Interactions between astrocytes and extracellular matrix structures contribute to neuroinflammation-associated epilepsy pathology
- 1Neuroscience Graduate Program, Neuroscience Department, University of Virginia, Charlottesville, VA, United States
- 2Neuroscience Department, University of Virginia, Charlottesville, VA, United States
Often considered the “housekeeping” cells of the brain, astrocytes have of late been rising to the forefront of neurodegenerative disorder research. Identified as crucial components of a healthy brain, it is undeniable that when astrocytes are dysfunctional, the entire brain is thrown into disarray. We offer epilepsy as a well-studied neurological disorder in which there is clear evidence of astrocyte contribution to diseases as evidenced across several different disease models, including mouse models of hippocampal sclerosis, trauma associated epilepsy, glioma-associated epilepsy, and beta-1 integrin knockout astrogliosis. In this review we suggest that astrocyte-driven neuroinflammation, which plays a large role in the pathology of epilepsy, is at least partially modulated by interactions with perineuronal nets (PNNs), highly structured formations of the extracellular matrix (ECM). These matrix structures affect synaptic placement, but also intrinsic neuronal properties such as membrane capacitance, as well as ion buffering in their immediate milieu all of which alters neuronal excitability. We propose that the interactions between PNNs and astrocytes contribute to the disease progression of epilepsy vis a vis neuroinflammation. Further investigation and alteration of these interactions to reduce the resultant neuroinflammation may serve as a potential therapeutic target that provides an alternative to the standard anti-seizure medications from which patients are so frequently unable to benefit.
1 Introduction
1.1 Epilepsy
Affecting approximately 50 million people (World Health Organization, 2019), epilepsy is one of the most common neurological disorders in the world. Epilepsy is characterized by an individual suffering from repeated unprovoked seizures, which are a result of synchronous discharge of thousands of neurons which give rise to an abnormal EEG and are associated with a variety of behavioral abnormalities.
Temporal lobe epilepsy (TLE), which designates seizures originating in the temporal lobe, is the most common form of epilepsy observed in adults and adolescents (Blair, 2012). The majority of patients with TLE have seizures originating from internal structures of the region, which is further classified as mesial TLE (MTLE). It is well characterized by the pathological hallmark of mesial temporal lobe or hippocampal sclerosis (HS), which involves clearly demarcated regions of neuronal loss and reactivity of glial cells, or gliosis, throughout the subfields of the hippocampus and surrounding areas (Thom, 2014). Approximately one-third of patients with epilepsy are treatment-resistant, with MTLE-HS patients making up the majority of those, highlighting the need for therapeutic treatments that can specifically address the neuronal loss and gliosis that characterize HS. Although the roles of neurons and the consequences of their loss are crucial to understanding the progression of epilepsy, it has become quite evident that glial cells, particularly reactive astrocytes, can contribute to epileptogenesis; that is, the processes occurring in the brain that lead to seizures and subsequent epilepsy.
1.2 Astrocytes
Astrocytes have long been acknowledged as essential for normal brain function as well as being major contributors to injury and diseases. These specialized glial cells tile the entire brain and contact vasculature, synapses, and each other, forming gap junctions between the individual cells. In the healthy brain, these interactions enable astrocytes to be engaged in energy metabolism, blood-brain-barrier maintenance, glutamate clearance, and neurotransmitter uptake and homeostasis.
At the synaptic level, astrocytes effectuate not only neurotransmitter regulation but also synaptic formation, maturation, pruning, and stability (Dityatev and Schachner, 2003; Dityatev and Rusakov, 2011; Chung et al., 2015; Hösli et al., 2022). They do so by extending branching processes with small terminal extensions, often called leaflets, to contact and stabilize pre and postsynaptic partners (Khakh and Sofroniew, 2015; Torres-Ceja and Olsen, 2022), resulting in the classic “tripartite synapse.” The presence of astrocytic leaflets, which contain a variety of membrane receptors, permits astrocytes to closely monitor and respond to molecular changes in their immediate domains. These are crucial in regulating and redistributing molecules associated with neuronal firing released into the extracellular space (ECS), particularly potassium and glutamate.
Astrocytic processes are highly enriched in potassium (K+) channels, enabling them to clear K+ from the synaptic cleft and surrounding area following neuronal activity. Under normal homeostatic conditions, their inwardly rectifying potassium channels (Kir) maintain a membrane potential that hovers around the equilibrium potential for K+, so that upon K+ concentration increase in the ECS, astrocytes are able to swiftly take up excess K+ ions. They are then conveyed via K+-permeable gap junctions to neighboring astrocytes, enabling them to redistribute ions from regions of high to low K+ concentration (Olsen and Sontheimer, 2008; Blutstein and Haydon, 2014; Ohno, 2018). A similarly astrocyte-driven mechanism controls the extracellular concentration of the excitatory neurotransmitter glutamate, which influences neuronal excitability and can become excitotoxic if allowed to remain in the ECS. Astrocyte-specific glutamate transporters EAAT1 (GLAST) and EAAT2 (GLT-1) transport glutamate into the astrocyte along with 3Na+ in exchange for 1K+, after which the glutamate is converted to glutamine by glutamine synthetase (GS) and shuttled back to the neurons.
At each step of these synaptic processes, astrocytes inherently alter and are altered by their interactions with not only neurons, but also immune cells, signaling molecules, and even non-cellular components of the brain such as extracellular matrix. In pathological states, astrocytes can easily become reactive and transition to an inflammatory state, altering their interactions with the other brain constituents and potentially creating neuroinflammatory feedback loops.
2 Neuroinflammation
Neuroinflammation, which refers broadly to the innate immune response of the entire CNS, involves a non-specific immune system response to trauma, infection, disease, or other injurious challenge. This innate response of the CNS consists of a number of well-characterized responses including activation of microglia and increased production of cytokines, chemokines, antibodies, and other inflammatory molecules and mediators. Neuroinflammation can of course be beneficial by addressing and resolving the injury; alternatively, it can lead to dysfunction in the organism, dependent on what specific cytokines and chemokines are expressed and how long the tissue and cells are exposed to the signaling molecules. The main glial responders in the brain are microglia; however, astrocytes are also strongly associated with neuroinflammation and the inflammatory response, and in fact exhibit some of the swiftest inflammatory reactions following a brain injury.
2.1 Reactive astrocytes and astrocytic dysfunction in disease
In a neuroinflammatory situation, astrocytes can very quickly become reactive. Also referred to as “astrogliosis,” “astrocytosis,” “gliosis,” or “reactive gliosis,” these astrocytes undergo molecular, chemical, morphological, proliferative, and functional changes following an immune challenge (Sofroniew and Vinters, 2010; Vezzani et al., 2011; Zamanian et al., 2012; Robel et al., 2015; Escartin et al., 2019). These changes vary in degree of reactivity depending on the intensity or nature of the initial instigator; in fact, the heterogeneity of astrocytic responses seems to be the one agreed-upon facet of this widespread immune reaction (Escartin et al., 2019). Some reactive astrocytes are considered more beneficial or neuroprotective as they release more anti-inflammatory and health-associated signaling molecules, and others are considered more harmful or neurodegenerative as they release more pro-inflammatory, disease-associated molecules like cytokines and chemokines. This has led to a classic “good vs. bad” taxonomy of reactive astrocytes that some consider too disparate. An excellent consensus paper covers this topic (Escartin et al., 2021). For this paper, it is sufficient to express that reactive astrocytes exist along a spectrum and a single astrocyte can express both beneficial and detrimental growth factors and signaling molecules.
Keeping in mind their clearly important roles in supporting normal brain function, astrocytic dysfunction is linked to many pathologies that involve neurodegeneration including Alzheimer disease (AD) (Nwaobi et al., 2016; Pajarillo et al., 2019), Huntington’s disease (HD) (Tong et al., 2014), and amyotrophic lateral sclerosis (ALS) (Rossi et al., 2008; Ferrer, 2017; Neal and Richardson, 2018). Astrocytes and astrogliosis are also heavily implicated in epilepsy and epileptogenesis, as evidenced in the brains of human epilepsy patients (Lee et al., 1995; Crespel et al., 2002; Wetherington et al., 2008; Das et al., 2012; Devinsky et al., 2013; Eid et al., 2013; Gibbons et al., 2013; Bedner et al., 2015; Coulter and Steinhaeuser, 2015; Hayatdavoudi et al., 2022), and recapitulated in a variety of animal models.
2.2 Neuroinflammation in epilepsy
Neuroinflammation and its associated changes have been found in practically every neurodegenerative disorder (Escartin et al., 2019). Many studies have linked neuroinflammation with epilepsy in human patients (Ravizza et al., 2008; Aronica et al., 2012; Gibbons et al., 2013; Bedner et al., 2015; Ferrer, 2017; DeSena et al., 2018; Wenzel et al., 2019; Tan et al., 2021; Aulická et al., 2022), which has been replicated by a variety of animal epilepsy models including but not limited to: traumatic brain injury (TBI) associated epilepsy (Abdul-Muneer et al., 2016; Kim et al., 2016; Webster et al., 2017; Sharma et al., 2019; Zhou et al., 2020; Gao et al., 2022; Golub and Reddy, 2022), post-ischemic stroke epilepsy (Tröscher et al., 2021), glioma-associated epilepsy (Olsen and Sontheimer, 2008; Buckingham et al., 2011; Buckingham and Robel, 2013; MacKenzie et al., 2016; Tewari et al., 2018; Campbell et al., 2020; Komiyama, 2022), kainic acid (KA)-induced epilepsy (Canto et al., 2022; Han et al., 2019; Huang et al., 2022; Hubbard et al., 2016; McRae et al., 2010; Takahashi et al., 2010; Wolinski et al., 2022; Wu, Z et al., 2021), pilocarpine-induced epilepsy (Borges et al., 2003; Canto et al., 2022; Han et al., 2019; Kong et al., 2012; Mátyás, A et al., 2021; Ravizza et al., 2008; Schauwecker, 2012; Shapiro et al., 2008; Wyeth et al., 2012), kindling models of epilepsy (Kołosowska et al., 2016; Ueno et al., 2020), and a β1-integrin knockout astrogliosis mouse model (Robel et al., 2015). The models particularly analogous to human MTLE-HS include the pilocarpine model and the KA model, which exhibit varying degrees of HS in addition to upregulation of proteins associated with immune responses and inflammation (Canto et al., 2022).
Notably, both short-term and chronic exposure to inflammation can increase brain excitability and lead to lower seizure thresholds (Inyushin et al., 2010; Vezzani et al., 2013). In fact, application of lipopolysaccharide (LPS) to induce neuroinflammation in rat models of epilepsy has been shown to increase susceptibility to KA, pilocarpine, and pentylenetetrazol (PTZ)-induced seizures, as well as increased hippocampus neuronal degeneration (Galic et al., 2008; Huang et al., 2022).
2.2.1 Specific inflammatory molecules in epilepsy
Some of the specific neuroinflammatory pathways and signals that are particularly tied to epileptic activity and epileptogenesis include cytokines such as interleukin-1β (IL-1β) (Balosso et al., 2008; Sinha et al., 2008; Maroso et al., 2010; Arisi et al., 2015; Kołosowska et al., 2016; Semple et al., 2017; Webster et al., 2017; Soltani Khaboushan et al., 2022; Zhang, 2022), the TGF-β pathway (Ivens et al., 2007; Lachos et al., 2011; Das et al., 2012; Mercado-Gómez et al., 2014; Levy et al., 2015; Kim et al., 2017), high mobility group protein B1 (HMGB1) (Maroso et al., 2010; Zurolo et al., 2012; Balosso et al., 2014; Webster et al., 2017; Zaben et al., 2021; Zhang, 2022), and tumor necrosis factor α (TNF-α) (Galic et al., 2008; Soltani Khaboushan et al., 2022), as well as chemokine C-C motif ligands 2, 3, 4, and 5 (CCL2-5) (Wu et al., 2008; Fabene et al., 2010; Kan et al., 2012; Arisi et al., 2015; Srivastava et al., 2017; Wolinski et al., 2022).
2.2.1.1 IL-1β
The cytokine interleukin-1β (IL-1β) is considered to be a pro-inflammatory and has a variety of inflammation-associated downstream effectors including some of those mentioned above such as TNF-α and IL-6 (Vezzani et al., 2008). Increases or overexpression in IL-1β have been found in human patients with TLE (Zaben et al., 2021), HS and cortical dysplasia tissue (Srivastava et al., 2017), TBI associated epilepsy (Webster et al., 2017), and tumor associated epilepsy (Sun et al., 2022). This has been recapitulated in experimental epilepsy models including KA (Balosso et al., 2008; Tian et al., 2017; Wolinski et al., 2022), pilocarpine (Arisi et al., 2015), and electrical stimulation (De Simoni et al., 2000). In an epileptic setting, IL-1β is considered to be primarily secreted by activated astrocytes and microglia (Maroso et al., 2011); its receptor IL-1R1 is furthermore overexpressed in epileptic neurons and glia (Ravizza et al., 2008). Application of its endogenous antagonist IL-1Ra acts as an anticonvulsant in mice (Vezzani et al., 2000); thus, IL-1β itself may be considered a proconvulsant (Vezzani et al., 2008), although it also mediates other cell signaling pathways.
2.2.1.2 TGF-β
Transforming growth factor-β (TGF-β), a family of hormonal polypeptides, is well associated with tissue homeostasis, development, and remodeling (Massagué and Chen, 2000; Stewart et al., 2018) as well as inflammation and immune modulation. The first step in a pathway with highly variable outcomes, the members of the TGF-β family function by activating Smad proteins which enter the nucleus to regulate target genes.
Activation of TGF-β signaling is associated with epilepsy (Mercado-Gómez et al., 2014; Kim et al., 2017; Webster et al., 2017), particularly when triggered by expression of extravascular albumin, i.e., in event of blood-brain barrier (BBB) leakage (Ivens et al., 2007; Webster et al., 2017). Notably, increase in albumin uptake by astrocytes has been found to correlate with downregulation of Kir4.1 channels and reduced astrocytic buffering, further contributing to epileptiform activity (Ivens et al., 2007). Other neuroinflammatory molecules associated with epilepsy such as TLR, HMGB1, and NF-κB are also affected by TGF-β signaling (Kim et al., 2017; Webster et al., 2017).
2.2.2 Astrocytes and neuroinflammation in epilepsy
Although both systemic inflammation and astrogliosis are well-correlated with increased risk of or susceptibility to seizures (Wilcox et al., 2015), astrocytic roles in the overall progression of epilepsy, and whether they play a more contributory or compensatory role, are still debated. The heterogeneity of reactive astrocytes does not easily lend itself to an answer to this question; indeed, even adjacent astrocytes exposed to the same insult may exhibit differences in reactivity (Zamanian et al., 2012). Notably, though neuroinflammation can increase seizure susceptibility, seizure activity itself can upregulate the production of inflammatory markers and mediators, thus creating a vicious epileptogenic feedback loop.
We suggest that one way to investigate the correlation between neuroinflammation and epilepsy would be to investigate astrocyte interactions with a portion of the brain that has long been considered part of the support network, much like the historical role of astrocytes- the extracellular matrix.
3 ECM and PNNs
Rather than being a simple fluid-filled space, the gaps between adjacent cells in the brain are occupied by extracellular matrix (ECM), a loosely organized structure comprised of a variety of proteoglycans, link proteins, and hyaluronic acid. The ECM subsists in three categories: the basement membrane, which is closely associated with vasculature and blood vessels; the interstitial matrix, which is loosely structured and more associated with support and scaffolding; and perineuronal nets, which are more structured and form in only specific regions of the brain. Although all three are important, it is the perineuronal nets, hereafter referred to as “PNNs,” which will be the main focus of this review, as they are closely associated with astrocytic leaflets.
PNNs primarily form around parvalbumin-positive (PV+), fast-spiking GABAergic interneurons, where they surround the soma and generally extend along the axon initial segment and other neurites (Celio and Blumcke, 1994; Härtig et al., 1999; Slaker et al., 2016). Their physical appearance has historically been likened to “armor,” “lattice,” or “netting,” from which they derive their name. They are found in a number of brain regions including the cortex with high levels of density and intensity, specifically in the somatosensory cortex, visual cortex, and whisker barrel cortex in rodents, but are also present in the amygdala, hypothalamus, basal ganglia, and cerebellum (McRae et al., 2007; Bozzelli et al., 2018). Although PNNs also condense sparsely around cells in the hippocampus, they are expressed almost exclusively around excitatory neurons in the CA2 region (Carstens et al., 2016; Lensjø et al., 2017).
3.1 Components
The molecular components of perineuronal nets are both neuronal and glial in origin (Brückner et al., 1993; Giamanco and Matthews, 2012) and include hyaluronic acid, hyaluronan and proteoglycan link (Hapln) proteins, tenascins R and C, and a variety of chondroitin sulfate proteoglycans (CSPGs), mainly of the lectican family, including aggrecan, versican, brevican, and neurocan (Figure 1).
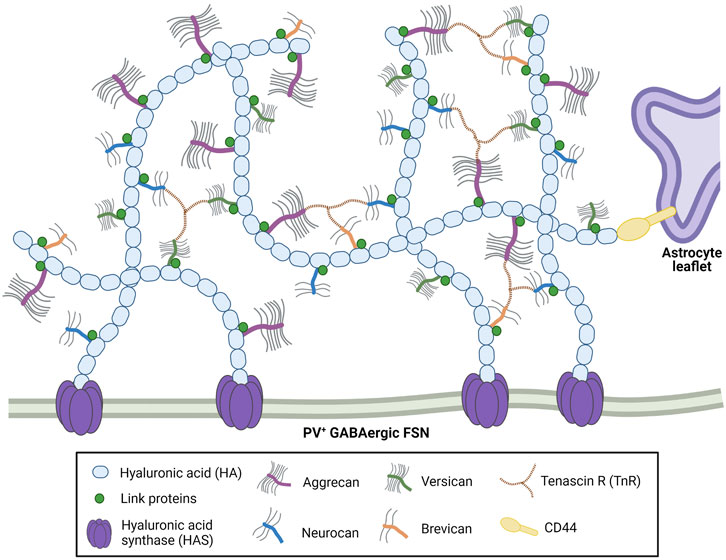
FIGURE 1. Perineuronal nets are comprised of long hyaluronic acid (HA) chains linked together with the CSPG lecticans aggrecan, versican, neurocan and brevican. Hyaluronan and proteoglycan link (Hapln) proteins and tenascin-R stabilize the CSPGs. These net-like structures are anchored by HA and hyaluronic acid synthase (HAS) on the enveloped neurons, and by HA-CD44 interactions on nearby astrocytes. Created with Biorender.com.
Aggrecan is the primary lectican component of PNNs (Giamanco and Matthews, 2012; Morawski et al., 2012; Wen T. H. et al., 2018) as well as the most well-studied; it is the loss of aggrecan that is most associated with critically impaired (Kwok et al., 2010) to practically ablated (Rowlands et al., 2018) PNN structures. Aggrecan and its fellow lecticans are anchored to neuronal cell membranes by hyaluronic acid (HA), which is produced by hyaluronic acid synthase (HAS) and stabilized by Hapln proteins, predominantly Hapln1 and 4 (Kwok et al., 2010; Mohamedi et al., 2020; Jakovljević et al., 2021). HA, HAS and Hapln proteins are also critical for PNN formation (Carulli et al., 2010; Giamanco et al., 2010; Kwok et al., 2010), as is tenascin-R (TnR). TnR, which links the lecticans of the structure, is a direct component of the PNNs, whereas tenascin-C (TnC) is affiliated with the structure but does not appear to physically contribute to it (Morawski et al., 2014). Instead, it interacts with cell surface receptors like integrins and cell adhesion molecules, and indirectly modulates the other constituents of the ECM (Jakovljević et al., 2021). Aggrecan is primarily produced by neurons (Giamanco et al., 2010) and CA2 pyramidal neurons (Carstens et al., 2016), whereas most of the other CSPGs appear to be expressed by astrocytes, which express transcripts for HAPLN1, TnR, and the other three lecticans (Giamanco and Matthews, 2012). Hyaluronic acid binding protein (HABP) is associated with both neurons and glia; glial removal results in diminished but not completely depleted HABP expression in vitro (Giamanco and Matthews, 2012).
Visualization of these structures is most often achieved using the plant lectin marker Wisteria floribunda agglutinin (WFA) (Figure 2), which binds to the glycosaminoglycan (GAG) side chains of the PNNs and is considered a fairly universal marker (Giamanco et al., 2010; Slaker et al., 2016). GAGs, which adhere to the CSPG/lectican backbone of the structure, express various sulfation patterns that contribute heavily to the negative charge of PNNs as well as influencing their overall heterogeneity, dividing CSPGs into primarily two groups with either 4-sulfated or 6-sulfated GAG chains (Bonneh-Barkay, 2009; Miyata et al., 2018). These sulfation patterns- much like the nets themselves- are dynamic and have been observed to change during development, adolescence, and through adulthood (Carulli et al., 2010; Yutsudo and Kitagawa, 2015).
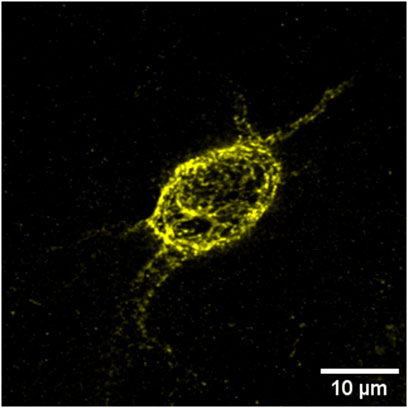
FIGURE 2. WFA+ PNNs (yellow) typically surround the soma and can extend along the axon initial segment and dendrites of inhibitory, parvalbumin-positive interneurons.
3.2 Known PNN functions
Despite being first immortalized in published form by Camillo Golgi in 1898 (Celio and Blumcke, 1994), the purposes of PNNs are still not fully elucidated. In general, the ECM is important for organization, support, and maintenance of the neural and glial cells it surrounds and encapsulates. PNNs specifically are furthermore intimately involved with the formation, stability and remodeling of synapses and synaptic signaling (Dityatev and Schachner, 2003; Frischknecht and Gundelfinger, 2012; Bozzelli et al., 2018; Lipachev et al., 2019; Reichelt et al., 2019) and thus neuronal plasticity and learning and memory (Romberg et al., 2013; Tsien, 2013; Thompson et al., 2018; Bosiacki et al., 2019; Wei et al., 2019; Chelyshev et al., 2022; Fawcett et al., 2022).
Juvenile animals still in early development display experience-dependent neuronal plasticity. This capability, observed during what is referred to as the “critical period,” is fairly depleted by the time postnatal development ends, which also coincides with the formation of PNNs (Pizzorusso et al., 2002; Hensch, 2004; Gundelfinger et al., 2010; Miyata and Kitagawa, 2015; Cornez et al., 2018). PNNs appear to stabilize or “lock” synapses into place to reduce synaptic plasticity at this point, as their physical presence restricts the placement of astrocytic leaflets and presynaptic boutons. This is particularly relevant in the sensory system, where incoming sensory information competes for cortical representation. In the visual system, for example, the closure of the ocular dominance that allocates cortical territories to each of the eyes is marked by the deposition of PNNs. Experimental degradation of the visual system PNNs using the enzymatic drug chondroitinase ABC (ChABC) reverses this process, restoring a more adolescent-like plasticity in adult animal models (Pizzorusso et al., 2002; McRae et al., 2007; Carulli et al., 2010; Hou et al., 2017). Degradation of PNNs around CA1 and CA2 hippocampal regions in mice replicated these findings, shifting the excitatory/inhibitory balance and reinstating juvenile-like plasticity (Carstens et al., 2016; Khoo et al., 2019). This characteristic stabilization of synapses further suggests a role in the formation or retention of memory (Romberg et al., 2013; Howell et al., 2015; Yang et al., 2015; Rowlands et al., 2018; Thompson et al., 2018; Wei et al., 2019) [See (Wingert and Sorg, 2021; Fawcett et al., 2022) for thorough reviews on PNNs in plasticity and memory]. Application of ChABC for treatment of glial scars, often associated with areas of neuroinflammation, was found to promote axonal regeneration and a return to plasticity in the spinal cord after injury as well (Bradbury et al., 2002; Massey et al., 2006). It is therefore proposed that PNN structures form a “repulsive barrier” that inhibits axonal and dendritic growth, not only by physically blocking leaflet and bouton formation but also via their highly negative charges as well as their interactions with growth-suppressing signaling molecules (Bonneh-Barkay, 2009; Sharma et al., 2012) [For a thorough review on glial scar formation and its immunological interactions, see (Raposo, 2014)].
Other studies have alluded to further purposes of PNNs such as helping to regulate extracellular reactive oxygen species (ROS) or protect against oxidative stress (Morawski, 2004; Beurdeley et al., 2012; Cabungcal et al., 2013) and enabling the inhibitory, GABAergic PV+ fast-spiking neurons (FSNs) that they envelop to fire action potentials at extremely high rates (Balmer, TS, 2016; Tewari et al., 2018). They also appear to play a role in ion buffering, as indicated by their highly anionic structures mentioned above (Brückner et al., 1993; Brückner et al., 1998; Härtig et al., 1999).
4 Astrocyte-ECM interactions
Astrocytes and ECM mutually interact at multiple levels in normal physiology. As discussed previously, multiple ECM components are produced by astrocytes including HAPLN1, TnR, HABP, neurocan, brevican, and versican. In the event of an injury, for example, activated astrocytes will increase secretion of CSPGs to form a glial scar around the area (Silver and Miller, 2004; Haist et al., 2012). Other ECM molecules such as TnC, laminins, and thrombospondins, while also produced by astrocytes, feature in more cell-cell signaling and cell-matrix interaction capacities instead of contributing to the physical ECM structure, and are often considered “matricellular” proteins (Eroglu, 2009; Morawski et al., 2014). Thrombospondin, for example, appears to be necessary for synaptogenesis in vitro and in vivo (Christopherson et al., 2005; Crawford et al., 2012) [See Eroglu (2009) and Jones and Bouvier (2014) for thorough reviews on astrocytically released matricellular proteins]. Astrocytes additionally are known to regulate ECM by producing molecules that degrade, remodel, and dictate the matrix structure (Kim et al., 2016), including matrix metalloproteinases and a group of metalloproteases called “a disintegrin and metalloproteinase” with and without thrombospondin motifs (ADAMs and ADAMTSs, respectively) (Ethell and Ethell, 2007; Cieplak and Strongin, 2017).
However, ECM components are also important to astrocytes, notably in development and injury response. Supplying astrocyte cultures with varying ECM proteins revealed that ECM composition determined the ability of the astrocytes to regrow following injury (Johnson et al., 2015), and alteration or removal of ECM components in development has been found to affect normal astrocytic development. Upregulation of TnC was observed post-injury (Laywell et al., 1992), as well as being associated with increased GFAP+ astrocytes (Karus et al., 2011). Similarly, knockout of aggrecan in chicken embryos results in altered glial precursor differentiation, favoring GFAP+ astrocytic cells (Domowicz et al., 2008). The glycoprotein component TnC appears to be essential for proper gliogenesis, maturation, proliferation, and differentiation (Wiese et al., 2012), with knockout resulting in changes in early astrocyte development and proliferation, and tiling in vitro (Ikeshima-Kataoka et al., 2007; Karus et al., 2011), and later increases in astrocytic GFAP expression in vivo (Karus et al., 2011).
Lastly, astrocytes also interact with the more condensed ECM structures of the brain, PNNs, on multiple levels as well. Although these have been mentioned above and will be described in later sections with greater detail, PNNs are thought to interact with astrocytes to facilitate synaptic activity, neurotransmitter uptake, and ionic buffering, all of which can be altered in inflammatory or disease states, i.e., that of the epileptic brain.
5 ECM and PNN alterations in epilepsy
A variety of ECM components and related molecules are altered in epilepsy. Although PNN expression varies across brain regions (Brückner et al., 1993; Yamada and Jinno, 2013), changes in the hippocampus are most often described. In human patients, studies have ranged from finding degradation of PNNs and decreases in PNN expression around PV+ fast-spiking neurons in chronic TLE (Perosa et al., 2002; Kim et al., 2017), to increases in diffuse ECM expression in HS(Sitaš et al., 2022) and increased expression of CSPGs and HA in MTLE hippocampus (Perosa et al., 2002). That is not to say that all individuals with epilepsy display altered brain ECM; one study even found no perceptible differences in the ECM or PNNs of adolescent or adult TLE patients (Rogers et al., 2018). However, the majority of these findings have been well replicated in the literature, primarily in rodent models of epilepsy (see Table 1).
PNNs have generally been found to be degraded or depleted in patients and experimental models of epilepsy, whereas ECM expression overall is often similar to non-epileptic controls or may even be increased. This may be explained by several mechanisms, including but not limited to a) the fact that the most common immunohistochemical PNN marker, WFA, stains for CSPGs of the PNNs and can still mark CSPG cleavage products after degradation, or b) the possibility that astrocytes and neurons that produce ECM components ramp up production in ECM-depleting circumstances as described below.
6 ECM remodeling
As previously alluded to, PNNs are dynamic assemblies constantly undergoing remodeling in the healthy brain. Fluctuations in their presence, structure, density, and intensity occur during normal physiological stages from development to adulthood, mediated by the expression of remodeling enzymes that also oscillate over time and development. Recent studies also suggest seasonal behavior-based (Cornez et al., 2020; Marchand and Schwartz, 2020) and circadian or diurnal rhythm based (Pantazopoulos et al., 2020; Harkness et al., 2021) changes in PNN intensities and expression. However, loss, alteration, and malfunction of PNNs have been increasingly associated with pathological states, including trauma or injury (Lipachev et al., 2019; Mahmud et al., 2022) and a variety of psychiatric disorders (Pantazopoulos et al., 2010; De Luca and Papa, 2016; Alcaide et al., 2019; Murthy et al., 2019; Brown and Sorg, 2022). Aberrant changes in PNN expression are heavily implicated in neurodegenerative disorders as well, including AD and dementia (Baig, S et al., 2005; Crapser et al., 2020b; Logsdon et al., 2021; Yang et al., 2015), Huntington’s disease (Crapser et al., 2020a), ischemia or stroke (Härtig, 2016; Tröscher et al., 2021), and epilepsy (Kim et al., 2016; McRae et al., 2012, 2010; Rankin-Gee et al., 2015; Rogers et al., 2018; Ueno et al., 2019; Wegrzyn, D. et al., 2020).
6.1 MMPs and other remodeling molecules regulate ECM and inflammatory markers
6.1.1 MMPs
Matrix metalloproteinases (MMPs) are a family of zinc-dependent endopeptidase enzymes expressed in and secreted by neurons, glia, and other cell types in the developing and adult nervous system (Ethell and Ethell, 2007; Reinhard et al., 2015). Once activated (by serine proteases, reactive oxygen species, nitric oxide, or other MMPs), MMPs can cleave their substrates including ECM proteins such as brevican, tenascin, aggrecan, laminin, and collagens (from which their name is derived), synaptically associated proteins such as cadherins and ephrins, growth factors and cell adhesion molecules, and cytokines such as TNF-α (Ethell and Ethell, 2007; Cieplak and Strongin, 2017). Activation of MMPs, although important in normal physiological states, is also associated with the regulation of many pathological processes, especially in the CNS wherein MMP-2, MMP-3, and MMP-9 are most abundantly found and studied. MMP-9 especially is thought to be important for brain development, critical periods, and synaptic structuring and plasticity (Reinhard et al., 2015). Notably, this MMP specifically contributes to ECM degradation following monocular deprivation (MD), leading to increased plasticity in the visual cortex which is not observed in MMP-9 knockout mice (Kelly et al., 2015; Murase et al., 2017).
Although they stimulate inflammation-associated molecules such as IL-1β and TNF-α, MMPs can also be regulated by them, including but not limited to interleukins IL-1, IL-4, and IL-6. Other enzymes, proteases and cytokines that regulate MMPs include TGF-β, TNF-α, tissue inhibitors of metalloproteinases (TIMPs), tissue plasminogen activator (tPA), and “a disintegrin and metalloproteinase” with and without thrombospondin motifs (ADAMs and ADAMTSs, respectively) (Cieplak and Strongin, 2017). TIMPs, small endogenous inhibitor proteins, can bind to and inhibit both MMPs and ADAMs/ADAMTs (Ethell and Ethell, 2007; Arpino et al., 2015). Notably, these interactions are not merely unidirectional as the ECM can also affect remodeling molecules. TGF-βs, for example- TGF-β1 in particular-are held in place in the ECM and must be released before being able to activate their signaling pathways (Hinz, 2015).
6.1.2 ADAMTSs
ADAMTSs are a subgroup of cell surface metalloproteases released by neurons and glia which are associated with neurodegeneration, inflammation, adhesion to integrins, shedding of cytokines and growth factors, and degradation of ECM proteoglycans- specifically lecticans (Bonneh-Barkay, 2009; Kelwick et al., 2015; Song and Dityatev, 2018; Mohamedi et al., 2020). They themselves also regulate MMP activity, but are primarily associated with regulating ECM composition and function (Kelwick et al., 2015). ADAMTS-4 and ADAMTS-5, two of the group of ADAMTS referred to as aggrecanases or proteoglycanases, target CSPGs including aggrecan, brevican, neurocan, and versican (Nakada et al., 2005; Kelwick et al., 2015).
6.1.3 tPA
tPA, another protease enzyme, activates microglia, upregulates MMP-3 and MMP-9, and promotes leakage of the BBB when activated (Shapiro, 1998; Dzwonek et al., 2004; Bonneh-Barkay, 2009; Rosenberg, 2009; Mehra et al., 2016). Increased proteolytic activity of tPA is further associated with the loss of dendritic spines in visual cortex MD; when tPA was blocked, MD associated spine loss was prevented (Mataga et al., 2004), supporting the idea that PNNs may assist in stabilizing synapses. As discussed previously, albumin leakage into the parenchyma can also trigger activation of TGF-β signaling, release of inflammatory factors such as IL-1β, and result in increased astrocytic MMP-9 levels (Ranaivo et al., 2012), which are then available to degrade ECM components and further stimulate inflammatory molecules (i.e., IL-1β). Furthermore, exposure to albumin is associated with changes in ECM components including HA, TnC, and neurocan (see Table 1).
6.2 Inflammatory markers associated with ECM remodeling and epilepsy
6.2.1 TGF-β
Although well associated with neuroinflammation and epilepsy as covered previously, TGF-β is also known to be crucial in a number of peripheral nervous system disorders wherein tissue straining, stiffening, or scarring plays a role, including obstructive lung diseases and numerous cancers (Hinz, 2015; Chakravarthy et al., 2018; Stewart et al., 2018). Activation of TGF-β and a variety of dependent Smad proteins has been linked to ECM synthesis, remodeling, and deposition, especially in wound healing and repair (Li et al., 2003; Hinz, 2015), and increases in astrocytic TGF-β activation have been observed in PNN degradation and hyperexcitability, likely contributing to epileptogenesis (Kim et al., 2017).
6.2.2 Chemokine C-C motif ligands (CCLs)
Chemokines of the CCL family, especially CCL5 and its receptor CCR5, are thought to be key in ECM regulation. A number of studies have found increased expression or upregulation of CCL5 in human epilepsy patients (Fabene et al., 2010; Srivastava et al., 2017), recapitulated in rodent models of pilocarpine (Arisi et al., 2015) and KA (Wolinski et al., 2022; Zhang et al., 2023) induced epilepsy. CCL5 has also been found to induce the expression of MMP-9 via monocytes, and is well-associated with a variety of cancers, mainly assisting in increasing MMP secretion to promote tumor invasion and dissemination (Aldinucci et al., 2020), as well as being correlated with astrocytic activation in a KA mouse model of epilepsy (Zhang et al., 2023). Experimental application of a CCL5/CCR5 antagonist was found to attenuate neuroinflammation, preventing neurodegeneration and activation of microglia (Zhang et al., 2023) and indicating the role of CCL5 in neurodegeneration in this model. Increases in another CCL, CCL2, and its corresponding receptor CCR2, have also been found in human TLE (Wu et al., 2008) as well as pilocarpine (Foresti et al., 2009; Mercado-Gómez et al., 2014; Arisi et al., 2015) and KA (Manley et al., 2007; Tian et al., 2017) induced epilepsy, where it plays a crucial role in inflammation, neuronal death, and activation of the downstream effectors STAT3 and IL-1β (Tian et al., 2017).
6.3 Remodeling molecules are associated with neuroinflammation and seizure activity
Though expressed and active in normal healthy physiology due to the constant turnover of ECM components, MMPs and their regulators are important mediators in CNS inflammation (Klein and Bischoff, 2011; Gaudet and Popovich, 2014) and neuroinflammatory processes (Rosenberg, 2002; Berezin et al., 2014; Reinhard et al., 2015) and have been associated with pathological disorders and diseases such as TBI(Abdul-Muneer et al., 2016; Pijet et al., 2018), stroke (Pielecka-Fortuna et al., 2015; Akol et al., 2022), glioma (Markovic et al., 2005; Varol and Sagi, 2018), and of course epilepsy (Wilczynski et al., 2008; Rankin-Gee et al., 2015; Zybura-Broda et al., 2016; Dubey et al., 2017; Pijet et al., 2018).
Systemic inflammation is well associated with increases in remodeling enzymes, especially with regards to epileptic activity. MMP-2 and MMP-9 in particular have been found to be upregulated in glia and neurons in general seizure activity, TLE, and post-status epilepticus (Dubey et al., 2017; Dzwonek et al., 2004; Kim et al., 2017; Lukasiuk et al., 2011; Pijet et al., 2018; Reinhard et al., 2015; Szklarczyk et al., 2002; Ulbrich, P. et al., 2020; Wegrzyn, D. et al., 2020; Wilczynski et al., 2008; Zybura-Broda et al., 2016). Rankin-Gee et al. (2015) find that seizure activity increases MMP proteolysis of aggrecan, which suggests a mechanism by which PNNs are degraded in epilepsy and thus contribute to the progression of the disorder. Indeed, one study found that two different strains of MMP-9 overexpressing rats displayed higher seizure susceptibility to PTZ kindling than wild type rats (Wilczynski et al., 2008). Kim et al. (2017) found upregulation of genes encoding MMP9 and 14 and ADAMTS1 in multiple brain injury and BBB leakage models as well as in resected tissue from human TLE patients (Kim et al., 2017).
In glioma-associated epilepsy, for example, epileptic activity in peritumoral areas may be attributed to MMP-driven PNN degradation. Glioma cells and tumor-associated macrophages (TAMs) release MMPs, but the host’s inflammatory cells can also release MMPs in response to the tumor cells. Glioma has also been found to overexpress ADAMTS-5, which as mentioned previously targets CSPGs, specifically cleaving brevican (Nakada et al., 2005). Furthermore, the ECM itself is thought to actively promote cancer growth by altering collagen degradation and re-deposition via remodeling enzymes so as to clear space and allow progression and growth of the tumor (Shapiro, 1998; Markovic et al., 2005; Varol and Sagi, 2018). Peritumoral areas immediately surrounding resected low-grade epilepsy-associated tumors exhibit not only increased inflammatory markers, but also an increased ripple rate, possibly implicating an MMP-driven discrepancy in excitatory-inhibitory balance (Sun et al., 2022).
Treatment of cultured rat astrocytes and microglia with inflammatory mediators such as IL-1β, TNF-α, and LPS also stimulates the production of MMP-2 and MMP-9 (Gottschall and Deb, 1996; Shapiro, 1998; Dzwonek et al., 2004), and accelerates the epileptogenesis process and/or increases seizure susceptibility in rat models of kindling induced seizures (Wilczynski et al., 2008; Kołosowska et al., 2016). In concurrence, application of MMP inhibitors or knockout of MMP-9 seemingly protected mice and rats against KA-induced and kindling-induced seizures (Wilczynski et al., 2008; Pollock et al., 2014) as well as TBI-induced spontaneous seizures (Pijet et al., 2018). Notably, a critical amount of MMPs seems to be required for optimal function-inhibiting MMP-2 and MMP-9 can suppress plasticity in the visual cortex, but briefly inhibiting the same MMPs post-stroke can rescue plasticity (Akol et al., 2022), indicating that intervention timing and intensity are crucial.
6.4 ECM cleavage products are associated with neuroinflammation
Degraded segments of PNNs and ECM are known to act as alarmins or damage-associated molecular patterns (DAMPs) and thus amplify CNS inflammation (Gaudet and Popovich, 2014; Jang et al., 2020). Buildup of fragmented HA in particular, specifically the low molecular weight (LMW) HA (10–500 kDa) generated due to ECM damage, is known to serve as an injury and inflammatory signal, binding to CD-44 and TLR4 to induce pathways such as NFκB signaling and increasing IL-1β and TNF-α in vitro (Noble, 2002; Wang et al., 2006). Hyaluronidase treatment of cultured rat astrocytes induced more stellate-like, branching morphology, indicating cleavage of HA may be associated with astrocytic form and/or function (Konopka et al., 2016).
CSPGs and tenascins are released from activated astrocytes following CNS injury, with TnC specifically increasing after exposure to IL-1β, TNF-α and INF-γ (Laywell et al., 1992; Jang et al., 2020). TnC serves as an activator of TLR4, which is well associated with increased pro-inflammatory cytokines and neuroinflammation (Midwood et al., 2009; Eidson et al., 2017). Studies have also found increased CSPG expression in the pathological hallmarks of neurodegenerative diseases with chronic inflammation components such as AD plaques and tangles and MS lesions (Jang et al., 2020).
This is not to say that all ECM components and cleavage products are pro-inflammatory; in fact, the GAG sidechains of CSPGs may have different effects due to increased or decreased affinity for specific chemokines depending on their sulfation pattern [see (Monneau et al., 2016) for a thorough review on chemokine-GAG interactions]. 6-sulfated CSPGs, for example, appear to help suppress microglial activation and production of IL-6 and TNF-α (Tan and Tabata, 2014; Jang et al., 2020).
7 Inflammatory astrocyte-ECM interactions contribute to epileptogenesis and epilepsy
Experimental degradation of PNNs or removal of its components can lead to increased propensity to epileptic activity, but may also in and of itself cause spontaneous seizure activity (Arranz et al., 2014; Tewari et al., 2018; Balashova et al., 2019; Wegrzyn. et al., 2020). Seizure activity, however, appears to cause degradation of ECM and PNNs (McRae et al., 2012; Pollock et al., 2014; Dubey et al., 2017; Ueno et al., 2019). Thus, one incidence of epileptogenic activity or PNN alteration could easily begin a feedback loop of increased degradation accompanied by increased seizure activity.
We suggest that some of these correlations are due to neuroinflammatory pathways triggered by changes in how PNNs and astrocytes are interacting, specifically at the levels of a) synapses, b) ionic buffering, and c) other biophysical properties such as cell membrane capacitance.
8 Synapses
Astrocytes and PNNs interact at the synapse in ways that may lead to neuroinflammation, thus feeding into the potential for increased susceptibility to or increased severity of epilepsy.
8.1 Reactive astrocytes affect ECM components
Many of the molecules released by reactive astrocytes can indirectly or directly lead to ECM-altering outcomes, including changes in the expression of HA, CSPGs, and tenascin proteins (Wiese et al., 2012; Bosiacki et al., 2019). Activated astrocytes are known to migrate to injury sites in the CNS and release inflammatory factors such as CCL2 and 3 (Fabene et al., 2010), as well as increasing secretion of CSPGs including neurocan, versican, and brevican, likely via TGF-β and subsequent signaling (Schiller et al., 2004). Genes associated with ECM and integrin signaling are also significantly upregulated in rat models of kainic acid and pilocarpine epilepsy after SE (Han et al., 2019) as well as being associated with genes upregulated in astrogliosis (Zamanian et al., 2012). PTZ-induced seizures were found to trigger astrogliosis in the targeted hippocampus and many cortical areas, as well as overall increases in the amount of extracellular matrix (Ueno et al., 2020) [For a thorough review of glial-ECM remodeling, see (Kim et al., 2016)].
8.2 Changes in CSPGs and tenascins alter synapses
Removal of multiple lecticans in vivo and in vitro is associated with not only abnormal PNN morphology, but also altered synaptic function, including reduced inhibitory synapses and increased excitatory presynaptic markers (Geissler et al., 2013) (Mueller-Buehl et al., 2022). Aggrecan knockout cells show a complete lack of PNNs in vitro (Kwok et al., 2010), but removal of other CSPGs does not appear to lead to such drastic changes. Brevican, for example, appears to be required for modulating synapses and excitatory contacts of inhibitory interneurons; lack of brevican at PV+ interneurons led to altered pruning of excitatory synapses and thus alterations in spike properties and miniature EPSCs (Favuzzi et al., 2017). Although a genetically deleted brevican mouse model did not show changes in the structure of the PNN itself, multiple studies reported seeing significant alterations of synaptic plasticity and transmission (Frischknecht and Gundelfinger, 2012; Blosa et al., 2016). A knockout mouse model of neurocan also correlated with notable decreases in brevican mRNA levels and visibly altered brevican ECM structures (Sonntag et al., 2018; Schmidt et al., 2020), though neurocan itself did not appear to be altered. Astrocytically released ECM molecules such as TnR and laminins interact directly with voltage-gated Ca2 channels, AMPARs, and GABARs, and as such, influence synaptic organization and function (Dityatev and Schachner, 2006); thus, upon astrogliosis, upregulation or alteration of ECM components and signaling molecules can easily follow.
8.3 Changes in CSPGs also alter neural networks
Replicating CSPG degradation along with removal of HA in vitro was found to increase synaptogenesis and decrease glutamate sensitivity (Pyka, 2011), both of which could readily lead to increases in excitability. Increases in excitability are not the only method by which the excitation/inhibition balance can be altered; in fact, a study in which PNNs were experimentally degraded using ChABC showed reduced excitability of PV+ neurons and inhibitory synaptic transmission in the visual cortex (Liu et al., 2019). Similarly, a recent study (Dzyubenko, 2021) that also experimentally degraded PNNs saw a decrease in the density of inhibitory synapses to both excitatory and inhibitory neurons, along with an increase in the strength of inhibitory synapses. However, the action potential threshold for excitatory neurons also decreased, and as such, the strengthened inhibitory neuron outputs were insufficient to balance the excitatory activity, leading to overall network changes (Dzyubenko, 2021).
8.4 Neuroinflammation-associated molecules regulate synapses
8.4.1 IL-33
The cytokine interleukin-33 (IL-33) is well established as a mediator of ECM remodeling, provided by both neurons and astrocytes. Release of IL-33 from hippocampal neurons in an experience-dependent matter has been found to activate microglia engulfment and remodeling of ECM, thus resulting in synaptic plasticity (Yasuoka et al., 2011; Vainchtein et al., 2018; Nguyen et al., 2020). Furthermore, astrocytic IL-33 mRNA and protein results in proliferation of microglia and increased proinflammatory cytokines like IL-1β and TNF-α (Yasuoka et al., 2011).
Suppression of hippocampal neuronal activity increases astrocytic release of IL-33 and has been found to promote increased excitatory synaptogenesis (Hudson et al., 2008; Wang et al., 2020). Notably, astrocytic IL-33 expression increases upon exposure to pathogen-associated molecular patterns (PAMPs) (Hudson et al., 2008), meaning that immune activation may be associated with increased excitation. If the increased neuronal activity is then suppressed, release of IL-33 may also increase, leading to an inflammatory-synaptogenesis feedback loop which would affect not only individual neuronal activity but again, overall network changes and thus the potential for epileptic activity.
8.4.2 HA-CD44 interactions
CD44 is a widely expressed transmembrane protein that serves as a receptor for HA and has been associated with cell adhesion, inflammation, and production of cytokines (Levesque and Haynes, 1997; Puré and Cuff, 2001). When expressed in myeloid cells, CD44 has been implicated in increased production of MMP-9, TNF-α, and IL-1β in vitro via TLR2 activation (Ivanova, 2022). However, other studies suggest an anti-inflammatory role of the CD44 receptor (Neal, 2018).
Expression of HA within the synaptic cleft decreases towards the end of postnatal development but increases around the synaptic cleft as the critical period is ending, when the formation of PNNs is being finalized (Wilson and Litwa, 2021; Allnoch et al., 2022). This increase in expression is likely due to the role of HA in anchoring the PNN structure to astrocytic leaflets at the synapse via its binding interactions with CD44 (Dzwonek et al., 2004; Carulli et al., 2006; Kwok et al., 2010; Miyata and Kitagawa, 2017; Wen et al., 2018b), indicating its importance in the stability of said synapse. Indeed, overexpression of HAS2 seems to inhibit the occurrence of spontaneous activity through synaptic HA synthesis (Wilson and Litwa, 2021), although the mechanism of how the HA is altering this is unclear. One potential process may involve overproduced HA anchoring PNN components to leaflets in an overly abundant manner, going so far as to interfere with normal synaptic function.
Conversely, a mouse knockout of HAS2 is associated with not only decreased HA levels in the cortex, but also an increase in epileptic seizures (Arranz et al., 2014; Perkins et al., 2017), implicating the loss of stable PNNs as epileptogenic. CD44 also seems to play a role, as HA-CD44 interactions can influence morphological changes in astrocytes via Rac1 signaling, providing evidence that ECM-driven alterations circle back to alter astrocytes (Konopka et al., 2016). Knockdown of CD44 in hippocampal neurons is associated with altered spine morphology and decreased functional synapses, as well as significantly decreased spontaneous excitatory activity (Roszkowska et al., 2016), again likely enhancing the instability of synapses and the lack of normal functional synapses via loss of the PNNs as a stabilizing component.
Notably AMPARs, which mediate excitatory currents, are restricted and stabilized by the presence of PNNs (Frischknecht et al., 2009). Alteration and/or destabilization of PNNs could thereby increase the mobility and exchange of AMPARs, thus altering activity at the excitatory synapses, not to mention plasticity and overall network excitability. Wilson and Litwa (Wilson and Litwa, 2021) further note that overexpression of CD44 decreases excitatory synapse formation, which aligns well with the proposed role of PNNs in anchoring AMPARs. Interestingly, blocking AMPARs after PTZ-induced seizures ameliorated seizure activity, but also greatly increased the overall levels of aggrecan, TnR, and neurocan in the brain (Chen, 2016).
8.5 Neuroinflammation-induced PNN changes affect astrocytes
TnC, which interacts with other cell surface receptors and helps to regulate cell growth, adhesion, and migration, is upregulated early in inflammation, either by the pro-inflammatory IL-1 pathway or possibly by IL-4, IL-13, or TGF-β, which are considered anti-inflammatory (Chiquet-Ehrismann and Chiquet, 2003). It is expressed at high concentrations in disorders characterized by chronic inflammation, and its production further induces inflammatory responses, as seen in astrocytes in vitro (Wiese et al., 2012) and a mouse model of AD, for example (Xie et al., 2013). TnC further appears to regulate astrocytic maturation during embryonic development in cortical cells and in the spinal cord (Karus et al., 2011; Wiese et al., 2012), implicating this ECM molecule in astrocyte development and providing a potential feedback loop effect of altered PNNs affecting astrocytes. HA also interacts with astrocytes at two specific molecular weights (low HA, 10–500 kDa and high HA, >500 kDa), both of which appear to modulate astrocytic responses to TLR agonists and upregulate IL-10 expression via TLR pathways (Chistyakov et al., 2019).
Taken together, it is clear that the presence of PNNs is well-associated with astrocytes at the synapse, and that neuroinflammation can not only alter these interactions but can be upregulated as a result of these interactions as well.
9 Ion buffering
Astrocytes’ maintenance of potassium (K+) and glutamate concentrations in the healthy brain is well established, as is their dysfunction in times of immune challenge, injury, and disease. As excessive extracellular K+ is associated with increased excitation and/or hyperexcitability, K+ spatial buffering in the healthy brain serves as a regulatory and protective necessity. Glutamate regulation by astrocytes is crucial as well, as excessive extracellular glutamate can also lead to excitotoxicity and hyperexcitability.
These regulatory processes are not just left to the astrocytes, however: PNNs also have a role in ionic buffering. One school of thought is that the strong negative charge of the structures, endowed by the negatively charged sulfated GAG side chains, enables PNNs to quickly bind up extracellular K+ to clear the ECS, thus preventing a buildup of excess positive charges and associated hyperexcitability, which allows the local neurons to continue firing (Brückner et al., 1993; Härtig et al., 1999).
Another is that the negative charge of the PNNs has less to do with quickly clearing the cations, but more about capturing and holding them as a type of “reservoir” to keep them readily available for altering the local ionic gradients (Morawski et al., 2015). In fact, one study proposes that the anionic charges of the ECM surrounding hippocampal neurons actually change the Cl- gradient across the membrane, thereby making GABA receptors excitable (Glykys et al., 2014). It must be further noted that the acknowledged role of PNNs in maintaining extracellular space also affects diffusion of molecules within the ECS, with degradation or reduction of PNN structure contributing to increased diffusion capabilities (Syková, 2004; Morawski et al., 2015). Cations released in the somatosensory cortex and auditory cortex, which have the most dense expression of PNNs, display a more restrained pattern of diffusion than other regions, and degradation of ECM restores a more regular isotropic diffusion of the released charges (Morawski et al., 2015). The GAGs present in the PNNs and on cell surfaces additionally interact with chemokines upon inflammatory stimuli, creating the concentration gradient necessary for chemokine-induced leukocyte recruitment and migration (Crijns et al., 2020); this too can alter diffusion in the ECS.
Lastly, the concrete physical presence of the PNNs- which influences astrocytic leaflet placement, as covered in the synapse segment previously-can certainly influence the presence of leaflets and thus their ability to take up excess extracellular ions at the synapse.
As mentioned above, dysfunctional regulation of K+ and glutamate in reactive astrocytes is already well associated with epilepsy and hyperexcitability [see (Robel and Sontheimer, 2016) for review]. However, we propose that one of the driving forces behind epileptic activity is neuroinflammation due to astrocyte-PNN interactions that change how ions are regulated at the synapse and in the ECS.
9.1 Potassium and glutamate regulation is altered in epilepsy
9.1.1 Potassium
Dysfunctional transportation or uptake of K+ is an established finding in reactive and tumor-associated astrocytes (Campbell et al., 2020), TBI-associated epilepsy (Coulter and Steinhaeuser, 2015), and MTLE-HS (Thom, 2014; Coulter and Steinhaeuser, 2015), and has been associated with hyperexcitability and epileptic activity. Reduced K+ buffering has been found to facilitate EPSPs (David et al., 2009; Kinboshi, M et al., 2020), which affects not only individual neuronal firing but can thus alter network excitation. This alteration is likely due to decreased Kir currents, which has been observed in many epilepsy models (Inyushin et al., 2010; Das et al., 2012; Robel et al., 2015; Nwaobi et al., 2016; Kinboshi et al., 2020; Akyuz et al., 2022). Rodent models in which Kir4.1 is specifically knocked out exhibit astrocytic membrane depolarization and subsequently dysregulated K+ and glutamate homeostasis, contributing to increased seizure susceptibility or activity (Djukic et al., 2007; Olsen and Sontheimer, 2008; Inyushin et al., 2010) and further solidifying Kir4.1 as an essential potassium channel in glial and neuronal homeostasis. A recent study found that KA-treated rats had an increased susceptibility to seizures via TNFα-mediated necroptosis altering BBB integrity, as well as increased levels of K+ and glutamate in the extracellular space (Huang et al., 2022), which could indicate dysregulation of glutamate and potassium.
Additionally, although astrocytes can take up albumin that enters the parenchyma due to damaged or otherwise altered BBB, that uptake is associated with transcriptional downregulation of Kir4.1 and Kir2.3 channels. This Kir downregulation can result in impaired gap junction coupling [associated with local inflammation (Karpuk et al., 2011)], altered potassium buffering, and hyperexcitability (David et al., 2009; Aronica et al., 2012; Coulter and Steinhaeuser, 2015), all of which are established as correlative in epileptogenesis. Notably, mRNA and protein expression of Kir4.1 appear to be at least partially regulated by cytokine activity in both human epileptic patients as well as in rodent models (Zurolo et al., 2012; Huang et al., 2022), implicating inflammation (and specifically the cytokine IL-1β) as a contributing pathway.
9.1.2 Glutamate
In addition to impaired Kir channels, reactive astrocytes in the MTLE brain express altered expression of glutamine synthetase (GS), leading to impaired uptake, metabolism of, and release of glutamate (Eid et al., 2013).
Dysregulation of glutamate is associated with the breakdown of normal astrocyte function (Takahashi et al., 2010; Khakh and Sofroniew, 2015), and excessive glutamate in the ECS is a well-known feature of and contributor to epilepsy in both human patients and animal models (Buckingham et al., 2011; Eid et al., 2013; Coulter and Steinhaeuser, 2015; Robel et al., 2015; Robert et al., 2015) as well as being associated with neuroinflammation, specifically inflammatory cytokines such as IL-1β and TNF-α, which have been found to attenuate astrocytic glutamate uptake (Ye and Sontheimer, 1996) in turn.
Potassium and glutamate dysregulation are not ubiquitous in neuroinflammatory states or even in seizure disorders, however. For example, potassium currents were found to be unimpaired in a KA-induced TLE rat model which also exhibited increased gap junction coupling, and surprisingly, a more efficient transport cascade of glutamate (Takahashi et al., 2010). The authors further noted that the KA-treated rats that developed epilepsy displayed swifter synaptic glutamate clearance but no changes in GLT-1 or GLAST receptors [Notably, dysregulation associated with both upregulation and downregulation of EAAT1 and EAAT2 has been found in epilepsy (Coulter and Steinhaeuser, 2015; Hubbard et al., 2016)]. A potential mechanism could involve the degradation of ECM allowing for increased ionic diffusion as seen in the study of ECS diffusion previously mentioned (Syková, 2004). Lastly, Chaunsali et al. (2021) propose that the negative charge of PNNs plays a neuroprotective role by repelling negatively charged extracellular glutamate, thus opening neurons to glutamatergic excitotoxicity and possibly cell death when PNNs are degraded.
As PNNs are being altered, either within normal or inflammatory bounds, placement of astrocytes and thus the expression of EAAT receptors and Kir channels at the synapse are also changed. If these ECM structures are deficient or completely absent from the synapses and cannot regulate K+ and glutamate as per their standard role, the potential excitotoxicity of excess ionic concentrations in the ECS could easily shift the balance of not only individual neuronal excitation but overall circuit and brain excitation as well.
10 Membrane capacitance and intrinsic neuronal properties
Considering that PNNs preferentially surround PV+, GABAergic inhibitory interneurons, it is reasonable to hypothesize that altering the surrounding PNN affects the functionality or inherent capabilities and characteristics of these fast-spiking cells (Brückner et al., 1993; Härtig et al., 1999). In exploring the biophysical properties of PV+ FSNs, we and others were surprised to find that enzymatic removal or glioma-associated loss of PNNs altered the characteristics of the enveloped cells, resulting in decreased excitation as well as a decrease in cell membrane capacitance (Balmer, 2016; Tewari et al., 2018). This has resulted in the hypothesis that PNNs act as an insulator, reducing the specific membrane capacitance of the cell in a myelin-like manner to enable extremely high firing rates. A more recent study found that degradation of PNNs disrupts not only the PV+ cells themselves but also their role of stabilizing local circuits and network activity in the medial entorhinal cortex (Christensen et al., 2021). Thus, PNNs appear to be required for consistent, fast firing of inhibitory neurons, and their degradation (i.e., by astrocytically-released remodeling agents, or by changes in the primarily astrocytically-secreted ECM components such as brevican, neurocan, versican, HAPLNs, and TnR) can lead to decreased inhibition, resulting in asynchronized local network activity and overall circuit hyperexcitability.
Following these surprising findings, further exploration of the broader applicability of PNNs’ effects on firing properties and capacitance is required. For one, individual PNN constituents may have biophysical property-altering effects. An early mouse model of brevican depletion, for example, showed minimal abnormalities in PNNs but significant deficiencies in hippocampal LTP (Brakebusch et al., 2002), which may be attributed to brevican’s influence on AMPAR and K+ channel localization (Favuzzi et al., 2017). Altering brevican in a PV+ cell results in altered electrophysiological patterns, wherein its presence correlates with higher numbers of excitatory synaptic inputs, and deletion increases the intrinsic excitability of the cell by lowering the action potential threshold and decreasing latency to firing (Favuzzi et al., 2017). Interestingly, the authors additionally observed that resected human tissue from TLE patients also expressed decreased brevican levels in the cortex, further implicating loss of brevican in altered excitability in TLE.
Degradation of PNNs can alter biophysical cell properties in more than one way, however. Fragments of CSPGs, as discussed previously, can trigger immune responses, and one study found that free CS proteins can also trigger cell depolarization in rat hippocampal neurons in vitro via AMPA and kainate receptors (Maroto et al., 2013). The authors posit that injury or pathology-induced MMP degradation of CSPGs releases free CSs to effectuate Ca2+ signal via AMPARs in order to facilitate cell migratory or axonal regrowth.
PNN-enveloped PV+ interneurons thus appear to rely on their PNN coatings to maintain normal physiological functions including cell membrane capacitance and firing rate, not to mention ion channel and receptor localization. As such, we suggest that abnormal PNN-astrocyte interactions brought about by neuroinflammation can alter these properties, and may further induce neuroinflammatory reactions themselves, thus feeding back into an epileptogenic brain environment.
11 Discussion
Astrocytes and PNNs interact to induce and increase neuroinflammation, leading to a susceptibility to or increase in seizures and epilepsy. We have summarized three ways in which they interact, suggesting that altered synaptic placement, ionic buffering, and biophysical cellular properties such as capacitance can influence and be influenced by neuroinflammation, and thus contribute to epileptogenesis (Figure 3). This is not a completely new hypothesis; in fact, it has been proposed that even just the composition of the ECM determines astrocyte responses to mechanical and inflammatory stimuli (Johnson et al., 2015). This is not to say that neuroinflammation in and of itself is necessary and sufficient to cause epileptic activity, but we suggest it serves as a key contributor to the process. We further propose that dysfunctional interactions between PNNs and astrocytes can serve as a feedback loop, inducing and/or enhancing neuroinflammation-thus potentially acting as both a cause and consequence of epilepsy.
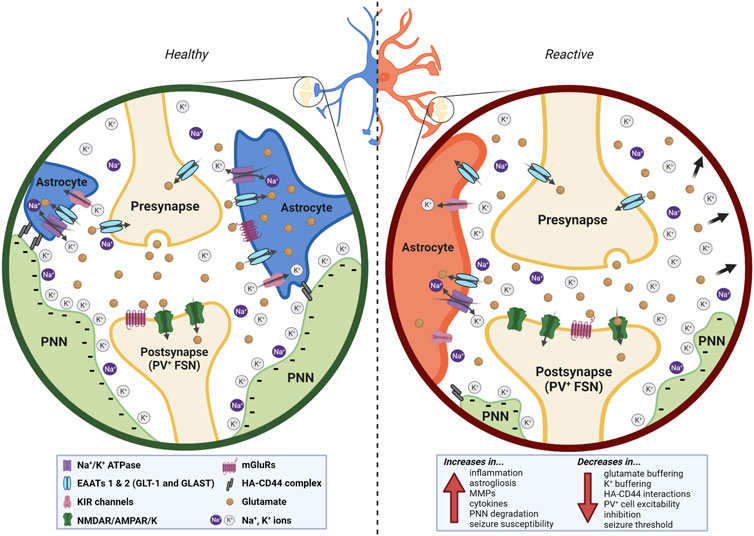
FIGURE 3. In the healthy brain (left), astrocytes and ECM interact at multiple levels. The presence of the PNN around the PV+ FSN postsynaptic bouton of the synapse helps to stabilize the astrocytic leaflets and serves as a highly anionic structure that redistributes and buffers cations released in and around the synaptic cleft. Glutamate, K+, and Na+ are sufficiently redistributed and taken up. The PNN additionally functions as insulation to the FSN it surrounds. In a neuroinflammatory state (right), astrocytes and PNNs interact abnormally due to the degradation of PNNs and the reactivity of the astrocytes, resulting in a variety of both upregulated and downregulated effects (bottom right) that stem from and contribute to the neuroinflammatory state. Created with Biorender.com.
11.1 Controversies and fundamental concepts, issues, and problems
At this point, there appears general agreement that PNNs are crucial for synaptic function and retention of memory, and that removal of or otherwise diminished PNNs can be associated with a variety of brain disorders. Whether PNNs are altered in neurodegenerative disorders specifically has historically been controversial. A recent study (Crapser et al., 2020b) has provided strong evidence for microglial engulfment of PNNs in AD, further showing that induction of an inflammatory state using LPS injections induced similar PNN-degrading phenotypes in wild type mice. There have also been a number of studies looking at alterations of PNNs in psychiatric disorders in particular [see (Carceller et al., 2022) for a thorough review].
Due to the inflammation aspect of our hypothesis, although it was not discussed, other immune cells such as microglia certainly have their own interactions with astrocytes and PNNs. As mentioned above, Crapser et al. (2020b) found that activated microglia are heavily implicated in PNN degradation, whether directly or indirectly, and another 2020 study (Nguyen et al., 2020) found that cytokine IL-33 released by hippocampal neurons induces microglial ECM remodeling. Astrocytically-released IL-33 has also been found to drive synaptic engulfment by microglia (Vainchtein et al., 2018) and microglial activation has been linked to epilepsy in general (Shapiro et al., 2008; Hiragi et al., 2018) [see (Andoh and Koyama, 2021) for review of microglia and plasticity]. Another paper looking specifically at depletion of microglia in Huntington’s disease found that knockout of microglia resulted in decreased PNN degradation, with denser PNN expression in all brain regions as well as reduced astrogliosis (Crapser et al., 2020a).
The time course of all this PNN remodeling may still be up for debate as well; one study suggests PNN modification occurs during each sleep cycle, varying with circadian rhythms (Pantazopoulos et al., 2020). However, such a quick turnaround of PNN degradation and production would likely have larger implications in multiple disease states as well as in healthy brains, where again, PNNs appear to play critical roles in plasticity and stability and are required for the normal function of the enveloped PV+ FSNs. A more recent study observed that although PNN expression did not change diurnally, it does increase in the absence of microglia, which display changes in ramification during the circadian cycle in mice (Barahona et al., 2022). If PNN integrity does in fact alter every 24-h cycle, the question of how normal brain function is maintained-especially with regards to inhibitory neuronal activity from FSNs-comes to the forefront.
Lastly, although touched upon earlier, the physiology of reactive astrocytes and the classification of such has continued to be controversial. Formerly considered in more binary terms such as “reactive” versus “nonreactive,” or “neuroprotective” vs. “neurotoxic,” astrocytes are now more likely to be classified holistically and along a continuum, categorized by their morphological, functional, and molecular changes, as well as taking into consideration their immunoreactivity markers and the brain regions they are expressed in, amongst others factors (Escartin et al., 2021).
11.2 Current research gaps and potential developments in the field
To fully explore this hypothesis, designing experiments to artificially alter the proposed PNN-astrocyte interactions is the crucial next step. Although there are enzymes that can be applied to degrade ECM and PNNs in vitro and in vivo and a viable aggrecan knockout mouse has been developed (Rowlands et al., 2018), there is currently no method of artificially inducing ECM growth or PNN formation. As discussed above, removal of or interfering with normal microglia function results in more highly condensed, intense, or concentrated PNNs (Liu et al., 2021; Barahona et al., 2022), but does not appear to result in de novo synthesis of the structures. To this end, the advent of a true PNN synthesis method would be a significant step towards truly confirming and/or revealing the roles of these structures in healthy and diseased brains alike.
One of the other stumbling blocks in determining the purposes and characteristics of PNNs and ECM in general is the fact that so many molecules comprise these complex structures that it becomes difficult to study. However, efforts to analyze and replicate its complexity have resulted in widely used biomaterials like basement membrane-like matrix (Matrigel) (Benton et al., 2011) and a variety of ECM-based polymers used for 3D modeling [see (Vigier, 2016) for a thorough review].
In addition to therapeutically targeting PNNs and the ECM to treat disorders such as epilepsy and AD, some suggest that manipulating these structures may be a potential anti-aging technique (Yang et al., 2021). As discussed previously, removal of PNNs using ChABC or hyase can restore the plasticity of the brain to critical period-like levels, implicating careful “editing” of the brain as a way to potentially mitigate or rewind the effects of age on memory formation and retention.
12 Summary
Epilepsy is a complex neurodegenerative disorder characterized by spontaneous, recurrent seizure activity, often expressed differently in every individual who suffers from it. The understanding of this disorder and its underlying causes is progressing, but it is inherently intricate and there are likely untold number of variables that contribute to epileptogenesis. Although neuroinflammation is only a part of the whole picture, we propose that astrocyte-PNN interactions both contribute to and result from neuroinflammation, thus exacerbating and enhancing epilepsy pathology and providing both a novel perspective as well as a potential therapeutic direction.
Author contributions
HS conceived of idea, supervised project and acquired funding. AW conducted literature review, created figures, and wrote outline, draft, and manuscript. All authors contributed to the article and approved the submitted version.
Funding
This work was supported by the National Institutes of Health grants R01NS036692 and R01AG065836 awarded to HS.
Conflict of interest
The authors declare that the research was conducted in the absence of any commercial or financial relationships that could be construed as a potential conflict of interest.
Publisher’s note
All claims expressed in this article are solely those of the authors and do not necessarily represent those of their affiliated organizations, or those of the publisher, the editors and the reviewers. Any product that may be evaluated in this article, or claim that may be made by its manufacturer, is not guaranteed or endorsed by the publisher.
Abbreviations
AD, Alzheimer disease; ADAMTSs, a disintegrin and metalloproteinase with thrombospondin motifs; AMPA, α-amino-3-hydroxy-5-methyl-4-isoxazolepropionic acid; CA1, CA2, cornu ammonis 1 and 2; CCL, chemokine C-C motif ligands; ChABC, chondroitinase ABC; CSPG, chondroitin sulfate proteoglycan; ECM, extracellular matrix; ECS, extracellular space; EEG, electroencephalogram; EPSC, EPSP, excitatory post-synaptic current or potential; FSN, fast-spiking neuron; GABA, γ-aminobutyric acid; HA, hyaluronic acid; Hapln, hyaluronan and proteoglycan link; HS, hippocampal sclerosis; IL, interleukin; LPS, lipopolysaccharide; MMP, matrix metalloproteinase; MTLE, medial TLE; PNN, perineuronal net; PTZ, pentylenetetrazol; PV, parvalbumin; TBI, traumatic brain injury; TLE, temporal lobe epilepsy; TLR, toll-like receptor; TNF-α, tumor necrosis factor α; WFA, Wisteria floribunda agglutinin.
References
Abdul-Muneer, P., Pfister, B., Haorah, J., and Chandra, N. (2016). Role of matrix metalloproteinases in the pathogenesis of traumatic brain injury. Mol. Neurobiol. 53, 6106–6123. doi:10.1007/s12035-015-9520-8
Akol, I., Kalogeraki, E., Pielecka-Fortuna, J., Fricke, M., and Löwel, S. (2022). MMP2 and MMP9 activity is crucial for adult visual cortex plasticity in healthy and stroke-affected mice. J. Neurosci. 42, 16–32. doi:10.1523/JNEUROSCI.0902-21.2021
Akyuz, E., Koklu, B., Uner, A., Angelopoulou, E., and Paudel, Y. N. (2022). Envisioning the role of inwardly rectifying potassium (Kir) channel in epilepsy. J. Neurosci. Res. 100, 413–443. doi:10.1002/jnr.24985
Alcaide, J., Guirado, R., Crespo, C., Blasco-Ibáñez, J. M., Varea, E., Sanjuan, J., et al. (2019). Alterations of perineuronal nets in the dorsolateral prefrontal cortex of neuropsychiatric patients. Int. J. Bipolar Disord. 1, 24. doi:10.1186/s40345-019-0161-0
Aldinucci, D., Borghese, C., and Casagrande, N. (2020). The CCL5/CCR5 Axis in cancer progression. Cancers 12, 1765. doi:10.3390/cancers12071765
Allnoch, L., Leitzen, E., Zdora, I., Baumgärtner, W., and Hansmann, F. (2022). Astrocyte depletion alters extracellular matrix composition in the demyelinating phase of Theiler’s murine encephalomyelitis. PLoS ONE 17, e0270239. doi:10.1371/journal.pone.0270239
Andoh, M., and Koyama, R. (2021). Microglia regulate synaptic development and plasticity. Dev. Neurobiol. 81, 568–590. doi:10.1002/dneu.22814
Arisi, G. M., Foresti, M. L., Katki, K., and Shapiro, L. A. (2015). Increased CCL2, CCL3, CCL5, and IL-1β cytokine concentration in piriform cortex, hippocampus, and neocortex after pilocarpine-induced seizures. J. Neuroinflammation 12, 129. doi:10.1186/s12974-015-0347-z
Aronica, E., Ravizza, T., Zurolo, E., and Vezzani, A. (2012). Astrocyte immune responses in epilepsy. GLIA 60, 1258–1268. doi:10.1002/glia.22312
Arpino, V., Brock, M., and Gill, S. E. (2015). The role of TIMPs in regulation of extracellular matrix proteolysis. Mater. Extracell. Matrix Biol. 44–46, 247–254. doi:10.1016/j.matbio.2015.03.005
Arranz, A. M., Perkins, K. L., Irie, F., Lewis, D. P., Hrabe, J., Xiao, F., et al. (2014). Hyaluronan deficiency due to Has3 knock-out causes altered neuronal activity and seizures via reduction in brain extracellular space. J. Neurosci. 34, 6164–6176. doi:10.1523/JNEUROSCI.3458-13.2014
Aulická, S., Česká, K., Šána, J., Siegl, F., Brichtová, E., Ošlejšková, H., et al. (2022). Cytokine-chemokine profiles in the hippocampus of patients with mesial temporal lobe epilepsy and hippocampal sclerosis. Epilepsy Res. 180, 106858. doi:10.1016/j.eplepsyres.2022.106858
Baig, S., Wilcock, G. K., and Love, S. (2005). Loss of perineuronal net N-acetylgalactosamine in Alzheimer’s disease. Acta Neuropathol. (Berl.) 110, 393–401. doi:10.1007/s00401-005-1060-2
Balashova, A., Pershin, V., Zaborskaya, O., Tkachenko, N., Mironov, A., et al. (2019). Enzymatic digestion of hyaluronan-based brain extracellular matrix in vivo can induce seizures in neonatal mice. Front. Neurosci. 13, 1033. doi:10.3389/fnins.2019.01033
Balmer, T. S. (2016). Perineuronal nets enhance the excitability of fast-spiking neurons. eNeuro 3, ENEURO.0112. doi:10.1523/ENEURO.0112-16.2016
Balosso, S., Liu, J., Bianchi, M. E., and Vezzani, A. (2014). Disulfide-containing high mobility group box-1 promotes N-methyl-D-aspartate receptor function and excitotoxicity by activating Toll-like receptor 4-dependent signaling in hippocampal neurons. Antioxid. Redox Signal. 21, 1726–1740. doi:10.1089/ars.2013.5349
Balosso, S., Maroso, M., Sanchez-Alavez, M., Ravizza, T., Frasca, A., Bartfai, T., et al. (2008). A novel non-transcriptional pathway mediates the proconvulsive effects of interleukin-1beta. Brain J. Neurol. 131, 3256–3265. doi:10.1093/brain/awn271
Barahona, R. A., Morabito, S., Swarup, V., and Green, K. N. (2022). Cortical diurnal rhythms remain intact with microglial depletion. Sci. Rep. 12, 114. doi:10.1038/s41598-021-04079-w
Bedner, P., Dupper, A., Hüttmann, K., Müller, J., Herde, M. K., Dublin, P., et al. (2015). Astrocyte uncoupling as a cause of human temporal lobe epilepsy. Brain J. Neurol. 138, 1208–1222. doi:10.1093/brain/awv067
Benton, G., Kleinman, H. K., George, J., and Arnaoutova, I. (2011). Multiple uses of basement membrane-like matrix (BME/Matrigel) in vitro and in vivo with cancer cells. Int. J. Cancer 128, 1751–1757. doi:10.1002/ijc.25781
Berezin, V., Walmod, P. S., Filippov, M., and Dityatev, A. (2014). “Chapter 15 - targeting of ECM molecules and their metabolizing enzymes and receptors for the treatment of CNS diseases,” in Progress in brain research. Editors A. Dityatev, B. Wehrle-Haller, and A. Pitkänen (Netherlands: Elsevier), 353–388. doi:10.1016/B978-0-444-63486-3.00015-3
Beurdeley, M., Spatazza, J., Lee, H. H. C., Sugiyama, S., Bernard, C., Di Nardo, A. A., et al. (2012). Otx2 binding to perineuronal nets persistently regulates plasticity in the mature visual cortex. J. Neurosci. 32, 9429–9437. doi:10.1523/JNEUROSCI.0394-12.2012
Blair, R. D. G. (2012). Temporal lobe epilepsy semiology. Epilepsy Res. Treat. 2012, 751510. doi:10.1155/2012/751510
Blondiaux, A., Jia, S., Friscknecht, R., Fejtova, A., Dityatev, A., Gundelfinger, E., et al. (2020). Modulation of the hyaluronan-based extracellular matrix in mouse models of epilepsy. Berlin, Germany: Springer. doi:10.13140/RG.2.2.19273.77929
Blosa, M., Bursch, C., Weigel, S., Holzer, M., Jäger, C., Janke, C., et al. 2016. Reorganization of synaptic connections and perineuronal nets in the deep cerebellar nuclei of purkinje cell degeneration mutant mice. Neural Plast. 2016, 2828536. doi:10.1155/2016/2828536
Blutstein, T., and Haydon, P. G. (2014). “Chapter five - the tripartite synapse: A role for glial cells in modulating synaptic transmission,” in The synapse. Editors V. Pickel, and M. Segal (Boston: Academic Press), 155–172. doi:10.1016/B978-0-12-418675-0.00005-5
Bonneh-Barkay, D., and Wiley, C. A. (2009). Brain extracellular matrix in neurodegeneration. Brain Pathol. Zurich Switz. 19, 573–585. doi:10.1111/j.1750-3639.2008.00195.x
Borges, K., Gearing, M., McDermott, D. L., Smith, A. B., Almonte, A. G., Wainer, B. H., et al. (2003). Neuronal and glial pathological changes during epileptogenesis in the mouse pilocarpine model. Exp. Neurol. 182, 21–34. doi:10.1016/S0014-4886(03)00086-4
Bosiacki, M., Gąssowska-Dobrowolska, M., Kojder, K., Fabiańska, M., Jeżewski, D., Gutowska, I., et al. (2019). Perineuronal nets and their role in synaptic homeostasis. Int. J. Mol. Sci. 20, 4108. doi:10.3390/ijms20174108
Bozzelli, P. L., Alaiyed, S., Kim, E., Villapol, S., and Conant, K., (2018). Proteolytic remodeling of perineuronal nets: Effects on synaptic plasticity and neuronal population dynamics. Neural Plast. 2018, 5735789. doi:10.1155/2018/5735789
Bradbury, E. J., Moon, L. D. F., Popat, R. J., King, V. R., Bennett, G. S., Patel, P. N., et al. (2002). Chondroitinase ABC promotes functional recovery after spinal cord injury. Nature 416, 636–640. doi:10.1038/416636a
Brakebusch, C., Seidenbecher, C. I., Asztely, F., Rauch, U., Matthies, H., Meyer, H., et al. (2002). Brevican-deficient mice display impaired hippocampal CA1 long-term potentiation but show no obvious deficits in learning and memory. Mol. Cell. Biol. 22, 7417–7427. doi:10.1128/MCB.22.21.7417-7427.2002
Brown, T. E., and Sorg, B. A. (2022). Net gain and loss: Influence of natural rewards and drugs of abuse on perineuronal nets. Neuropsychopharmacology 48, 3–20. doi:10.1038/s41386-022-01337-x
Brückner, G., Härtig, W., Seeger, J., Rübsamen, R., Reimer, K., and Brauer, K. (1998). Cortical perineuronal nets in the gray short-tailed opossum (Monodelphis domestica): A distribution pattern contrasting with that shown in placental mammals. Anat. Embryol. (Berl.) 197, 249–262. doi:10.1007/s004290050135
Brückner, G., Brauer, K., Härtig, W., Wolff, J. R., Rickmann, M. J., Derouiche, A., et al. (1993). Perineuronal nets provide a polyanionic, glia-associated form of microenvironment around certain neurons in many parts of the rat brain. Glia 8, 183–200. doi:10.1002/glia.440080306
Buckingham, S. C., Campbell, S. L., Haas, B. R., Montana, V., Robel, S., Ogunrinu, T., et al. (2011). Glutamate release by primary brain tumors induces epileptic activity. Nat. Med. 17, 1269–1274. doi:10.1038/nm.2453
Buckingham, S. C., and Robel, S. (2013). Glutamate and tumor-associated epilepsy: Glial cell dysfunction in the peritumoral environment. Neurochem. Int., Special issue Glial cells epilepsy 63, 696–701. doi:10.1016/j.neuint.2013.01.027
Cabungcal, J.-H., Steullet, P., Morishita, H., Kraftsik, R., Cuenod, M., Hensch, T. K., et al. (2013). Perineuronal nets protect fast-spiking interneurons against oxidative stress. Proc. Natl. Acad. Sci. U. S. A. 110, 9130–9135. doi:10.1073/pnas.1300454110
Campbell, S. C., Muñoz-Ballester, C., Chaunsali, L., Mills, W. A., Yang, J. H., Sontheimer, H., et al. (2020). Potassium and glutamate transport is impaired in scar-forming tumor-associated astrocytes. Neurochem. Int. 133, 104628. doi:10.1016/j.neuint.2019.104628
Canto, A. M., Godoi, A. B., Matos, A. H. B., Geraldis, J. C., Rogerio, F., Alvim, M. K. M., et al. (2022). Benchmarking the proteomic profile of animal models of mesial temporal epilepsy. Ann. Clin. Transl. Neurol. 9, 454–467. doi:10.1002/acn3.51533
Carceller, H., Gramuntell, Y., Klimczak, P., and Nacher, J. (2022). Perineuronal nets: Subtle structures with large implications. Neurosci. 2022, 107385842211063. doi:10.1177/10738584221106346
Carstens, K. E., Phillips, M. L., Pozzo-Miller, L., Weinberg, R. J., and Dudek, S. M. (2016). Perineuronal nets suppress plasticity of excitatory synapses on CA2 pyramidal neurons. J. Neurosci. 36, 6312–6320. doi:10.1523/JNEUROSCI.0245-16.2016
Carulli, D., Pizzorusso, T., Kwok, J. C. F., Putignano, E., Poli, A., Forostyak, S., et al. (2010). Animals lacking link protein have attenuated perineuronal nets and persistent plasticity. Brain 133, 2331–2347. doi:10.1093/brain/awq145
Carulli, D., Rhodes, K. E., Brown, D. J., Bonnert, T. P., Pollack, S. J., Oliver, K., et al. (2006). Composition of perineuronal nets in the adult rat cerebellum and the cellular origin of their components. J. Comp. Neurol. 494, 559–577. doi:10.1002/cne.20822
Celio, M. R., and Blumcke, I. (1994). Perineuronal nets — A specialized form of extracellular matrix in the adult nervous system. Brain Res. Rev. 19, 128–145. doi:10.1016/0165-0173(94)90006-X
Chakravarthy, A., Khan, L., Bensler, N. P., Bose, P., and De Carvalho, D. D. (2018). TGF-β-associated extracellular matrix genes link cancer-associated fibroblasts to immune evasion and immunotherapy failure. Nat. Commun. 9, 4692. doi:10.1038/s41467-018-06654-8
Chaunsali, L., Tewari, B. P., and Sontheimer, H. (2021). Perineuronal net dynamics in the pathophysiology of epilepsy. Epilepsy Curr. 21, 273–281. doi:10.1177/15357597211018688
Chelyshev, Y. A., Kabdesh, I. M., and Mukhamedshina, Y. O. (2022). Extracellular matrix in neural plasticity and regeneration. Cell. Mol. Neurobiol. 42, 647–664. doi:10.1007/s10571-020-00986-0
Chen, W., Li, Y. S., Gao, J., Lin, X. Y., and Li, X. H. (2016). AMPA receptor antagonist NBQX decreased seizures by normalization of perineuronal nets. PloS One 11, 01666722–e166716. doi:10.1371/journal.pone.0166672
Chiquet-Ehrismann, R., and Chiquet, M. (2003). Tenascins: Regulation and putative functions during pathological stress. J. Pathol. 200, 488–499. doi:10.1002/path.1415
Chistyakov, D. V., Astakhova, A. A., Azbukina, N. V., Goriainov, S. V., Chistyakov, V. V., and Sergeeva, M. G. (2019). High and low molecular weight hyaluronic acid differentially influences oxylipins synthesis in course of neuroinflammation. Int. J. Mol. Sci. 20, 3894. doi:10.3390/ijms20163894
Christensen, A. C., Lensjø, K. K., Lepperød, M. E., Dragly, S.-A., Sutterud, H., Blackstad, J. S., et al. (2021). Perineuronal nets stabilize the grid cell network. Nat. Commun. 12, 253. doi:10.1038/s41467-020-20241-w
Christopherson, K. S., Ullian, E. M., Stokes, C. C. A., Mullowney, C. E., Hell, J. W., Agah, A., et al. (2005). Thrombospondins are astrocyte-secreted proteins that promote CNS synaptogenesis. Cell 120, 421–433. doi:10.1016/j.cell.2004.12.020
Chung, W.-S., Allen, N. J., and Eroglu, C. (2015). Astrocytes control synapse formation, function, and elimination. Cold Spring Harb. Perspect. Biol. 7, a020370. doi:10.1101/cshperspect.a020370
Cieplak, P., and Strongin, A. Y. (2017). Matrix metalloproteinases – from the cleavage data to the prediction tools and beyond. Matrix Met. 1864, 1952–1963. doi:10.1016/j.bbamcr.2017.03.010
Cornez, G., Collignon, C., Cornil, C. A., Balthazart, J., Müller, W., and Ball, G. F. (2020). Seasonal changes of perineuronal nets and song learning in adult canaries (Serinus canaria). Behav. Brain Res. 380, 112437. doi:10.1016/j.bbr.2019.112437
Cornez, G., Jonckers, E., Ter Haar, S. M., Van Der Linden, A., Cornil, C. A., and Balthazart, J. (2018). Timing of perineuronal net development in the zebra finch song control system correlates with developmental song learning. Proc. R. Soc. B Biol. Sci. 285, 20180849. doi:10.1098/rspb.2018.0849
Coulter, D. A., and Steinhaeuser, C. (2015). Role of astrocytes in epilepsy. COLD SPRING Harb. Perspect. Med. 5, a022434. doi:10.1101/cshperspect.a022434
Crapser, J. D., Spangenberg, E. E., Barahona, R. A., Arreola, M. A., Hohsfield, L. A., and Green, K. N. (2020b). Microglia facilitate loss of perineuronal nets in the Alzheimer’s disease brain. EBioMedicine 58, 102919. doi:10.1016/j.ebiom.2020.102919
Crapser, J. D., Spangenberg, E. E., Barahona, R. A., Arreola, M. A., Hohsfield, L. A., and Green, K. N. (2020a). Microglial depletion prevents extracellular matrix changes and striatal volume reduction in a model of Huntington’s disease. Brain J. Neurol. 143, 266–288. doi:10.1093/brain/awz363
Crawford, D. C., Jiang, X., Taylor, A., and Mennerick, S. (2012). Astrocyte-derived thrombospondins mediate the development of hippocampal presynaptic plasticity in vitro. J. Neurosci. Off. J. Soc. Neurosci. 32, 13100–13110. doi:10.1523/JNEUROSCI.2604-12.2012
Crespel, A., Coubes, P., Rousset, M.-C., Brana, C., Rougier, A., Rondouin, G., et al. (2002). Inflammatory reactions in human medial temporal lobe epilepsy with hippocampal sclerosis. Brain Res. 952, 159–169. doi:10.1016/s0006-8993(02)03050-0
Crijns, H., Vanheule, V., and Proost, P. (2020). Targeting chemokine--glycosaminoglycan interactions to inhibit inflammation. Front. Immunol. 11, 483. doi:10.3389/fimmu.2020.00483
Das, A., Wallace, I. G. C., Holmes, C., McDowell, M. L., Smith, J. A., Marshall, J. D., et al. (2012). Hippocampal tissue of patients with refractory temporal lobe epilepsy is associated with astrocyte activation, inflammation, and altered expression of channels and receptors. Neuroscience 220, 237–246. doi:10.1016/j.neuroscience.2012.06.002
David, Y., Cacheaux, L. P., Ivens, S., Lapilover, E., Heinemann, U., Kaufer, D., et al. (2009). Astrocytic dysfunction in epileptogenesis: Consequence of altered potassium and glutamate homeostasis? J. Neurosci. Off. J. Soc. Neurosci. 29, 10588–10599. doi:10.1523/JNEUROSCI.2323-09.2009
De Luca, C., and Papa, M. (2016). Looking inside the matrix: Perineuronal nets in plasticity, maladaptive plasticity and neurological disorders. Neurochem. Res. 41, 1507–1515. doi:10.1007/s11064-016-1876-2
De Simoni, M. G., Perego, C., Ravizza, T., Moneta, D., Conti, M., Marchesi, F., et al. (2000). Inflammatory cytokines and related genes are induced in the rat hippocampus by limbic status epilepticus. Eur. J. Neurosci. 12, 2623–2633. doi:10.1046/j.1460-9568.2000.00140.x
DeSena, A. D., Do, T., and Schulert, G. S. (2018). Systemic autoinflammation with intractable epilepsy managed with interleukin-1 blockade. J. Neuroinflammation 15, 38. doi:10.1186/s12974-018-1063-2
Devinsky, O., Vezzani, A., Najjar, S., De Lanerolle, N. C., and Rogawski, M. A. (2013). Glia and epilepsy: Excitability and inflammation. Trends Neurosci. 36, 174–184. doi:10.1016/j.tins.2012.11.008
Dityatev, A., and Rusakov, D. A. (2011). Molecular signals of plasticity at the tetrapartite synapse. Curr. Opin. Neurobiol. 21, 353–359. doi:10.1016/j.conb.2010.12.006
Dityatev, A., and Schachner, M. (2003). Extracellular matrix molecules and synaptic plasticity. Nat. Rev. Neurosci. 4, 456–468. doi:10.1038/nrn1115
Dityatev, A., and Schachner, M. (2006). The extracellular matrix and synapses. Cell Tissue Res. 326, 647–654. doi:10.1007/s00441-006-0217-1
Dityatev, A., Seidenbecher, C. I., and Schachner, M. (2010). Compartmentalization from the outside: The extracellular matrix and functional microdomains in the brain. Trends Neurosci. 33, 503–512. doi:10.1016/j.tins.2010.08.003
Djukic, B., Casper, K. B., Philpot, B. D., Chin, L.-S., and McCarthy, K. D. (2007). Conditional knock-out of Kir4.1 leads to glial membrane depolarization, inhibition of potassium and glutamate uptake, and enhanced short-term synaptic potentiation. J. Neurosci. 27, 11354–11365. doi:10.1523/JNEUROSCI.0723-07.2007
Domowicz, M. S., Sanders, T. A., Ragsdale, C. W., and Schwartz, N. B. (2008). Aggrecan is expressed by embryonic brain glia and regulates astrocyte development. Dev. Biol. 315, 114–124. doi:10.1016/j.ydbio.2007.12.014
Dubey, D., McRae, P. A., Rankin-Gee, E. K., Baranov, E., Wandrey, L., Rogers, S., et al. (2017). Increased metalloproteinase activity in the hippocampus following status epilepticus. Epilepsy Res. 132, 50–58. doi:10.1016/j.eplepsyres.2017.02.021
Dzwonek, J., Rylski, M., and Kaczmarek, L. (2004). Matrix metalloproteinases and their endogenous inhibitors in neuronal physiology of the adult brain. FEBS Lett. 567, 129–135. doi:10.1016/j.febslet.2004.03.070
Dzyubenko, E., Fleischer, M., Manrique-Castano, D., Borbor, M., Kleinschnitz, C., Faissner, A., et al. (2021). Inhibitory control in neuronal networks relies on the extracellular matrix integrity. Cell. Mol. Life Sci. CMLS 78, 5647–5663. doi:10.1007/s00018-021-03861-3
Eid, T., Tu, N., Lee, T.-S. W., and Lai, J. C. K. (2013). Regulation of astrocyte glutamine synthetase in epilepsy. Neurochem. Int., Special issue Glial cells epilepsy 63, 670–681. doi:10.1016/j.neuint.2013.06.008
Eidson, L. N., Inoue, K., Young, L. J., Tansey, M. G., and Murphy, A. Z. (2017). Toll-like receptor 4 mediates morphine-induced neuroinflammation and tolerance via soluble tumor necrosis factor signaling. Neuropsychopharmacology 42, 661–670. doi:10.1038/npp.2016.131
Eroglu, C. (2009). The role of astrocyte-secreted matricellular proteins in central nervous system development and function. J. Cell Commun. Signal. 3, 167–176. doi:10.1007/s12079-009-0078-y
Escartin, C., Galea, E., Lakatos, A., O’Callaghan, J. P., Petzold, G. C., Serrano-Pozo, A., et al. (2021). Reactive astrocyte nomenclature, definitions, and future directions. Nat. Neurosci. 24. 312–325. doi:10.1038/s41593-020-00783-4
Escartin, C., Guillemaud, O., and Carrillo-de Sauvage, M.-A. (2019). Questions and (some) answers on reactive astrocytes. Glia 67, 2221–2247. doi:10.1002/glia.23687
Ethell, I. M., and Ethell, D. W. (2007). Matrix metalloproteinases in brain development and remodeling: Synaptic functions and targets. J. Neurosci. Res. 85, 2813–2823. doi:10.1002/jnr.21273
Fabene, P. F., Bramanti, P., and Constantin, G. (2010). The emerging role for chemokines in epilepsy. J. Neuroimmunol. 224, 22–27. doi:10.1016/j.jneuroim.2010.05.016
Favuzzi, E., Marques-Smith, A., Deogracias, R., Winterflood, C. M., Sánchez-Aguilera, A., Mantoan, L., et al. (2017). Activity-dependent gating of parvalbumin interneuron function by the perineuronal net protein brevican. Neuron 95, 639–655. doi:10.1016/j.neuron.2017.06.028
Fawcett, J. W., Fyhn, M., Jendelova, P., Kwok, J. C. F., Ruzicka, J., and Sorg, B. A. (2022). The extracellular matrix and perineuronal nets in memory. Mol. Psychiatry 27, 3192–3203. doi:10.1038/s41380-022-01634-3
Ferrer, I. (2017). Diversity of astroglial responses across human neurodegenerative disorders and brain aging. Brain Pathol. 27, 645–674. doi:10.1111/bpa.12538
Foresti, M. L., Arisi, G. M., Katki, K., Montañez, A., Sanchez, R. M., and Shapiro, L. A. (2009). Chemokine CCL2 and its receptor CCR2 are increased in the hippocampus following pilocarpine-induced status epilepticus. J. Neuroinflammation 6, 40. doi:10.1186/1742-2094-6-40
Frischknecht, R., and Gundelfinger, E. D. (2012). The brain’s extracellular matrix and its role in synaptic plasticity. Adv. Exp. Med. Biol. 970, 153–171. doi:10.1007/978-3-7091-0932-8_7
Frischknecht, R., Heine, M., Perrais, D., Seidenbecher, C. I., Choquet, D., and Gundelfinger, E. D. (2009). Brain extracellular matrix affects AMPA receptor lateral mobility and short-term synaptic plasticity. Nat. Neurosci. 12, 897–904. doi:10.1038/nn.2338
Galic, M. A., Riazi, K., Heida, J. G., Mouihate, A., Fournier, N. M., Spencer, S. J., et al. (2008). Postnatal inflammation increases seizure susceptibility in adult rats. J. Neurosci. Off. J. Soc. Neurosci. 28, 6904–6913. doi:10.1523/JNEUROSCI.1901-08.2008
Gao, X., Li, W., Syed, F., Yuan, F., Li, P., and Yu, Q. (2022). PD-L1 signaling in reactive astrocytes counteracts neuroinflammation and ameliorates neuronal damage after traumatic brain injury. J. NEUROINFLAMMATION 19, 43. doi:10.1186/s12974-022-02398-x
Gaudet, A. D., and Popovich, P. G. (2014). Extracellular matrix regulation of inflammation in the healthy and injured spinal cord. Exp. Neurol. 258, 24–34. doi:10.1016/j.expneurol.2013.11.020
Geissler, M., Gottschling, C., Aguado, A., Rauch, U., Wetzel, C. H., Hatt, H., et al. (2013). Primary hippocampal neurons, which lack four crucial extracellular matrix molecules, display abnormalities of synaptic structure and function and severe deficits in perineuronal net formation. J. Neurosci. Off. J. Soc. Neurosci. 33, 7742–7755. doi:10.1523/JNEUROSCI.3275-12.2013
Giamanco, K. A., and Matthews, R. T. (2012). Deconstructing the perineuronal net: Cellular contributions and molecular composition of the neuronal extracellular matrix. Neuroscience 218, 367–384. doi:10.1016/j.neuroscience.2012.05.055
Giamanco, K. A., Morawski, M., and Matthews, R. T. (2010). Perineuronal net formation and structure in aggrecan knockout mice. Neuroscience 170, 1314–1327. doi:10.1016/j.neuroscience.2010.08.032
Gibbons, M. B., Smeal, R. M., Takahashi, D. K., Vargas, J. R., and Wilcox, K. S. (2013). Contributions of astrocytes to epileptogenesis following status epilepticus: Opportunities for preventive therapy? Neurochem. Int., Special issue Glial cells epilepsy 63, 660–669. doi:10.1016/j.neuint.2012.12.008
Glykys, J., Dzhala, V., Egawa, K., Balena, T., Saponjian, Y., Kuchibhotla, K. V., et al. (2014). Local impermeant anions establish the neuronal chloride concentration. Science 343, 670–675. doi:10.1126/science.1245423
Golub, V. M., and Reddy, D. S. (2022). Contusion brain damage in mice for modelling of post-traumatic epilepsy with contralateral hippocampus sclerosis: Comprehensive and longitudinal characterization of spontaneous seizures, neuropathology, and neuropsychiatric comorbidities. Exp. Neurol. 348, 113946. doi:10.1016/j.expneurol.2021.113946
Gottschall, P. E., and Deb, S. (1996). Regulation of matrix metalloproteinase expressions in astrocytes, microglia and neurons. Neuroimmunomodulation 3, 69–75. doi:10.1159/000097229
Gundelfinger, E. D., Frischknecht, R., Choquet, D., and Heine, M. (2010). Converting juvenile into adult plasticity: A role for the brain’s extracellular matrix. Eur. J. Neurosci. 31, 2156–2165. doi:10.1111/j.1460-9568.2010.07253.x
Haist, V., Ulrich, R., Kalkuhl, A., Deschl, U., and Baumgärtner, W. (2012). Distinct spatio-temporal extracellular matrix accumulation within demyelinated spinal cord lesions in Theiler’s murine encephalomyelitis. Brain Pathol. Zurich Switz. 22, 188–204. doi:10.1111/j.1750-3639.2011.00518.x
Han, C.-L., Zhao, X.-M., Liu, Y.-P., Wang, K.-L., Chen, N., Hu, W., et al. (2019). Gene expression profiling of two epilepsy models reveals the ECM/integrin signaling pathway is involved in epiletogenesis. Neuroscience 396, 187–199. doi:10.1016/j.neuroscience.2018.10.021
Harkness, J. H., Gonzalez, A. E., Bushana, P. N., Jorgensen, E. T., Hegarty, D. M., Di Nardo, A. A., et al. (2021). Diurnal changes in perineuronal nets and parvalbumin neurons in the rat medial prefrontal cortex. Brain Struct. Funct. 226, 1135–1153. doi:10.1007/s00429-021-02229-4
Härtig, W., Appel, S., Suttkus, A., Grosche, J., and Michalski, D. (2016). Abolished perineuronal nets and altered parvalbumin-immunoreactivity in the nucleus reticularis thalami of wildtype and 3xTg mice after experimental stroke. Neuroscience 337, 66–87. doi:10.1016/j.neuroscience.2016.09.004
Härtig, W., Derouiche, A., Welt, K., Brauer, K., Grosche, J., Mäder, M., et al. (1999). Cortical neurons immunoreactive for the potassium channel Kv3.1b subunit are predominantly surrounded by perineuronal nets presumed as a buffering system for cations. Brain Res. 842, 15–29. doi:10.1016/S0006-8993(99)01784-9
Hayatdavoudi, P., Hosseini, M., Hajali, V., Hosseini, A., and Rajabian, A. (2022). The role of astrocytes in epileptic disorders. Physiol. Rep. 10, e15239. doi:10.14814/phy2.15239
Hensch, T. K. (2004). Critical period regulation. Annu. Rev. Neurosci. 27, 549–579. doi:10.1146/annurev.neuro.27.070203.144327
Hinz, B. (2015). The extracellular matrix and transforming growth factor-β1: Tale of a strained relationship. Matrix Biol. 47, 54–65. doi:10.1016/j.matbio.2015.05.006
Hiragi, T., Ikegaya, Y., and Koyama, R. (2018). Microglia after seizures and in epilepsy. Cells 7, 26. doi:10.3390/cells7040026
Hoffmann, K., Sivukhina, E., Potschka, H., Schachner, M., Löscher, W., and Dityatev, A. (2009). Retarded kindling progression in mice deficient in the extracellular matrix glycoprotein tenascin-R. Epilepsia 50, 859–869. doi:10.1111/j.1528-1167.2008.01774.x
Hösli, L., Binini, N., Ferrari, K. D., ThierenLooser, Z. J., Zuend, M., Zanker, H. S., et al. (2022). Decoupling astrocytes in adult mice impairs synaptic plasticity and spatial learning. Cell Rep. 38, 110484. doi:10.1016/j.celrep.2022.110484
Hou, X., Yoshioka, N., Tsukano, H., Sakai, A., Miyata, S., Watanabe, Y., et al. (2017). Chondroitin sulfate is required for onset and offset of critical period plasticity in visual cortex. Sci. Rep. 7, 12646. doi:10.1038/s41598-017-04007-x
Howell, M. D., Bailey, L. A., Cozart, M. A., Gannon, B. M., and Gottschall, P. E. (2015). Hippocampal administration of chondroitinase ABC increases plaque-adjacent synaptic marker and diminishes amyloid burden in aged APPswe/PS1dE9 mice. Acta Neuropathol. Commun. 3, 54. doi:10.1186/s40478-015-0233-z
Huang, W.-Y., Lai, Y.-L., Liu, K.-H., Lin, S., Chen, H.-Y., Liang, C.-H., et al. (2022). TNFα-mediated necroptosis in brain endothelial cells as a potential mechanism of increased seizure susceptibility in mice following systemic inflammation. J. Neuroinflammation 19, 29. doi:10.1186/s12974-022-02406-0
Hubbard, J. A., Szu, J. I., Yonan, J. M., and Binder, D. K. (2016). Regulation of astrocyte glutamate transporter-1 (GLT1) and aquaporin-4 (AQP4) expression in a model of epilepsy. Exp. Neurol. 283, 85–96. doi:10.1016/j.expneurol.2016.05.003
Hudson, C. A., Christophi, G. P., Gruber, R. C., Wilmore, J. R., Lawrence, D. A., and Massa, P. T. (2008). Induction of IL-33 expression and activity in central nervous system glia. J. Leukoc. Biol. 84, 631–643. doi:10.1189/jlb.1207830
Ikeshima-Kataoka, H., Saito, S., and Yuasa, S. (2007). Tenascin-C is required for proliferation of astrocytes in primary culture. Vivo 21, 629–633.
Inyushin, M., Kucheryavykh, L. Y., Kucheryavykh, Y. V., Nichols, C. G., Buono, R. J., Ferraro, T. N., et al. (2010). Potassium channel activity and glutamate uptake are impaired in astrocytes of seizure-susceptible DBA/2 mice. Epilepsia 51, 1707–1713. doi:10.1111/j.1528-1167.2010.02592.x
Ivanova, E., Costa, B., Eisemann, T., Lohr, S., Boskovic, P., Eichwald, V., et al. (2022). CD44 expressed by myeloid cells promotes glioma invasion. Front. Oncol. 12, 969787. doi:10.3389/fonc.2022.969787
Ivens, S., Kaufer, D., Flores, L. P., Bechmann, I., Zumsteg, D., Tomkins, O., et al. (2007). TGF-beta receptor-mediated albumin uptake into astrocytes is involved in neocortical epileptogenesis. Brain J. Neurol. 130, 535–547. doi:10.1093/brain/awl317
Jakovljević, A., Tucić, M., Blažiková, M., Korenić, A., Missirlis, Y., Stamenković, V., et al. (2021). Structural and functional modulation of perineuronal nets: In search of important players with highlight on tenascins. Cells 10, 1345. doi:10.3390/cells10061345
Jang, D. G., Sim, H. J., Song, E. K., Kwon, T., and Park, T. J. (2020). Extracellular matrixes and neuroinflammation. BMB Rep. 53, 491–499. doi:10.5483/BMBRep.2020.53.10.156
Johnson, K. M., Milner, R., and Crocker, S. J. (2015). Extracellular matrix composition determines astrocyte responses to mechanical and inflammatory stimuli. Neurosci. Lett. 600, 104–109. doi:10.1016/j.neulet.2015.06.013
Jones, E. V., and Bouvier, D. S., 2014. Astrocyte-secreted matricellular proteins in CNS remodelling during development and disease. Neural Plast. 2014, 321209. doi:10.1155/2014/321209
Kan, A. A., van der Hel, W. S., Kolk, S. M., Bos, I. W. M., Verlinde, S. A. M. W., van Nieuwenhuizen, O., et al. (2012). Prolonged increase in rat hippocampal chemokine signalling after status epilepticus. J. Neuroimmunol. 245, 15–22. doi:10.1016/j.jneuroim.2012.01.012
Karpuk, N., Burkovetskaya, M., Fritz, T., Angle, A., and Kielian, T. (2011). Neuroinflammation leads to region-dependent alterations in astrocyte gap junction communication and hemichannel activity. J. Neurosci. Off. J. Soc. Neurosci. 31, 414–425. doi:10.1523/JNEUROSCI.5247-10.2011
Karus, M., Denecke, B., ffrench-Constant, C., Wiese, S., and Faissner, A. (2011). The extracellular matrix molecule tenascin C modulates expression levels and territories of key patterning genes during spinal cord astrocyte specification. Dev. Camb. Engl. 138, 5321–5331. doi:10.1242/dev.067413
Kelly, E. A., Russo, A. S., Jackson, C. D., Lamantia, C. E., and Majewska, A. K. (2015). Proteolytic regulation of synaptic plasticity in the mouse primary visual cortex: Analysis of matrix metalloproteinase 9 deficient mice. Front. Cell. Neurosci. 9, 369. doi:10.3389/fncel.2015.00369
Kelwick, R., Desanlis, I., Wheeler, G. N., and Edwards, D. R. (2015). The ADAMTS (A disintegrin and metalloproteinase with thrombospondin motifs) family. Genome Biol. 16, 113. doi:10.1186/s13059-015-0676-3
Khakh, B. S., and Sofroniew, M. V. (2015). Diversity of astrocyte functions and phenotypes in neural circuits. Nat. Neurosci. 18, 942–952. doi:10.1038/nn.4043
Khoo, G. H., Lin, Y.-T., Tsai, T.-C., and Hsu, K.-S. (2019). Perineuronal nets restrict the induction of long-term depression in the mouse hippocampal CA1 region. Mol. Neurobiol. 56, 6436–6450. doi:10.1007/s12035-019-1526-1
Kim, S. Y., Porter, B. E., Friedman, A., and Kaufer, D. (2016). A potential role for glia-derived extracellular matrix remodeling in postinjury epilepsy. J. Neurosci. Res. 94, 794–803. doi:10.1002/jnr.23758
Kim, S. Y., Senatorov, V. V., Morrissey, C. S., Lippmann, K., Vazquez, O., Milikovsky, D. Z., et al. (2017). TGFβ signaling is associated with changes in inflammatory gene expression and perineuronal net degradation around inhibitory neurons following various neurological insults. Sci. Rep. 7, 7711. doi:10.1038/s41598-017-07394-3
Kinboshi, M., Ikeda, A., and Ohno, Y. (2020). Role of astrocytic inwardly rectifying potassium (Kir) 4.1 channels in epileptogenesis. Front. Neurol. 11, 626658. doi:10.3389/fneur.2020.626658
Klein, T., and Bischoff, R. (2011). Physiology and pathophysiology of matrix metalloproteases. Amino Acids 41, 271–290. doi:10.1007/s00726-010-0689-x
Kołosowska, K., Maciejak, P., Szyndler, J., Turzyńska, D., Sobolewska, A., and Płaźnik, A. (2016). The role of IL-1β and glutamate in the effects of lipopolysaccharide on the hippocampal electrical kindling of seizures. J. Neuroimmunol. 298, 146–152. doi:10.1016/j.jneuroim.2016.07.018
Komiyama, K., Iijima, K., Kawabata-Iwakawa, R., Fujihara, K., Kakizaki, T., Yanagawa, Y., et al. (2022). Glioma facilitates the epileptic and tumor-suppressive gene expressions in the surrounding region. Sci. Rep. 12, 6805–6812. doi:10.1038/s41598-022-10753-4
Kong, Q., Takahashi, K., Schulte, D., Stouffer, N., Lin, Y., and Lin, C.-L. G. (2012). Increased glial glutamate transporter EAAT2 expression reduces epileptogenic processes following pilocarpine-induced status epilepticus. Neurobiol. Dis. 47, 145–154. doi:10.1016/j.nbd.2012.03.032
Konopka, A., Zeug, A., Skupien, A., Kaza, B., Mueller, F., Chwedorowicz, A., et al. (2016). Cleavage of hyaluronan and CD44 adhesion molecule regulate astrocyte morphology via Rac1 signalling. PLoS ONE 11, e0155053. doi:10.1371/journal.pone.0155053
Kwok, J. C. F., Carulli, D., and Fawcett, J. W. (2010). In vitro modeling of perineuronal nets: Hyaluronan synthase and link protein are necessary for their formation and integrity. J. Neurochem. 114, 1447–1459. doi:10.1111/j.1471-4159.2010.06878.x
Lachos, J., Zattoni, M., Wieser, H.-G., Fritschy, J.-M., Langmann, T., Schmitz, G., et al. (2011). Characterization of the gene expression profile of human Hippocampus in mesial temporal lobe epilepsy with hippocampal sclerosis. Epilepsy Res. Treat. 2011, 758407. doi:10.1155/2011/758407
Laywell, E. D., Dörries, U., Bartsch, U., Faissner, A., Schachner, M., and Steindler, D. A. (1992). Enhanced expression of the developmentally regulated extracellular matrix molecule tenascin following adult brain injury. Proc. Natl. Acad. Sci. U. S. A. 89, 2634–2638. doi:10.1073/pnas.89.7.2634
Lee, S. H., Magge, S., Spencer, D. D., Sontheimer, H., and Cornell-Bell, A. H. (1995). Human epileptic astrocytes exhibit increased gap junction coupling. Glia 15, 195–202. doi:10.1002/glia.440150212
Lensjø, K. K., Christensen, A. C., Tennøe, S., Fyhn, M., and Hafting, T., 2017. Differential expression and cell-type specificity of perineuronal nets in Hippocampus, medial entorhinal cortex, and visual cortex examined in the rat and mouse. eneuro 4, 0379. doi:10.1523/ENEURO.0379-16.2017
Levesque, M. C., and Haynes, B. F. (1997). Cytokine induction of the ability of human monocyte CD44 to bind hyaluronan is mediated primarily by TNF-alpha and is inhibited by IL-4 and IL-13. J. Immunol. Balt. 159, 6184–6194. doi:10.4049/jimmunol.159.12.6184
Levy, N., Milikovsky, D. Z., Baranauskas, G., Vinogradov, E., David, Y., Ketzef, M., et al. (2015). Differential TGF-β signaling in glial subsets underlies IL-6-mediated epileptogenesis in mice. J. Immunol. Balt. 195, 1713–1722. doi:10.4049/jimmunol.1401446
Li, J. H., Huang, X. R., Zhu, H.-J., Johnson, R., and Lan, H. Y. (2003). Role of TGF-β signaling in extracellular matrix production under high glucose conditions. Kidney Int. 63, 2010–2019. doi:10.1046/j.1523-1755.2003.00016.x
Lipachev, N., Arnst, N., Melnikova, A., Jäälinoja, H., Kochneva, A., Zhigalov, A., et al. (2019). Quantitative changes in perineuronal nets in development and posttraumatic condition. J. Mol. Histol. 50, 203–216. doi:10.1007/s10735-019-09818-y
Liu, Y.-J., Spangenberg, E. E., Tang, B., Holmes, T. C., Green, K. N., and Xu, X. (2021). Microglia elimination increases neural circuit connectivity and activity in adult mouse cortex. J. Neurosci. 41, 1274–1287. doi:10.1523/JNEUROSCI.2140-20.2020
Liu, Y. U., Ying, Y., Li, Y., Eyo, U. B., Chen, T., Zheng, J., et al. (2019). Neuronal network activity controls microglial process surveillance in awake mice via norepinephrine signaling. Nat. Neurosci. 22, 1771–1781. doi:10.1038/s41593-019-0511-3
Logsdon, A. F., Francis, K. L., Richardson, N. E., Hu, S. J., Faber, C. L., Setthavongsack, N., et al. (2021). Decoding perineuronal net glycan sulfation patterns in the Alzheimer’s disease brain. ALZHEIMERS Dement. 18, 942–954. doi:10.1002/alz.12451
Lukasiuk, K., Wilczynski, G. M., and Kaczmarek, L. (2011). Extracellular proteases in epilepsy. Epilepsy Res. 96, 191–206. doi:10.1016/j.eplepsyres.2011.08.002
MacKenzie, G., O’Toole, K. K., Moss, S. J., and Maguire, J. (2016). Compromised GABAergic inhibition contributes to tumor-associated epilepsy. Epilepsy Res. 126, 185–196. doi:10.1016/j.eplepsyres.2016.07.010
Mahmud, K. A., Hasan, F., Khan, M. I., and Adnan, A. (2022). Shock-induced damage mechanism of perineuronal nets. Biomolecules 12, 10. doi:10.3390/biom12010010
Manley, N. C., Bertrand, A. A., Kinney, K. S., Hing, T. C., and Sapolsky, R. M. (2007). Characterization of monocyte chemoattractant protein-1 expression following a kainate model of status epilepticus. Brain Res. 1182, 138–143. doi:10.1016/j.brainres.2007.08.092
Marchand, A., and Schwartz, C. (2020). Perineuronal net expression in the brain of a hibernating mammal. Brain Struct. Funct. 225, 45–56. doi:10.1007/s00429-019-01983-w
Markovic, D., Glass, R., Synowitz, M., Rooijen, N., and Kettenmann, H. (2005). Microglia stimulate the invasiveness of glioma cells by increasing the activity of metalloprotease-2. J. Neuropathol. Exp. Neurol. 64, 754–762. doi:10.1097/01.jnen.0000178445.33972.a9
Maroso, M., Balosso, S., Ravizza, T., Liu, J., Aronica, E., Iyer, A. M., et al. (2010). Toll-like receptor 4 and high-mobility group box-1 are involved in ictogenesis and can be targeted to reduce seizures. Nat. Med. 16, 413–419. doi:10.1038/nm.2127
Maroso, M., Balosso, S., Ravizza, T., Liu, J., Bianchi, M. E., and Vezzani, A. (2011). Interleukin-1 type 1 receptor/Toll-like receptor signalling in epilepsy: The importance of IL-1beta and high-mobility group box 1. J. Intern. Med. 270, 319–326. doi:10.1111/j.1365-2796.2011.02431.x
Maroto, M., Fernández-Morales, J.-C., Padín, J. F., González, J. C., Hernández-Guijo, J. M., Montell, E., et al. (2013). Chondroitin sulfate, a major component of the perineuronal net, elicits inward currents, cell depolarization, and calcium transients by acting on AMPA and kainate receptors of hippocampal neurons. J. Neurochem. 125, 205–213. doi:10.1111/jnc.12159
Massagué, J., and Chen, Y.-G. (2000). Controlling TGF-β signaling. Genes Dev. 14, 627–644. doi:10.1101/gad.14.6.627
Massey, J. M., Hubscher, C. H., Wagoner, M. R., Decker, J. A., Amps, J., Silver, J., et al. (2006). Chondroitinase ABC digestion of the perineuronal net promotes functional collateral sprouting in the cuneate nucleus after cervical spinal cord injury. J. Neurosci. 26, 4406–4414. doi:10.1523/JNEUROSCI.5467-05.2006
Mataga, N., Mizuguchi, Y., and Hensch, T. K. (2004). Experience-dependent pruning of dendritic spines in visual cortex by tissue plasminogen activator. Neuron 44, 1031–1041. doi:10.1016/j.neuron.2004.11.028
Mátyás, A., Borbély, E., and Mihály, A. (2021). Hippocampal sclerosis in pilocarpine epilepsy: Survival of peptide-containing neurons and learning and memory disturbances in the adult NMRI strain mouse. Int. J. Mol. Sci. 23, 204. doi:10.3390/ijms23010204
McRae, P. A., Baranov, E., Rogers, S. L., and Porter, B. E. (2012). Persistent decrease in multiple components of the perineuronal net following status epilepticus. Eur. J. Neurosci. 36, 3471–3482. doi:10.1111/j.1460-9568.2012.08268.x
McRae, P. A., Baranov, E., Sarode, S., Brooks-Kayal, A. R., and Porter, B. E. (2010). Aggrecan expression, a component of the inhibitory interneuron perineuronal net, is altered following an early-life seizure. Neurobiol. Dis. 39, 439–448. doi:10.1016/j.nbd.2010.05.015
McRae, P. A., Rocco, M. M., Kelly, G., Brumberg, J. C., and Matthews, R. T. (2007). Sensory deprivation alters aggrecan and perineuronal net expression in the mouse barrel cortex. J. Neurosci. Off. J. Soc. Neurosci. 27, 5405–5413. doi:10.1523/JNEUROSCI.5425-06.2007
Mehra, A., Ali, C., Parcq, J., Vivien, D., and Docagne, F. (2016). The plasminogen activation system in neuroinflammation. BBA - Mol. Basis Dis. 1862, 395–402. doi:10.1016/j.bbadis.2015.10.011
Mercado-Gómez, O., Landgrave-Gómez, J., Arriaga-Avila, V., Nebreda-Corona, A., and Guevara-Guzmán, R. (2014). Role of TGF-β signaling pathway on Tenascin C protein upregulation in a pilocarpine seizure model. Epilepsy Res. 108, 1694–1704. doi:10.1016/j.eplepsyres.2014.09.019
Midwood, K., Sacre, S., Piccinini, A. M., Inglis, J., Trebaul, A., Chan, E., et al. (2009). Tenascin-C is an endogenous activator of Toll-like receptor 4 that is essential for maintaining inflammation in arthritic joint disease. Nat. Med. 15, 774–780. doi:10.1038/nm.1987
Miyata, S., and Kitagawa, H. (2015). Mechanisms for modulation of neural plasticity and axon regeneration by chondroitin sulphate. J. Biochem. (Tokyo) 157, 13–22. doi:10.1093/jb/mvu067
Miyata, S., and Kitagawa, H. (2017). Formation and remodeling of the brain extracellular matrix in neural plasticity: Roles of chondroitin sulfate and hyaluronan. Biochim. Biophys. Acta Gen. Subj. 1861, 2420–2434. doi:10.1016/j.bbagen.2017.06.010
Miyata, S., Nadanaka, S., Igarashi, M., and Kitagawa, H. (2018). Structural variation of chondroitin sulfate chains contributes to the molecular heterogeneity of perineuronal nets. Front. Integr. Neurosci. 12, 3. doi:10.3389/fnint.2018.00003
Mohamedi, Y., Fontanil, T., Cobo, T., Cal, S., and Obaya, A. J. (2020). New insights into ADAMTS metalloproteases in the central nervous system. Biomol 10, 403. doi:10.3390/biom10030403
Monneau, Y., Arenzana-Seisdedos, F., and Lortat-Jacob, H. (2016). The sweet spot: How GAGs help chemokines guide migrating cells. J. Leukoc. Biol. 99, 935–953. doi:10.1189/jlb.3MR0915-440R
Morawski, M., Dityatev, A., Hartlage-Rübsamen, M., Blosa, M., Holzer, M., Flach, K., et al. (2014). Tenascin-R promotes assembly of the extracellular matrix of perineuronal nets via clustering of aggrecan. Philos. Trans. R. Soc. Lond. B. Biol. Sci. 369, 20140046. doi:10.1098/rstb.2014.0046
Morawski, M., Reinert, T., Meyer-Klaucke, W., Wagner, F. E., Tröger, W., Reinert, A., et al. (2015). Ion exchanger in the brain: Quantitative analysis of perineuronally fixed anionic binding sites suggests diffusion barriers with ion sorting properties. Sci. Rep. 5, 16471. doi:10.1038/srep16471
Morawski, M., Brückner, G., Arendt, T., and Matthews, R. T. (2012). Aggrecan: Beyond cartilage and into the brain. Int. J. Biochem. Cell Biol. 44, 690–693. doi:10.1016/j.biocel.2012.01.010
Morawski, M., Brückner, M. K., Riederer, P., Brückner, G., and Arendt, T. (2004). Perineuronal nets potentially protect against oxidative stress. Exp. Neurol. 188, 309–315. doi:10.1016/j.expneurol.2004.04.017
Mueller-Buehl, C., Reinhard, J., Roll, L., Bader, V., Winklhofer, K. F., and Faissner, A. (2022). Brevican, neurocan, tenascin-C, and tenascin-R act as important regulators of the interplay between perineuronal nets, synaptic integrity, inhibitory interneurons, and Otx2. Front. Cell Dev. Biol. 10, 886527. doi:10.3389/fcell.2022.886527
Murase, S., Lantz, C. L., and Quinlan, E. M. (2017). Light reintroduction after dark exposure reactivates plasticity in adults via perisynaptic activation of MMP-9. eLife 6, e27345. doi:10.7554/eLife.27345
Murthy, S., Kane, G. A., Katchur, N. J., Lara Mejia, P. S., Obiofuma, G., Buschman, T. J., et al. (2019). Perineuronal nets, inhibitory interneurons, and anxiety-related ventral hippocampal neuronal oscillations are altered by early life adversity. Biol. Psychiatry 85, 1011–1020. doi:10.1016/j.biopsych.2019.02.021
Naffah-Mazzacoratti, M. G., PorcionattoArgañarazM, G. A. A., Scorza, F. A., Amado, D., Silva, R., Bellissimo, M. I., et al. (1999). Selective alterations of glycosaminoglycans synthesis and proteoglycan expression in rat cortex and hippocampus in pilocarpine-induced epilepsy. Brain Res. Bull. 50, 229–239. doi:10.1016/S0361-9230(99)00195-1
Nakada, M., Miyamori, H., Kita, D., Takahashi, T., Yamashita, J., Sato, H., et al. (2005). Human glioblastomas overexpress ADAMTS-5 that degrades brevican. Acta Neuropathol. (Berl.) 110, 239–246. doi:10.1007/s00401-005-1032-6
Neal, M. L., Boyle, A. M., Budge, K. M., Safadi, F. F., and Richardson, J. R. (2018). The glycoprotein GPNMB attenuates astrocyte inflammatory responses through the CD44 receptor. J. Neuroinflammation 15, 73–81. doi:10.1186/s12974-018-1100-1
Neal, M., and Richardson, J. R. (2018). Epigenetic regulation of astrocyte function in neuroinflammation and neurodegeneration. Biochim. Biophys. Acta BBA - Mol. Basis Dis. 1864, 432–443. doi:10.1016/j.bbadis.2017.11.004
Nguyen, P. T., Dorman, L. C., Pan, S., Vainchtein, I. D., Han, R. T., Nakao-Inoue, H., et al. (2020). Microglial remodeling of the extracellular matrix promotes synapse plasticity. Cell 182, 388–403. doi:10.1016/j.cell.2020.05.050
Noble, P. W. (2002). Hyaluronan and its catabolic products in tissue injury and repair. Matrix Biol. J. Int. Soc. Matrix Biol. 21, 25–29. doi:10.1016/s0945-053x(01)00184-6
Nwaobi, S. E., Cuddapah, V. A., Patterson, K. C., Randolph, A. C., and Olsen, M. L. (2016). The role of glial-specific Kir4.1 in normal and pathological states of the CNS. Acta Neuropathol. (Berl.) 132, 1–21. doi:10.1007/s00401-016-1553-1
Ohno, Y. (2018). Astrocytic Kir4.1 potassium channels as a novel therapeutic target for epilepsy and mood disorders. Neural Regen. Res. 13, 651–652. doi:10.4103/1673-5374.230355
Okamoto, M., Sakiyama, J., Mori, S., Kurazono, S., Usui, S., Hasegawa, M., et al. (2003). Kainic acid-induced convulsions cause prolonged changes in the chondroitin sulfate proteoglycans neurocan and phosphacan in the limbic structures. Exp. Neurol. 184, 179–195. doi:10.1016/S0014-4886(03)00251-6
Olsen, M. L., and Sontheimer, H. (2008). Functional implications for Kir4.1 channels in glial biology: From K+ buffering to cell differentiation. J. Neurochem. 107, 589–601. doi:10.1111/j.1471-4159.2008.05615.x
Pajarillo, E., Rizor, A., Lee, J., Aschner, M., and Lee, E. (2019). The role of astrocytic glutamate transporters GLT-1 and GLAST in neurological disorders: Potential targets for neurotherapeutics. Neuropharmacol. Neurotransmitter Transp. 161, 107559. doi:10.1016/j.neuropharm.2019.03.002
Pantazopoulos, H., Gisabella, B., Rexrode, L., Benefield, D., Yildiz, E., Seltzer, P., et al. (2020). Circadian rhythms of perineuronal net composition. eNeuro 7, ENEURO.0034. doi:10.1523/ENEURO.0034-19.2020
Pantazopoulos, H., Woo, T.-U. W., Lim, M. P., Lange, N., and Berretta, S. (2010). Extracellular matrix-glial abnormalities in the amygdala and entorhinal cortex of subjects diagnosed with schizophrenia. Arch. Gen. Psychiatry 67, 155–166. doi:10.1001/archgenpsychiatry.2009.196
Perkins, K. L., Arranz, A. M., Yamaguchi, Y., and Hrabetova, S. (2017). Brain extracellular space, hyaluronan, and the prevention of epileptic seizures. Rev. Neurosci. 28, 869–892. doi:10.1515/revneuro-2017-0017
Perosa, S. R., Porcionatto, M. A., Cukiert, A., Martins, J. R. M., Passeroti, C. C., Amado, D., et al. (2002). Glycosaminoglycan levels and proteoglycan expression are altered in the hippocampus of patients with mesial temporal lobe epilepsy. Brain Res. Bull. 58, 509–516. doi:10.1016/S0361-9230(02)00822-5
Pielecka-Fortuna, J., Kalogeraki, E., Fortuna, M. G., and Löwel, S. (2015). Optimal level activity of matrix metalloproteinases is critical for adult visual plasticity in the healthy and stroke-affected brain. eLife 5, e11290. doi:10.7554/eLife.11290
Pijet, B., Stefaniuk, M., Kostrzewska-Ksiezyk, A., Tsilibary, P.-E., Tzinia, A., and Kaczmarek, L. (2018). Elevation of MMP-9 levels promotes epileptogenesis after traumatic brain injury. Mol. Neurobiol. 55, 9294–9306. doi:10.1007/s12035-018-1061-5
Pizzorusso, T., Medini, P., Berardi, N., Chierzi, S., Fawcett, J. W., and Maffei, L. (2002). Reactivation of ocular dominance plasticity in the adult visual cortex. Science 298, 1248–1251. doi:10.1126/science.1072699
Pollock, E., Everest, M., Brown, A., and Poulter, M. O. (2014). Metalloproteinase inhibition prevents inhibitory synapse reorganization and seizure Genesis. Neurobiol. Dis. 70, 21–31. doi:10.1016/j.nbd.2014.06.003
Puré, E., and Cuff, C. A. (2001). A crucial role for CD44 in inflammation. Trends Mol. Med. 7, 213–221. doi:10.1016/s1471-4914(01)01963-3
Pyka, M., Wetzel, C., Aguado, A., Geissler, M., Hatt, H., and Faissner, A. (2011). Chondroitin sulfate proteoglycans regulate astrocyte-dependent synaptogenesis and modulate synaptic activity in primary embryonic hippocampal neurons. Eur. J. Neurosci. 33, 2187–2202. doi:10.1111/j.1460-9568.2011.07690.x
Ranaivo, H. R., Hodge, J. N., Choi, N., and Wainwright, M. S. (2012). Albumin induces upregulation of matrix metalloproteinase-9 in astrocytes via MAPK and reactive oxygen species-dependent pathways. J. Neuroinflammation 9, 68. doi:10.1186/1742-2094-9-68
Rankin-Gee, E. K., McRae, P. A., Baranov, E., Rogers, S., Wandrey, L., and Porter, B. E. (2015). Perineuronal net degradation in epilepsy. Epilepsia 56, 1124–1133. doi:10.1111/epi.13026
Raposo, C., and Schwartz, M. (2014). Glial scar and immune cell involvement in tissue remodeling and repair following acute CNS injuries. Glia 62, 1895–1904. doi:10.1002/glia.22676
Ravizza, T., Gagliardi, B., Noé, F., Boer, K., Aronica, E., and Vezzani, A. (2008). Innate and adaptive immunity during epileptogenesis and spontaneous seizures: Evidence from experimental models and human temporal lobe epilepsy. Neurobiol. Dis. 29, 142–160. doi:10.1016/j.nbd.2007.08.012
Reichelt, A. C., Hare, D. J., Bussey, T. J., and Saksida, L. M. (2019). Perineuronal nets: Plasticity, protection, and therapeutic potential. Trends Neurosci. 42, 458–470. doi:10.1016/j.tins.2019.04.003
Reinhard, S. M., Razak, K., and Ethell, I. M. (2015). A delicate balance: Role of MMP-9 in brain development and pathophysiology of neurodevelopmental disorders. Front. Cell. Neurosci. 9, 280. doi:10.3389/fncel.2015.00280
Robel, S., Buckingham, S. C., Boni, J. L., Campbell, S. L., Danbolt, N. C., Riedemann, T., et al. (2015). Reactive astrogliosis causes the development of spontaneous seizures. J. Neurosci. 35, 3330–3345. doi:10.1523/JNEUROSCI.1574-14.2015
Robel, S., and Sontheimer, H. (2016). Glia as drivers of abnormal neuronal activity. Nat. Neurosci. 19, 28–33. doi:10.1038/nn.4184
Robert, S. M., Buckingham, S. C., Campbell, S. L., Robel, S., Holt, K. T., Ogunrinu-Babarinde, T., et al. (2015). SLC7A11 expression is associated with seizures and predicts poor survival in patients with malignant glioma. Sci. Transl. Med. 7, 289ra86. doi:10.1126/scitranslmed.aaa8103
Rogers, S. L., Rankin-Gee, E., Risbud, R. M., Porter, B. E., and Marsh, E. D. (2018). Normal development of the perineuronal net in humans; in patients with and without epilepsy. Neuroscience 384, 350–360. doi:10.1016/j.neuroscience.2018.05.039
Romberg, C., Yang, S., Melani, R., Andrews, M. R., Horner, A. E., Spillantini, M. G., et al. (2013). Depletion of perineuronal nets enhances recognition memory and long-term depression in the perirhinal cortex. J. Neurosci. Off. J. Soc. Neurosci. 33, 7057–7065. doi:10.1523/JNEUROSCI.6267-11.2013
Rosenberg, G. A. (2009). Matrix metalloproteinases and their multiple roles in neurodegenerative diseases. Lancet Neurol. 8, 205–216. doi:10.1016/S1474-4422(09)70016-X
Rosenberg, G. A. (2002). Matrix metalloproteinases in neuroinflammation. Glia 39, 279–291. doi:10.1002/glia.10108
Rossi, D., Brambilla, L., Valori, C. F., Roncoroni, C., Crugnola, A., Yokota, T., et al. (2008). Focal degeneration of astrocytes in amyotrophic lateral sclerosis. Cell Death Differ. 15, 1691–1700. doi:10.1038/cdd.2008.99
Roszkowska, M., Skupien, A., Wójtowicz, T., Konopka, A., Gorlewicz, A., Kisiel, M., et al. (2016). CD44: A novel synaptic cell adhesion molecule regulating structural and functional plasticity of dendritic spines. Mol. Biol. Cell 27, 4055–4066. doi:10.1091/mbc.E16-06-0423
Rowlands, D., Lensjø, K. K., Dinh, T., Yang, S., Andrews, M. R., Hafting, T., et al. (2018). Aggrecan directs extracellular matrix-mediated neuronal plasticity. J. Neurosci. 38, 10102–10113. doi:10.1523/JNEUROSCI.1122-18.2018
Schauwecker, P. E. (2012). Strain differences in seizure-induced cell death following pilocarpine-induced status epilepticus. Neurobiol. Dis. 45, 297–304. doi:10.1016/j.nbd.2011.08.013
Schiller, M., Javelaud, D., and Mauviel, A. (2004). TGF-beta-induced SMAD signaling and gene regulation: Consequences for extracellular matrix remodeling and wound healing. J. Dermatol. Sci. 35, 83–92. doi:10.1016/j.jdermsci.2003.12.006
Schmidt, S., Arendt, T., Morawski, M., and Sonntag, M. (2020). Neurocan contributes to perineuronal net development. Neuroscience 442, 69–86. doi:10.1016/j.neuroscience.2020.06.040
Semple, B. D., O’Brien, T. J., Gimlin, K., Wright, D. K., Kim, S. E., Casillas-Espinosa, P. M., et al. (2017). Interleukin-1 receptor in seizure susceptibility after traumatic injury to the pediatric brain. J. Neurosci. Off. J. Soc. Neurosci. 37, 7864–7877. doi:10.1523/JNEUROSCI.0982-17.2017
Shapiro, L. A., Wang, L., and Ribak, C. E. (2008). Rapid astrocyte and microglial activation following pilocarpine-induced seizures in rats. Epilepsia 49, 33–41. doi:10.1111/j.1528-1167.2008.01491.x
Shapiro, S. D. (1998). Matrix metalloproteinase degradation of extracellular matrix: Biological consequences. Curr. Opin. Cell Biol. 10, 602–608. doi:10.1016/S0955-0674(98)80035-5
Sharma, K., Selzer, M. E., and Li, S. (2012). Scar-mediated inhibition and CSPG receptors in the CNS. Exp. Neurol. 237, 370–378. doi:10.1016/j.expneurol.2012.07.009
Sharma, R., Leung, W. L., Zamani, A., O’Brien, T. J., Casillas Espinosa, P. M., and Semple, B. D. (2019). Neuroinflammation in post-traumatic epilepsy: Pathophysiology and tractable therapeutic targets. Brain Sci. 9, 318. doi:10.3390/brainsci9110318
Silver, J., and Miller, J. H. (2004). Regeneration beyond the glial scar. Nat. Rev. Neurosci. 5, 146–156. doi:10.1038/nrn1326
Sinha, S., Patil, S. A., Jayalekshmy, V., and Satishchandra, P. (2008). Do cytokines have any role in epilepsy? Epilepsy Res. 82, 171–176. doi:10.1016/j.eplepsyres.2008.07.018
Sitaš, B., Bobić-Rasonja, M., Mrak, G., Trnski, S., Krbot Skorić, M., Orešković, D., et al. (2022). Reorganization of the brain extracellular matrix in hippocampal sclerosis. Int. J. Mol. Sci. 23, 8197. doi:10.3390/ijms23158197
Slaker, M. L., Harkness, J. H., and Sorg, B. A. (2016). A standardized and automated method of perineuronal net analysis using Wisteria floribunda agglutinin staining intensity. IBRO Rep. 1, 54–60. doi:10.1016/j.ibror.2016.10.001
Sofroniew, M. V., and Vinters, H. V. (2010). Astrocytes: Biology and pathology. Acta Neuropathol. (Berl.) 119, 7–35. doi:10.1007/s00401-009-0619-8
Soltani Khaboushan, A., Yazdanpanah, N., and Rezaei, N. (2022). Neuroinflammation and proinflammatory cytokines in epileptogenesis. Mol. Neurobiol. 59, 1724–1743. doi:10.1007/s12035-022-02725-6
Song, I., and Dityatev, A. (2018). Crosstalk between glia, extracellular matrix and neurons. Brain Res. Bull., Mol. Mech. astrocyte-neuron Signal. 136, 101–108. doi:10.1016/j.brainresbull.2017.03.003
Sonntag, M., Blosa, M., Schmidt, S., Reimann, K., Blum, K., Eckrich, T., et al. (2018). Synaptic coupling of inner ear sensory cells is controlled by brevican-based extracellular matrix baskets resembling perineuronal nets. BMC Biol. 16, 99. doi:10.1186/s12915-018-0566-8
Srivastava, A., Dixit, A. B., Paul, D., Tripathi, M., Sarkar, C., Chandra, P. S., et al. (2017). Comparative analysis of cytokine/chemokine regulatory networks in patients with hippocampal sclerosis (HS) and focal cortical dysplasia (FCD). Sci. Rep. 7, 15904. doi:10.1038/s41598-017-16041-w
Stewart, A. G., Thomas, B., and Koff, J. (2018). TGF-β: Master regulator of inflammation and fibrosis. Respirology 23, 1096–1097. doi:10.1111/resp.13415
Sun, D., van Klink, N. E. C., Bongaarts, A., Zweiphenning, W. E. J. M., van ’t Klooster, M. A., Gebbink, T. A., et al. (2022). High frequency oscillations associate with neuroinflammation in low-grade epilepsy associated tumors. Clin. Neurophysiol. 133, 165–174. doi:10.1016/j.clinph.2021.08.025
Syková, E. (2004). Extrasynaptic volume transmission and diffusion parameters of the extracellular space. Neuroscience 129, 861–876. doi:10.1016/j.neuroscience.2004.06.077
Szklarczyk, A., Lapinska, J., Rylski, M., McKay, R. D. G., and Kaczmarek, L. (2002). Matrix metalloproteinase-9 undergoes expression and activation during dendritic remodeling in adult hippocampus. J. Neurosci. Off. J. Soc. Neurosci. 22, 920–930. doi:10.1523/JNEUROSCI.22-03-00920.2002
Takahashi, D. K., Vargas, J. R., and Wilcox, K. S. (2010). Increased coupling and altered glutamate transport currents in astrocytes following kainic-acid-induced status epilepticus. Neurobiol. Dis. 40, 573–585. doi:10.1016/j.nbd.2010.07.018
Tan, G.-K., and Tabata, Y. (2014). Chondroitin-6-sulfate attenuates inflammatory responses in murine macrophages via suppression of NF-κB nuclear translocation. Acta Biomater. 10, 2684–2692. doi:10.1016/j.actbio.2014.02.025
Tan, T. H.-L., Perucca, P., O’Brien, T. J., Kwan, P., and Monif, M. (2021). Inflammation, ictogenesis, and epileptogenesis: An exploration through human disease. Epilepsia 62, 303–324. doi:10.1111/epi.16788
Tewari, B. P., Chaunsali, L., Campbell, S. L., Patel, D. C., Goode, A. E., and Sontheimer, H. (2018). Perineuronal nets decrease membrane capacitance of peritumoral fast spiking interneurons in a model of epilepsy. Nat. Commun. 9, 4724. doi:10.1038/s41467-018-07113-0
Thom, M. (2014). Review: Hippocampal sclerosis in epilepsy: A neuropathology review. Neuropathol. Appl. Neurobiol. 40, 520–543. doi:10.1111/nan.12150
Thompson, E. H., Lensjø, K. K., Wigestrand, M. B., Malthe-Sørenssen, A., Hafting, T., and Fyhn, M. (2018). Removal of perineuronal nets disrupts recall of a remote fear memory. Proc. Natl. Acad. Sci. U. S. A. 115, 607–612. doi:10.1073/pnas.1713530115
Tian, D.-S., Peng, J., Murugan, M., Feng, L.-J., Liu, J.-L., Eyo, U. B., et al. (2017). Chemokine CCL2-CCR2 signaling induces neuronal cell death via STAT3 activation and IL-1β production after status epilepticus. J. Neurosci. Off. J. Soc. Neurosci. 37, 7878–7892. doi:10.1523/JNEUROSCI.0315-17.2017
Tong, X., Ao, Y., Faas, G. C., Nwaobi, S. E., Xu, J., Haustein, M. D., et al. (2014). Astrocyte Kir4. 1 ion channel deficits contribute to neuronal dysfunction in Huntington’s disease model mice. Nat. Neurosci. 17, 694–703. doi:10.1038/nn.3691
Torres-Ceja, B., and Olsen, M. L. (2022). A closer look at astrocyte morphology: Development, heterogeneity, and plasticity at astrocyte leaflets. Curr. Opin. Neurobiol. 74, 102550. doi:10.1016/j.conb.2022.102550
Tröscher, A. R., Gruber, J., Wagner, J. N., Böhm, V., Wahl, A.-S., and von Oertzen, T. J. (2021). Inflammation mediated epileptogenesis as possible mechanism underlying ischemic post-stroke epilepsy. Front. Aging Neurosci. 13, 781174. doi:10.3389/fnagi.2021.781174
Tsien, R. Y. (2013). Very long-term memories may be stored in the pattern of holes in the perineuronal net. Proc. Natl. Acad. Sci. U. S. A. 110, 12456–12461. doi:10.1073/pnas.1310158110
Ueno, H., Suemitsu, S., Murakami, S., Kitamura, N., Wani, K., Takahashi, Y., et al. (2019). Alteration of extracellular matrix molecules and perineuronal nets in the Hippocampus of pentylenetetrazol-kindled mice. Neural Plast. 2019, 8924634. doi:10.1155/2019/8924634
Ueno, H., Suemitsu, S., Murakami, S., Kitamura, N., Wani, K., Takahashi, Y., et al. (2020). Pentylenetetrazol kindling induces cortical astrocytosis and increased expression of extracellular matrix molecules in mice. Brain Res. Bull. 163, 120–134. doi:10.1016/j.brainresbull.2020.07.019
Ulbrich, P., Khoshneviszadeh, M., Jandke, S., Schreiber, S., and Dityatev, A. (2020). Interplay between perivascular and perineuronal extracellular matrix remodelling in neurological and psychiatric diseases. Eur. J. Neurosci. 53, 3811–3830. doi:10.1111/ejn.14887
Vainchtein, I., Chin, G., Cho, F. S., Kelley, K. W., Miller, J. G., Chien, E. C., et al. (2018). Astrocyte-derived interleukin-33 promotes microglial synapse engulfment and neural circuit development. Science 359, 1269–1273. doi:10.1126/science.aal3589
Varol, C., and Sagi, I. (2018). Phagocyte—Extracellular matrix crosstalk empowers tumor development and dissemination. FEBS J. 285, 734–751. doi:10.1111/febs.14317
Vezzani, A., Aronica, E., Mazarati, A., and Pittman, Q. J. (2013). Epilepsy and brain inflammation. Spec. Issue Epilepsy 244, 11–21. doi:10.1016/j.expneurol.2011.09.033
Vezzani, A., Balosso, S., and Ravizza, T. (2008). The role of cytokines in the pathophysiology of epilepsy. Brain. Behav. Immun. 22, 797–803. doi:10.1016/j.bbi.2008.03.009
Vezzani, A., French, J., Bartfai, T., and Baram, T. Z. (2011). The role of inflammation in epilepsy. Nat. Rev. Neurol. 7, 31–40. doi:10.1038/nrneurol.2010.178
Vezzani, A., Moneta, D., Conti, M., Richichi, C., Ravizza, T., De Luigi, A., et al. (2000). Powerful anticonvulsant action of IL-1 receptor antagonist on intracerebral injection and astrocytic overexpression in mice. Proc. Natl. Acad. Sci. U. S. A. 97, 11534–11539. doi:10.1073/pnas.190206797
Vigier, S. (2016). “Exploring the extracellular matrix to create biomaterials,” in Composition and function of the extracellular matrix in the human body. Editor T. F. F. Travascio (Rijeka: IntechOpen). doi:10.5772/62979
Wang, C., Yue, H., Hu, Z., Shen, Y., Ma, J., Li, J., et al. (2020). Microglia mediate forgetting via complement-dependent synaptic elimination. Science 367, 688–694. doi:10.1126/science.aaz2288
Wang, M.-J., Kuo, J.-S., Lee, W.-W., Huang, H.-Y., Chen, W.-F., and Lin, S.-Z. (2006). Translational event mediates differential production of tumor necrosis factor-alpha in hyaluronan-stimulated microglia and macrophages. J. Neurochem. 97, 857–871. doi:10.1111/j.1471-4159.2006.03776.x
Webster, K. M., Sun, M., Crack, P., O’Brien, T. J., Shultz, S. R., and Semple, B. D. (2017). Inflammation in epileptogenesis after traumatic brain injury. J. Neuroinflammation 14, 10. doi:10.1186/s12974-016-0786-1
Wegrzyn, D., Kostka, M., Faissner, A., Juckel, G., Manitz, M.-P., and Freund, N. (2020). Poly I:C-induced maternal immune challenge reduces perineuronal net area and raises spontaneous network activity of hippocampal neurons in vitro. Eur. J. Neurosci. 53, 3920–3941. doi:10.1111/ejn.14934
Wei, S., Xiangbo, W., Wang, X., Du, S., Liu, W., Song, J., et al. (2019). Perineuronal nets protect long-term memory by limiting activity-dependent inhibition from parvalbumin interneurons. Proc. Natl. Acad. Sci. 116, 27063–27073. doi:10.1073/pnas.1902680116
Wen, T. H., Afroz, S., Reinhard, S. M., Palacios, A. R., Tapia, K., Binder, D. K., et al. (2018a). Genetic reduction of matrix metalloproteinase-9 promotes formation of perineuronal nets around parvalbumin-expressing interneurons and normalizes auditory cortex responses in developing Fmr1 knock-out mice. Cereb. Cortex N. Y. N. 28, 3951–3964. doi:10.1093/cercor/bhx258
Wen, T. H., Binder, D. K., Ethell, I. M., and Razak, K. A. (2018b). The perineuronal “safety” net? Perineuronal net abnormalities in neurological disorders. Front. Mol. Neurosci. 11, 270. doi:10.3389/fnmol.2018.00270
Wenzel, T. J., Bajwa, E., and Klegeris, A. (2019). Cytochrome c can be released into extracellular space and modulate functions of human astrocytes in a toll-like receptor 4-dependent manner. Biochim. Biophys. Acta Gen. Subj. 1863, 129400. doi:10.1016/j.bbagen.2019.07.009
Wetherington, J., Serrano, G., and Dingledine, R. (2008). Astrocytes in the epileptic brain. Neuron 58, 168–178. doi:10.1016/j.neuron.2008.04.002
Wiese, S., Karus, M., and Faissner, A. (2012). Astrocytes as a source for extracellular matrix molecules and cytokines. Front. Pharmacol. 3, 120. doi:10.3389/fphar.2012.00120
Wilcox, K. S., Gee, J. M., Gibbons, M. B., Tvrdik, P., and White, J. A. (2015). Altered structure and function of astrocytes following status epilepticus. Epilepsy Behav. SI:Status Epilepticus 49, 17–19. doi:10.1016/j.yebeh.2015.05.002
Wilczynski, G. M., Konopacki, F. A., Wilczek, E., Lasiecka, Z., Gorlewicz, A., Michaluk, P., et al. (2008). Important role of matrix metalloproteinase 9 in epileptogenesis. J. Cell Biol. 180, 1021–1035. doi:10.1083/jcb.200708213
Wilson, E. S., and Litwa, K. (2021). Synaptic hyaluronan synthesis and CD44-mediated signaling coordinate neural circuit development. Cells 10, 2574. doi:10.3390/cells10102574
Wingert, J. C., and Sorg, B. A. (2021). Impact of perineuronal nets on electrophysiology of parvalbumin interneurons, principal neurons, and brain oscillations: A review. Front. Synaptic Neurosci. 13, 673210. doi:10.3389/fnsyn.2021.673210
Wolinski, P., Ksiazek-Winiarek, D., and Glabinski, A. (2022). Cytokines and neurodegeneration in epileptogenesis. Brain Sci. 12, 380. doi:10.3390/brainsci12030380
Wu, Y., Wang, X., Mo, X., Xi, Z., Xiao, F., Li, J., et al. (2008). Expression of monocyte chemoattractant protein-1 in brain tissue of patients with intractable epilepsy. Clin. Neuropathol. 27, 55–63. doi:10.5414/npp27055
Wu, Z., Deshpande, T., Henning, L., Bedner, P., Seifert, G., and Steinhäuser, C. (2021). Cell death of hippocampal CA1 astrocytes during early epileptogenesis. Epilepsia 62, 1569–1583. doi:10.1111/epi.16910
Wyeth, M. S., Zhang, N., and Houser, C. R. (2012). Increased cholecystokinin labeling in the hippocampus of a mouse model of epilepsy maps to spines and glutamatergic terminals. Neuroscience 202, 371–383. doi:10.1016/j.neuroscience.2011.11.056
Xie, K., Liu, Y., Hao, W., Walter, S., Penke, B., Hartmann, T., et al. (2013). Tenascin-C deficiency ameliorates Alzheimer’s disease-related pathology in mice. Neurobiol. Aging 34, 2389–2398. doi:10.1016/j.neurobiolaging.2013.04.013
Yamada, J., and Jinno, S. (2013). Spatio-temporal differences in perineuronal net expression in the mouse hippocampus, with reference to parvalbumin. Neuroscience 253, 368–379. doi:10.1016/j.neuroscience.2013.08.061
Yang, S., Cacquevel, M., Saksida, L. M., Bussey, T. J., Schneider, B. L., Aebischer, P., et al. (2015). Perineuronal net digestion with chondroitinase restores memory in mice with tau pathology. Exp. Neurol. 265, 48–58. doi:10.1016/j.expneurol.2014.11.013
Yang, S., Gigout, S., Molinaro, A., Naito-Matsui, Y., Hilton, S., Foscarin, S., et al. (2021). Chondroitin 6-sulphate is required for neuroplasticity and memory in ageing. Mol. Psychiatry 26, 5658–5668. doi:10.1038/s41380-021-01208-9
Yasuoka, S., Kawanokuchi, J., Parajuli, B., Jin, S., Doi, Y., Noda, M., et al. (2011). Production and functions of IL-33 in the central nervous system. Brain Res. 1385, 8–17. doi:10.1016/j.brainres.2011.02.045
Ye, Z.-C., and Sontheimer, H. (1996). Cytokine modulation of glial glutamate uptake: A possible involvement of nitric oxide. NeuroReport 7, 2181–2185. doi:10.1097/00001756-199609020-00025
Yuan, W., Matthews, R. T., Sandy, J. D., and Gottschall, P. E. (2002). Association between protease-specific proteolytic cleavage of brevican and synaptic loss in the dentate gyrus of kainate-treated rats. Neuroscience 114, 1091–1101. doi:10.1016/S0306-4522(02)00347-0
Yutsudo, N., and Kitagawa, H. (2015). Involvement of chondroitin 6-sulfation in temporal lobe epilepsy. Integr. Neuronal Funct. 274, 126–133. doi:10.1016/j.expneurol.2015.07.009
Zaben, M., Haan, N., Sharouf, F., Ahmed, A., Sundstrom, L. E., and Gray, W. P. (2021). IL-1β and HMGB1 are anti-neurogenic to endogenous neural stem cells in the sclerotic epileptic human hippocampus. J. Neuroinflammation 18, 218. doi:10.1186/s12974-021-02265-1
Zamanian, J. L., Xu, L., Foo, L. C., Nouri, N., Zhou, L., Giffard, R. G., et al. (2012). Genomic analysis of reactive astrogliosis. J. Neurosci. 32, 6391–6410. doi:10.1523/JNEUROSCI.6221-11.2012
Zhang, S., Chen, F., Zhai, F., and Liang, S. (2022). Role of HMGB1/TLR4 and IL-1β/IL-1R1 signaling pathways in epilepsy. Front. Neurol. 13, 904225–904311. doi:10.3389/fneur.2022.904225
Zhang, Z., Li, Y., Jiang, S., Shi, F.-D., Shi, K., and Jin, W.-N. (2023). Targeting CCL5 signaling attenuates neuroinflammation after seizure. CNS Neurosci. Ther. 29, 317–330. doi:10.1111/cns.14006
Zhou, Y., Shao, A., Yao, Y., Tu, S., Deng, Y., and Zhang, J. (2020). Dual roles of astrocytes in plasticity and reconstruction after traumatic brain injury. Cell Commun. Signal. 18, 62. doi:10.1186/s12964-020-00549-2
Zurolo, E., de Groot, M., Iyer, A., Anink, J., van Vliet, E. A., Heimans, J. J., et al. (2012). Regulation of Kir4.1 expression in astrocytes and astrocytic tumors: A role for interleukin-1 β. J. Neuroinflammation 9, 280. doi:10.1186/1742-2094-9-280
Keywords: astrocytes, neuroinflammation, extracellular matrix, perineuronal nets (PNNs), epilepsy, epileptogenesis, neurodegenerative disease
Citation: Woo AM and Sontheimer H (2023) Interactions between astrocytes and extracellular matrix structures contribute to neuroinflammation-associated epilepsy pathology. Front. Mol. Med. 3:1198021. doi: 10.3389/fmmed.2023.1198021
Received: 31 March 2023; Accepted: 31 May 2023;
Published: 14 June 2023.
Edited by:
Rita Sattler, Barrow Neurological Institute (BNI), United StatesReviewed by:
Katherine Conant, Georgetown University Medical Center, United StatesYongjie Yang, Tufts University, United States
Copyright © 2023 Woo and Sontheimer. This is an open-access article distributed under the terms of the Creative Commons Attribution License (CC BY). The use, distribution or reproduction in other forums is permitted, provided the original author(s) and the copyright owner(s) are credited and that the original publication in this journal is cited, in accordance with accepted academic practice. No use, distribution or reproduction is permitted which does not comply with these terms.
*Correspondence: Harald Sontheimer, sontheimer@virginia.edu