- 1 ETT s.r.l., Genova, Italy
- 2 Systems Toxicology Unit, Institute for Health and Consumer Protection, Joint Research Centre, Ispra, Varese, Italy
- 3 Badische Anilin- und Soda-Fabrik Societas Europaea, Ludwigshafen, Germany
Detection and characterization of chemically induced toxic effects in the nervous system represent a challenge for the hazard assessment of chemicals. In vivo, neurotoxicological assessments exploit the fact that the activity of neurons in the central and peripheral nervous system has functional consequences. And so far, no in vitro method for evaluating the neurotoxic hazard has yet been validated and accepted for regulatory purpose. The micro-electrode array (MEA) assay consists of a culture chamber into which an integrated array of micro-electrodes is capable of measuring extracellular electrophysiology (spikes and bursts) from electro-active tissues. A wide variety of electrically excitable biological tissues may be placed onto the chips including primary cultures of nervous system tissue. Recordings from this type of in vitro cultured system are non-invasive, give label free evaluations and provide a higher throughput than conventional electrophysiological techniques. In this paper, 20 substances were tested in a blinded study for their toxicity and dose–response curves were obtained from fetal rat cortical neuronal networks coupled to MEAs. The experimental procedure consisted of evaluating the firing activity (spiking rate) and modification/reduction in response to chemical administration. Native/reference activity, 30 min of activity recording per dilution, plus the recovery points (after 24 h) were recorded. The preliminary data, using a set of chemicals with different mode-of-actions (13 known to be neurotoxic, 2 non-neuroactive and not toxic, and 5 non-neuroactive but toxic) show good predictivity (sensitivity: 0.77; specificity: 0.86; accuracy: 0.85). Thus, the MEA with a neuronal network has the potency to become an effective tool to evaluate the neurotoxicity of substances in vitro.
Introduction
The determination of the toxicity profile of different chemical, biological, and pharmacological compounds is outlined in the current international testing guidelines (OECD, 1997; US EPA, 1998). An important element of the hazard assessment is the evaluation of potential neurotoxic effects (Crofton et al., 2004; Coecke et al., 2006). An agent is considered neurotoxic if an alteration in the structure or function in any part of the central and/or peripheral nervous system can be observed following acute or chronic exposure, at concentrations that do not affect general viability (Costa, 1998). A neurotoxic effect can be the direct alteration of the neurons structure or activity or can be the result of cascade effects due to glia activation and glia-neuron interactions; a neurotoxic effect can manifest immediately or delayed after the substance administration, it can be permanent or reversible, and it can affect the whole nervous system as well as parts of it (Monnet-Tschudi et al., 1997; Philbert et al., 2000; Tabakman et al., 2004; Coecke et al., 2006).
Current directives for the evaluation of neurotoxic hazard (OECD, 1997; US EPA, 1998) are based on in vivo studies assessing neurophysiological, neuropathological, neurobehavioral, and neurochemical endpoints (Johnstone et al., 2010). These methods are expensive and time consuming, have a low throughput, and involve the use of a larger amount of test substances and animals.
The need efficient testing and recent directives on animal use for laboratory tests is pushing the development and validation of new testing strategies based on alternative methods (Hartung et al., 2003, 2004), in which the use of time, materials, and animals is reduced and refined or animal use is completely replaced (3R).
To date, no in vitro method has been validated for the neurotoxicology assessment, and one of the recent and most promising tools for neurotoxicity assessment is the measurement of electrical activity using micro-electrode array (MEA) chips. This technique is recording whole neuronal ensembles as functional networks and provides more relevant physiological information than other methods for electrophysiology assessment, e.g., patch clamps. The MEA-based recordings testing techniques dates back to the early eighties (Gross et al., 1982) and the technology behind has been improved since then (Gross et al., 1993; Breckenridge et al., 1995; Potter, 2001). Today many different in vitro models can be studied by MEA-based systems such as hippocampus slices, primary mammalian dissociated cultures and stem cells.
Mammalian neuronal networks cultured from different brain structures on MEA chips remain spontaneously active and stable for many months (Gross et al., 1982; Potter and DeMarse, 2001; Gramowski et al., 2004; Van Pelt et al., 2004a, b). Moreover, these models respond to neurotransmitters and their blockers in a similar way as the in vivo situation (Streit, 1993; Gramowski et al., 2000; Keefer et al., 2001a, b; Martinoia et al., 2005). Primary cultures grown on MEA chips have been used in many studies of pharmacological and toxicological responses, and acute neurotoxicity detection (Gross et al., 1997; Gramowski et al., 2000, 2006; Morefield et al., 2000; Keefer et al., 2001b; Pancrazio et al., 2003; Xia and Gross, 2003; Xia et al., 2003; Sundstrom et al., 2005; Parviz and Gross, 2007; van Vliet et al., 2007). A very recent review (Johnstone et al., 2010) describes the state of the art of MEA-based assays for neurotoxicity assessment.
In this study, electrical activity measurements were evaluated to see whether they are a reliable, accurate, and robust endpoint for the detection of neurotoxicity and could be suitable for predictive purposes in the chemical industry. To this end, 20 substances were selected, blinded at BASF (Germany) and then sent to ETT (Italy) to perform the test. The substances were selected according to their neurotoxic effects in vivo: 13 substances known to be neuroactive, 2 non-neuroactive and non-toxic and 5 non-neuroactive but toxic.
Materials and Methods
Chemicals
For the study purpose 20 substances were selected at BASF ( Germany) and sent to ETT (Italy) to perform the blind test. Some substances were selected according to their already known effect; some others are completely new for the MEA-based system literature. A set of 20 chemicals with different mode-of-actions (2 non-neuroactive and not toxic, Table 1; 5 not neuroactive but toxic, Table 2; 13 known to be neurotoxic, Table 3 substances) was prepared for the blind test. In particular the following set was selected and labeled as follows: (S1) Ibuprofen (CAS#:15687-27-1), (S2) 1,2,4-Trichlorobenzene (CAS#: 120-82-1), (S3) Trimethyltin chloride (CAS#: 1066-45-1), (S4) (2,4-Dichlorophenoxy) acetic acid sodium salt (CAS#: 2702-72-9), (S5) p-Cresol (CAS#: 106-44-5), (S6) Ethanol (CAS#: 64-17-5), (S7) Salicylic acid (CAS#: 69-72-7), (S8) Mepiquat chloride (CAS#: 24307-26-4), (S11) 1,2-Propandiol (CAS#: 57-55-6), (S13) Tetrahydroisoquinoline (THIQ; CAS#: 91-21-4), (S14) Toluene (CAS#: 108-88-3), (S15) Aniline (CAS#: 62-53-3), (S16) Nicotine (CAS#: 54-11-5), (S17) Fipronil (CAS#: 120068-37-3), (S19) Quinmerac (CAS#: 90717-03-6), (S20) Carbaryl (CAS#: 63-25-2), (S21) Nomifensine maleate salt (CAS#: 24526-64-5), (S22) Paraquat Dichloride (CAS#: 1910-42-5), (S23) Eugenol (CAS#: 97-53-0), (S24) Diphenhydramine hydrochloride (CAS#: 147-24-0). The 20 substances (12 powders and 8 liquids) were shipped in sealed amber glass vials labeled with numbers only (numbers from 1 to 24, 4 substances were omitted from the test). The set included 8 water soluble substances, and 12 water insoluble ones, which were diluted using DMSO. Each substance was dissolved to a 100-mM stock solution and then divided in aliquots stored at −20°C.
Cell Culture
Three different rat strains, namely Wistar SPF, Sprague-Dawley, and CD SPF/VAF F were employed to prove the reliability of results on the same district from different animal sources. The primary cultures of cortical neurons were prepared from fetal day 18 rats according to previously described procedures (Chiappalone et al., 2006). Briefly, the cortex was dissociated through enzymatic and mechanical dissociation (0.125% Trypsin – DNAse I 0.025 mg/ml – BSA 0.3% solution in HBSS without calcium and magnesium). The cells were seeded on standard 60-electrode TiN-SiN MEA chips with internal reference (Multi Channel Systems, Reutlingen, Germany) pre-coated with poly-D-lysine and laminin (0.1 mg/ml diluted in sterile MilliQ water) as 50 μl droplets (1500–2000 cells/mm2), with subsequent addition of 1 ml of medium after the cells were attached (approximately 2 h). Cultures were maintained in neurobasal (NB) medium supplemented with 2% B27 and 1% Glutamax-I, and half volume of the medium was exchanged once a week. Cells were maintained at 37°C in a humidified atmosphere of 5% CO2 until their use.
Experimental Layout
Experiments were carried from 25 to 54 days in vitro (DIV), when neuronal networks are mature (Novellino and Zaldívar, 2010). Each substance was tested at least three times and using neuronal networks from different neuronal isolations. Any neuronal network was used only once. In order to stabilize the culture condition, a 50% medium change was performed 48 h before testing. The day of the experiment the seven test dilutions (100 nM, 1 μM, 10 μM, 100 μM, 1 mM, 10 mM, 100 mM) were freshly made by diluting the thawed mother solution into the solvent (H2O or DMSO). The culture medium volume was checked to be 1 ml before any experimental session.
Reagents were then introduced by the following pipetting procedure to ensure proper mixing: 200 μl of medium was removed from the medium bath covering the networks, mixed with a small volume (1 μl) of the reagent dilution and carefully returned to the medium bath in order to minimize any osmotic or hydrodynamic stress. In case of water as solvent additional 0.9 μL of DMSO was added to the culture medium at any administration in order to expose any neuronal network to the same amount of DMSO. Typically concentration–response relationships were determined in a cumulative manner, in which the concentration of drug present in the medium was increased in a stepwise method in log units.
At least two recovery tests were performed for any substance after 24 h, in particular the medium was completely changed after the experiment, and MEA returned to the incubator for 24 h. The spontaneous activity was recorded for 30 min and compared to the activity recorded from the same culture the day before.
Data Recordings and Signal Processing
Standard 60-electrode MEA chips (with 30 μm diameter electrodes, 200 μm inter-electrode spacing with/without an integrated reference electrode) were employed. The activity was recorded by the MEA1060 System from Multi Channel Systems (MCS GmbH, Reutlingen, Germany, http://www.multichannelsystems.com). The system also included a temperature controller (TC02, MCS GmbH) that allowed heating of the MEA chips and thus the medium from the bottom. During recordings, cells were kept at 37°C and in a controlled humid atmosphere (5% CO2, 20% O2 and N2) to buffer the supernatant pH (pH was 7.1 ± 0.1 during whole experiments). The MEA chips were placed into the MEA Amplifier (Gain 1000×) and data were recorded by the MC_Rack software at a sampling rate of 10 kHz. A band pass digital filter (60–4000 Hz) was applied to the raw signal in order to remove electrical background noise. Spike trains were extracted by the MC_Rack spike detection: if the electric signal overcomes the spike detection threshold (i.e., 6.0 ± 0.5 times the SD of the mean square root noise) the spike is identified and recorded.
All analyses were conducted on binned data with bin size of 60 s. Data from experimental episodes were averaged for the last 20 min over the 30-min time window of recording for each concentration and the network mean firing rate (MFR) was extracted as a descriptor of the network activity level. Each time point of the experiment was the average of the firing rate over a 60-s time period. A stable level of spontaneous activity was required in order to start the experiment and was considered as the reference. In general, there is a transition period until equilibrium is achieved which has been established by each laboratory with post hoc analysis in previous experiments. The response during this transition time window has not been considered for the concentration–response analysis The percent change in firing rate at each concentration was then determined relative to the reference spontaneous activity period.
To determine the changes of network activity with time, mean network spike rate of all active channels over the course of the whole experiment were considered. For the purpose of obtaining the IC50 values from the dose–response curve the changes in MFR were considered. Plots were also used to determine the concentration that stopped the activity completely.
Channel Inclusion/Exclusion Criterion
Some simple rules were used for considering the neuronal network and its activity acceptable. The first criterion was based on the network morphology evaluation done by a trained and experienced operator. On a regular basis during the culture time and prior to MEA recording each chip was inspected under microscope to check for the neuronal network morphology and growth basing on the presence of a dense and uniform distribution of neuronal cells and the presence of neuronal connections on the recording area as previously described (Hogberg et al., 2011). The second criterion was based on the electrophysiological activity analysis, and both noisy and silent channels were excluded from the analysis. In particular during the reference activity if the spontaneous firing rate was lower than 10 spikes/bin (bin size of 60 s) the channel was considered a silent channel, and if the spontaneous firing rate was higher than 200 spikes/bin the channel was considered a noisy channel. The average of the excluded channel was 19.96 ± 0.77.
Single dose–Response Acceptance Criteria
The aim of the investigation was to extract the mean effect of the chemical on the activity of the neuronal network once the interaction between the chemical and the neurons’ receptors had reached the saturation phase, the CVTIME of spike rate, i.e., the coefficient of variation of the spike rate in time, was studied to check the stability of the neuronal network activity after the chemical administration. The CV, in general calculated by CV = SD/mean, was used to describe the spatiotemporal behavior of the network activity (Keefer et al., 2001a; Gramowski et al., 2004) and the CVTIME quantify the spatiotemporal behavior reflecting temporal dynamics and the fundamental interactions within the networks. The CVTIME was extracted for each channel and the average of the CVTIME was used as a further exclusion criterion. If the CVTIME never reached and stabilized the 20% for more than one administration, the experiment was rejected. In average the CVTIME reached and stabilized down the threshold by 10 min after the chemical administration.
Statistical Analysis
The Igor Pro 6.1 (Wavemetrics Inc., USA) program was used for statistical analyses. All data given are means of at least three independent experiments ± SEM. Student’s one tail paired t-test was performed to assess differences between basal spontaneous activity and activity after chemical administration. Statistical significance was indicated for P < 0.05. The averaged spike rate as a function of the concentration was fitted to both Hill Equation and Sigmoid Fitting tool for estimating the IC50 value.
The predictivity parameters have been calculated according to the following formulae:
Specificity = number of true negatives/number of true negatives + number of false positives
Sensitivity = number of true positives/number of true positives + number of false negatives
Accuracy = correct identified analytes (positives and negatives)/ total number of analytes.
Results
To assess the maturation of a neuronal network on MEA, the spontaneous activity of a MEA chip was measured every week. As already described (Van Pelt et al., 2005; Wagenaar et al., 2006; Hogberg et al., 2011), at the early time point (7 DIV) only a few of the cultures have shown spontaneous activity that was weak (few active channels and no synchronized pattern), then the activity increased over time and became more and more synchronized with the typical bursting and spiking pattern of cortical neuronal cultures at the age of 28 DIV.
At the maturation time, i.e., after 4 week of in vitro culture, the neuronal networks exhibited spontaneous activity in their respective media, consistent with previously reported effects (Xia et al., 2003; Gramowski et al., 2004; Chiappalone et al., 2006; Shafer et al., 2008).
Network spike rates ranged from (mean ± SEM) 55.06 ± 6.98 spikes/s (n = 60).
During experiments any neuronal network was exposed to DMSO at a final concentration of 0.7%. In order to check the DMSO induced effects, control experiments were performed both with a one-step as well as with progressive administrations and no effects of DMSO on spontaneous activity were recorded (data not shown).
The tested substances were supposed to fall in three main groups according their biological activities based on legal classification (EBC–JRC) and published in vivo and in vitro data, namely (1) not neuroactive and not toxic, (2) toxic but not neuroactive, and (3) neurotoxic substances. The results obtained are summarized in Table 4.
Not Neuroactive and Not Toxic Substances
In accordance to their in vivo data, these two substances had no significant effect on network activity (Figure 1). 1,2 Propandiol caused a slight increase in the firing rate at high concentrations, but this effect is not statistically significant.
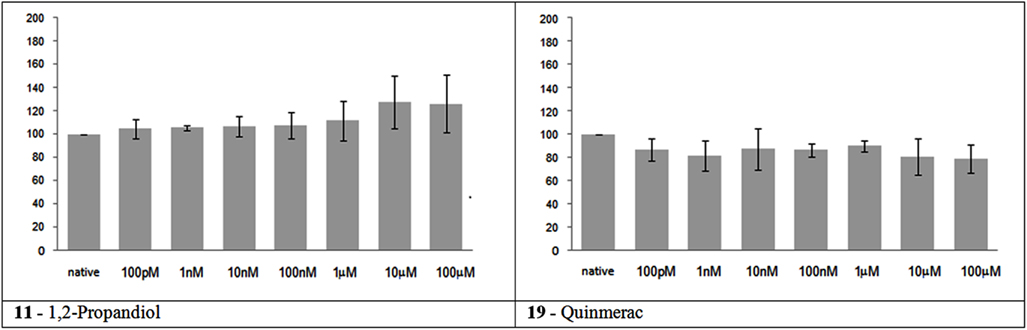
Figure 1. Electrical activity effects on neuronal cultures after administration of not neuroactive and not toxic substances. Electrical activity following administration of substances classified in the not neuroactive and not toxic group (at the concentration indicated under the bars) has been recorded and normalized in respect to native activity (percent of control, indicated as 100 in the ordinate axis). All data are means of at least three independent experiments ± SEM. Student’s one tail paired t-test was performed to assess differences between basal spontaneous activity and activity after chemical administration. Statistical significance was indicated by * for P < 0.05.
Toxic but Not Neuroactive Substances
The substances in this group had no significant effect on MFR, as expected, at the concentrations tested, with the exception of aniline that presented a mild but significant progressive increase in the MFR during the experiment (Figure 2).
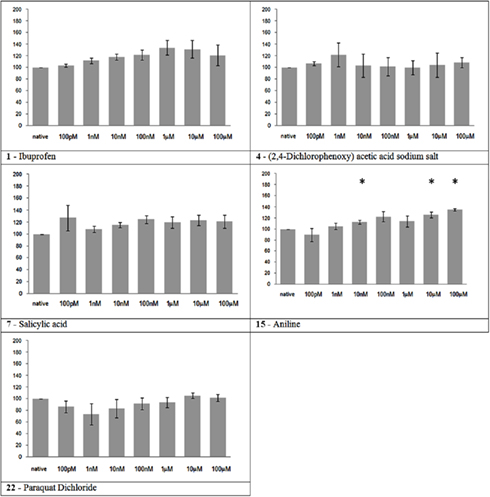
Figure 2. Electrical activity effects on neuronal cultures after administration of toxic but not neuroactive substances. Electrical activity following administration of substances classified in the toxic but not neuroactive group (at the concentration indicated under the bars) has been recorded and normalized in respect to native activity (percent of control, indicated as 100 in the ordinate axis). All data are means of at least three independent experiments ± SEM. Student’s one tail paired t-test was performed to assess differences between basal spontaneous activity and activity after chemical administration. Statistical significance was indicated by * for P < 0.05.
Neuroactive Substances
Nine of the thirteen substances in this group showed a significant effect on neuronal network activity (Figure 3). All the substances that caused an MFR reduction, or its total cessation, belongs to this group. Nicotine showed a slight increase in MFR at the maximum tested concentration. And three substances, namely ethanol, mepiquat, and toluene, did not show a significant effect.
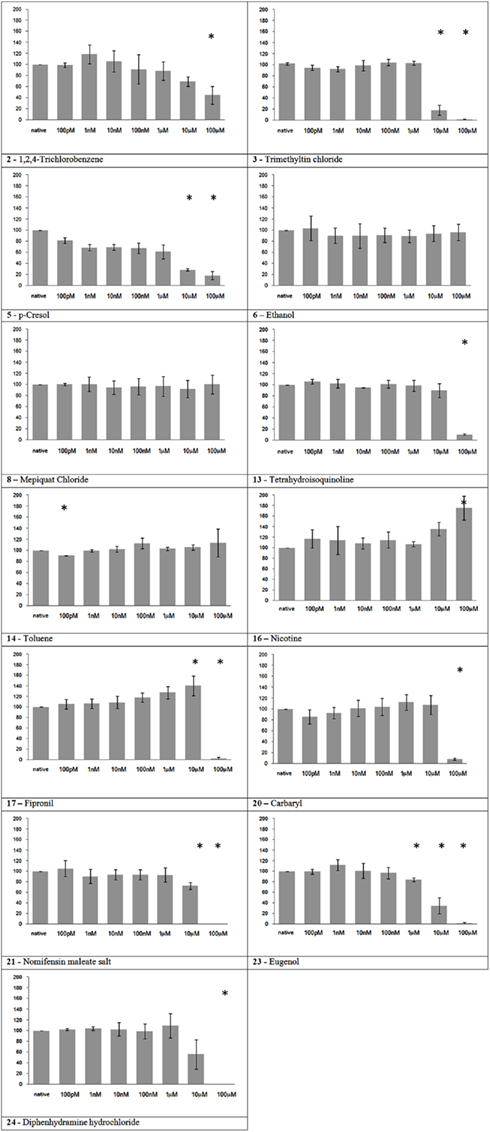
Figure 3. Electrical activity effects on neuronal cultures after administration of neuroactive substances. Electrical activity following administration of substances classified in the neuroactive group (at the concentration indicated under the bars) has been recorded and normalized in respect to native activity (percent of control, indicated as 100 in the ordinate axis). All data are means of at least three independent experiments ± SEM. Student’s one tail paired t-test was performed to assess differences between basal spontaneous activity and activity after chemical administration. Statistical significance was indicated by * for P < 0.05.
Recovery Tests
To have an indication about the reversibility of the effects of the substances utilized, a recovery test was performed after 24 h following a complete medium wash-out, and the electrical activity compared to the basal activity recorded on the same culture the day before. The recovery results were classified according to a three level scale: A – good recovery (level of activity more than 70% of the spontaneous activity recorded during the reference period the day before); B – average recovery (level of activity between 35 and 70%); C – poor recovery (recovered activity less than 35%). Five substances showed a good recovery (Table 4), and two of them [Carbaryl (S20) and Nomifensine (S21)] initially showed high neuroactivity, indicating the reversibility of their effects. TMT (S3) and Eugenol (S23) had a poor recovery, which indicates that there was a strong neuroactive effect that ended in cytotoxicity. For all the other substances the recovery was average.
Discussion
The problem of toxicity assessment of all chemicals sold in Europe according to the REACH regulation is particularly challenging: a recent research (Hartung and Rovida, 2009) presented a very critical situation. Industries are already facing the submission of existing toxicity data and animal-testing plans for part of the “old” chemicals because much information, such as reproductive toxicity, neurotoxicity, developmental neurotoxicity, etc., is missing. Current in vivo test methods are based on behavioral and sensory perturbations coupled with routine histopathological investigations. In spite of the empirical usefulness of these tests, they are not always sensitive enough, and often they do not provide information that facilitates a detailed understanding of potential mechanisms of toxicity, thus enabling predictions. Regarding the status of in vitro tests, they are generally not used to detect neurotoxicity for prediction of hazards to human health and so far they play a complementary role to the in vivo tests (Bal-Price et al., 2010). Furthermore, the current in vitro test methods are far from being capable of testing large numbers of chemicals in a short time. High throughput and high content screening (HTS/HTC) methods have been proposed as an integral component of future toxicity testing strategies (Costa, 1998; Coecke et al., 2006; Lein et al., 2007; NRC, 2007). However, they are often not designed to assess the physiology and functionality of living neurons, which may result in missing the effects of some chemicals and/or hamper the identification of toxicity pathways related to higher level functions of the nervous system. The set up and validation of an alternative in vitro method constitute therefore a topic of central interest in order to reduce, refine and replace animal-testing.
So far, the MEA technique has been used in several pharmacological and toxicological studies (Gross et al., 1997; Gramowski et al., 2000,2006; Morefield et al., 2000; Keefer et al., 2001b; Sundstrom et al., 2005; Parviz and Gross, 2007; van Vliet et al., 2007) and the IC50 ranges have been established in the same range as those published for other toxicological studies and are in general in agreement with those obtained from animal experiments (Xia and Gross, 2003; Xia et al., 2003). However, until now MEAs were not tested as an in vitro model for neurotoxicity testing of large varieties of chemicals. Comparing the results from this study with neuronal network on MEA to published in vivo data, there were 10 substances identified as true positives, 1 as false positive, 6 true negative and 3 false negative. Based on these data the sensitivity of the assay was 77% and the specificity was 86%. This makes an overall accuracy of 80% for the assay.
Parameters Extraction/Activity Evaluation Methods
Despite the large number of applications and studies related to the use of MEA-based systems, there are still a few universally accepted methodologies to extract information to assess neurotoxicity. While bursting behavior and bursting synchronization over active channels are important criteria for verifying the maturation of the in vitro neuronal network (i.e., quality of the activity), burst parameters such as mean bursting rate, burst duration, mean spikes in burst, etc., that were shown to provide important information for basic research (e.g., Wagenaar et al., 2006; Novellino et al., 2007), did not give any particular advantage for neurotoxicity assessment. As the burst parameters are extracted from spike trains the accuracy and reliability were mostly the same as the MFR (data not shown), while the computation time and complexity were higher. Available methods are based on spike detection algorithms that produce multi-site spike, which can be further analyzed for spike sorting, first and higher order statistics (e.g., burst analysis) and cross correlation based methods. A recent paper from Maccione et al. (2009) demonstrated that the accuracy of spike detection methods is quite comparable, and if the goal of the study is not the precise timing but rather the average effects on a large binning window (e.g., 1 min of activity) the computational requirement for accurate spike detection is not necessary since a simple spike threshold identification provides a simple yet sufficient method.
Effects of Administered Chemicals
The results of this blinded study consistently determined the effects for 20 chemicals based on the inhibition of spontaneous neuronal network activity (i.e., MFR at neuronal network level). Indeed, the estimated IC50 values for all 20 compounds were within less than one order of magnitude. This reproducibility, despite differences in sources of cells and age at recording, demonstrates the robustness of MEA approaches for neurotoxicity tests. For this investigation a wide range of concentrations have been explored, spanning through six orders of magnitude, from 100 pM to 100 μM. The lowest did not show significant activity variations, but have been useful to test the robustness of the system in regard to mechanical manipulation. Furthermore, given the sensibility of the system and the explorative nature of the study, it was considered useful to test a wide range of concentrations. The MEA-based paradigm can be very helpful for recognizing toxic and non-toxic compounds in a middle throughput screening assay. Although it is useful that the assay accurately and sensitively predict the toxic range of a substance, in case of chemical labeling and risk assessment the most important question is whether the chemical is toxic and which is the order of magnitude of the concentration in which it starts being toxic. Following this approach, the estimated IC50 are indicative of the order of magnitude of the registered effects in order to deal with the obtained results, and not meant to constitute a precise toxicological characterization of the analytes. Refinement of the employed conditions is surely required for a more precise and refined description of the action mechanism of the different substances.
The tested substances were supposed to fall into three main groups according their biological activities based on legal classification (EBC–JRC) and published in vivo data, namely (1) non-neuroactive and non-toxic, (2) toxic but non-neuroactive and (3) neuroactive substances. The substances of the first two groups showed no detectable effect on neuronal activity in NN MEA, with the exception of Aniline, which caused a gradual increase in MFR. This represents the only false positive detected in this study. The slight increase in MFR registered following 1,2-Propandiol administration was not significant, and likely connected to unspecific effects. Regarding the 13 substances classified as neuroactive in vivo, the NN MEA assay registered nine substances causing a significant decrease of the MFR, one substance (nicotine) giving a significant increase at the highest concentration, and three substances, namely ethanol, mepiquat, and toluene, that showed no significant activity.
In vitro active neuroactive substances
All the MFR reductions registered in the present paper, either complete or partial, have been registered following the application substances belonging to this group.
Trimethyltin chloride. In agreement with its high neurotoxicity, trimethyltin chloride demonstrated the strongest action on cortical network activity between the tested chemicals. The registered behavior is in agreement with a previous study on MEAs reporting an EC50 of 4.3 μM for auditory cortex and 1.5 μM for spinal cord tissue derived from mouse embryonic tissue (Gramowski et al., 2000). Moreover, Kuramoto et al. (2011) showed in primary mouse cultures that trimethyltin toxicity is initially caused by activation of the caspase pathway in cortical neurons, which is in agreement with our recovery result, that show no reversible network activity on the next day.
Nicotine. In our experiment, nicotine showed an increase of the MFR at the highest tested concentration (100 μM). This result is apparently in contrast with its high potency in vivo. To date, the exact role of brain nAChRs is already a debated topic. In vivo, nicotine effects are variable depending on the subtype and density of the different nicotinic receptors existing in the distinct brain regions (Toledano et al., 2010). A high density of nicotine receptors is found in the thalamus, caudate nucleus, and substantia nigra (Paterson and Nordberg, 2000), composed by various homomeric or heteromeric combinations of 12 different subunits (Mansvelder et al., 2009). Their location in the brain is not limited to postsynaptic but also to pre-, peri-, and extra-synaptic sites where they may modulate neuronal function by a variety of actions (Lindstro, 1997). In particular, presynaptic nAChRs exist on several cell populations in cortical, hippocampal, and cerebellar brain regions (Wonnacott, 1997). Although a subset of nAChRs are postsynaptic located on a subset of GABAergic interneurons in cortical layer, nAChRs in cortex are mostly found on afferent terminals, in particular from thalamic neurons (Matherate, 2004), and consequently not present in cortical neuron preparations. This nAChRs distribution in the brain may explain the slight increase of activity at the highest concentration in NN MEA. In addition it suggests a need to consider different brain regions in NN MEA assays.
Fipronil. The initial excitatory effect is probably caused by the antagonist action on GABAa receptors, while the final inhibition might be due to a neurotoxic effect that affect the generation of spontaneous oscillatory activity of the network.
Eugenol. Eugenol showed, somehow unexpectedly, a rather strong neuroactive action. Eugenol’s effects on sensorial peripheral nerves have been studied, but there are few papers on its action on CNS. In a recent report, rat neocortical and hippocampal slices, eugenol (10–100 μM) showed inhibitory effects on elicited epileptiform discharges and potassium-induced spreading depression (Müller et al., 2006). Another study reported decreased population spikes amplitude in hippocampal slices (Ardjmand et al., 2006). The discussed findings are in accordance with our observation of a clear-cut inhibitory effect starting at 10 μM with almost complete cessation at 100 μM.
In vitro not-active neuroactive substances
As previously discussed, three substances belonging to the neuroactive group, showed no impairment of electrical activity in our experiment.
Toluene, despite its well known neurotoxic actions, did not show any effect in our experiments (the slight decrease of activity at 100 pM is likely due to the mechanical manipulation rather than a true substance-related effect). Toluene volatility could have diminished its availability for the cultured neurons, even if after administration MEAs have been covered with semipermeable caps to avoid evaporation. Indeed, a transient decrease of network activity has been observed immediately after the administration of the maximum concentration, but disappeared a few minutes later (data not shown, personal observation of the experimenter).
Regarding ethanol, it is worth noting that the maximum concentration of 100 μM in our tests equals a blood alcohol level of 0.005% which does not cause pronounced effects in humans. Accordingly, the lack of effects on neuronal network activity at lower concentrations has previously been reported; initial inhibition was only observed at 20 mM and total activity cessation at 100 mM (Xia and Gross, 2003).
Also Mepiquat chloride had no effect on our experiments, even if neurotoxicity has been reported in in vivo studies. The first mild diminution in activity at the first tested concentration that results as statistically significant is probably due to mechanical manipulation. Interestingly, Mepiquat neuroactive effects are already in part mediated by nicotinic receptor binding (EFSA, 2008), and this could be a critical aspect to detect in cortical neurons network as discussed for nicotine effects.
The evaluation of the effects on MFR after substance administration is certainly informative about neuronal activity, but is not a clear description for a neuroactive effect. A strong cytotoxic compound will end in a total cessation too, by measuring the electrical activity. Therefore it is important to test also cytotoxic markers to be able to distinguish between neuroactive and cytotoxic effects. We decided to investigate in a first experiment the recovery of the neuronal networks after a wash-out of the chemical substance as an indicator for cytotoxicity. Some of the substances showed absolutely no recovery (as shown, e.g., for TMT), the majority of substances showed a moderate recovery of activity, between 35 and 70%, which makes it difficult to distinguish between incomplete recovery, already present chemical agent, cytotoxic actions, or other effects related to manipulation. Multiple medium exchanges would be required to achieve a total wash-out, especially for water insoluble compounds, and together with different times of recovery before registration could help in generating more clear data. However, looking at a method to get information about the way of toxicity action of different substances, we propose that other endpoints could be more informative as an integration to electrophysiological measurements, such as the cytotoxicity assessment (for example by measuring the release of lactate dehydrogenase into the culture medium), or the evaluation of the metabolic state of the cells. The alterations of electrical activity induced by a compound’s application (functional neuroactivity) is indeed often not associated to cell death (cytotoxicity), or direct cellular physiology impairment. This is consistent with the acute neurotoxicity of many xenobiotics (e.g., ethanol, pyrethroids, tetrodotoxin, etc.) that cause the organism death prior to the onset of significant cytotoxicity in the nervous system. While not examined in these experiments, other studies (Novellino et al., 2011) showed that the compounds modified neuronal network activity in the absence of cytotoxicity. The evaluation of the previously mentioned endpoints could help in distinguishing between the different toxic effects.
Conclusion
For toxicological prediction of a compound, the detection and characterization of chemical-induced toxic effects in the central and peripheral nervous system represents a major challenge for employing newly developed technologies in the field of neurotoxicity (Dunlop et al., 2008). The use of neuronal cultures growing on MEAs innovates the field of neurotoxicity: a neuronal network coupled to MEA represents a simplified model of the nervous system in which the electric activity is measured in real-time while being exposed to tested substances. The presented feasibility study provides a further evidence of the potential use and usefulness of the proposed paradigm as an alternative method for gathering neurotoxicity information for chemical risk assessment, especially under a chemical industry perspective. So far the assay appears to be a reliable tool to exclude neuroactivity of chemicals in an early screening, and therefore we shall extend the evaluation of the NN MEA as a screening tool for the neurotoxic effects of chemicals.
Conflict of Interest Statement
The authors declare that the research was conducted in the absence of any commercial or financial relationships that could be construed as a potential conflict of interest.
References
Abe, K., Saitoh, T., Horiguchi, Y., Utsunomiya, I., and Taguchi, K. (2005). Synthesis and neurotoxicity of tetrahydroisoquinoline derivatives for studying Parkinson’s disease. Biol. Pharm. Bull. 28, 1355–1362.
Ardjmand, A., Fathollahi, Y., Sayyah, M., Kamalinejad, M., and Omrani, A. (2006). Eugenol depresses synaptic transmission but does not prevent the induction of long-term potentiation in the CA1 region of rat hippocampal slices. Phytomedicine 13, 146–151.
Bal-Price, A. K., Hogberg, H. T., Buzanska, L., Lenas, P., van Vliet, E., and Hartung, T. (2010). In vitro developmental neurotoxicity (DNT) testing: relevant models and endpoints. Neurotoxicology 31, 545–554.
Battaglia, V., Salvi, M., and Toninello, A. (2005). Oxidative stress is responsible for mitochondrial permeability transition induction by salicylate in liver mitochondria. J. Biol. Chem. 280, 33864–33872.
Bouldin, T. W., Goines, N. D., Bagnell, R. C., and Krigman, M. R. (1981). Pathogenesis of trimethyltin neuronal toxicity. Ultrastructural and cytochemical observations. Am. J. Pathol. 104, 237–249.
Breckenridge, L. J., Wilson, R. J., Connolly, P., Curtis, A. S., Dow, J. A., Blackshaw, S. E., and Wilkinson, C. D. (1995). Advantages of using microfabricated extracellular electrodes for in vitro neuronal recording. J. Neurosci. Res. 42, 266–276.
Brown, A. W., Aldridge, W. N., Street, B. W., and Verschoyle, R. D. (1979). The behavioral and neuropathologic sequelae of intoxication by trimethyltin compounds in the rat. Am. J. Pathol. 97, 59–82.
Chang, L. W., Tiemeyer, T. M., Wenger, G. R., and McMillan, D. E. (1982). Neuropathology of mouse hippocampus in acute trimethyltin intoxication. Neurobehav. Toxicol. Teratol. 4, 149–156.
Chiappalone, M., Bove, M., Vato, A., Tedesco, M., and Martinoia, S. (2006). Dissociated cortical networks show spontaneously correlated activity patterns during in vitro development. Brain Res. 1093, 41–53.
Coecke, S., Eskes, C., Garlton, J., Kinsner, A., Price, A., van Vliet, E., Prieto, P., Boveri, M., Bremer, S., Adler, S., Pellizzer, C., Wendel, A., and Hartung, T. (2006). The value of alternative testing for neurotoxicity in the context of regulatory needs. Environ. Toxicol. Pharmacol. 21, 153–157.
Costa, L. G. (1998). Biochemical and molecular neurotoxicology: relevance to biomarker development, neurotoxicity testing and risk assessment. Toxicol. Lett. 102–103, 417–421.
Crofton, K. M., Makris, S. L., Sette, W. F., Mendez, E., and Raffaele, K. C. (2004). A qualitative retrospective analysis of positive control data in developmental neurotoxicity studies. Neurotoxicol. Teratol. 26, 345–352.
Deleu, D., and Hanssens, Y. (2000). Cerebellar dysfunction in chronic toluene abuse: beneficial response to amantadine hydrochloride. J. Toxicol. Clin. Toxicol. 38, 37–41.
D’Hooge, R., Van de Vijver, G., Van Bogaert, P. P., Marescau, B., Vanholder, R., and De Deyn, P. P. (2003). Involvement of voltage- and ligand-gated Ca2 ( channels in the neuroexcitatory and synergistic effects of putative uremic neurotoxins. Kidney Int. 63, 1764–1775.
Dinis-Oliveira, R. J., Duarte, J. A., Sánchez-Navarro, A., Remião, F., Bastos, M. L., and Carvalho, F. (2008). Paraquat poisonings: mechanisms of lung toxicity, clinical features, and treatment. Crit. Rev. Toxicol. 38, 13–71.
Dunlop, J., Bowlby, M., Peri, R., Vasilyev, D., and Arias, R. (2008). High-throughput electrophysiology: an emerging paradigm for ion-channel screening and physiology. Nat. Rev. Drug Discov. 7, 358–368.
Dyer, R. S., Walsh, T. J., Wonderlin, W. F., and Bercegeay, M. (1982). The trimethyltin syndrome in rats. Neurobehav. Toxicol. Teratol. 4, 127–133.
EFSA. (2008). Conclusion regarding the peer review of the pesticide risk assessment of the active substance mepiquat. Scientific Report 2008 146, 1–73. Available at: http://www.efsa.europa.eu/en/efsajournal/doc/praper_concl_sr146_mepiquat_en_web,0.pdf?ssbinary=true
Flanagan, R. J., Braithwaite, R. A., Brown, S. S., Widdop, B., and de Wolff, F. A. (1995). The International Programme on Chemical Safety. Geneva: Basic Analytical Toxicology WHO
Gelpí, E., Posada de la Paz, M., Terracini, B., Abaitua, I., de la Cámara, A. G., Kilbourne, E. M., Lahoz, C., Nemery, B., Philen, R. M., Soldevilla, L., and Tarkowski, S. (2002). The spanish toxic oil syndrome 20 years after its onset: a multidisciplinary review of scientific knowledge. Environ. Health Perspect. 110, 457–464.
Gordon, C. J., Herr, D., Gennings, C., Gennings, C., Graff, J., and McMurray, M. (2006). Thermoregulatory response to an organophosphate and carbamate insecticide mixture: testing the assumption of dose additivity. Toxicology 217, 1–13.
Gramowski, A., Jugelt, K., Weiss, D. G., and Gross, G. W. (2004). Substance identification by quantitative characterization of oscillatory activity in murine spinal cord networks on microelectrode arrays. Eur. J. Neurosci. 19, 2815–2825.
Gramowski, A., Schiffmann, D., and Gross, G. W. (2000). Quantification of acute neurotoxic effects of trimethyltin using neuronal networks cultured on microelectrode arrays. Neurotoxicology 21, 331–342.
Gramowski, A., Stuewe, S., Juegelt, K., Schiffmann, D., Loock, J., Schroeder, O., Gross, G. W., and Weiss, D. G. (2006). Detecting neurotoxicity through electrical activity changes of neuronal networks on multielectrodeneurochips. ALTEX 23(Suppl.), 410–415.
Gross, G. W., Harsch, A., Rhoades, B. K., and Gopel, W. (1997). Odor, drug and toxin analysis with neuronal networks in vitro: extracellular array recording of network responses. Biosens. Bioelectron. 12, 373–393.
Gross, G. W., Rhoades, B. K., Reust, D. L., and Schwalm, F. U. (1993). Stimulation of monolayer networks in culture through thin-film indium-tin oxide recording electrodes. J. Neurosci. Methods 50, 131–143.
Gross, G. W., Williams, A. N., and Lucas, J. H. (1982). Recording of spontaneous activity with photoetched microelectrode surfaces from mouse spinal neurons in culture. J. Neurosci. Methods 5, 13–22.
Grossmann, K., Kwiatkowski, J., and Tresch, S. (2001). Auxin herbicides induce H(2)O(2) overproduction and tissue damage in cleavers (Galium aparine L.). J. Exp. Bot. 52, 1811–1816.
Guenette, S. A., Beaudry, F., Marier, J. F., and Vachon, P. (2006). Pharmacokinetics and anesthetic activity of eugenol in male Sprague-Dawley rats. J. Vet. Pharmacol. Ther. 29, 265–270.
Hartung, T., Bremer, S., Casati, S., Coecke, S., Corvi, R., Fortaner, S., Gribaldo, L., Halder, M., Hoffmann, S., Roi, A. J., Prieto, P., Sabbioni, E., Scott, L., Worth, A., and Zuang, V. (2003). ECVAM’s response to the changing political environment for alternatives: consequences of the European Union chemicals and cosmetics policies. Altern. Lab. Anim. 31, 473–481.
Hartung, T., Bremer, S., Casati, S., Coecke, S., Corvi, R., Fortaner, S., Gribaldo, L., Halder, M., Hoffmann, S., Roi, A. J., Prieto, P., Sabbioni, E., Scott, L., Worth, A., and Zuang, V. (2004). A modular approach to the ECVAM principles on test validity. Altern. Lab. Anim. 32, 467–472.
Hartung, T., and Rovida, C. (2009). That which must not, can not be …. A reply to the EChA and EDF responses to the REACH analysis of animal use and costs. ALTEX 26, 307–311.
Hogberg, T. H., Sobanski, T., Novellino, A., Weiss, D. G., van Vliet, E., Whelan, M., and Bal-Price, A. K. (2011). Application of micro electrode arrays (MEAs) as an emerging technology for developmental neurotoxicity: evaluation of domoic acid-induced effects in primary cultures of rat cortical neurons. Neurotoxicology 32, 158–168.
IPCS. (1991). “Chlorobenzenes other than hexachlorobenzene,” in International Programme on Chemical Safety. Environmental Health Criteria 128 (Geneva: World Health Organization), 252
Johnstone, A. F., Gross, G. W., Weiss, D. G., Schroeder, O. H., Gramowski, A., and Shafer, T. J. (2010). Microelectrode arrays: a physiologically based neurotoxicity testing platform for the 21st century. Neurotoxicology 31, 331–350.
Kamran, S., and Bakshi, R. (1998). MRI in chronic toluene abuse: low signal in the cerebral cortex on T2-weighted images. Neuroradiology 40, 519–521.
Kavitha, V., and Palanivelu, K. (2005). Destruction of cresols by Fenton oxidation process. Water Res. 39, 3062–3072.
Keefer, E. W., Gramowski, A., and Gross, G. W. (2001a). NMDA receptor-dependent periodic oscillations in cultured spinal cord networks. J. Neurophysiol. 86, 3030–3042.
Keefer, E. W., Norton, S. J., Boyle, N. A., Talesa, V., and Gross, G. W. (2001b). Acute toxicity screening of novel AChE inhibitors using neuronal networks on microelectrode arrays. Neurotoxicology 22, 3–12.
Kinney, J. L. (1985). Nomifensine maleate – a new 2nd-generation antidepressant. Clin. Pharm. 4, 625–636.
Kuramoto, N., Seko, K., Sugiyama, C., Shuto, M., and Ogita, K. (2011). Trimethyltin initially activates the caspase 8/caspase 3 pathway for damaging the primary cultured cortical neurons derived from embryonic mice. J. Neurosci. Res. 89, 552–561.
Lane, B. W., Ellenhorn, M. J., Hulbert, T. V., and McCarron, M. (1991). Clove oil ingestion in an infant. Hum. Exp. Toxicol. 10, 291–294.
Lein, P., Locke, P., and Goldberg, A. (2007). Meeting report: alternatives for developmental neurotoxicity testing. Environ. Health Perspect. 115, 764–768.
Lindstro, J. (1997). Nicotinic acetylcholine receptors in health and disease. Mol. Neurobiol. 15, 193–222.
Maccione, A., Gandolfo, M., Massobrio, P., Novellino, A., Martinoia, S., and Chiappalone, M. (2009). A novel algorithm for precise identification of spikes in extracellularly recorded neuronal signals. J. Neurosci. Methods 177, 241–249.
Mansvelder, H. D., Mertz, M., and Role, L. W. (2009). Nicotinic modulation of synaptic transmission and plasticity in cortico-limbic circuits. Semin. Cell Dev. Biol. 20, 432–440.
Martinoia, S., Bonzano, L., Chiappalone, M., Tedesco, M., Marcoli, M., and Maura, G. (2005). In vitro cortical neuronal networks as a new high-sensitive system for biosensing applications. Biosens. Bioelectron. 20, 2071–2078.
Miller, G. W. (2007). Paraquat: the red herring of Parkinson’s disease research. Toxicol. Sci. 100, 1–2.
Monnet-Tschudi, F., Zurich, M. G., and Honegger, P. (1997). “Aggregate cell cultures for neurotoxicity testing: the importance of cell-cell interactions,” in Animal Alternatives, Welfare and Ethics, eds L. F. M. van Zutphen, and M. Balls (Amsterdam: Elsevier), 641–649.
Morefield, S. I., Keefer, E. W., Chapman, K. D., and Gross, G. W. (2000). Drug evaluations using neuronal networks cultured on microelectrode arrays. Biosens. Bioelectron. 15, 383–396.
Müller, M., Pape, H. C., Speckmann, E. J., and Gorji, A. (2006). Effect of eugenol on spreading depression and epileptiform discharges in rat neocortical and hippocampal tissues. Neuroscience 140, 743–751.
Nanau, R. M., and Neuman, M. G. (2010). Ibuprofen-induced hypersensitivity syndrome. Transl. Res. 155, 275–293.
Novellino, A., D’Angelo, P., Cozzi, L., Chiappalone, M., Sanguineti, V., and Martinoia, S. (2007). Connecting neurons to a robot: an in vitro bidirectional neural interface. Comput. Intell. Neurosci. 2007, 121725.
Novellino, A., Scelfo, B., Palosaari, T., Price, A., Sobanski, T., Shafer, T. J., Johnstone, A. F. M., Gross, G. W., Gramowski, A., Schroeder, O., Chiappalone, M., Benfenati, F., Martinoia, S., Tedesco, M. T., Defranchi, E., D’Angelo, P., and Whelan, M. (2011). Development of micro-electrode array based tests for neurotoxicity: assessment of interlaboratory reproducibility with neuroactive chemicals. Front. Neuroeng. 4:4.
Novellino, A., and Zaldívar, J. M. (2010). Recurrence quantification analysis of spontaneous electrophysiological activity during development: characterization of in vitro neuronal networks cultured on multi electrode array chips. Adv. Artif. Intell. 1–10.
NRC. (2007). Committee on Toxicity Testing and Assessment of Environmental Agents, National Research Council. Toxicity Testing in the 21st Century: A Vision and a Strategy. National Research Council; National Academies of Science. Washington, DC: The National Academies Press. Available at: http://www.nap.edu/catalog.php?record_id=11970
Nuwayhid, S. J., and Werling, L. L. (2006). Sigma(2) (sigma(2)) receptors as a target for cocaine action in rat striatum. Eur. J. Pharmacol. 535, 98–103.
Obach, R. S., and Dalvie, D. K. (2006). Metabolism of nomifensine to a dihydroisoquinolinium ion metabolite by human myeloperoxidase, hemoglobin, monoamine oxidase A, and cytochrome P450 enzymes. Drug Metab. Dispos. 34, 1310–1316.
OECD. (1997). Test Guideline No. 424: OECD Guideline for Testing of Chemicals. Neurotoxicity Study in Rodents. Available at: http://www.oecdilib rary.org/docserver/download/fullte xt/9742601e.pdf?expires=126089388 0&id=0000&accname=freeContent &checksum=FA8DCD77CAB11C14 460B2CCB0B650DD6
Ossowska, K., Smialowska, M., Kuter, K., Wieronska, J., Zieba, B., Wardas, J., Nowak, P., Dabrowska, J., Bortel, A., Biedka, I., Schulze, G., and Rommelspacher, H. (2006). Degeneration of dopaminergic mesocortical neurons and activation of compensatory processes induced by a long-term paraquat administration in rats: implications for Parkinson’s disease. Neuroscience 141, 2155–2165.
Pancrazio, J. J., Gray, S. A., Shubin, Y. S., Kulagina, N., Cuttino, D. S., Shaffer, K. M., Eisemann, K., Curran, A., Zim, B., Gross, G. W., and O’Shaughnessy, T. J. (2003). A portable microelectrode array recording system incorporating cultured neuronal networks for neurotoxin detection. Biosens. Bioelectron. 18, 1339–1347.
Parviz, M., and Gross, G. W. (2007). Quantification of zinc toxicity using neuronal networks on microelectrode arrays. Neurotoxicology 28, 520–531.
Paterson, D., and Nordberg, A. (2000). Neuronal nicotinic receptors in the human brain. Prog. Neurobiol. 61, 75–111.
Philbert, M. A., Billingsley, M. L., and Reuhl, K. R. (2000). Mechanisms of injury in the central nervous system. Toxicol. Pathol. 28, 43–53.
Pohl-Guimaraes, F., Calaza, K. D., Yamasaki, E. N., Kubrusly, R. C. C., and Reis, R. A. D. (2010). Ethanol increases GABA release in the embryonic avian retina. Int. J. Dev. Neurosci. 28, 189–194.
Potter, S. M. (2001). Distributed processing in cultured neuronal networks. Prog. Brain Res. 130, 49–62.
Potter, S. M., and DeMarse, T. B. (2001). A new approach to neural cell culture for long-term studies. J. Neurosci. Methods 110, 17–24.
Schepers, E., Meert, N., Glorieux, G., Goeman, J., Van der Eycken, J., and Vanholder, R. (2007). P-cresylsulphate, the main in vivo metabolite of p-cresol, activates leucocyte free radical production. Nephrol. Dial. Transplant. 22, 592–596.
Sewell, G., Nanry, K. P., Kennedy, J., Stiger, T. R., and Harmon, R. E. (1985). Supra-additive toxic interaction of nicotine with antihistamines, and enhancement by the proconvulsant pentylenetetrazole. Pharmacol. Biochem. Behav. 22, 469–477.
Shafer, T. J., Rijal, S. O., and Gross, G. W. (2008). Complete inhibition of spontaneous activity in neuronal networks in vitro by deltamethrin and permethrin. Neurotoxicology 29, 203–212.
Streit, J. (1993). Regular oscillations of synaptic activity in spinal networks in vitro. J. Neurophysiol. 70, 871–878.
Sundstrom, L., Morrison, B. III, Bradley, M., and Pringle, A. (2005). Organotypic cultures as tools for functional screening in the CNS. Drug Discov. Today 10, 993–1000.
Tabakman, R., Lecht, S., Sephanova, S., Arien-Zakay, H., and Lazarovici, P. (2004). Interactions between the cells of the immune and nervous system: neurotrophins as neuroprotection mediators in CNS injury. Prog. Brain Res. 146, 387–401.
Tayeb, W., Nakbi, A., Trabelsi, M., Attia, N., Miled, A., and Hammami, M. (2010). Hepatotoxicity induced by sub-acute exposure of rats to 2,4-dichlorophenoxyacetic acid based herbicide Désormonelourd. J. Hazard. Mater. 180, 225–233.
Thrash, B., Uthayathas, S., Karuppagounder, S. S., Suppiramaniam, V., and Dhanasekaran, M. (2007). Paraquat and maneb induced neurotoxicity. Proc. West. Pharmacol. Soc. 50, 31–42.
Toledano, A., Alvarez, M. I., and Toledano-Díaz, A. (2010). Diversity and variability of the effects of nicotine on different cortical regions of the brain – therapeutic and toxicological implications. Cent. Nerv. Syst. Agents Med. Chem. 10, 180–206.
US EPA. (1994). 1,2,4-Trichlorobenzene. U.S. Environmental Protection Agency. Integrated Risk Information System (IRIS) Online. Cincinnati, OH: Office of Health and Environmental Assessment, USEPA
US EPA. (1998). Health Effects Guidelines OPPTS 870.6300 Developmental Neurotoxicity Study. Available at: http://www.epa.gov/ opptsfrs/publications/Test_Guide lines/series870.htm
Uyanikgil, Y., Ates, U., Baka, M., Biçer, S., Oztas, E., and Ergen, G. (2009). Immunohistochemical and histopathological evaluation of 2,4-dichlorophenoxyacetic acid-induced changes in rat kidney cortex. Bull. Environ. Contam. Toxicol. 82, 749–755.
Van Pelt, J., Corner, M. A., Wolters, P. S., Rutten, W. L., and Ramakers, G. J. (2004a). Long-term stability and developmental changes in spontaneous network burst firing patterns in dissociated rat cerebral cortex cell cultures on multielectrode arrays. Neurosci. Lett. 361, 86–89.
Van Pelt, J., Wolters, P. S., Corner, M. A., Rutten, W. L., and Ramakers, G. J. (2004b). Long-term characterization of firing dynamics of spontaneous bursts in cultured neural
Van Pelt, J., Vajda, I., Wolters, P. S., Corner, M. A., and Ramakers, G. J. (2005). Dynamics and plasticity in developing neuronal networks in vitro. Prog. Brain Res. 147, 173–188.
van Vliet, E., Stoppini, L., Balestrino, M., Eskes, C., Griesinger, C., Sobanski, T., Whelan, M., Hartung, T., and Coecke, S. (2007). Electrophysiological recording of re-aggregating brain cell cultures on multi-electrode arrays to detect acute neurotoxic effects. Neurotoxicology 28, 1136–1146.
Xia, Y., Gopal, K. V., and Gross, G. W. (2003). Differential acute effects of fluoxetine on frontal and auditory cortex networks in vitro. Brain Res. 973, 151–160.
Xia, Y., and Gross, G. W. (2003). Histiotypic electrophysiological responses of cultured neuronal networks to ethanol. Alcohol 30, 167–174.
Yamanouchi, N., Okada, S., Kodama, K., Hirai, S., Sekine, H., Murakami, A., Komatsu, N., Sakamoto, T., and Sato, T. (1995). White matter changes caused by chronic solvent abuse. AJNR Am. J. Neuroradiol. 16, 1643–1649.
Wagenaar, D. A., Pine, J., and Potter, S. M. (2006). An extremely rich repertoire of bursting patterns during the development of cortical cultures. BMC Neurosci. 7, 11.
Keywords: neurotoxicity, neuronal networks, in vitro assay, micro-electrode array, chemical test
Citation: Defranchi E, Novellino A, Whelan M, Vogel S, Ramirez T, van Ravenzwaay B and Landsiedel R (2011) Feasibility assessment of micro-electrode chip assay as a method of detecting neurotoxicity in vitro. Front. Neuroeng. 4:6. doi: 10.3389/fneng.2011.00006
Received: 24 December 2010; Paper pending published: 26 January 2011;
Accepted: 03 April 2011; Published online: 28 April 2011.
Edited by:
Guenter W. Gross, University of North Texas, USAReviewed by:
Arti Ahluwalia, University of Pisa, ItalyJohn M. Beggs, Indiana University, USA
Guillermo Peris Fajarnes, University of Valencia, Spain
Copyright: © 2011 Defranchi, Novellino, Whelan, Vogel, Ramirez, van Ravenzwaay and Landsiedel. This is an open-access article subject to a non-exclusive license between the authors and Frontiers Media SA, which permits use, distribution and reproduction in other forums, provided the original authors and source are credited and other Frontiers conditions are complied with.
*Correspondence: Antonio Novellino, ETT s.r.l., via Sestri 37, Genova 16154, Italy. e-mail:YW50b25pby5ub3ZlbGxpbm9AZXR0c29sdXRpb25zLmNvbQ==