the leukocyte recruitment. Pharmacol Res. (2016) 112:37–48. doi: 10.1016/j.phrs.2016.01.015
57. Kanter J, Lanzkron S. Innovations in Targeted Anti-Adhesion Treatment for Sickle Cell Disease. Clin Pharmacol Ther. (2020) 107:140–6. doi: 10.1002/cpt.1682
58. DeAngelo DJ, Erba HP, Jonas BA, O'Dwyer ME, Marlton P, Huls G, et al. A Double-Blind, Placebo-Controlled, Phase 3 Registration Trial to Evaluate the Efficacy of Uproleselan (GMI-1271) with Standard Salvage Chemotherapy in Patients with Relapsed/Refractory (R/R) Acute Myeloid Leukemia. Washington, DC: American Society of Hematology (2019).
59. Devata S, Angelini DE, Blackburn S, Hawley A, Myers DD, Schaefer JK, et al. Use of GMI-1271, an E-selectin antagonist, in healthy subjects and in 2 patients with calf vein thrombosis. Res Pract Thromb Haemost. (2020) 4:193–204. doi: 10.1002/rth2.12279
60. Telen MJ, Batchvarova M, Shan S, Bovee-Geurts PH, Zennadi R, Leitgeb A, et al. Sevuparin binds to multiple adhesive ligands and reduces sickle red blood cell-induced vaso-occlusion. Br J Haematol. (2016) 175:935–48. doi: 10.1111/bjh.14303
61. Glassman PM, Myerson JW, Ferguson LT, Kiseleva RY, Shuvaev VV, Brenner JS, et al. Targeting drug delivery in the vascular system: Focus on endothelium. Adv Drug Deliv Rev. (2020) 157:96–117. doi: 10.1016/j.addr.2020.06.013
62. Kiseleva RY, Glassman PM, Greineder CF, Hood ED, Shuvaev VV, Muzykantov VR. Targeting therapeutics to endothelium: are we there yet? Drug Deliv Transl Res. (2018) 8:883–902. doi: 10.1007/s13346-017-0464-6
63. Stahn R, Grittner C, Zeisig R, Karsten U, Felix S, Wenzel K. Sialyl Lewisx-liposomes as vehicles for site-directed, E-selectin-mediated drug transfer into activated endothelial cells. Cell Mol Life Sci. (2001) 58:141. doi: 10.1007/PL00000774
64. Murohara T, Margiotta J, Phillips LM, Paulson JC, DeFrees S, Zalipsky S, et al. Cardioprotection by liposome-conjugated sialyl Lewisx-oligosaccharide in myocardial ischaemia and reperfusion injury. Cardiovasc Res. (1995) 30:965–74. doi: 10.1016/S0008-6363(95)00157-3
65. Alekseeva A, Kapkaeva M, Shcheglovitova O, Boldyrev I, Pazynina G, Bovin N, et al. Interactions of antitumour Sialyl Lewis X liposomes with vascular endothelial cells. Biochim Biophys Acta. (2015) 1848:1099–110. doi: 10.1016/j.bbamem.2015.01.016
66. Vodovozova EL, Moiseeva EV, Grechko GK, Gayenko GP, Nifant'Ev N, Bovin N, et al. Antitumour activity of cytotoxic liposomes equipped with selectin ligand SiaLeX, in a mouse mammary adenocarcinoma model. Eur J Cancer. (2000) 36:942–9. doi: 10.1016/S0959-8049(00)00029-0
67. Kuznetsova NR, Stepanova EV, Peretolchina NM, Khochenkov DA, Boldyrev IA, Bovin NV, et al. Targeting liposomes loaded with melphalan prodrug to tumour vasculature via the Sialyl Lewis X selectin ligand. J Drug Target. (2014) 22:242–50. doi: 10.3109/1061186X.2013.862805
68. Hirai M, Minematsu H, Hiramatsu Y, Kitagawa H, Otani T, Iwashita S, et al. Novel and simple loading procedure of cisplatin into liposomes and targeting tumor endothelial cells. Int J Pharm. (2010) 391:274–83. doi: 10.1016/j.ijpharm.2010.02.030
69. Hashida N, Ohguro N, Yamazaki N, Arakawa Y, Oiki E, Mashimo H, et al. High-efficacy site-directed drug delivery system using sialyl-Lewis X conjugated liposome. Exp Eye Res. (2008) 86:138–49. doi: 10.1016/j.exer.2007.10.004
70. Minaguchi J, Oohashi T, Inagawa K, Ohtsuka A, Ninomiya Y. Transvascular accumulation of Sialyl Lewis X conjugated liposome in inflamed joints of collagen antibody-induced arthritic (CAIA) mice. Arch Histol Cytol. (2008) 71:195–203. doi: 10.1679/aohc.71.195
71. Robbins GP, Saunders RL, Haun JB, Rawson J, Therien MJ, Hammer DA. Tunable leuko-polymersomes that adhere specifically to inflammatory markers. Langmuir. (2010) 26:14089–96. doi: 10.1021/la1017032
72. Jubeli E, Moine L, Nicolas V, Barratt G. Preparation of E-selectin-targeting nanoparticles and preliminary in vitro evaluation. Int J Pharm. (2012) 426:291–301. doi: 10.1016/j.ijpharm.2012.01.029
73. Chantarasrivong C, Ueki A, Ohyama R, Unga J, Nakamura S, Nakanishi I, et al. Synthesis and functional characterization of novel sialyl LewisX mimic-decorated liposomes for E-selectin-mediated targeting to inflamed endothelial cells. Mol Pharm. (2017) 14:1528–37. doi: 10.1021/acs.molpharmaceut.6b00982
74. Shamay Y, Paulin D, Ashkenasy G, David A. Multivalent display of quinic acid based ligands for targeting E-selectin expressing cells. J Med Chem. (2009) 52:5906–15. doi: 10.1021/jm900308r
75. Mizrachi A, Shamay Y, Shah J, Brook S, Soong J, Rajasekhar VK, et al. Tumour-specific PI3K inhibition via nanoparticle-targeted delivery in head and neck squamous cell carcinoma. Nat Commun. (2017) 8:1–10. doi: 10.1038/ncomms14292
76. Shu G, Lu C, Wang Z, Du Y, Xu X, Xu M, et al. Fucoidan-based micelles as P-selectin targeted carriers for synergistic treatment of acute kidney injury. Nanomed Nanotechnol Biol Med. (2021) 32:102342. doi: 10.1016/j.nano.2020.102342
77. Ásgeirsdóttir SA, Zwiers PJ, Morselt HW, Moorlag HE, Bakker HI, Heeringa P, et al. Inhibition of proinflammatory genes in anti-GBM glomerulonephritis by targeted dexamethasone-loaded AbEsel liposomes. Am J Physiol Renal Physiol. (2008) 294:F554–61. doi: 10.1152/ajprenal.00391.2007
78. Adrian JE, Morselt HW, Süss R, Barnert S, Kok JW, Ásgeirsdóttir SA, et al. Targeted SAINT-O-Somes for improved intracellular delivery of siRNA and cytotoxic drugs into endothelial cells. J Control Release. (2010) 144:341–9. doi: 10.1016/j.jconrel.2010.03.003
79. Kowalski PS, Lintermans LL, Ht Morselt W, Leus NG, Ruiters MH, Molema G, et al. Anti-VCAM-1 and anti-E-selectin SAINT-O-Somes for selective delivery of siRNA into inflammation-activated primary endothelial cells. Mol Pharm. (2013) 10:033–3044. doi: 10.1021/mp4001124
80. de Bittencourt Jr PIH, Lagranha DJ, Maslinkiewicz A, Senna SM, Tavares AM, Baldissera LP, et al. LipoCardium: endothelium-directed cyclopentenone prostaglandin-based liposome formulation that completely reverses atherosclerotic lesions. Atherosclerosis. (2007) 193:245–58. doi: 10.1016/j.atherosclerosis.2006.08.049
81. Hua S, Cabot PJ. Targeted nanoparticles that mimic immune cells in pain control inducing analgesic and anti-inflammatory actions: a potential novel treatment of acute and chronic pain conditions. Pain Physician. (2013) 16:E199–216. doi: 10.36076/ppj.2013/16/E199
82. Li S, Chen L, Wang G, Xu L, Hou S, Chen Z, et al. Anti-ICAM-1 antibody-modified nanostructured lipid carriers: a pulmonary vascular endothelium-targeted device for acute lung injury therapy. J Nanobiotechnology. (2018) 16:1–14. doi: 10.1186/s12951-018-0431-5
83. David A. Peptide ligand-modified nanomedicines for targeting cells at the tumor microenvironment. Adv Drug Deliv Rev. (2017) 119:120–42. doi: 10.1016/j.addr.2017.05.006
84. Martens CL. Cwirla SE, Lee RYW, Whitehorn E, Chen EYF, Bakker A, et al. Peptides Which Bind to E-selectin and Block Neutrophil Adhesion (*). J Biol Chem. (1995) 270:21129–36. doi: 10.1074/jbc.270.36.21129
85. Shamay Y, Paulin D, Ashkenasy G, David A. E-selectin binding peptide–polymer–drug conjugates and their selective cytotoxicity against vascular endothelial cells. Biomaterials. (2009) 30:6460–8. doi: 10.1016/j.biomaterials.2009.08.013
86. Shamay Y, Raviv L, Golan M, Voronov E, Apte RN, David A. Inhibition of primary and metastatic tumors in mice by E-selectin-targeted polymer–drug conjugates. J Control Release. (2015) 217:102–12. doi: 10.1016/j.jconrel.2015.08.029
87. Tsoref O, Tyomkin D, Amit U, Landa N, Cohen-Rosenboim O, Kain D, et al. E-selectin-targeted copolymer reduces atherosclerotic lesions, adverse cardiac remodeling, and dysfunction. J Control Release. (2018) 288:136–47. doi: 10.1016/j.jconrel.2018.08.029
88. Liu Y, Yang B, Zhao X, Xi M, Yin Z. E-Selectin-binding peptide–modified bovine serum albumin nanoparticles for the treatment of acute lung injury. AAPS PharmSciTech. (2019) 20:1–11. doi: 10.1208/s12249-019-1403-2
89. Han X, Dong X, Li J, Wang M, Luo L, Li Z, et al. Free paclitaxel-loaded E-selectin binding peptide modified micelle self-assembled from hyaluronic acid-paclitaxel conjugate inhibit breast cancer metastasis in a murine model. Int J Pharm. (2017) 528:33–46. doi: 10.1016/j.ijpharm.2017.05.063
90. Wodicka JR, Morikis VA, Dehghani T, Simon SI, Panitch A. Selectin-Targeting Peptide–Glycosaminoglycan Conjugates Modulate Neutrophil–Endothelial Interactions. Cell Mol Bioeng. (2019) 12:21–130. doi: 10.1007/s12195-018-0555-6
91. Fukuda MN, Ohyama C, Lowitz K, Matsuo O, Pasqualini R, Ruoslahti E, et al. A peptide mimic of E-selectin ligand inhibits sialyl Lewis X-dependent lung colonization of tumor cells. Cancer Res. (2000) 60:450–6.
92. Hao T, Fu Y, Yang Y, Yang S, Liu J, Tang J, et al. Tumor vasculature-targeting PEGylated peptide-drug conjugate prodrug nanoparticles improve chemotherapy and prevent tumor metastasis. Eur J Med Chem. (2021) 219:113430. doi: 10.1016/j.ejmech.2021.113430
93. Kawano S, Iyaguchi D, Sasaki Y, Sekizaki H, Toyota E. Identification of a novel carbohydrate-mimicking octapeptide from chemical peptide library and characterization as selectin inhibitor. Biol Pharm Bull. (2011) 34:883–9. doi: 10.1248/bpb.34.883
94. Fernandes AC, Liu M, Sorbo T, Appold LC, Ilbert M, Ferracci G, et al. A computational and experimental study to develop E-selectin targeted peptides for molecular imaging applications. Future Med Chem. (2018) 10:2695–711. doi: 10.4155/fmc-2018-0244
95. Zhang Y, Zhu X, Chen X, Chen Q, Zhou W, Guo Q, et al. Activated platelets-targeting micelles with controlled drug release for effective treatment of primary and metastatic triple negative breast cancer. Adv Funct Mater. (2019) 29:1806620. doi: 10.1002/adfm.201806620
96. Li S, Li L, Lin X, Chen C, Luo C, Huang Y. Targeted Inhibition of Tumor Inflammation and Tumor-Platelet Crosstalk by Nanoparticle-Mediated Drug Delivery Mitigates Cancer Metastasis. ACS Nano. (2021) 16:50–67. doi: 10.1021/acsnano.1c06022
97. Chen X, Wang Q, Liu L, Sun T, Zhou W, Chen Q, et al. Double-sided effect of tumor microenvironment on platelets targeting nanoparticles. Biomaterials. (2018) 183:258–67. doi: 10.1016/j.biomaterials.2018.07.005
98. Simion V, Constantinescu CA, Stan D, Deleanu M, Tucureanu MM, Butoi E, et al. P-selectin targeted dexamethasone-loaded lipid nanoemulsions: a novel therapy to reduce vascular inflammation. Mediators Inflamm. (2016) 2016:1625149. doi: 10.1155/2016/1625149
99. Chittasupho C, Xie SX, Baoum A, Yakovleva T, Siahaan TJ, Berkland CJ. ICAM-1 targeting of doxorubicin-loaded PLGA nanoparticles to lung epithelial cells. Eur J Pharm Sci. (2009) 37:141–50. doi: 10.1016/j.ejps.2009.02.008
100. Calin M, Stan D, Schlesinger M, Simion V, Deleanu M, Constantinescu CA, et al. VCAM-1 directed target-sensitive liposomes carrying CCR2 antagonists bind to activated endothelium and reduce adhesion and transmigration of monocytes. Eur J Pharm Biopharmac. (2015) 89:18–29. doi: 10.1016/j.ejpb.2014.11.016
101. Roblek M, Calin M, Schlesinger M, Stan D, Zeisig R, Simionescu M, et al. Targeted delivery of CCR2 antagonist to activated pulmonary endothelium prevents metastasis. J Control Release. (2015) 220:341–7. doi: 10.1016/j.jconrel.2015.10.055
102. Fuior EV, Deleanu M, Constantinescu CA, Rebleanu D, Voicu G. M. Simionescu, et al. Functional role of VCAM-1 targeted flavonoid-loaded lipid nanoemulsions in reducing endothelium inflammation. Pharmaceutics. (2019) 11:391. doi: 10.3390/pharmaceutics11080391
103. Imanparast F, Faramarzi MA, Vatannejad A, Paknejad M, Deiham B, Kobarfard F, et al. mZD7349 peptide-conjugated PLGA nanoparticles directed against VCAM-1 for targeted delivery of simvastatin to restore dysfunctional HUVECs. Microvasc Res. (2017) 112:14–9. doi: 10.1016/j.mvr.2017.02.002
104. Mann AP, Tanaka T, Somasunderam A, Liu X, Gorenstein DG, Ferrari M. E-Selectin-targeted porous silicon particle for nanoparticle delivery to the bone marrow. Adv Mater. (2011) 23:H278–82. doi: 10.1002/adma.201101541
105. Mann AP, Bhavane RC, Somasunderam A, Montalvo-Ortiz BL, Ghaghada KB, Volk D, et al. Thioaptamer conjugated liposomes for tumor vasculature targeting. Oncotarget. (2011) 2:298. doi: 10.18632/oncotarget.261
106. Ma S, Tian XY, Zhang Y, Mu C, Shen H, Bismuth J, et al. E-selectin-targeting delivery of microRNAs by microparticles ameliorates endothelial inflammation and atherosclerosis. Sci Rep. (2016) 6:1–11. doi: 10.1038/srep22910
107. Liang B, Deng T, Li J, Ouyang X, Na W, Deng D. Biomimetic theranostic strategy for anti-metastasis therapy of breast cancer via the macrophage membrane camouflaged superparticles. Mater Sci Eng C. (2020) 115:111097. doi: 10.1016/j.msec.2020.111097
108. Park JH, Jiang Y, Zhou J, Gong H, Mohapatra A, Heo J, et al. Genetically engineered cell membrane–coated nanoparticles for targeted delivery of dexamethasone to inflamed lungs. Sci Adv. (2021) 7:eabf7820. doi: 10.1126/sciadv.abf7820
109. Mann AP, Somasunderam A, Nieves-Alicea R, Li X, Hu A, Sood AK, et al. Identification of thioaptamer ligand against E-selectin: potential application for inflamed vasculature targeting. PLoS ONE. (2010) 5:e13050. doi: 10.1371/journal.pone.0013050
110. Hahn U. SDA and IDA–Two aptamers to inhibit cancer cell adhesion. Biochimie. (2018) 145:84–90. doi: 10.1016/j.biochi.2017.10.018
111. Chacko AM, Hood ED, Zern BJ, Muzykantov VR. Targeted nanocarriers for imaging and therapy of vascular inflammation. Curr Opin Colloid Interface Sci. (2011) 16:215–27. doi: 10.1016/j.cocis.2011.01.008
112. Tsourkas A, Shinde-Patil VR, Kelly KA, Patel P, Wolley A, Allport JR, et al. In vivo imaging of activated endothelium using an anti-VCAM-1 magnetooptical probe. Bioconjug Chem. (2005) 16:576–81. doi: 10.1021/bc050002e
113. Rucher G, Cameliere L, Fendri J, Anfray A, Abbas A, Kamel S, et al. Molecular imaging of endothelial activation and mineralization in a mouse model of accelerated atherosclerosis. EJNMMI Res. (2019) 9:1–9. doi: 10.1186/s13550-019-0550-5
114. Reynolds PR, Larkman DJ, Haskard DO, Hajnal JV, Kennea NL, George AJ, et al. Detection of vascular expression of E-selectin in vivo with MR imaging. Radiology. (2006) 241:469–76. doi: 10.1148/radiol.2412050490
115. Rossin R, Muro S, Welch MJ, Muzykantov VR, Schuster DP. In vivo imaging of 64Cu-labeled polymer nanoparticles targeted to the lung endothelium. J Nucl Med. (2008) 49:103–11. doi: 10.2967/jnumed.107.045302
116. Kang HW, Josephson L, Petrovsky A, Weissleder R, Bogdanov A. Magnetic resonance imaging of inducible E-selectin expression in human endothelial cell culture. Bioconjug Chem. (2002) 13:122–7. doi: 10.1021/bc0155521
117. van Kasteren SI, Campbell SJ, Serres S, Anthony DC, Sibson NR, Davis BG. Glyconanoparticles allow pre-symptomatic in vivo imaging of brain disease. Proc Nat Acad Sci. (2009) 106:18–23. doi: 10.1073/pnas.0806787106
118. Boutry S, Laurent S, Elst LV, Muller RN. Specific E-selectin targeting with a superparamagnetic MRI contrast agent. Contrast Media Mol Imaging. (2006) 1:15–22. doi: 10.1002/cmmi.87
119. Narkhede AA, Sherwood JA, Antone A, Coogan KR, Bolding MS, Deb S, et al. Role of surface chemistry in mediating the uptake of ultrasmall iron oxide nanoparticles by cancer cells. ACS Appl Mater Interfaces. (2019) 11:17157–66. doi: 10.1021/acsami.9b00606
120. Kelly KA, Allport JR, Tsourkas A, Shinde-Patil VR, Josephson L, Weissleder R. Detection of vascular adhesion molecule-1 expression using a novel multimodal nanoparticle. Circ Res. (2005) 96:327–36. doi: 10.1161/01.RES.0000155722.17881.dd
121. McCarthy JR, Weissleder R. Multifunctional magnetic nanoparticles for targeted imaging and therapy. Adv Drug Deliv Rev. (2008) 60:1241–51. doi: 10.1016/j.addr.2008.03.014
122. Sun X, Li W, Zhang X, Qi M, Zhang Z, Zhang XE, et al. In vivo targeting and imaging of atherosclerosis using multifunctional virus-like particles of simian virus 40. Nano Lett. (2016) 16:6164–71. doi: 10.1021/acs.nanolett.6b02386
123. Nahrendorf M, Jaffer FA, Kelly KA, Sosnovik DE, Aikawa E, Libby P, et al. Noninvasive vascular cell adhesion molecule-1 imaging identifies inflammatory activation of cells in atherosclerosis. Circulation. (2006) 114:1504–11. doi: 10.1161/CIRCULATIONAHA.106.646380
124. Unnikrishnan S, Du Z, Diakova GB, Klibanov AL. Formation of microbubbles for targeted ultrasound contrast imaging: practical translation considerations. Langmuir. (2018) 35:10034–41. doi: 10.1021/acs.langmuir.8b03551
125. Weinkauf CC, Concha-Moore K, Lindner JR, Marinelli ER, Hadinger KP, Bhattacharjee S, et al. Endothelial vascular cell adhesion molecule 1 is a marker for high-risk carotid plaques and target for ultrasound molecular imaging. J Vasc Surg. (2018) 68:105S−113S. doi: 10.1016/j.jvs.2017.10.088
126. Leng X, Wang J, Carson A, Chen X, Fu H, Ottoboni S, et al. Ultrasound detection of myocardial ischemic memory using an E-selectin targeting peptide amenable to human application. Mol Imaging. (2014) 13:7290. doi: 10.2310/7290.2014.00006
127. Fokong S, Fragoso A, Rix A, Curaj A, Wu Z, Lederle W, et al. Ultrasound molecular imaging of E-selectin in tumor vessels using poly n-butyl cyanoacrylate microbubbles covalently coupled to a short targeting peptide. Invest Radiol. (2013) 48:843–50. doi: 10.1097/RLI.0b013e31829d03ec
128. Jin A, Tuor U, Rushforth D, Filfil R, Kaur J, Ni F, et al. Magnetic resonance molecular imaging of post-stroke neuroinflammation with a P-selectin targeted iron oxide nanoparticle. Contrast Media Mol Imaging. (2009) 4:305–11. doi: 10.1002/cmmi.292
129. Xu J, Zhou J, Zhong Y, Zhang Y, Ye M, Hou J, et al. EWVDV-Mediated Platelet-Targeting Nanoparticles for the Multimodal Imaging of Thrombi at Different Blood Flow Velocities. Int J Nanomed. (2020) 15:1759. doi: 10.2147/IJN.S233968
130. Xu J, Zhou J, Zhong Y, Zhang Y, Liu J, Chen Y, et al. Phase transition nanoparticles as multimodality contrast agents for the detection of thrombi and for targeting thrombolysis: in vitro and in vivo experiments. ACS Appl Mater Interfaces. (2017) 9:42525–35. doi: 10.1021/acsami.7b12689
131. Chinnappan R, Al Faraj A, Abdel Rahman AM, Abu-Salah KM, Mouffouk F, Zourob M. Anti-VCAM-1 and Anti-IL4Rα Aptamer-Conjugated Super Paramagnetic Iron Oxide Nanoparticles for Enhanced Breast Cancer Diagnosis and Therapy. Molecules. (2020) 25:3437. doi: 10.3390/molecules25153437
132. Whiting N, Hu J, Zacharias NM, Lokesh GL, Volk DE, Menter DG, et al. Developing hyperpolarized silicon particles for in vivo MRI targeting of ovarian cancer. J Med Imaging. (2016) 3:036001. doi: 10.1117/1.JMI.3.3.036001
133. Weitz-Schmidt G, Stokmaier D, Scheel G. Nifant'ev NE, Tuzikov AB, Bovin NV. An E-selectin binding assay based on a polyacrylamide-type glycoconjugate. Anal Biochem. (1996) 238:184–90. doi: 10.1006/abio.1996.0273
134. Game SM, Rajapurohit PK, Clifford M, Bird MI, Priest R, Bovin NV, et al. Scintillation proximity assay for E-, P-, and L-selectin utilizing polyacrylamide-based neoglycoconjugates as ligands. Anal Biochem. (1998) 258:127–35. doi: 10.1006/abio.1998.2576
135. Moog KE, Barz M, Bartneck M, Beceren-Braun F, Mohr N, Wu Z, et al. Polymeric selectin ligands mimicking complex carbohydrates: from selectin binders to modifiers of macrophage migration. Angew Chem Int Ed Engl. (2017) 56:1416–21. doi: 10.1002/anie.201610395
136. Pochechueva T, Galanina O, Ushakova N, Preobrazhenskaya M, Sablina M, Nifantiev N, et al. Uncharged P-selectin blockers. Glycoconj J. (2003) 20:91–7. doi: 10.1023/B:GLYC.0000018583.63140.91
137. Weinhart M, Gröger D, Enders S, Riese SB, Dernedde J, Kainthan RK, et al. The role of dimension in multivalent binding events: structure–activity relationship of dendritic polyglycerol sulfate binding to L-selectin in correlation with size and surface charge density. Macromol Biosci. (2011) 11:1088–98. doi: 10.1002/mabi.201100051
138. Dernedde J, Rausch A, Weinhart M, Enders S, Tauber R, Licha K, et al. Dendritic polyglycerol sulfates as multivalent inhibitors of inflammation. Proc Nat Acad Sci. (2010) 107:19679–84. doi: 10.1073/pnas.1003103107
139. Rele SM, Cui W, Wang L, Hou S, Barr-Zarse G, Tatton D, et al. Dendrimer-like PEO glycopolymers exhibit anti-inflammatory properties. J Am Chem Soc. (2005) 127:10132–3. doi: 10.1021/ja0511974
140. Bartneck M, Schlößer CT, Barz M, Zentel R, Trautwein C, Lammers T, et al. Immunomodulatory therapy of inflammatory liver disease using selectin-binding glycopolymers. ACS Nano. (2017) 11:9689–700. doi: 10.1021/acsnano.7b04630
141. Oishi K, Hamaguchi Y, Matsushita T, Hasegawa M, Okiyama N, Dernedde J, et al. A Crucial Role of L-Selectin in C Protein–Induced Experimental Polymyositis in Mice. Arthritis Rheumatol. (2014) 66:1864–71. doi: 10.1002/art.38630
142. Milošević N, Rütter M, Ventura Y, Kezerle Y, Feinshtein V, David A. Attenuation of neutrophil-mediated liver injury in mice by drug-free E-selectin binding polymer. J Control Release. (2020) 319:475–86. doi: 10.1016/j.jconrel.2019.12.018
143. Wodicka JR, Chambers AM, Sangha GS, Goergen CJ, Panitch A. Development of a glycosaminoglycan derived, selectin targeting anti-adhesive coating to treat endothelial cell dysfunction. Pharmaceuticals. (2017) 10:36. doi: 10.3390/ph10020036
144. Zaiden M, Feinshtein V, David A. Inhibition of CD44v3 and CD44v6 function blocks tumor invasion and metastatic colonization. J Control Release. (2017) 257:10–20. doi: 10.1016/j.jconrel.2017.01.021
145. Chittasupho C, Shannon L, Siahaan TJ, Vines CM, Berkland C. Nanoparticles targeting dendritic cell surface molecules effectively block T cell conjugation and shift response. ACS Nano. (2011) 5:1693–702. doi: 10.1021/nn102159g
146. Cecioni S, Imberty A, Vidal S. Glycomimetics versus multivalent glycoconjugates for the design of high affinity lectin ligands. Chem Rev. (2015) 115:525–61. doi: 10.1021/cr500303t
147. Li J, Yu F, Chen Y, Oupický D. Polymeric drugs: advances in the development of pharmacologically active polymers. J Control Release. (2015) 219:369–82. doi: 10.1016/j.jconrel.2015.09.043
148. Vonnemann J, Liese S, Kuehne C, Ludwig K, Dernedde J, Böttcher C, et al. Size dependence of steric shielding and multivalency effects for globular binding inhibitors. J Am Chem Soc. (2015) 137:2572–9. doi: 10.1021/ja5114084
149. Bhatia S, Lauster D, Bardua M, Ludwig K, Angioletti-Uberti S, Popp N, et al. Linear polysialoside outperforms dendritic analogs for inhibition of influenza virus infection in vitro and in vivo. Biomaterials. (2017) 138:22–34. doi: 10.1016/j.biomaterials.2017.05.028
150. Ferber S, Tiram G, Sousa-Herves A, Eldar-Boock A, Krivitsky A, Scomparin A, et al. Co-targeting the tumor endothelium and P-selectin-expressing glioblastoma cells leads to a remarkable therapeutic outcome. Elife. (2017) 6:e25281. doi: 10.7554/eLife.25281
151. Rütter M, Milošević N, David A. Say no to drugs: Bioactive macromolecular therapeutics without conventional drugs. J Control Release. (2021) 330:1191–207. doi: 10.1016/j.jconrel.2020.11.026
152. Tavares MR, Pechar M, Chytil P, Etrych T. Polymer-Based Drug-Free Therapeutics for Anticancer, Anti-Inflammatory, Antibacterial Treatment. Macromol Biosci. (2021) 21:2100135. doi: 10.1002/mabi.202100135
153. Kang SA, Tsolmon B, Mann AP, Zheng W, Zhao L, Zhao YD, et al. Safety evaluation of intravenously administered mono-thioated aptamer against E-selectin in mice. Toxicol Appl Pharmacol. (2015) 287:86–92. doi: 10.1016/j.taap.2015.05.011
154. Ou Z, Dolmatova E, Lassègue B, Griendling KK. β 1-and β 2-integrins: central players in regulating vascular permeability and leukocyte recruitment during acute inflammation. Am J Physiol Heart Circ Physiol. (2021) 320:H734–9. doi: 10.1152/ajpheart.00518.2020
155. Ahmad K, Lee EJ, Shaikh S, Kumar A, Rao KM, Park SY, et al. Targeting integrins for cancer management using nanotherapeutic approaches: recent advances and challenges. Semin Cancer Biol. (2021) 69:325–36. doi: 10.1016/j.semcancer.2019.08.030
156. Ludwig BS, Kessler H, Kossatz S, Reuning U. RGD-binding integrins revisited: how recently discovered functions and novel synthetic ligands (re-) shape an ever-evolving field. Cancers. (2021) 13:1711. doi: 10.3390/cancers13071711
157. Arosio D, Casagrande C. Advancement in integrin facilitated drug delivery. Adv Drug Deliv Rev. (2016) 97:111–43. doi: 10.1016/j.addr.2015.12.001
158. Schnittert J, Bansal R, Storm G, Prakash J. Integrins in wound healing, fibrosis and tumor stroma: High potential targets for therapeutics and drug delivery. Adv Drug Deliv Rev. (2018) 129:37–53. doi: 10.1016/j.addr.2018.01.020
159. Avraamides CJ, Garmy-Susini B, Varner JA. Integrins in angiogenesis and lymphangiogenesis. Nat Rev Cancer. (2008) 8:604–17. doi: 10.1038/nrc2353
160. Alday-Parejo B, Stupp R, Rüegg C. Are integrins still practicable targets for anti-cancer therapy? Cancers. (2019) 11:978. doi: 10.3390/cancers11070978
161. Kang S, Lee S, Park S, iRGD. Peptide as a Tumor-Penetrating Enhancer for Tumor-Targeted Drug Delivery. Polymers. (2020) 12:1906. doi: 10.3390/polym12091906
162. Storrs RW, Tropper FD, Li HY, Song CK, Kuniyoshi JK, Sipkins DA, et al. Paramagnetic polymerized liposomes: synthesis, characterization, and applications for magnetic resonance imaging. J Am Chem Soc. (1995) 117:7301–6. doi: 10.1021/ja00133a001
163. Hood JD, Bednarski M, Frausto R, Guccione S, Reisfeld RA, Xiang R, et al. Tumor regression by targeted gene delivery to the neovasculature. Science. (2002) 296:2404–7. doi: 10.1126/science.1070200
164. Xie J, Shen Z, Li KC, Danthi N. Tumor angiogenic endothelial cell targeting by a novel integrin-targeted nanoparticle. Int J Nanomed. (2007) 2:479.
165. Nagaraj R, Stack T, Yi S, Mathew B, Shull KR, Scott EA, et al. High density display of an anti-angiogenic peptide on micelle surfaces enhances their inhibition of αvβ3 integrin-mediated neovascularization in vitro. Nanomaterials. (2020) 10:581. doi: 10.3390/nano10030581
166. Yang H, Kuo YH, Smith ZI, Spangler J. Targeting cancer metastasis with antibody therapeutics. Wiley Interdiscip Rev Nanomed Nanobiotechnol. (2021) 13:e1698. doi: 10.1002/wnan.1698
167. Yan F, Wu H, Liu H, Deng Z, Liu H, Duan W, et al. Molecular imaging-guided photothermal/photodynamic therapy against tumor by iRGD-modified indocyanine green nanoparticles. J Control Release. (2016) 224:217–28. doi: 10.1016/j.jconrel.2015.12.050
168. Zhang J, Zhu J, Zhao L, Mao K, Gu Q, Li D, et al. RGD-modified multifunctional nanoparticles encapsulating salvianolic acid A for targeted treatment of choroidal neovascularization. J Nanobiotechnol. (2021) 19:1–16. doi: 10.1186/s12951-021-00939-9
169. Weller GE, Villanueva FS, Tom EM, Wagner WR. Targeted ultrasound contrast agents: In vitro assessment of endothelial dysfunction and multi-targeting to ICAM-1 and sialyl Lewisx. Biotechnol Bioeng. (2005) 92:780–8. doi: 10.1002/bit.20625
170. Ferrante E, Pickard J, Rychak J, Klibanov A, Ley K. Dual targeting improves microbubble contrast agent adhesion to VCAM-1 and P-selectin under flow. J Control Release. (2009) 140:100–7. doi: 10.1016/j.jconrel.2009.08.001
171. Yan F, Sun Y, Mao Y, Wu M, Deng Z, Li S, et al. Ultrasound molecular imaging of atherosclerosis for early diagnosis and therapeutic evaluation through leucocyte-like multiple targeted microbubbles. Theranostics. (2018) 8:1879. doi: 10.7150/thno.22070
172. Fromen CA, Fish MB, Zimmerman A, Adili R, Holinstat M, Eniola-Adefeso O. Evaluation of receptor-ligand mechanisms of dual-targeted particles to an inflamed endothelium. Bioeng Transl Med. (2016) 1:103–15. doi: 10.1002/btm2.10008
173. Molinaro R, Corbo C, Martinez JO, Taraballi F, Evangelopoulos M, Minardi S, et al. Biomimetic proteolipid vesicles for targeting inflamed tissues. Nat Mater. (2016) 15:1037–46. doi: 10.1038/nmat4644
174. Zinger A, Sushnitha M, Naoi T, Baudo G, De Rosa E, Chang J, et al. Enhancing inflammation targeting using tunable leukocyte-based biomimetic nanoparticles. ACS Nano. (2021) 15:6326–39. doi: 10.1021/acsnano.0c05792
175. Martinez JO, Molinaro R, Hartman KA, Boada C, Sukhovershin R, De Rosa E, et al. Biomimetic nanoparticles with enhanced affinity towards activated endothelium as versatile tools for theranostic drug delivery. Theranostics. (2018) 8:1131. doi: 10.7150/thno.22078
176. Mohammadkarim A, Tabatabaei M, Parandakh A, Mokhtari-Dizaji M, Tafazzoli-Shadpour M, Khani MM. Radiation therapy affects the mechanical behavior of human umbilical vein endothelial cells. J Mech Behav Biomed Mater. (2018) 85:188–93. doi: 10.1016/j.jmbbm.2018.06.009
177. Gabryś D, Greco O, Patel G, Prise KM, Tozer GM, Kanthou C. Radiation effects on the cytoskeleton of endothelial cells and endothelial monolayer permeability. Int J Radiat Oncol Biol Phys. (2007) 69:1553–62. doi: 10.1016/j.ijrobp.2007.08.039
178. Nübel T, Dippold W, Kaina B, Fritz G. Ionizing radiation-induced E-selectin gene expression and tumor cell adhesion is inhibited by lovastatin and all-trans retinoic acid. Carcinogenesis. (2004) 25:1335–44. doi: 10.1093/carcin/bgh133
179. Rodriguez-Ruiz ME, Garasa S, Rodriguez I, Solorzano JL, Barbes B, Yanguas A, et al. Intercellular adhesion molecule-1 and vascular cell adhesion molecule are induced by ionizing radiation on lymphatic endothelium. Int J Radiat Oncol Biol Phys. (2017) 97:389–400. doi: 10.1016/j.ijrobp.2016.10.043
180. Sharp CD, Jawahar A, Warren AC, Elrod JW, Nanda A, Alexander JS. Gamma knife irradiation increases cerebral endothelial expression of intercellular adhesion molecule 1 and E-selectin. Neurosurgery. (2003) 53:154–61. doi: 10.1227/01.NEU.0000068840.84484.DA
181. Hariri G, Zhang Y, Fu A, Han Z, Brechbiel M, Tantawy M, et al. Radiation-guided P-selectin antibody targeted to lung cancer. Ann Biomed Eng. (2008) 36:821–30. doi: 10.1007/s10439-008-9444-9
182. Corso CD, Ali AN, Diaz R. Radiation-induced tumor neoantigens: imaging and therapeutic implications. Am J Cancer Res. (2011) 1:390.
183. Kiani MF, Yuan H, Chen X, Smith L, Gaber MW, Goetz DJ. Targeting microparticles to select tissue via radiation-induced upregulation of endothelial cell adhesion molecules. Pharm Res. (2002) 19:1317–22. doi: 10.1023/a:1020350708672
184. Zhu Y, Ling Y, Zhong J, Liu X, Wei K, Huang S. Magnetic resonance imaging of radiation-induced brain injury using targeted microparticles of iron oxide. Acta radiol. (2012) 53:812–9. doi: 10.1258/ar.2012.120040
185. Pattillo CB, Venegas B, Donelson FJ, Del Valle L. Knight LC,Chong PLG, et al. Radiation-guided targeting of combretastatin encapsulated immunoliposomes to mammary tumors. Pharm Res. (2009) 26:1093–100. doi: 10.1007/s11095-009-9826-1
186. Xu J, Lee SSY, Seo H, Pang L, Jun Y, Zhang RY, et al. Quinic Acid-Conjugated Nanoparticles Enhance Drug Delivery to Solid Tumors via Interactions with Endothelial Selectins. Small. (2018) 14:1803601. doi: 10.1002/smll.201803601
187. Shamay Y, Elkabets M, Li H, Shah J, Brook S, Wang F, et al. P-selectin is a nanotherapeutic delivery target in the tumor microenvironment. Sci Transl Med. (2016) 8:345ra87. doi: 10.1126/scitranslmed.aaf7374
188. Lee S, Son B, Park G, Kim H, Kang H, Jeon J, et al. Immunogenic effect of hyperthermia on enhancing radiotherapeutic efficacy. Int J Mol Sci. (2018) 19:2795. doi: 10.3390/ijms19092795
189. Shah A, Unger E, Bain M, Bruce R, Bodkin J, Ginnetti J, et al. Cytokine and adhesion molecule expression in primary human endothelial cells stimulated with fever-range hyperthermia. Int J Hyperthermia. (2002) 18:534–51. doi: 10.1080/02656730210157843
190. Montfort A, Colacios C, Levade T, Andrieu-Abadie N, Meyer N, Ségui B. The TNF paradox in cancer progression and immunotherapy. Front Immunol. (2019) 10:1818. doi: 10.3389/fimmu.2019.01818
191. Lejeune FJ, Liénard D, Matter M, Rüegg C. Efficiency of recombinant human TNF in human cancer therapy. Cancer Immun Arch. (2006) 6:6.
192. Clark RA, Huang SJ, Murphy GF, Mollet IG, Hijnen D, Muthukuru M, et al. Human squamous cell carcinomas evade the immune response by down-regulation of vascular E-selectin and recruitment of regulatory T cells. J Exp Med. (2008) 205:2221–34. doi: 10.1084/jem.20071190
193. Dirkx AE, Egbrink Go M, Castermans K, Van Der Schaft DW, Thijssen VL, Dings RP, et al. Anti-angiogenesis therapy can overcome endothelial cell anergy and promote leukocyte-endothelium interactions and infiltration in tumors. The FASEB J. (2006) 20:621–30. doi: 10.1096/fj.05-4493com
194. Zhang H, Issekutz AC. Down-modulation of monocyte transendothelial migration and endothelial adhesion molecule expression by fibroblast growth factor: reversal by the anti-angiogenic agent SU6668. Am J Pathol. (2002) 160:2219–30. doi: 10.1016/S0002-9440(10)61169-8
195. Luo J, Lin J, Paranya G, Bischoff J. Angiostatin upregulates E-selectin in proliferating endothelial cells. Biochem Biophys Res Commun. (1998) 245:906–11. doi: 10.1006/bbrc.1998.8529
196. Santin AD, Hermonat PL, Ravaggi A, Chiriva-Internati M, Hiserodt JC, Pecorelli S, et al. Effects of retinoic acid combined with irradiation on the expression of major histocompatibility complex molecules and adhesion/costimulation molecules ICAM-1 in human cervical cancer. Gynecol Oncol. (1998) 70:195–201. doi: 10.1006/gyno.1998.5060
197. Zern BJ, Chacko AM, Liu J, Greineder CF, Blankemeyer ER, Radhakrishnan R, et al. Reduction of nanoparticle avidity enhances the selectivity of vascular targeting and PET detection of pulmonary inflammation. ACS Nano. (2013) 7:2461–9. doi: 10.1021/nn305773f
198. Simone EA, Zern BJ, Chacko AM, Mikitsh JL, Blankemeyer ER, Muro S, et al. Endothelial targeting of polymeric nanoparticles stably labeled with the PET imaging radioisotope iodine-124. Biomaterials. (2012) 33:5406–13. doi: 10.1016/j.biomaterials.2012.04.036
199. Peiseler M, Kubes P. More friend than foe: the emerging role of neutrophils in tissue repair. J Clin Invest. (2019) 129:2629–39. doi: 10.1172/JCI124616
200. Woodside DG, Vanderslice P. Inflammation and Regulation by Integrin Cell Adhesion Antagonists, in Translational Inflammation. Elsevier. (2019). pp. 43–68. doi: 10.1016/B978-0-12-813832-8.00003-0
201. Pardoll DM. The blockade of immune checkpoints in cancer immunotherapy. Nat Rev Cancer. (2012) 12:252–64. doi: 10.1038/nrc3239
Keywords: cancer, inflammation, active drug targeting, diagnosis, vascular endothelial cells, imaging
Citation: Milošević N, Rütter M and David A (2022) Endothelial Cell Adhesion Molecules- (un)Attainable Targets for Nanomedicines. Front. Med. Technol. 4:846065. doi: 10.3389/fmedt.2022.846065
Received: 30 December 2021; Accepted: 15 February 2022;
Published: 07 April 2022.
Edited by:
Gianfranco Pasut, University of Padua, ItalyReviewed by:
Maria J. Vicent, Principe Felipe Research Center (CIPF), SpainMaria Luisa Torre, University of Pavia, Italy
Copyright © 2022 Milošević, Rütter and David. This is an open-access article distributed under the terms of the Creative Commons Attribution License (CC BY). The use, distribution or reproduction in other forums is permitted, provided the original author(s) and the copyright owner(s) are credited and that the original publication in this journal is cited, in accordance with accepted academic practice. No use, distribution or reproduction is permitted which does not comply with these terms.
*Correspondence: Ayelet David, YXllbGV0ZGFAYmd1LmFjLmls