- 1School of Medicine, Western Sydney University, Campbelltown, NSW, Australia
- 2The MARCS Institute, Western Sydney University, Campbelltown, NSW, Australia
Neuronal signalling is a key element in neuronal communication and is essential for the proper functioning of the CNS. Astrocytes, the most prominent glia in the brain play a key role in modulating neuronal signalling at the molecular, synaptic, cellular, and network levels. Over the past few decades, our knowledge about astrocytes and their functioning has evolved from considering them as merely a brain glue that provides structural support to neurons, to key communication elements. Astrocytes can regulate the activity of neurons by controlling the concentrations of ions and neurotransmitters in the extracellular milieu, as well as releasing chemicals and gliotransmitters that modulate neuronal activity. The aim of this review is to summarise the main processes through which astrocytes are modulating brain function. We will systematically distinguish between direct and indirect pathways in which astrocytes affect neuronal signalling at all levels. Lastly, we will summarize pathological conditions that arise once these signalling pathways are impaired focusing on neurodegeneration.
Introduction
Astrocytes are increasingly accepted as “Master-regulators” of brain function, mainly due to their ability to modulate various neuronal processes via direct and indirect pathways at the molecular, synaptic, cellular, and network levels (Walz, 2000; Pascual et al., 2005; Santello and Volterra, 2009; Chung et al., 2015; Anderson et al., 2016; Heithoff et al., 2021). Mounting evidence suggests that astrocytes directly modulate synaptic activity, including synaptic transmission, formation, and elimination as well as neuronal repair (Newman, 2003; Achour and Pascual, 2010; Chung et al., 2015; Burda et al., 2016). Moreover, astrocytes can affect neuronal activity indirectly via regulation of the transfer of metabolites through the blood-brain barrier, provision of metabolic support, and maintenance of ionic homeostasis in the extracellular environment (Simard and Nedergaard, 2004; Deitmer et al., 2019; Rose et al., 2020b), see also Table 1. In the below section, we have attempted to summarise the molecular signalling pathways underlying the astrocytic effect on neuronal signalling and function.
Astrocytic modulation of neuronal signalling at the molecular level
Ca2+ signalling
Ca2+ ions are one of the most important secondary messenger molecules in the brain. They play a key role in various signalling cascades and processes that account for both normal physiological functioning (Blaustein, 1985), as well as pathological conditions (Shigetomi et al., 2019). Although astrocytes are not capable of generating action potentials, they can communicate with coupled astrocytes and other neurons in their vicinity through transient elevations of intracellular Ca2+ concentration (termed Ca2+ oscillations or waves). Indeed these Ca2+ signals are referred to as the main readouts of astrocytic function (Bazargani and Attwell, 2016). Astrocytic Ca2+ signals affect the diffusion of ions across the astrocytic syncytium, and the release of chemical messengers (Newman, 2003; Parpura and Verkhratsky, 2012), thereby effecting both excitatory and inhibitory neurotransmission, basal synaptic activity, and synaptic plasticity directly or indirectly (Shigetomi et al., 2008; Panatier et al., 2011; Matos et al., 2018).
The role of astrocytic Ca2+ signalling in regulating neuronal physiology is a debatable topic within the scientific community (see (Bazargani and Attwell, 2016) for a detailed overview). On one hand, a group of studies advocated that a rise in astrocytic intracellular Ca2+ concentrations [Ca2+]i leads to an increase in neuronal [Ca2+]i indicating that synaptic activity is associated with and influenced by fluctuations in the astrocytic [Ca2+]i (Nedergaard, 1994; Parpura et al., 1994), while on the other hand, a group of studies argued that selective stimulation of astrocytic Ca2+ does not increase the neuronal Ca2+ levels thereby having no effect on the excitatory synaptic activity (Fiacco et al., 2007; Petravicz et al., 2008). Nonetheless, several studies have emphasized the role of astrocytic Ca2+ in regulating neuronal activity. In primary co-culture of neurons and astrocytes, it was discovered that spontaneous synchronous calcium oscillations (SSCO) in neurons can be suppressed by dopamine-induced Ca2+ signals in astrocytes and could be modified by stimulation of astrocytic Ca2+ rise leading to the release of GABA or adrenaline (Berezhnov et al., 2021). Astrocytic Ca2+ also increases basal synaptic transmission by activation of pre-synaptic α-2 adrenergic receptor (AA2 receptors) (Panatier et al., 2011) and is found necessary for intact functioning of the tripartite synapses in the hippocampus (Tanaka et al., 2013).
Other studies have pointed out the crucial role of astrocytic Ca2+ oscillations in mediating synaptic plasticity including LTP in the hippocampus. (Gómez-Gonzalo et al., 2015; Sherwood et al., 2017). Ca2+modulate astrocytic release of D-serine which activates NMDARs in the vicinity and thus contributing to the induction of hippocampal LTP (Henneberger et al., 2010) implying the involvement of astrocytes in learning and memory processes, which is contradictory to earlier studies (Petravicz et al., 2008; Agulhon et al., 2010). Moreover, Ca2+ signals were found to serve as a bridge in converting cholinergic activity into somatosensory plasticity which is associated with sensory functions (Takata et al., 2011) and provides evidence for astrocytic-neuronal interaction during sensory information processing (Lines et al., 2020). Furthermore, Lezmy and colleagues discovered that the astrocytic Ca2+ mediated ATP release into the extracellular milieu plays a key role in regulating the excitability and conduction velocity of myelinated cortical axons, suggesting that astrocytic [Ca2+]i directly controls the flow of information, neuronal signalling, and modulates action potentials (Sasaki et al., 2011; Lezmy et al., 2021). Recent studies suggested that astrocytic Ca2+ signalling is associated with regulating inhibitory synapses in a specific population of neurons wherein, elevated astrocytic Ca2+ leads to increased Somatostatin-expressing interneurons (SOM-INs) inhibition of pyramidal cells through the release of ATP (Lalo et al., 2014; Matos et al., 2018) or by endocytosis of the GABA transporter (GAT) from the plasma membrane of astrocytes (Zhang et al., 2017). Together, these studies suggest that astrocytic Ca2+ signalling is imperative in regulating neuronal activity, shaping the network function and thus forming a gateway for astrocytic-neuronal communication.
Na+ signalling
Intracellular Na+ [Na+]i transients are a fundamental property of both protoplasmic and fibroblastic astrocytes (Moshrefi-Ravasdjani et al., 2017) which represent a mechanism for fast and local signalling at the single perisynaptic level and determine the functional activity of astrocytes (Kirischuk et al., 2012). Na+ signals in astrocytes develop following the activation of excitatory synaptic transmission, which activates glutamate uptake into astrocytes and induces Na+ signals that propagate into the astrocytic syncytium via gap junctions (Langer et al., 2012; Langer et al., 2017). These signals are involved in a wide range of processes, including utilization of glutamate and lactate, K+ buffering, and transport of neurotransmitters (Voutsinos-Porche et al., 2003; Shimizu et al., 2007; Reyes et al., 2012; Hertz et al., 2015). Astrocytes express a plethora of Na+-permeable ion channels (iGluRs, ATPase’s- Na/K+ ATPase), exchangers and cotransporters (NKCC1; NCX; NHE; NBC, Nax) which are essential for developing ionic gradients required for Na+ signalling on the one hand, and maintain Na+ homeostasis on the other, reviewed by (Kirischuk et al., 2012; Rose and Karus, 2013). As most of the ionic channels that are expressed by astrocytes are also expressed by neurons, deciphering the selective impact of the different Na+ channels and transporters on astrocytic functioning is highly challenging.
The majority of the astrocytic Na+ transients affect neuronal signalling and function indirectly. One of the fundamental functions of astrocytic Na+ signals is to provide adequate metabolic support to neurons (neuro-metabolic coupling) for the transportation of neurotransmitters, ions, amino acids, and molecules across the membrane through the “lactate shuttle”, which is essential to form long-term memory (Bélanger et al., 2011; Langer et al., 2017; Descalzi et al., 2019). The level of various neurotransmitters such as glutamate, GABA, and glycine in the extracellular milieu is modulated by their respective transporters and enzymes whose activity is steered by astrocytic Na+ levels (Kirischuk et al., 2007; Héja et al., 2009; Unichenko et al., 2012), for details review see (Kirischuk et al., 2016). For example, the regulation of glutamate recycling from excitatory synapses is mediated by glutamine release from astrocytes, which is found to be modulated by elevated astrocytic [Na+]i via the Sodium-coupled neutral amino acid transporter 3 (SNAT-3) (Uwechue et al., 2012; Todd et al., 2017). Astrocytes also govern the GABAergic transmission and metabolism by direct uptake of GABA via the Na+ dependant GAT3 pathway and indirectly via glutamine synthesis to sustain in neuronal terminals, indicating GABA sensitivity to the astrocytic Na+ dependant supply of glutamine (Ortinski et al., 2010; Andersen et al., 2017; Andersen et al., 2020).
Recently, the reversal operation of the NCX exchanger in astrocytes has gained a lot of attention as it is capable of converting Na+ currents to Ca2+ signals in the astrocytic soma, perisynaptic cradle and thin astrocytic processes and thus, can potentially regulate the excitatory and inhibitory activity of neurons as discussed above (Brazhe et al., 2018; Verveyko et al., 2019; Wade et al., 2019; Rose et al., 2020a; Héja and Kardos, 2020). In particular, the activity of GAT-3 in hippocampal astrocytes leads to an increase in [Na+]i that translates to Ca2+ signals via NCX and triggers the release of ATP, which result in presynaptic inhibition of glutamate release in the adjacent neurons and heterosynaptic depression (Boddum et al., 2016). Moreover, NCX facilitates Ca2+-dependent glutamatergic gliotransmission at the tripartite synapse, directly affecting synaptic and neuronal activity (Reyes et al., 2012). Astrocytic Na+ transients also regulate the neuronal [Na+]i loads during hyper-synchronised activity by restricting Na+ discharge duration through glutamate and K+ uptake, hence aiding neurons to recover from Na+ loads that are induced during epileptic activity (Karus et al., 2015). Differences in the magnitude of astrocytic Na+ currents in the cortex and hippocampal regions have been observed, suggesting their diverse functional roles (Ziemens et al., 2019). Interestingly, axonal glutamate-evoked astrocytic Na+ currents were reported in the white matter, a brain region devoid of synapses and were spread to oligodendrocytes and NG2 glia oligodendrocytes, a process termed as ‘Panglial passage’ (Moshrefi-Ravasdjani et al., 2017). These astrocytic Na+ currents in the white matter can potentially translate to Ca2+ currents via reversal of NCX, thus speculating the indirect role of astrocytic Na+ currents in modulating neuronal signal conduction and propagation via translated Ca2+ currents (Dutta et al., 2018; Lezmy et al., 2021). Moreover, the fact that astrocytic glutamate uptake from the synaptic cleft is driven by Na+ currents indicates its paramount importance in defining glutamate excitotoxicity and homeostasis implicated in various neurodegenerative diseases (Li et al., 2021). In conclusion, astrocytic Na+ transients are important in regulating the neurotransmitter pool and affect the intracellular astrocytic Ca2+ levels thereby having indirect control over neuronal signalling, processing, and activity.
K+ signalling
Potassium ions are critical in determining neuronal activity as neurons are extremely sensitive to K+ ions (Kocsis et al., 1983). Following neuronal activity, local extracellular K+ concentration [K+]o increases, which leads to depolarization of both neurons (Baylor and Nicholls, 1969; Yarom et al., 1982) and glia (Orkand et al., 1966). Long-standing high [K+]o can affect the ability of neurons to fire action potentials, transmit synaptic signals, and re-uptake of neurotransmitters. Therefore, clearance of [K+]o from the extracellular milieu is of paramount importance for brain function. Most [K+]o clearance in the brain is facilitated by astrocytes via various mechanisms, including ‘K+ uptake’ via inwardly rectifying K+ (Kir) channels (Larsen and MacAulay, 2014), Na+/K+-ATPase (NKA) and Na+/K+/2Cl- (NKCC) cotransporters (Larsen and MacAulay, 2014; Sibille et al., 2015), and spatial buffering via astrocytic coupled gap junctions (Orkand et al., 1966; Ransom, 1996; Ma et al., 2016) which underscores the role of astrocytes in maintaining K+ homeostasis.
Astrocytic K+ signalling is involved in K+ and glutamate homeostasis (Djukic et al., 2007; Kucheryavykh et al., 2007), regulation of neuronal oscillations (Bellot-Saez et al., 2018), neural rhythms, hyperexcitability, synaptic plasticity, and locomotor behaviour (Bellot-Saez et al., 2017; Kelley et al., 2018; Barbay et al., 2023). Retrieval of K+ from the extracellular milieu is essential to prevent pathological accumulation of K+ in the extracellular space (Ransom, 1996; Bellot-Saez et al., 2017; Bellot-Saez et al., 2021), if not can result in depolarization of nearby neurons that affects their excitability profile (Do-Ha et al., 2018). By carrying out specific knock-out, pharmacological, and genetic inhibition of astrocytic Kir4.1 channels, Djukic and colleagues reported an increase in [K+]o, resulting in astrocyte’s inability to retrieve K+ and glutamate at the tripartite synapse, making the neurons vulnerable to hyperexcitable states (Djukic et al., 2007; Bay and Butt, 2012; Tong et al., 2014; Tyurikova et al., 2022) that is often observed in pathological conditions (Bellot-Saez et al., 2017). Moreover, increased [K+]o hampered glutamate uptake and led to a reduction in mEPSC frequency, indicating a negative feedback system which caused suppression of basal excitatory neurotransmission during the physiological state (Rimmele et al., 2017). By modelling the tripartite synapse and performing electrophysiological recordings, it was shown that Kir4.1 channels remarkably contribute to bringing the K+ and neuronal excitability to basal levels, particularly in response to repetitive stimulation and mainly modulates the neuronal theta rhythmic activity (Sibille et al., 2015). Moreover, Kir 4.1 conditional KO mice experience an increase in the LTP (Djukic et al., 2007), and a subsequent study provided evidence that astrocytic Kir4.1 channels suppress short-term synaptic plasticity responses specifically induced by prolonged repetitive stimulation and post-tetanic potentiation (PTP). (Sibille et al., 2014).Taken together, astrocytic K+ clearance is vital for shaping neuronal activity at both cellular and network levels as well as behaviour.
Glutamate signalling
Glutamate is one of the most abundant amino acids in the brain and owing to its property as an excitatory neurotransmitter, it is essential to restrict its availability within the synaptic cleft, as excess glutamate leads to excitotoxicity and neuronal death (Curtis and Johnston, 1974; Fonnum, 1984; Buskila et al., 2005; Dong et al., 2009). Approximately 80% of glutamate is retrieved from the synaptic cleft by astrocytes via glutamate transporters, namely, glutamate-aspartate transporter (GLAST), glutamate transporter-1 (GLT-1) (Rothstein et al., 1995; Rothstein et al., 1996), and excitatory amino acid transporters (EAATs) (Magi et al., 2019). Astrocytes actively act as scavengers of glutamate by increasing the surface diffusion of affinity glutamate transporters (Al Awabdh et al., 2016). The senescence of astrocytes is linked to cortical neuronal excitability due to heightened glutamate toxicity (Limbad et al., 2020). Computational studies suggest that any elevations in astrocytic glutamate concentrations can retain the glutamate in the synaptic cleft for longer periods and thus lead to an increased magnitude of slow inward currents (SICs), potentially resulting in hyperexcitability (Li et al., 2016a; Flanagan et al., 2018). Therefore, the activity of glutamate transporters in astrocytes is strictly regulated by transmembrane Na+ concentrations, that indirectly contribute to shaping synaptic transmission (Verkhratsky and Steinhäuser, 2000; Lalo et al., 2011). Particularly, Bergmann glial (BG) GLAST is indispensable for the excitatory synaptic wiring and wrapping of Purkinje cells in the cerebellar cortex (Miyazaki et al., 2017) and its reduced expression causes increased Purkinje cell firing, hyperactivity, and subsequent loss of Purkinje cells contributing to myotonic dystrophy and spinocerebellar ataxia type 1 (SCA1) (Cvetanovic, 2015; Sicot et al., 2017). The glutamate taken up is converted into glutamine-a precursor for glutamate and GABA synthesis by the action of the astrocytic enzyme glutamine synthetase (GS) and transported to neurons via Na+-coupled neutral amino acid transporters (SNATs) and their release is driven by astrocytic intracellular Ca2+ concentration. This conversion is vital to avert glutamate spill over events and unnecessary peri/extrasynaptic NMDAR-excitatory postsynaptic currents in pyramidal cells (Trabelsi et al., 2017). The glutamate uptake kinetics in astrocytes varies significantly from neonatal to adult and exhibits regional heterogeneity, explaining the possible specialized functions of astrocytes that are circuit-specific (Hanson et al., 2015; Romanos et al., 2019). This astrocytic glutamate-neuronal signalling accounts for synaptic plasticity, contributes to the synchronous activity in neuronal domains and modulates the cortical UP states by tuning the spatiotemporal levels of glutamate in the extracellular space (Fellin et al., 2004; Carmignoto and Fellin, 2006; Bonansco et al., 2011; Poskanzer and Yuste, 2011). Interestingly, astrocytic glutamate signalling also contributes to synaptic plasticity and learning and memory processes by glutamate uptake during early and late LTP (Pita-Almenar et al., 2012), as well as release of glutamate to induce NMDAR mediated LTP (Han et al., 2013; Gómez-Gonzalo et al., 2015; Park et al., 2015). It further contributes to the developmental transition from spike timing-dependent long-term depression (t-LTD) to t-LTP in the CA3-CA1 synapses of the postnatal hippocampus, thus highlighting the importance of astrocytic glutamate signalling in regulating neuronal activity from postnatal to mature stages of development (Falcón-Moya et al., 2020).
GABA signalling
Astrocytes express a plethora of GABA receptors and transporters, including the ionotropic GABAA and metabotropic GABAB receptors (Fraser et al., 1994; Oka et al., 2006), and the GAT-1 and GAT-3 transporters, which facilitate GABA uptake from the synaptic cleft as part of the Glutamate/GABA-glutamine cycle (Ribak et al., 1996; Yan and Ribak, 1998; Boisvert et al., 2018; Ghirardini et al., 2018). Astrocytes are also capable of releasing a considerable amount of GABA (Lee et al., 2010b; Le Meur et al., 2012) and regulates its concentration in the extrasynaptic space (Schousboe et al., 1977). Similar to glutamate, GABA evokes Ca2+ oscillations in astrocytes (Meier et al., 2008) which are mainly mediated by either GABA receptors or GAT transporters. These oscillations lead to the release of GABA, glutamate, and ATP which can regulate and modulate local synaptic activity in several ways, as discussed below (Le Meur et al., 2012; Oh et al., 2012; Shlosberg et al., 2012; Boddum et al., 2016; Mariotti et al., 2016).
Recent studies indicated that GABA signalling in astrocytes can affect both the excitatory and inhibitory activity of neurons emphasising the dual role astrocytes play in network activity. Indeed, Mariotti and colleagues, recently showed that GABA-activated astrocytes release glutamate, which in turn triggered slow inward currents (SICs) and firing of nearby pyramidal neurons (Mariotti et al., 2016). Moreover, astrocytic GABA signalling was found to be involved in the homeostatic regulation of excitatory transmission by activation of astrocytic GAT-3, which on the one hand triggers Ca2+ mediated release of ATP that hinders presynaptic glutamate release, and on the other hand is required for heterosynaptic depression (hLTD) that is usually accompanied during LTP (Chen et al., 2013; Boddum et al., 2016).
GABA-activated astrocytes are also involved in regulating the inhibitory activity of neurons, as activation of somatostatin-expressing interneurons (SOM-INs) can trigger astrocytic GABAB receptor and GATs activity to induce synaptic depression in pyramidal cells (Shlosberg et al., 2003; Shlosberg et al., 2012; Matos et al., 2018; Shen et al., 2022). It is important to note that astrocytes can also synthesise GABA through various enzymes and pathways, namely, GABA synthetase or glutamic acid decarboxylase (GAD67 or GAD65) (Chattopadhyaya et al., 2007; Walls et al., 2010), monoamine oxidase B (MAOB) involving putrescine (Yoon et al., 2014), and diamine oxidase (DAO) (Kim et al., 2015). The direct effect of astrocytic GABA on neurons was reported by Yoon et al., where they showed that the amount of GABA released by astrocytic Best1 channels directly correlates to the magnitude of tonic inhibition in several brain areas including the cerebellum, thalamus, and dentate gyrus (Yoon et al., 2011). Nevertheless, it is obscure whether astrocytic synthesis of GABA alone is enough for its release and exerts a direct effect on neurons. To evaluate this, Lee and colleagues generated astrocyte-specific MAOB conditional knockout mice and found a decrease in MAOB and astrocytic GABA levels in the cerebellum and striatum which contributed to a 74%–76% reduction of tonic GABA currents in neurons, confirming that astrocytic source of GABA contributes to tonic GABA currents (Yoon et al., 2014; Lee et al., 2022c). Additionally, astrocytes of the dorsal horn also release GABA in response to glutamate suggesting their role in sensory information processing (Christensen et al., 2018). Moreover, astrocytic tonic GABA inhibition of lemniscal synapses in the thalamus can increase temporal fidelity and thus improve tactile discrimination (Kwak et al., 2020). Astrocytes also maintain the extracellular GABA concentration and are accountable for the modulation of both tonic and phasic GABA currents (Lalo et al., 2014).
Lastly, it is important to mention that GABA-activated and GABA-releasing astrocytes are capable of shifting the inhibitory signals to excitatory signals and vice versa through diverse mechanisms. For instance, Heja and colleagues described one of the mechanisms wherein, upon the intense excitatory activity of the neurons, astrocytes uptake glutamate which leads to the synthesis of GABA from polyamine putrescine and releases GABA via reverse operation of GAT-1/3 that result in neuronal tonic inhibition (Héja et al., 2012). In contrast, by using GABAB receptor conditional knockout mice (GB1-cKO mice) specifically in astrocytes, Perea and colleagues reported that Ca2+ events initiated by astrocytic GABAB receptors contribute to interneuron induced synaptic potentiation via activation of mGluRs (Perea et al., 2016). A plausible explanation for this dual role of astrocytes is that astrocytic response is usually specific to neuronal inputs. For example, the intensity of interneuron firing decides the type of gliotrasmitter released by astrocytes (glutamate, ATP, GABA) that acts at the pre-synapse either resulting in potentiating or inhibiting the neurotransmitter release. This clearly demonstrates the differential control of synaptic transmission by astrocytes (Covelo and Araque, 2018). In summary, astrocytic GABA signalling regulates the excitatory and inhibitory activity of neurons and controls information processing via modulation of local network activity.
Astrocytic regulation of neuronal signals at the synaptic and cellular levels
The previous sections emphasised the astrocytic influence on neuronal function at a molecular level. However, mounting evidence suggests that astrocytes can also affect neuronal signalling at synaptic and cellular levels, modulating synapse formation, function and communication with other neurons and glia (Figure 1).
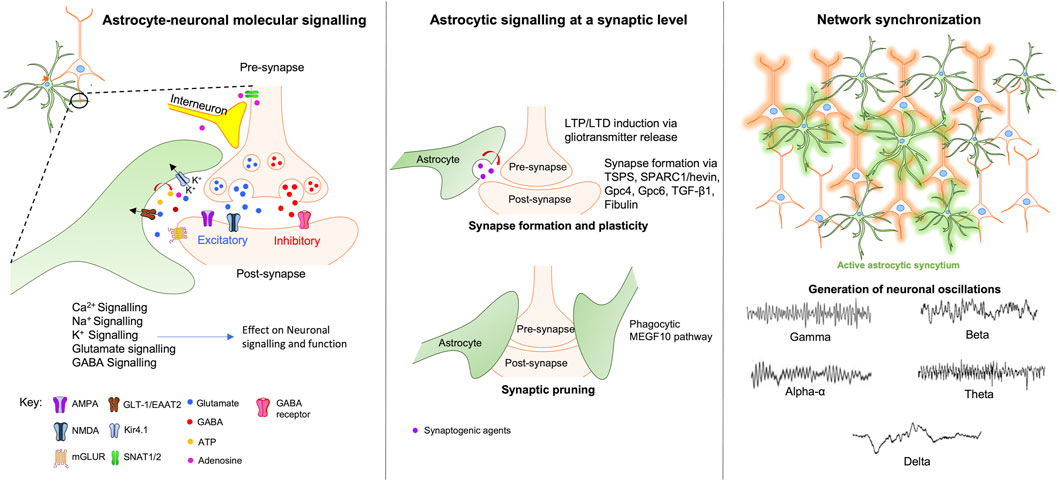
FIGURE 1. Astrocytic modulation of neuronal signalling at the molecular, synaptic and network levels. At the molecular level (left window) astrocytes regulate and modulate neuronal activity via various direct and indirect mechanisms involving Ca2+, Na+, K+, Glutamate, and GABA signalling pathways. At the synaptic level (middle window), astrocytes maintain synaptic integrity by modulating synaptic plasticity, formation, and pruning through the release of neurotransmitters, gliotransmitters and synaptogenic agents. Right window - neuronal network coordination and synchronization require the activation of astrocytic syncytium which leads to the generation of neuronal oscillations that forms the basis of various complex behaviours ranging from sleep-awake states to complex higher-order cognitive functions. Abbreviations: TSPS-Thrombospondins; SPARC1-secreted protein acidic enriched in cysteine like-1; Gpc4-glypicans 4; Gpc6-glypicans 6; TGF-β1-transforming growth factor-beta; MEGF10-Multiple EGF-like-domains 10.
Synapse formation, pruning and refinement
Since the conceptualization of the “Tripartite Synpase” (Araque et al., 1999) the role of astrocytic function has evolved from being mere neuroglial cells that nourish the neurons to a key player in the formation, elimination, integration, and stabilization of synapses (Chung et al., 2015). Indeed, multiple studies have shed light on the essential role of astrocytes during neuronal differentiation, formation, and maturation of synapses (Klapper et al., 2019; Van Horn and Ruthazer, 2019). Astrocytes do so via various mechanisms, including the secretion of synaptogenetic and neurotrophic factors (Baldwin and Eroglu, 2017), dynamic changes in their morphology (Lawal et al., 2022), and through uptake and release of neurotransmitters (Buskila and Amitai, 2010).
Neuronal circuits are constant shapeshifters, mainly due to synaptic plasticity processes that strengthen or weaken synapses according to one’s environmental experience. A Plethora of factors are involved in the formation and pruning of synapses. Astrocytes promote the formation and function of both excitatory (Eroglu et al., 2009) and inhibitory (Elmariah et al., 2005) synapses via the secretion of molecules that target both the pre and post-synaptic sites. Thrombospondins (TSPs) (Christopherson et al., 2005), hevin (Kucukdereli et al., 2011; Risher et al., 2014), and transforming growth factor-beta 1 (TGF-β1) (Diniz et al., 2014) are some of the major molecules secreted by astrocytes that are involved in the formation of synapses. It is interesting to note that the factors secreted by astrocytes and aid in synaptogenesis are pathway and neuron-specific and thus determine whether they become silent or active synapses. For example, thrombospondins have been associated with establishing silent glutamatergic synapses (Christopherson et al., 2005), while hevin found to be involved in synaptic refinement, particularly in thalamocortical synapses (Risher et al., 2014), and TGF-β1 found to be associated with the formation of both excitatory and inhibitory synapses (Diniz et al., 2012; Diniz et al., 2014). Complementary to this, glypicans 4 and 6 (Gpc4 and Gpc6) secreted by astrocytes found to be involved in the formation of active functional synapses (Allen et al., 2012; Farhy-Tselnicker et al., 2017). Moreover, a recent report indicated that astrocyte-derived small extracellular vesicles (SEVs) contain a synaptogenic cargo called Fibulin-2 which contributed to cortical dendritic spine and synapse formation in primary cortical neuronal cultures (Patel and Weaver, 2021). Subsequently, astrocytic lipid metabolism and mitochondrial biosynthesis are also found to play a critical role in synapse formation and maturation. The astrocytic lipid metabolism involves Sterol regulatory element binding proteins (SREBPs) whose activity is dependent on sterol sensor SREBP cleavage-activating protein (SCAP). Selective inactivation of astrocytic SCAP-SREBP-mediated lipid biogenesis led to impaired function of the pre-synaptic terminal, and short and long-term plasticity in the SCAP mutant mice (van Deijk et al., 2017). Likewise, astrocytic Fatty acid binding protein 7 (FABP7) is essential for normal dendritic morphology and miniature excitatory postsynaptic currents (mEPSCs) indicating the implication of astrocytes in regulating the excitatory synaptic function (Ebrahimi et al., 2016). The astrocytic mitochondrial biosynthesis is reliant on the metabolic regulator peroxisome proliferator-activated receptor gamma (PPARγ) co-activator 1α (PGC-1α) whose activity is governed by mGluR5. Conditional genetic ablation of PGC-1α led to abberant astrocytic proliferation and maturation, resulting in disrupting synaptogenesis and the excitatory synapse formation (Zehnder et al., 2021).
Apart from contributing to the formation of synapses, astrocytes also regulate the functions of synapses and take part in the process of synaptic pruning (Papouin et al., 2017; Luo and Gao, 2021). A recent study revealed that astrocytes phagocytose excitatory synapses in the hippocampus via the Multiple EGF-like-domains 10 (MEGF10) pathway and thus are accountable for replenishing memory traces via synapse elimination, which is essential for circuit homeostasis and synaptic connectivity (Lee et al., 2021). In parallel, the same astrocytic MEGF10 phagocytic receptor is responsible for the removal of thalamocortical synapses associated with ocular dominance plasticity (ODP), thus determining the synaptic plasticity that is experience-dependent (Lee et al., 2022b).
Contrary to the popular notion that the formation of synapses is involved in learning and memory, a study by Morizawa et al suggests that engulfment of the synapses by cerebellar Bergmann glia might result in enhanced motor learning and circuit refinement (Morizawa et al., 2022). Essentially astrocytes also produce extracellular matrix proteins and cell adhesion molecules that facilitate the integration of astrocytic processes to synapses to perform their respective functions (Hillen et al., 2018). On the whole, astrocytes are an integral part of the synapse and a key player regulating its functionality through various processes.
Astrocytic regulation of neuronal signals at the network and behavioural levels
Astrocytes influence neural synchrony, network oscillations, and behaviour
Neuronal synchronization and oscillations form the basis of several behaviours such as motor skills, (Davis et al., 2012), sleep-wake cycles (Adamantidis et al., 2019), and cognition (Kucewicz et al., 2014; Buskila et al., 2019a). In this section, we will highlight the importance of the astrocytic syncytium in maintaining neuronal synchronous activity that leads to the generation of synchronized oscillatory brain rhythms that underlie such behaviours.
Astrocytic-mediated neuronal synchrony
Neuronal synchronization occurs when two or more events associated with diverse aspects of neuronal activity appear at the same time. This mainly arises due to the dynamic interplay between neurons within a network and there are several mechanisms which explain how a population of neurons get synchronized (Timofeev et al., 2012). It is suggested that astrocytic Ca2+ signalling corresponds to the level of neuronal synchrony in neighbouring neurons (Sasaki et al., 2014), implying that astrocytic [Ca2+]i orchestras and maintains a collective of neuronal dynamics. Indeed, an in vivo study found that astrocytic Ca2+ regulates cortical state switching by actively regulating the extracellular glutamate levels, consequently accounting to slow neuronal rhythm thereby controlling the neural circuit states (Poskanzer and Yuste, 2016). Additionally, it has been reported that the absence of astrocytes in neuronal cultures results in desynchronized glutamate transmission that leads to perturbations in the action potential waveform and propagation (Sobieski et al., 2015).
Astrocytes facilitate various behaviours via neuronal synchrony. For example, synchrony of the neurons in the anterior cingulate cortex (ACC), a region responsible for visceral-pain-cognitive interactions is due to the astrocytic release of L-lactate, resulting in improved decision-making (Wang et al., 2017). In addition, the circadian synchrony of the suprachiasmatic nucleus (SCN) is maintained via astrocytic control of extracellular glutamate level, which is essential for normal molecular timekeeping (Brancaccio et al., 2017). Moreover, Sardinha and colleagues reported desynchronised theta oscillations between the dorsal hippocampus and the prefrontal cortex in a dominant negative SNARE (dnSNARE) mouse model with impaired exocytosis in astrocytes. They also observed reduced cognitive performances which were restored upon supplementation with D-serine (Sardinha et al., 2017), corroborating astrocytes are capable of modulating far neuronal networks, which elucidates their impact on remote synchronization activity. Furthermore, astrocytic synchronisation is preceded by neuronal synchronization, pointing to the involvement of neurons in Slow wave activity (SWA) that is associated with quite wakefulness and sleep states and emphasises that astrocytes are implicated in the generation of SWA and regulates the switching between sleep and wakefulness states, suggesting their role in sleep-wake cycle and memory consolidation (Szabó et al., 2017; Bojarskaite et al., 2020). Astrocytes also generate rhythms in the SCN and controls their period bidirectionally although to a lesser extent than neurons. Despite this, their activation does not affect the phase of the SCN in any way (Patton et al., 2022).
The regulation of neuronal synchrony by astrocytes is additionally underpinned by several neuronal-astrocytic computational models. By employing different astrocyte-neuronal simulation models, Amiri et al showed that astrocytes can potentially modulate the synchronous and asynchronous states of neurons by adjusting the threshold value of transition (Amiri et al., 2013). More recently, a simulation model revealed that the degree of neuronal output synchronisation increases with neuron-astrocytes interactions (Pankratova et al., 2019). Interestingly, a study employed neuronal cultures grown on a multielectrode array to explore the mechanism and origin of synchronised burst (SB) activity along with the application of computational modelling and has implicated the role of astrocytes in the generation reverberating activities in an SB (Huang et al., 2017). Similarly, astrocytic Ca2+ is predicted to be involved in the synchronised activity of large-scale neuronal ensembles (Li et al., 2016b; Polykretis et al., 2018) and can also cause intermittent neuron synchrony via slow astrocytic Ca2+ oscillations (Makovkin et al., 2020). In summary, both experimental and simulation studies suggest that astrocytes play a vital role in neuronal synchronization.
Astrocytic modulation of neuronal oscillations and behaviour
In a given network of neurons, the synchronised activity of individual neurons leads to rhythmic variations in membrane potential, which contributes to the generation of neuronal oscillations and brain waves. These neuronal oscillations have a characteristic power and frequency band and are categorised with distinct frequency bands that are linked with particular behaviours (Buzsáki and Draguhn, 2004). Several mechanisms underlie neuronal oscillations, which include astrocytic Ca2+ activity (Poskanzer and Yuste, 2011; Poskanzer and Yuste, 2016), uptake and homeostasis of glutamate and potassium ions (Li et al., 2016a; Bellot-Saez et al., 2018), astrocytic coupling, and release of gliotransmitters (Pirttimaki et al., 2017).
Neuronal gamma oscillations (30–80 Hz) are associated with learning, memory and cognitive functions (Thompson et al., 2021; Liu et al., 2022) and astrocytes have been implicated in their generation and modulation. S100B, a calcium-binding protein expressed explicitly in astrocytes, is secreted to the extracellular space and contributes to the enhanced amplitude of gamma oscillations in the hippocampus (Sakatani et al., 2008). Moreover, a recent study showed that infusion of S100β into astrocytes and activation of astrocytes using Designer Receptors Exclusively Activated by Designer Drugs (DREADD) in the medial pre-frontal complex (mPFC) of rats, contributed to a rise in phase-amplitude coupling between theta and gamma oscillations and led to improved performance in the attentional set-shifting task (ASST), indicating the astrocytic role in the enhancement of cognitive flexibility (Brockett et al., 2018). Additionally, astrocytes are also known to affect goal-directed behaviours and cortical information processing, as demonstrated through genetic ablation of astrocytic GABAB receptors in the mPFC, which caused a reduction in the power of low-gamma oscillations, and poor performance in goal-directed behaviour test (Mederos et al., 2021). Furthermore, lee and colleagues created a triple transgenic mouse where the astrocytic vesicular release can be reversibly controlled by the expression of tetanus neurotoxin (TeNT) in astrocytes. They observed blockade in glutamate release, shortened gamma oscillatory activity, reduction in EEG power, and impaired behaviour in novel object recognition test in these mice, indicating that astrocytes are involved in the maintenance of gamma oscillatory activity and are required for recognition memory (Lee et al., 2014).
Uptake and release of glutamate is dependent on [K]+o which is closely regulated by astrocytes, indicating its indirect control over neuronal oscillations (Sarantis and Attwell, 1990; Longuemare et al., 1999; Djukic et al., 2007). In line with this, in vitro experiments in the somatosensory cortex revealed that occluding K+ uptake via Kir4.1 channels or blockade of astrocytic gap junction connectivity impairs K+ clearance by astrocytes and thus contributes to increased excitability of the neuronal network, leading to changes in the oscillatory behaviour of individual neurons and an increase of the network oscillatory power, thereby unravelling a novel mechanism to fine-tune neuronal network oscillations (Bellot-Saez et al., 2018). Subsequently, a recent study investigated the effect of astrocytic decoupling on network activity by generating a double KO of connexin 30 (Cx30) and connexin 43 (Cx43), where they reported a reduction in excitability in CA1 pyramidal neurons, reduced LTP, disruption of D-serine homeostasis which led to pronounced spatial memory and learning impairment (Hösli et al., 2022). Additionally, Kelley et al demonstrated that astrocytic Kir 4.1 channels in the spinal cord are essential to induce and maintain muscle peak strength by fast alpha motor neurons, indicating that astrocytes impact the electrophysiological properties of alpha motor neurons and overall locomotor activity (Kelley et al., 2018). Moreover, dysfunctional astrocytic Kir4.1 channels in the spinal central pattern generator led to perturbed locomotor patterns and neuronal rhythmogenesis (Barbay et al., 2023) and it has been suggested that elevated cortical [K+]o can impact sensory and motor processing by altering neural activity, pointing to an astrocytic role in steering such behaviours (Rasmussen et al., 2019). Another potential mechanism in which astrocytes modulate neuronal oscillations has been suggested by (Shibasaki et al., 2014), who showed that transient receptor potential vanilloid 4 positive (TRPV+) astrocytes can release gliotransmitters, namely, glutamate and ATP, which regulates the excitability of neurons. In the recent decade, much focus has been laid down on the astrocytic regulation of various behavioural paradigms, including higher-order cognitive functions such as learning and information processing (Santello et al., 2019), neuropathic and chronic pain (Li et al., 2019), motor skills (Padmashri et al., 2015), anxiety (Abu-Ghanem et al., 2008; Shim et al., 2019) and sleep (Ingiosi and Frank, 2022).
Astrocytes are involved in both short-term and long-term memory formation. For instance, mice performance was found to be reduced in spontaneous alternation Y-maze and novel object replacement task when astrocytic Gq-GPCRs were specifically blocked, indicating an impaired working and short-term spatial memory (Nagai et al., 2021). Moreover, recent fear memory, a form of long-term memory was found to be impaired when muscarinic acetylcholine receptor M1 (m1-AChRs) was specifically deleted in astrocytes from the dentate gyrus (Li et al., 2022). It is interesting to note that astrocytic metabolism is required for learning activities. A study by Descalzi et al reported that astrocytic lactate is critical for de novo mRNA translation that is induced due to learning in both excitatory and inhibitory neurons and the same was confirmed by 3D electron microscopy studies suggesting astrocytic L-lactate serves as an energy store for synaptic plasticity (Descalzi et al., 2019; Vezzoli et al., 2020). Additionally, astrocytes are involved in regulating the reward system in the brain, where they can successfully encode the location of the reward in a spatial context only in a familiar environment indicating their indirect involvement in higher cognitive functions (Doron et al., 2022). The Central nucleus of amygdala (CeA) is a region that regulates fearful and stressful responses. Studies from Knockdown of astrocytic glucocorticoid receptors (GR) in mice specifically in CeA region showed that astrocytic GR is significantly involved in consolidation of aversive memory and few anxiety-related behaviours (Tertil et al., 2018; Wiktorowska et al., 2021).
Astrocytes are also capable of reversing chronic pain and modulating motor behaviour. Indeed, allodynia-like behaviour was reversed by astrocytic initiation of spine plasticity that eliminated those synapses formed shortly after partial sciatic nerve ligation, thus affecting the circuitry implementing this information (Takeda et al., 2022). Moreover, astrocytic calcium signalling was found to be imperative for motor skill learning (Padmashri et al., 2015) and for closure of the motor circuit critical period by invading the neuropil and affecting spine dynamics, dendritic length, and synaptic inputs (Ackerman et al., 2021).
Astrocytes regulate sleep via Ca2+ activity and by release of adenosine which affects the sleep-wake states and sleep deprivation states (Halassa et al., 2009; Florian et al., 2011). In particular, Rapid-eye movement (REM) sleep and its associated theta rhythm is modulated by astrocytic IP3/Ca2+ signalling (Foley et al., 2017). Indeed, the amount of sleep corresponds to the changes in astrocytic Ca2+ activity and reduced intracellular Ca2+ levels hamper homeostatic sleep response post-sleep deprivation (Ingiosi et al., 2020). Moreover, Vaidyanathan and colleagues observed that the duration and depth of non-rapid eye movement (NREM) sleep is determined by astrocytic G-protein coupled receptor signalling pathways, namely, Gi-GPCR (sleep depth) and GqGPCR (sleep duration) elucidating the contribution of network cortical astrocytes in modulating the sleep length and quality (Vaidyanathan et al., 2021). Overall, astrocytes play a critical role in regulating several behavioural attributes, which makes it extremely important to study them in greater depth.
Astrocytic modulation of neurodegeneration
Glial cells play a key role in the mechanisms which dispose the aged brain to neurodegeneration, especially as ion homeostasis is a function that is primarily carried out by glial cells. There is a growing number of reports pointing to the perturbations in astrocytic function as a risk factor, causation, and driving factor for neurodegenerative diseases such as Alzheimer’s disease (AD), Parkinson’s Disease (PD), Huntington’s disease (HD), and Amyotrophic lateral sclerosis (ALS), all of which are associated with the loss of a specific type of neurons confined to a particular region (Stevenson et al., 2020). In this section, we will be summarising the latest studies which uncover novel astrocytic mechanisms that contribute to neurodegeneration and how targeting them can be beneficial in improving disease progression and treatment.
Alzheimer’s disease (AD)
AD is one of the most common types of dementia and is characterised by the accumulation of extracellular ß-Amyloid (Aβ) plaques, intracellular neurofibrillary tangles consisting of hyperphosphorylated tau, neuronal loss, and high levels of reactive astrocytes (Ferri et al., 2005; Simpson et al., 2010; Buskila et al., 2013; Morley et al., 2018). Neurons are considered a primary repository of Aβ and apolipoprotein E4 (apoE4), a cholesterol transport protein that is considered one of the greatest risk factors for sporadic AD (Corder et al., 1993). However, a recent study has linked apoE, Aβ, and plaque formation suggesting that the production of beta-amyloid in neurons is strictly regulated by astrocytic cholesterol signalling, where apoE transports neuronal amyloid precursor protein (APP) across neuronal cell membranes using astrocyte-derived cholesterol, indicating that astrocytes are an indirect risk factor along with neuronal dysfunction (Wang et al., 2021).
The Aβ plaques are surrounded by reactive astrocytes which can either aggravate or ameliorate the disease progression. A recent study revealed that reactive astrocytes are present in the vicinity of Aβ plaques and tend to engulf, internalise, and degrade axonal dystrophic neurites associated with these plaques in both AD mouse models and patient samples (Gomez-Arboledas et al., 2018). This phagocytic nature of astrocytes indicates their involvement in clearing damaged neuronal circuits or even reducing the neuroinflammatory impact to limit the pathology of AD. Recently, Lee et al identified a specific population of astrocytes called autophagy-dysregulated astrocytes (APDAs) that have lost their ability to secrete synaptogenic factors and thus synapse elimination in AD and aged brain, which might be implicated in disease pathology (Lee et al., 2022a). Moreover, genetic ablation of proliferating reactive astrocytes in double APP23/GFAP-TK mice led to an increased aggregation of monomeric Aβ, which was accompanied by a reduction in synaptic and neuronal density, upregulation of pro-inflammatory markers such as TNFα, NFκB, nitric oxide synthase-1 (NOS1), and memory loss (Katsouri et al., 2020). In contrast, severe reactive astrocytes were accountable for exacerbating AD progression via H2O2 production and nitrosative stress contributing to neurodegeneration. It is interesting to note that only severe reactive astrocytic phenotype contributed to neurodegeneration whereas mild reactive astrocytic phenotype was reversible (Chun et al., 2020). In line with this study, a recent report used transcriptomic methods to show that astrocytes are equally capable of acquiring both neuroprotective as well as harmful states in Aβ and tau pathologies (Jiwaji et al., 2022). Indeed, modulation of astrocytic reactivity via the JAK2-STAT3 pathway led to reduced amyloid load and improved spatial learning (Ceyzériat et al., 2018), while specific deletion of STAT3 in the APP/PS1 mice model of AD alleviated AD symptoms such as spatial learning and memory impairments, indicating that targeting reactive astrocytes holds therapeutic benefits (Assefa et al., 2018; Reichenbach et al., 2019). Further evidence for dysregulated astrocytic Ca2+ (Brawek and Garaschuk, 2014) and glutamate homeostasis in the AD brain is reported as leading to hyperexcitability and neuronal death, which can be correlated to reduced GLT-1 expression and significant cognitive deficits (Brymer et al., 2023). In addition, a recent study reported regional differences in the astrocytic glutamine-glutamate cycle and impairment of synaptic mitochondrial functions in the early stages of AD which might play a key role in disease pathogenesis and progression (Andersen et al., 2021).
Parkinson’s disease (PD)
The second most common neurodegenerative disease across the globe is PD (De Lau and Breteler, 2006). Its symptoms include tremors, rigidity, bradykinesia and other non-motor symptoms (Sveinbjornsdottir, 2016). The specific loss of dopaminergic (DA) neurons in the substantia nigra pars compacta (SNpc) and aggregation of insoluble, misfolded a-synuclein protein is central to the disease pathology (Hurtig et al., 2000; Kordower et al., 2013), however recent studies showed that atrophy of astrocytes, as well as astrocytic dysfunction, play a significant role in disease development (Ramos-Gonzalez et al., 2021).
During disease progression, astrocytes transform from neuroprotective to neurotoxic signatures which exacerbate the disease. The misfolded a-synuclein released by neurons is taken up by astrocytes via endocytosis and triggers a pro-inflammatory response (Lee et al., 2010a; Rannikko et al., 2015), including the secretion of pro-inflammatory and neuroinhibitory factors (Kekesi et al., 2019). Astrocytic response to a-synuclein is correlated to elevated levels of the mammalian homologue of UNC-18 (Munc18) -a protein essential for vesicle exocytosis, accompanied by reactive astrocytic morphology and increased expression of IL-6 (Di Marco Vieira et al., 2020). Indeed, Cavaliere and colleagues demonstrated that Lewy body fractions containing human α-synuclein are taken up more efficiently by astrocytes rather than neurons and induces high expression of endogenous α-syn while the transport of α-synuclein takes place bi-directionally between both cell types and causes astrogliosis (Cavaliere et al., 2017). Moreover, some of the key symptoms widely expressed in PD brains, including impaired glutamate homeostasis and signalling, and neuronal hyperexcitability (Ferrarese et al., 2001; Iovino et al., 2020; Campanelli et al., 2022) have been observed in astrocytic-specific knockdown of glutamate transporter-1 (GLT-1) in the striatum and SNpc (Zhang et al., 2020; Ren et al., 2022). A recent seminal study indicated that in PD mice, α-synuclein promotes astrocytes to produce excess glutamate, which causes synaptic loss via increased tonic glutamatergic activation of extrasynaptic NMDARs, implying the direct role astrocytes play in dysregulated glutamate signalling in PD (Trudler et al., 2021). This finding is further supported by a recent study indicating dysregulation of astrocytic Ca2+ signalling and gliotransmitter release in PD mice that result in altered synaptic function (Nanclares et al., 2023).
During the initial stages of disease pathology, astrocytes play a neuroprotective role by facilitating phagocytosis, maintaining proteostasis, and reducing extracellular inflammatory responses (Morales et al., 2017; Yang et al., 2022). Indeed, a recent report identified a subpopulation of astrocytes positive for Vitamin D activating enzyme which might be neuroprotective and beneficial in mitigating disease pathology (Mazzetti et al., 2022). However, scientists have made efforts to comprehend and identify the intricate mechanisms by which astrocytes transform into pathological signatures. Some of the astrocytic characteristics, including impaired chaperone-mediated autophagy, macroautophagy (di Domenico et al., 2019), disruption of Ca2+ signalling, reactive phenotypes, altered metabolic functions such as reduced glycolysis (Sonninen et al., 2020), pro-inflammatory responses and regional heterogeneity (Kostuk et al., 2019; Basurco et al., 2023) are considered as pathological switching traits of astrocytes in disease causation and progression and therefore targeting these pathways hold great therapeutic opportunity.
Huntington’s disease (HD)
HD is an inherited neurodegenerative disorder with hallmarks such as progressive motor, cognitive and psychiatric dysfunction (Paulsen et al., 2001; Tabrizi et al., 2013) caused by an increased polyglutamine (PolyQ)-encoding CAG repeat (>36) in exon 1 of the huntingtin gene (HTT) (MacDonald et al., 1993). Both cortico-striatal and thalamo-cortical neural circuits are significantly affected in HD (Eidelberg and Surmeier, 2011). Rodent studies suggest that the accumulation of the mutant huntingtin protein (mHTT) is a direct contributor to HD disease pathology (Bradford et al., 2009). A recent study indicated that expression of mHTT specifically in astrocytes can worsen the disease progression, but still requires mHTT expression in neuronal cells to induce neurodegeneration (Jing et al., 2021). Additionally, engrafting mHTT expressing human glial progenitor cells into healthy mice elicited characteristics of HD (Benraiss et al., 2016). Conversely, reducing the accumulation of mHTT in astrocytes improved motor functions, decreased neuropsychiatric features and restored NMDA receptor function of striatal medium spiny neurons (MSNs) in the BACHD mice model of HD, therefore, highlighting the critical role of astrocytes in HD pathology (Wood et al., 2019).
Several astrocytic dysfunctions are associated with HD pathogenesis, such as low expression of glutamate transporters (Faideau et al., 2010), increased synthesis and release of astrocytic glutamate (Lee et al., 2013), and reduced extracellular glutamate uptake rate in the cortex and striatum (Lievens et al., 2001; Shin et al., 2005). Recently, reduced expression of astrocytic Kir 4.1 channels was reported in striatal astrocytes, causing elevated levels of extracellular K+ ions and thereby increasing the excitability of MSNs (Tong et al., 2014). Moreover, glutamate transporter GLT-1 activity and its impact on synaptic currents appears to be dependent on Kir4.1 conductivity, which is perturbed in the HD mice model (Dvorzhak et al., 2016). However, these phenotypes, including the aberrant K+ ion levels, MSNs excitability profile and motor deficits were restored via viral delivery of Kir4.1 channels, emphasising the critical role of K+ homeostasis in HD (Tong et al., 2014). In line with this study, Diaz- Castro and colleagues deciphered the link between astrocytic Ca2+ signalling, GLT-1, and Kir4.1 in HD mice model and found that loss in homeostatic functions of GLT-1 and Kir4.1 led to aberrant glutamate and Ca2+ signalling altering striatal MSNs (Jiang et al., 2016). In addition, mHTT astrocytes exhibit reduced cholesterol synthesis which does not support proper synaptic and neuronal functioning (Valenza et al., 2010; Valenza et al., 2015). Moreover, altered brain energy metabolism such as reduced glucose uptake is also evident in HD, indicating that astrocytic-neuronal cross-talk can aid in the early detection of the disease (Boussicault et al., 2014). Supporting proteomics studies revealed compromised astrocytic metabolism, and impaired glutamate/GABA-glutamine cycle causing disruptions in the synthesis and release of glutamine and GABA in HD. Subsequently, Garcia and colleagues investigated the electrophysiological properties of astrocytes derived from Huntington patients’ iPSCs. They reported longer astrocytic spontaneous Ca2+ signals, impaired K+ inward rectifying currents, lower cell membrane capacitance and the inability of astrocytes to shield neurons from glutamate excitotoxicity, indicating that HD astrocytes are not capable to provide enough support for the neuronal population to thrive (Garcia et al., 2019). Interestingly, a recent study employed a transcriptomics approach to decipher the factors that drive astrocytic dysfunction in HD discovered an inverse relationship between the length of the PolyQ tail and metabolic activity, whereas astrocytic reactivity and DNA damage remained a constant factor (Lange et al., 2023).
Amyotrophic lateral sclerosis (ALS)
ALS is a type of motor neuron disease (MNs) that is characterized by the gradual loss of upper and lower motor neurons which are responsible for muscle movement, speech, and breathing (Hardiman et al., 2017; Buskila et al., 2019b). Astrocytes have been held accountable for causing MN death in ALS via numerous mechanisms, including the release of soluble neurotoxic factors that contribute to the selective death of MNs (Nagai et al., 2007). Among these, lipocalin 2, an inducible factor that is produced by astrocytes having a mutant TAR DNA-binding protein 43 (TDP-43), RNA-binding proteins fused in sarcoma (FUS) genes, and inorganic polyphosphate (polyp) secreted by mutant SOD1, TARDBP, and C9ORF72 astrocytes, are known to selectively eradicate MNs (Bi et al., 2013; Kia et al., 2018; Arredondo et al., 2022). Moreover, neutralisation of TNF-α in mtFUS mice model for ALS or expression of mFUS in astrocytes of TNF-α KO mice did not exhibit motor dysfunctions and prevented MN death suggesting TNF-α as a potential therapeutic target (Jensen et al., 2022). This demonstrates that astrocytes secrete different neurotoxic factors according to the mutations they carry. Consistent with these studies, treatment of primary spinal culture with astrocytic culture medium from SOD1 mice (ACM-hSOD1G93A) led to elevated persistent sodium inward currents, increased intracellular Ca2+ transients, and hyperexcitability of MNs that ultimately result in their death (Fritz et al., 2013). In addition, dysfunctional astrocytic glutamate transporters, elevated [K+]o and glutamate concentrations make the MNs vulnerable to excitotoxicity and worsen the disease progression (Rothstein et al., 1995; Rothstein et al., 1996; Do-Ha et al., 2018).
Recently, metanalysis of astrocytes from ALS mice and patient-derived iPSCs suggested that ALS- astrocytes are characterised by overexpression of genes implicated in immune system response and endoplasmic reticulum stress, and reduced expression of genes that affect glutamate uptake, maintenance of synaptic integrity, and support to neurons. These characteristics can be considered as priming factors which aid astrocytes to achieve reactive and pro-inflammatory detrimental phenotypes (Ziff et al., 2022). In line with this study, specific knock-out of activation factors in astrocytes, such as IL-1α, TNFα, and C1q prolonged the survivability of SOD1G93A mice (Guttenplan et al., 2020). Interestingly, the expression of astrocytic neurotoxic factor TNF-α is dependent on the activation of NF-κB, which plays a critical role in determining disease progression, as its activation in the presymptomatic stage makes the astrocytes acquire neuroprotective traits, while in the symptomatic stage, its activation worsens the disease (Ouali Alami et al., 2018). Indeed, mutant astrocytes are considered to cause higher levels of oxidative stress and dysregulated autophagy (Madill et al., 2017; Birger et al., 2019). Furthermore, malfunctioning of astrocytic metabolism, such as reduced NADH and adenosine deaminase production (Allen et al., 2019), transformed adenosine, fructose and glycogen metabolism and impaired lactate shuttling (Madji Hounoum et al., 2017) are evident in mutant ALS astrocytes, which result in energy deprivation and starvation in both astrocytes and neurons. This can be related to the accelerated senescence of astrocytes in ALS due to the shutdown of astrocytic support required to maintain healthy neuronal function and prevent MN death (Das and Svendsen, 2015).
Conclusion
Traditionally, most research about neural signaling took a neuro-centric approach, focusing on neuronal dysfunction, connectivity, and morphology. Our understanding of the role astrocytes play in the modulation of neuronal signalling has come a long way in the past two decades, mainly due to the development of new techniques, including two-photon laser scanning microscopy, electron microscopy reconstruction, genetically encoded Ca2+ dyes (GCaMPs), viral vector delivery systems, optogenetic and chemogenetic tools (Goshi et al., 2020; Delgado and Navarrete, 2023). Neuronal-astrocytic interactions are complex, mainly due to the expression of many receptors and channels in both neurons and astrocytes. The fact that astrocytic heterogeneity changes between different brain regions and during ageing pose another layer of complexity. Thus, disecting specific astrocytic processes that affect neuronal activity raise limitations. To overcome these limitations, the field should focus on developing new techniques and tools that can be used in situ to target specific astrocytic channels and proteins in specific brain areas, as recently reviewed by (Yu et al., 2020). Indeed, emerging tools such as the recently developed ‘neuron-astrocyte proximity assay (NAPA) by Khakh group (Octeau et al., 2018) and the genetically encoded K+ indicators by Dong group (Shen et al., 2019) have great potential is securing progress in this field (Gao et al., 2016; Yu et al., 2020).
Neuronal astrocytic interactions are highly dynamic and span a wide spectrum ranging from molecular to network levels. While some of the impacts astrocytes convey on neuronal signalling are carried via direct pathways, including downregulation of synaptic receptors and LTP, other astrocytic processes, such as heterosynaptic depression and regulation of hyper-synchronised activity of neurons are carried indirectly. Elucidating the involvement of astrocytic dysfunction during ageing and neurodegeneration has great potential to develop future CNS-related therapeutic targets. Indeed, as astrocytes are dividing cells and more adaptable than neurons, therapies aimed at astrocytic dysfunction rather than at neurons may prove superior in treating CNS disorders.
Author contributions
All authors listed have made a substantial, direct, and intellectual contribution to the work and approved it for publication.
Conflict of interest
The authors declare that the research was conducted in the absence of any commercial or financial relationships that could be construed as a potential conflict of interest.
Publisher’s note
All claims expressed in this article are solely those of the authors and do not necessarily represent those of their affiliated organizations, or those of the publisher, the editors and the reviewers. Any product that may be evaluated in this article, or claim that may be made by its manufacturer, is not guaranteed or endorsed by the publisher.
References
Abu-Ghanem, Y., Cohen, H., Buskila, Y., Grauer, E., and Amitai, Y. (2008). Enhanced stress reactivity in nitric oxide synthase type 2 mutant mice: Findings in support of astrocytic nitrosative modulation of behavior. Neuroscience 156 (2), 257–265. doi:10.1016/j.neuroscience.2008.07.043
Achour, S. B., and Pascual, s.O. (2010). Glia: The many ways to modulate synaptic plasticity. Neurochem. Int. 57 (4), 440–445. doi:10.1016/j.neuint.2010.02.013
Ackerman, S. D., Perez-Catalan, N. A., Freeman, M. R., and Doe, C. Q. (2021). Astrocytes close a motor circuit critical period. Nature 592 (7854), 414–420. doi:10.1038/s41586-021-03441-2
Adamantidis, A. R., Gutierrez Herrera, C., and Gent, T. C. (2019). Oscillating circuitries in the sleeping brain. Nat. Rev. Neurosci. 20 (12), 746–762. doi:10.1038/s41583-019-0223-4
Agulhon, C., Fiacco, T. A., and McCarthy, K. D. (2010). Hippocampal short-and long-term plasticity are not modulated by astrocyte Ca2+ signaling. Science 327 (5970), 1250–1254. doi:10.1126/science.1184821
Al Awabdh, S., Gupta-Agarwal, S., Sheehan, D. F., Muir, J., Norkett, R., Twelvetrees, A. E., et al. (2016). Neuronal activity mediated regulation of glutamate transporter GLT-1 surface diffusion in rat astrocytes in dissociated and slice cultures. Glia 64 (7), 1252–1264. doi:10.1002/glia.22997
Allen, N. J., Bennett, M. L., Foo, L. C., Wang, G. X., Chakraborty, C., Smith, S. J., et al. (2012). Astrocyte glypicans 4 and 6 promote formation of excitatory synapses via GluA1 AMPA receptors. Nature 486 (7403), 410–414. doi:10.1038/nature11059
Allen, S. P., Hall, B., Castelli, L. M., Francis, L., Woof, R., Siskos, A. P., et al. (2019). Astrocyte adenosine deaminase loss increases motor neuron toxicity in amyotrophic lateral sclerosis. Brain 142 (3), 586–605. doi:10.1093/brain/awy353
Amiri, M., Hosseinmardi, N., Bahrami, F., and Janahmadi, M. (2013). Astrocyte-neuron interaction as a mechanism responsible for generation of neural synchrony: A study based on modeling and experiments. J. Comput. Neurosci. 34, 489–504. doi:10.1007/s10827-012-0432-6
Andersen, J. V., Jakobsen, E., Westi, E. W., Lie, M. E., Voss, C. M., Aldana, B. I., et al. (2020). Extensive astrocyte metabolism of γ-aminobutyric acid (GABA) sustains glutamine synthesis in the mammalian cerebral cortex. Glia 68 (12), 2601–2612. doi:10.1002/glia.23872
Andersen, J. V., McNair, L. F., Schousboe, A., and Waagepetersen, H. S. (2017). Specificity of exogenous acetate and glutamate as astrocyte substrates examined in acute brain slices from female mice using methionine sulfoximine (MSO) to inhibit glutamine synthesis. J. Neurosci. Res. 95 (11), 2207–2216. doi:10.1002/jnr.24038
Andersen, J. V., Skotte, N. H., Christensen, S. K., Polli, F. S., Shabani, M., Markussen, K. H., et al. (2021). Hippocampal disruptions of synaptic and astrocyte metabolism are primary events of early amyloid pathology in the 5xFAD mouse model of Alzheimer’s disease. Cell Death Dis. 12 (11), 954. doi:10.1038/s41419-021-04237-y
Anderson, M. A., Burda, J. E., Ren, Y., Ao, Y., O’Shea, T. M., Kawaguchi, R., et al. (2016). Astrocyte scar formation aids central nervous system axon regeneration. Nature 532 (7598), 195–200. doi:10.1038/nature17623
Araque, A., Parpura, V., Sanzgiri, R. P., and Haydon, P. G. (1999). Tripartite synapses: Glia, the unacknowledged partner. Trends Neurosci. 22 (5), 208–215. doi:10.1016/s0166-2236(98)01349-6
Arredondo, C., Cefaliello, C., Dyrda, A., Jury, N., Martinez, P., Díaz, I., et al. (2022). Excessive release of inorganic polyphosphate by ALS/FTD astrocytes causes non-cell-autonomous toxicity to motoneurons. Neuron 110 (10), 1656–1670.e12. doi:10.1016/j.neuron.2022.02.010
Assefa, B. T., Gebre, A. K., and Altaye, B. M. (2018). Reactive astrocytes as drug target in Alzheimer’s disease. BioMed Res. Int. 2018, 4160247, doi:10.1155/2018/4160247
Baldwin, K. T., and Eroglu, C. (2017). Molecular mechanisms of astrocyte-induced synaptogenesis. Curr. Opin. Neurobiol. 45, 113–120. doi:10.1016/j.conb.2017.05.006
Barbay, T., Pecchi, E., Ducrocq, M., Rouach, N., Brocard, F., and Bos, R. (2023). Astrocytic Kir4. 1 channels regulate locomotion by orchestrating neuronal rhythmicity in the spinal network. Glia 71, 1259. doi:10.1002/glia.24337
Basurco, L., Abellanas, M. A., Ayerra, L., Conde, E., Vinueza-Gavilanes, R., Luquin, E., et al. (2023). Microglia and astrocyte activation is region-dependent in the α-synuclein mouse model of Parkinson's disease. Glia 71 (3), 571–587. doi:10.1002/glia.24295
Bay, V., and Butt, A. M. (2012). Relationship between glial potassium regulation and axon excitability: A role for glial Kir4. 1 channels. Glia 60 (4), 651–660. doi:10.1002/glia.22299
Baylor, D. A., and Nicholls, J. G. (1969). Changes in extracellular potassium concentration produced by neuronal activity in the central nervous system of the leech. J. Physiol. 203 (3), 555–569. doi:10.1113/jphysiol.1969.sp008879
Bazargani, N., and Attwell, D. (2016). Astrocyte calcium signaling: The third wave. Nat. Neurosci. 19 (2), 182–189. doi:10.1038/nn.4201
Bélanger, M., Allaman, I., and Magistretti, P. J. (2011). Brain energy metabolism: Focus on astrocyte-neuron metabolic cooperation. Cell metab. 14 (6), 724–738. doi:10.1016/j.cmet.2011.08.016
Bellot-Saez, A., Cohen, G., van Schaik, A., Ooi, L., W Morley, J., and Buskila, Y. (2018). Astrocytic modulation of cortical oscillations. Sci. Rep. 8 (1), 11565–11613. doi:10.1038/s41598-018-30003-w
Bellot-Saez, A., Kékesi, O., Morley, J. W., and Buskila, Y. (2017). Astrocytic modulation of neuronal excitability through K+ spatial buffering. Neurosci. Biobehav. Rev. 77, 87–97. doi:10.1016/j.neubiorev.2017.03.002
Bellot-Saez, A., Stevenson, R., Kekesi, O., Samokhina, E., Ben-Abu, Y., Morley, J. W., et al. (2021). Neuromodulation of astrocytic K(+) clearance. Int. J. Mol. Sci. 22 (5), 2520. doi:10.3390/ijms22052520
Benraiss, A., Wang, S., Herrlinger, S., Li, X., Chandler-Militello, D., Mauceri, J., et al. (2016). Human glia can both induce and rescue aspects of disease phenotype in Huntington disease. Nat. Commun. 7 (1), 11758. doi:10.1038/ncomms11758
Berezhnov, A. V., Fedotova, E. I., Sergeev, A. I., Teplov, I. Y., and Abramov, A. Y. (2021). Dopamine controls neuronal spontaneous calcium oscillations via astrocytic signal. Cell Calcium 94, 102359. doi:10.1016/j.ceca.2021.102359
Bi, F., Huang, C., Tong, J., Qiu, G., Huang, B., Wu, Q., et al. (2013). Reactive astrocytes secrete lcn2 to promote neuron death. Proc. Natl. Acad. Sci. 110 (10), 4069–4074. doi:10.1073/pnas.1218497110
Birger, A., Ben-Dor, I., Ottolenghi, M., Turetsky, T., Gil, Y., Sweetat, S., et al. (2019). Human iPSC-derived astrocytes from ALS patients with mutated C9ORF72 show increased oxidative stress and neurotoxicity. EBioMedicine 50, 274–289. doi:10.1016/j.ebiom.2019.11.026
Blaustein, M. P. (1985). “Intracellular calcium as a second messenger,” in Calcium in biological systems (Germany: Springer), 23–33.
Boddum, K., Jensen, T. P., Magloire, V., Kristiansen, U., Rusakov, D. A., Pavlov, I., et al. (2016). Astrocytic GABA transporter activity modulates excitatory neurotransmission. Nat. Commun. 7 (1), 13572–13610. doi:10.1038/ncomms13572
Boisvert, M. M., Erikson, G. A., Shokhirev, M. N., and Allen, N. J. (2018). The aging astrocyte transcriptome from multiple regions of the mouse brain. Cell Rep. 22 (1), 269–285. doi:10.1016/j.celrep.2017.12.039
Bojarskaite, L., Bjørnstad, D. M., Pettersen, K. H., Cunen, C., Hermansen, G. H., Åbjørsbråten, K. S., et al. (2020). Astrocytic Ca2+ signaling is reduced during sleep and is involved in the regulation of slow wave sleep. Nat. Commun. 11 (1), 3240–3316. doi:10.1038/s41467-020-17062-2
Bonansco, C., Couve, A., Perea, G., Ferradas, C. . Á., Roncagliolo, M., and Fuenzalida, M. (2011). Glutamate released spontaneously from astrocytes sets the threshold for synaptic plasticity. Eur. J. Neurosci. 33 (8), 1483–1492. doi:10.1111/j.1460-9568.2011.07631.x
Boussicault, L., Hérard, A.-S., Calingasan, N., Petit, F., Malgorn, C., Merienne, N., et al. (2014). Impaired brain energy metabolism in the BACHD mouse model of Huntington's disease: Critical role of astrocyte–neuron interactions. J. Cereb. Blood Flow Metabolism 34 (9), 1500–1510. doi:10.1038/jcbfm.2014.110
Bradford, J., Shin, J.-Y., Roberts, M., Wang, C.-E., Li, X.-J., and Li, S. (2009). Expression of mutant huntingtin in mouse brain astrocytes causes age-dependent neurological symptoms. Proc. Natl. Acad. Sci. 106 (52), 22480–22485. doi:10.1073/pnas.0911503106
Brancaccio, M., Patton, A. P., Chesham, J. E., Maywood, E. S., and Hastings, M. H. (2017). Astrocytes control circadian timekeeping in the suprachiasmatic nucleus via glutamatergic signaling. Neuron 93 (6), 1420–1435. doi:10.1016/j.neuron.2017.02.030
Brawek, B., and Garaschuk, O. (2014). Network-wide dysregulation of calcium homeostasis in Alzheimer’s disease. Cell Tissue Res. 357, 427–438. doi:10.1007/s00441-014-1798-8
Brazhe, A. R., Verisokin, A. Y., Verveyko, D. V., and Postnov, D. E. (2018). Sodium–calcium exchanger can account for regenerative Ca2+ entry in thin astrocyte processes. Front. Cell. Neurosci. 12, 250. doi:10.3389/fncel.2018.00250
Brockett, A. T., Kane, G. A., Monari, P. K., Briones, B. A., Vigneron, P.-A., Barber, G. A., et al. (2018). Evidence supporting a role for astrocytes in the regulation of cognitive flexibility and neuronal oscillations through the Ca2+ binding protein S100β. PLoS One 13 (4), e0195726. doi:10.1371/journal.pone.0195726
Brymer, K. J., Hurley, E. P., Barron, J. C., Mukherjee, B., Barnes, J. R., Nafar, F., et al. (2023). Asymmetric dysregulation of glutamate dynamics across the synaptic cleft in a mouse model of Alzheimer’s disease. Acta Neuropathol. Commun. 11 (1), 27–20. doi:10.1186/s40478-023-01524-x
Burda, J. E., Bernstein, A. M., and Sofroniew, M. V. (2016). Astrocyte roles in traumatic brain injury. Exp. Neurol. 275, 305–315. doi:10.1016/j.expneurol.2015.03.020
Buskila, Y., and Amitai, Y. (2010). Astrocytic iNOS-dependent enhancement of synaptic release in mouse neocortex. J. Neurophysiol. 103 (3), 1322–1328. doi:10.1152/jn.00676.2009
Buskila, Y., Bellot-Saez, A., and Morley, J. W. (2019a). Generating brain waves, the power of astrocytes. Front. Neurosci. 13, 1125. doi:10.3389/fnins.2019.01125
Buskila, Y., Crowe, S. E., and Ellis-Davies, G. C. (2013). Synaptic deficits in layer 5 neurons precede overt structural decay in 5xFAD mice. Neuroscience 254, 152–159. doi:10.1016/j.neuroscience.2013.09.016
Buskila, Y., Farkash, S., Hershfinkel, M., and Amitai, Y. (2005). Rapid and reactive nitric oxide production by astrocytes in mouse neocortical slices. Glia 52 (3), 169–176. doi:10.1002/glia.20217
Buskila, Y., Kekesi, O., Bellot-Saez, A., Seah, W., Berg, T., Trpceski, M., et al. (2019b). Dynamic interplay between H-current and M-current controls motoneuron hyperexcitability in amyotrophic lateral sclerosis. Cell Death Dis. 10 (4), 310. doi:10.1038/s41419-019-1538-9
Buzsáki, G., and Draguhn, A. (2004). Neuronal oscillations in cortical networks. Science 304, 1926–1929. doi:10.1126/science.1099745
Campanelli, F., Natale, G., Marino, G., Ghiglieri, V., and Calabresi, P. (2022). Striatal glutamatergic hyperactivity in Parkinson's disease. Neurobiol. Dis. 168, 105697. doi:10.1016/j.nbd.2022.105697
Carmignoto, G., and Fellin, T. (2006). Glutamate release from astrocytes as a non-synaptic mechanism for neuronal synchronization in the hippocampus. J. Physiology-Paris 99 (2), 98–102. doi:10.1016/j.jphysparis.2005.12.008
Cavaliere, F., Cerf, L., Dehay, B., Ramos-Gonzalez, P., De Giorgi, F., Bourdenx, M., et al. (2017). In vitro α-synuclein neurotoxicity and spreading among neurons and astrocytes using Lewy body extracts from Parkinson disease brains. Neurobiol. Dis. 103, 101–112. doi:10.1016/j.nbd.2017.04.011
Ceyzériat, K., Ben Haim, L., Denizot, A., Pommier, D., Matos, M., Guillemaud, O., et al. (2018). Modulation of astrocyte reactivity improves functional deficits in mouse models of Alzheimer’s disease. Acta neuropathol. Commun. 6 (1), 104–123. doi:10.1186/s40478-018-0606-1
Chattopadhyaya, B., Di Cristo, G., Wu, C. Z., Knott, G., Kuhlman, S., Fu, Y., et al. (2007). GAD67-mediated GABA synthesis and signaling regulate inhibitory synaptic innervation in the visual cortex. Neuron 54 (6), 889–903. doi:10.1016/j.neuron.2007.05.015
Chen, J., Tan, Z., Zeng, L., Zhang, X., He, Y., Gao, W., et al. (2013). Heterosynaptic long-term depression mediated by ATP released from astrocytes. Glia 61 (2), 178–191. doi:10.1002/glia.22425
Christensen, R. K., Delgado-Lezama, R., Russo, R. E., Lind, B. L., Alcocer, E. L., Rath, M. F., et al. (2018). Spinal dorsal horn astrocytes release GABA in response to synaptic activation. J. Physiology 596 (20), 4983–4994. doi:10.1113/JP276562
Christopherson, K. S., Ullian, E. M., Stokes, C. C., Mullowney, C. E., Hell, J. W., Agah, A., et al. (2005). Thrombospondins are astrocyte-secreted proteins that promote CNS synaptogenesis. Cell 120 (3), 421–433. doi:10.1016/j.cell.2004.12.020
Chun, H., Im, H., Kang, Y. J., Kim, Y., Shin, J. H., Won, W., et al. (2020). Severe reactive astrocytes precipitate pathological hallmarks of Alzheimer’s disease via H2O2− production. Nat. Neurosci. 23 (12), 1555–1566. doi:10.1038/s41593-020-00735-y
Chung, W.-S., Allen, N. J., and Eroglu, C. (2015). Astrocytes control synapse formation, function, and elimination. Cold Spring Harb. Perspect. Biol. 7 (9), a020370. doi:10.1101/cshperspect.a020370
Corder, E. H., Saunders, A. M., Strittmatter, W. J., Schmechel, D. E., Gaskell, P. C., Small, G., et al. (1993). Gene dose of apolipoprotein E type 4 allele and the risk of Alzheimer's disease in late onset families. Science 261 (5123), 921–923. doi:10.1126/science.8346443
Covelo, A., and Araque, A. (2018). Neuronal activity determines distinct gliotransmitter release from a single astrocyte. Elife 7, e32237. doi:10.7554/eLife.32237
Curtis, D. R., and Johnston, G. A. (1974). Amino acid transmitters in the mammalian central nervous system. Ergebnisse der Physiologie Rev. Physiology 69, 97–188. doi:10.1007/3-540-06498-2_3
Cvetanovic, M. (2015). Decreased expression of glutamate transporter GLAST in Bergmann glia is associated with the loss of Purkinje neurons in the spinocerebellar ataxia type 1. Cerebellum 14 (1), 8–11. doi:10.1007/s12311-014-0605-0
Das, M. M., and Svendsen, C. N. (2015). Astrocytes show reduced support of motor neurons with aging that is accelerated in a rodent model of ALS. Neurobiol. aging 36 (2), 1130–1139. doi:10.1016/j.neurobiolaging.2014.09.020
Davis, N. J., Tomlinson, S. P., and Morgan, H. M. (2012). The role of beta-frequency neural oscillations in motor control. J. Neurosci. 32 (2), 403–404. doi:10.1523/JNEUROSCI.5106-11.2012
De Lau, L. M., and Breteler, M. M. (2006). Epidemiology of Parkinson's disease. Lancet Neurology 5 (6), 525–535. doi:10.1016/S1474-4422(06)70471-9
Deitmer, J. W., Theparambil, S. M., Ruminot, I., Noor, S. I., and Becker, H. M. (2019). Energy dynamics in the brain: Contributions of astrocytes to metabolism and pH homeostasis. Front. Neurosci. 13, 1301. doi:10.3389/fnins.2019.01301
Delgado, L., and Navarrete, M. (2023). Shining the light on astrocytic ensembles. Cells 12 (9), 1253. doi:10.3390/cells12091253
Descalzi, G., Gao, V., Steinman, M. Q., Suzuki, A., and Alberini, C. M. (2019). Lactate from astrocytes fuels learning-induced mRNA translation in excitatory and inhibitory neurons. Commun. Biol. 2 (1), 213–229. doi:10.1038/s42003-019-0495-2
di Domenico, A., Carola, G., Calatayud, C., Pons-Espinal, M., Muñoz, J. P., Richaud-Patin, Y., et al. (2019). Patient-specific iPSC-derived astrocytes contribute to non-cell-autonomous neurodegeneration in Parkinson's disease. Stem Cell Rep. 12 (2), 213–229. doi:10.1016/j.stemcr.2018.12.011
Di Marco Vieira, B., Radford, R. A., Hayashi, J., Eaton, E. D., Greenaway, B., Jambas, M., et al. (2020). Extracellular alpha-synuclein promotes a neuroinhibitory secretory phenotype in astrocytes. Life 10 (9), 183. doi:10.3390/life10090183
Diniz, L. P., Almeida, J. C., Tortelli, V., Lopes, C. V., Setti-Perdigão, P., Stipursky, J., et al. (2012). Astrocyte-induced synaptogenesis is mediated by transforming growth factor β signaling through modulation of D-serine levels in cerebral cortex neurons. J. Biol. Chem. 287 (49), 41432–41445. doi:10.1074/jbc.M112.380824
Diniz, L. P., Tortelli, V., Garcia, M. N., Araújo, A. P. B., Melo, H. M., Seixas da Silva, G. S., et al. (2014). Astrocyte transforming growth factor beta 1 promotes inhibitory synapse formation via CaM kinase II signaling. Glia 62 (12), 1917–1931. doi:10.1002/glia.22713
Djukic, B., Casper, K. B., Philpot, B. D., Chin, L.-S., and McCarthy, K. D. (2007). Conditional knock-out of Kir4. 1 leads to glial membrane depolarization, inhibition of potassium and glutamate uptake, and enhanced short-term synaptic potentiation. J. Neurosci. 27 (42), 11354–11365. doi:10.1523/JNEUROSCI.0723-07.2007
Do-Ha, D., Buskila, Y., and Ooi, L. (2018). Impairments in motor neurons, interneurons and astrocytes contribute to hyperexcitability in ALS: Underlying mechanisms and paths to therapy. Mol. Neurobiol. 55 (2), 1410–1418. doi:10.1007/s12035-017-0392-y
Dong, X.-x., Wang, Y., and Qin, Z.-h. (2009). Molecular mechanisms of excitotoxicity and their relevance to pathogenesis of neurodegenerative diseases. Acta Pharmacol. Sin. 30 (4), 379–387. doi:10.1038/aps.2009.24
Doron, A., Rubin, A., Benmelech-Chovav, A., Benaim, N., Carmi, T., Refaeli, R., et al. (2022). Hippocampal astrocytes encode reward location. Nature 609 (7928), 772–778. doi:10.1038/s41586-022-05146-6
Dutta, D. J., Woo, D. H., Lee, P. R., Pajevic, S., Bukalo, O., Huffman, W. C., et al. (2018). Regulation of myelin structure and conduction velocity by perinodal astrocytes. Proc. Natl. Acad. Sci. 115 (46), 11832–11837. doi:10.1073/pnas.1811013115
Dvorzhak, A., Vagner, T., Kirmse, K., and Grantyn, R. (2016). Functional indicators of glutamate transport in single striatal astrocytes and the influence of Kir4. 1 in normal and Huntington mice. J. Neurosci. 36 (18), 4959–4975. doi:10.1523/JNEUROSCI.0316-16.2016
Ebrahimi, M., Yamamoto, Y., Sharifi, K., Kida, H., Kagawa, Y., Yasumoto, Y., et al. (2016). Astrocyte-expressed FABP 7 regulates dendritic morphology and excitatory synaptic function of cortical neurons. Glia 64 (1), 48–62. doi:10.1002/glia.22902
Eidelberg, D., and Surmeier, D. J. (2011). Brain networks in Huntington disease. J. Clin. investigation 121 (2), 484–492. doi:10.1172/JCI45646
Elmariah, S. B., Oh, E. J., Hughes, E. G., and Balice-Gordon, R. J. (2005). Astrocytes regulate inhibitory synapse formation via Trk-mediated modulation of postsynaptic GABAA receptors. J. Neurosci. 25 (14), 3638–3650. doi:10.1523/JNEUROSCI.3980-04.2005
Eroglu, C., Allen, N. J., Susman, M. W., O'Rourke, N. A., Park, C. Y., Özkan, E., et al. (2009). Gabapentin receptor alpha2delta-1 is a neuronal thrombospondin receptor responsible for excitatory CNS synaptogenesis. Cell 139 (2), 380–392. doi:10.1016/j.cell.2009.09.025
Faideau, M., Kim, J., Cormier, K., Gilmore, R., Welch, M., Auregan, G., et al. (2010). In vivo expression of polyglutamine-expanded huntingtin by mouse striatal astrocytes impairs glutamate transport: A correlation with Huntington's disease subjects. Hum. Mol. Genet. 19 (15), 3053–3067. doi:10.1093/hmg/ddq212
Falcón-Moya, R., Pérez-Rodríguez, M., Prius-Mengual, J., Andrade-Talavera, Y., Arroyo-García, L. E., Pérez-Artés, R., et al. (2020). Astrocyte-mediated switch in spike timing-dependent plasticity during hippocampal development. Nat. Commun. 11 (1), 4388–4414. doi:10.1038/s41467-020-18024-4
Farhy-Tselnicker, I., van Casteren, A. C., Lee, A., Chang, V. T., Aricescu, A. R., and Allen, N. J. (2017). Astrocyte-secreted glypican 4 regulates release of neuronal pentraxin 1 from axons to induce functional synapse formation. Neuron 96 (2), 428–445. doi:10.1016/j.neuron.2017.09.053
Fellin, T., Pascual, O., Gobbo, S., Pozzan, T., Haydon, P. G., and Carmignoto, G. (2004). Neuronal synchrony mediated by astrocytic glutamate through activation of extrasynaptic NMDA receptors. Neuron 43 (5), 729–743. doi:10.1016/j.neuron.2004.08.011
Ferrarese, C., Tremolizzo, L., Rigoldi, M., Sala, G., Begni, B., Brighina, L., et al. (2001). Decreased platelet glutamate uptake and genetic risk factors in patients with Parkinson's disease. Neurol. Sci. 22, 65–66. doi:10.1007/s100720170049
Ferri, C. P., Prince, M., Brayne, C., Brodaty, H., Fratiglioni, L., Ganguli, M., et al. (2005). Global prevalence of dementia: A delphi consensus study. lancet 366 (9503), 2112–2117. doi:10.1016/S0140-6736(05)67889-0
Fiacco, T. A., Agulhon, C., Taves, S. R., Petravicz, J., Casper, K. B., Dong, X., et al. (2007). Selective stimulation of astrocyte calcium in situ does not affect neuronal excitatory synaptic activity. Neuron 54 (4), 611–626. doi:10.1016/j.neuron.2007.04.032
Flanagan, B., McDaid, L., Wade, J., Wong-Lin, K., and Harkin, J. (2018). A computational study of astrocytic glutamate influence on post-synaptic neuronal excitability. PLoS Comput. Biol. 14 (4), e1006040. doi:10.1371/journal.pcbi.1006040
Florian, C., Vecsey, C. G., Halassa, M. M., Haydon, P. G., and Abel, T. (2011). Astrocyte-derived adenosine and A1 receptor activity contribute to sleep loss-induced deficits in hippocampal synaptic plasticity and memory in mice. J. Neurosci. 31 (19), 6956–6962. doi:10.1523/JNEUROSCI.5761-10.2011
Foley, J., Blutstein, T., Lee, S., Erneux, C., Halassa, M. M., and Haydon, P. (2017). Astrocytic IP3/Ca2+ signaling modulates theta rhythm and REM sleep. Front. neural circuits 11, 3. doi:10.3389/fncir.2017.00003
Fonnum, F. (1984). Glutamate: A neurotransmitter in mammalian brain. J. Neurochem. 42 (1), 1–11. doi:10.1111/j.1471-4159.1984.tb09689.x
Fraser, D. D., Mudrick-Donnon, L. A., and Macvicar, B. A. (1994). Astrocytic GABA receptors. Glia 11 (2), 83–93. doi:10.1002/glia.440110203
Fritz, E., Izaurieta, P., Weiss, A., Mir, F. R., Rojas, P., Gonzalez, D., et al. (2013). Mutant SOD1-expressing astrocytes release toxic factors that trigger motoneuron death by inducing hyperexcitability. J. neurophysiology 109 (11), 2803–2814. doi:10.1152/jn.00500.2012
Gao, Y., Broussard, J., Haque, A., Revzin, A., and Lin, T. (2016). Functional imaging of neuron–astrocyte interactions in a compartmentalized microfluidic device. Microsystems Nanoeng. 2 (1), 15045–15049. doi:10.1038/micronano.2015.45
Garcia, V. J., Rushton, D. J., Tom, C. M., Allen, N. D., Kemp, P. J., Svendsen, C. N., et al. (2019). Huntington’s disease patient-derived astrocytes display electrophysiological impairments and reduced neuronal support. Front. Neurosci. 13, 669. doi:10.3389/fnins.2019.00669
Ghirardini, E., Wadle, S. L., Augustin, V., Becker, J., Brill, S., Hammerich, J., et al. (2018). Expression of functional inhibitory neurotransmitter transporters GlyT1, GAT-1, and GAT-3 by astrocytes of inferior colliculus and hippocampus. Mol. Brain 11 (1), 4–16. doi:10.1186/s13041-018-0346-y
Gómez-Gonzalo, M., Navarrete, M., Perea, G., Covelo, A., Martín-Fernández, M., Shigemoto, R., et al. (2015). Endocannabinoids induce lateral long-term potentiation of transmitter release by stimulation of gliotransmission. Cereb. cortex 25 (10), 3699–3712. doi:10.1093/cercor/bhu231
Gomez-Arboledas, A., Davila, J. C., Sanchez-Mejias, E., Navarro, V., Nuñez-Diaz, C., Sanchez-Varo, R., et al. (2018). Phagocytic clearance of presynaptic dystrophies by reactive astrocytes in Alzheimer's disease. Glia 66 (3), 637–653. doi:10.1002/glia.23270
Goshi, N., Morgan, R. K., Lein, P. J., and Seker, E. (2020). A primary neural cell culture model to study neuron, astrocyte, and microglia interactions in neuroinflammation. J. neuroinflammation 17 (1), 155–216. doi:10.1186/s12974-020-01819-z
Guttenplan, K. A., Weigel, M. K., Adler, D. I., Couthouis, J., Liddelow, S. A., Gitler, A. D., et al. (2020). Knockout of reactive astrocyte activating factors slows disease progression in an ALS mouse model. Nat. Commun. 11 (1), 3753. doi:10.1038/s41467-020-17514-9
Halassa, M. M., Florian, C., Fellin, T., Munoz, J. R., Lee, S.-Y., Abel, T., et al. (2009). Astrocytic modulation of sleep homeostasis and cognitive consequences of sleep loss. Neuron 61 (2), 213–219. doi:10.1016/j.neuron.2008.11.024
Han, K.-S., Woo, J., Park, H., Yoon, B.-J., Choi, S., and Lee, C. J. (2013). Channel-mediated astrocytic glutamate release via Bestrophin-1 targets synaptic NMDARs. Mol. Brain 6 (1), 4–9. doi:10.1186/1756-6606-6-4
Hanson, E., Armbruster, M., Cantu, D., Andresen, L., Taylor, A., Danbolt, N. C., et al. (2015). Astrocytic glutamate uptake is slow and does not limit neuronal NMDA receptor activation in the neonatal neocortex. Glia 63 (10), 1784–1796. doi:10.1002/glia.22844
Hardiman, O., Al-Chalabi, A., Chio, A., Corr, E. M., Logroscino, G., Robberecht, W., et al. (2017). Amyotrophic lateral sclerosis. Nat. Rev. Dis. Prim. 3 (1), 17071–17119. doi:10.1038/nrdp.2017.71
Heithoff, B. P., George, K. K., Phares, A. N., Zuidhoek, I. A., Munoz-Ballester, C., and Robel, S. (2021). Astrocytes are necessary for blood–brain barrier maintenance in the adult mouse brain. Glia 69 (2), 436–472. doi:10.1002/glia.23908
Héja, L., Barabás, P., Nyitrai, G., Kékesi, K. A., Lasztóczi, B., Tőke, O., et al. (2009). Glutamate uptake triggers transporter-mediated GABA release from astrocytes. PloS one 4 (9), e7153. doi:10.1371/journal.pone.0007153
Héja, L., and Kardos, J. (2020). NCX activity generates spontaneous Ca2+ oscillations in the astrocytic leaflet microdomain. Cell Calcium 86, 102137. doi:10.1016/j.ceca.2019.102137
Héja, L., Nyitrai, G., Kékesi, O., Dobolyi, Á., Szabó, P., Fiáth, R., et al. (2012). Astrocytes convert network excitation to tonic inhibition of neurons. BMC Biol. 10 (1), 26–21. doi:10.1186/1741-7007-10-26
Henneberger, C., Papouin, T., Oliet, S. H., and Rusakov, D. A. (2010). Long-term potentiation depends on release of D-serine from astrocytes. Nature 463 (7278), 232–236. doi:10.1038/nature08673
Hertz, L., Song, D., Xu, J., Peng, L., and Gibbs, M. E. (2015). Role of the astrocytic Na(+), K(+)-ATPase in K(+) homeostasis in brain: K(+) uptake, signaling pathways and substrate utilization. Neurochem. Res. 40 (12), 2505–2516. doi:10.1007/s11064-014-1505-x
Hillen, A. E., Burbach, J. P. H., and Hol, E. M. (2018). Cell adhesion and matricellular support by astrocytes of the tripartite synapse. Prog. Neurobiol. 165, 66–86. doi:10.1016/j.pneurobio.2018.02.002
Hösli, L., Binini, N., Ferrari, K. D., Thieren, L., Looser, Z. J., Zuend, M., et al. (2022). Decoupling astrocytes in adult mice impairs synaptic plasticity and spatial learning. Cell Rep. 38 (10), 110484. doi:10.1016/j.celrep.2022.110484
Huang, Y.-T., Chang, Y.-L., Chen, C.-C., Lai, P.-Y., and Chan, C. (2017). Positive feedback and synchronized bursts in neuronal cultures. PloS one 12 (11), e0187276. doi:10.1371/journal.pone.0187276
Hurtig, H., Trojanowski, J., Galvin, J., Ewbank, D., Schmidt, M., Lee, V.-Y., et al. (2000). Alpha-synuclein cortical Lewy bodies correlate with dementia in Parkinson’s disease. Neurology 54 (10), 1916–1921. doi:10.1212/wnl.54.10.1916
Ingiosi, A. M., and Frank, M. G. (2022). Goodnight, astrocyte: Waking up to astroglial mechanisms in sleep. FEBS J. 290, 2553–2564. doi:10.1111/febs.16424
Ingiosi, A. M., Hayworth, C. R., Harvey, D. O., Singletary, K. G., Rempe, M. J., Wisor, J. P., et al. (2020). A role for astroglial calcium in mammalian sleep and sleep regulation. Curr. Biol. 30 (22), 4373–4383. doi:10.1016/j.cub.2020.08.052
Iovino, L., Tremblay, M., and Civiero, L. (2020). Glutamate-induced excitotoxicity in Parkinson's disease: The role of glial cells. J. Pharmacol. Sci. 144 (3), 151–164. doi:10.1016/j.jphs.2020.07.011
Jensen, B. K., McAvoy, K. J., Heinsinger, N. M., Lepore, A. C., Ilieva, H., Haeusler, A. R., et al. (2022). Targeting TNFα produced by astrocytes expressing amyotrophic lateral sclerosis-linked mutant fused in sarcoma prevents neurodegeneration and motor dysfunction in mice. Glia 70 (7), 1426–1449. doi:10.1002/glia.24183
Jiang, R., Diaz-Castro, B., Looger, L. L., and Khakh, B. S. (2016). Dysfunctional calcium and glutamate signaling in striatal astrocytes from Huntington's disease model mice. J. Neurosci. 36 (12), 3453–3470. doi:10.1523/JNEUROSCI.3693-15.2016
Jing, L., Cheng, S., Pan, Y., Liu, Q., Yang, W., Li, S., et al. (2021). Accumulation of endogenous mutant huntingtin in astrocytes exacerbates neuropathology of Huntington disease in mice. Mol. Neurobiol. 58, 5112–5126. doi:10.1007/s12035-021-02451-5
Jiwaji, Z., Tiwari, S. S., Avilés-Reyes, R. X., Hooley, M., Hampton, D., Torvell, M., et al. (2022). Reactive astrocytes acquire neuroprotective as well as deleterious signatures in response to Tau and Aß pathology. Nat. Commun. 13 (1), 135. doi:10.1038/s41467-021-27702-w
Karus, C., Mondragão, M. A., Ziemens, D., and Rose, C. R. (2015). Astrocytes restrict discharge duration and neuronal sodium loads during recurrent network activity. Glia 63 (6), 936–957. doi:10.1002/glia.22793
Katsouri, L., Birch, A. M., Renziehausen, A. W., Zach, C., Aman, Y., Steeds, H., et al. (2020). Ablation of reactive astrocytes exacerbates disease pathology in a model of Alzheimer's disease. Glia 68 (5), 1017–1030. doi:10.1002/glia.23759
Kekesi, O., Liang, H., Munch, G., Morley, J. W., Gyengesi, E., and Buskila, Y. (2019). The differential impact of acute microglia activation on the excitability of cholinergic neurons in the mouse medial septum. Brain Struct. Funct. 224 (7), 2297–2309. doi:10.1007/s00429-019-01905-w
Kelley, K. W., Haim, L. B., Schirmer, L., Tyzack, G. E., Tolman, M., Miller, J. G., et al. (2018). Kir4 1-dependent astrocyte-fast motor neuron interactions are required for peak strength. Neuron 98 (2), 306–319. doi:10.1016/j.neuron.2018.03.010
Kia, A., McAvoy, K., Krishnamurthy, K., Trotti, D., and Pasinelli, P. (2018). Astrocytes expressing ALS-linked mutant FUS induce motor neuron death through release of tumor necrosis factor-alpha. Glia 66 (5), 1016–1033. doi:10.1002/glia.23298
Kim, J.-I., Ganesan, S., Luo, S. X., Wu, Y.-W., Park, E., Huang, E. J., et al. (2015). Aldehyde dehydrogenase 1a1 mediates a GABA synthesis pathway in midbrain dopaminergic neurons. Science 350 (6256), 102–106. doi:10.1126/science.aac4690
Kirischuk, S., Héja, L., Kardos, J., and Billups, B. (2016). Astrocyte sodium signaling and the regulation of neurotransmission. Glia 64 (10), 1655–1666. doi:10.1002/glia.22943
Kirischuk, S., Kettenmann, H., and Verkhratsky, A. (2007). Membrane currents and cytoplasmic sodium transients generated by glutamate transport in Bergmann glial cells. Pflügers Archiv-European J. Physiology 454 (2), 245–252. doi:10.1007/s00424-007-0207-5
Kirischuk, S., Parpura, V., and Verkhratsky, A. (2012). Sodium dynamics: Another key to astroglial excitability? Trends Neurosci. 35 (8), 497–506. doi:10.1016/j.tins.2012.04.003
Klapper, S. D., Garg, P., Dagar, S., Lenk, K., Gottmann, K., and Nieweg, K. (2019). Astrocyte lineage cells are essential for functional neuronal differentiation and synapse maturation in human iPSC-derived neural networks. Glia 67 (10), 1893–1909. doi:10.1002/glia.23666
Kocsis, J., Malenka, R., and Waxman, S. (1983). Effects of extracellular potassium concentration on the excitability of the parallel fibres of the rat cerebellum. J. Physiology 334 (1), 225–244. doi:10.1113/jphysiol.1983.sp014491
Kordower, J. H., Olanow, C. W., Dodiya, H. B., Chu, Y., Beach, T. G., Adler, C. H., et al. (2013). Disease duration and the integrity of the nigrostriatal system in Parkinson’s disease. Brain 136 (8), 2419–2431. doi:10.1093/brain/awt192
Kostuk, E. W., Cai, J., and Iacovitti, L. (2019). Subregional differences in astrocytes underlie selective neurodegeneration or protection in Parkinson's disease models in culture. Glia 67 (8), 1542–1557. doi:10.1002/glia.23627
Kucewicz, M. T., Cimbalnik, J., Matsumoto, J. Y., Brinkmann, B. H., Bower, M. R., Vasoli, V., et al. (2014). High frequency oscillations are associated with cognitive processing in human recognition memory. Brain 137 (8), 2231–2244. doi:10.1093/brain/awu149
Kucheryavykh, Y., Kucheryavykh, L., Nichols, C., Maldonado, H., Baksi, K., Reichenbach, A., et al. (2007). Downregulation of Kir4. 1 inward rectifying potassium channel subunits by RNAi impairs potassium transfer and glutamate uptake by cultured cortical astrocytes. Glia 55 (3), 274–281. doi:10.1002/glia.20455
Kucukdereli, H., Allen, N. J., Lee, A. T., Feng, A., Ozlu, M. I., Conatser, L. M., et al. (2011). Control of excitatory CNS synaptogenesis by astrocyte-secreted proteins Hevin and SPARC. Proc. Natl. Acad. Sci. 108 (32), E440–E449. doi:10.1073/pnas.1104977108
Kwak, H., Koh, W., Kim, S., Song, K., Shin, J.-I., Lee, J. M., et al. (2020). Astrocytes control sensory acuity via tonic inhibition in the thalamus. Neuron 108 (4), 691–706. doi:10.1016/j.neuron.2020.08.013
Lalo, U., Palygin, O., Rasooli-Nejad, S., Andrew, J., Haydon, P. G., and Pankratov, Y. (2014). Exocytosis of ATP from astrocytes modulates phasic and tonic inhibition in the neocortex. PLoS Biol. 12 (1), e1001747. doi:10.1371/journal.pbio.1001747
Lalo, U., Pankratov, Y., Parpura, V., and Verkhratsky, A. (2011). Ionotropic receptors in neuronal–astroglial signalling: What is the role of “excitable” molecules in non-excitable cells. Biochimica Biophysica Acta (BBA)-Molecular Cell Res. 1813 (5), 992–1002. doi:10.1016/j.bbamcr.2010.09.007
Lange, J., Gillham, O., Flower, M., Ging, H., Eaton, S., Kapadia, S., et al. (2023). PolyQ length-dependent metabolic alterations and DNA damage drive human astrocyte dysfunction in Huntington’s disease. Prog. Neurobiol. 225, 102448. doi:10.1016/j.pneurobio.2023.102448
Langer, J., Gerkau, N. J., Derouiche, A., Kleinhans, C., Moshrefi-Ravasdjani, B., Fredrich, M., et al. (2017). Rapid sodium signaling couples glutamate uptake to breakdown of ATP in perivascular astrocyte endfeet. Glia 65 (2), 293–308. doi:10.1002/glia.23092
Langer, J., Stephan, J., Theis, M., and Rose, C. R. (2012). Gap junctions mediate intercellular spread of sodium between hippocampal astrocytes in situ. Glia 60 (2), 239–252. doi:10.1002/glia.21259
Larsen, B. R., and MacAulay, N. (2014). Kir4 1-mediated spatial buffering of K+: Experimental challenges in determination of its temporal and quantitative contribution to K+ clearance in the brain. Channels 8 (6), 544–550. doi:10.4161/19336950.2014.970448
Lawal, O., Ulloa Severino, F. P., and Eroglu, C. (2022). The role of astrocyte structural plasticity in regulating neural circuit function and behavior. Glia 70 (8), 1467–1483. doi:10.1002/glia.24191
Le Meur, K., Mendizabal-Zubiaga, J., Grandes, P., and Audinat, E. (2012). GABA release by hippocampal astrocytes. Front. Comput. Neurosci. 6, 59. doi:10.3389/fncom.2012.00059
Lee, E., Jung, Y.-J., Park, Y. R., Lim, S., Choi, Y.-J., Lee, S. Y., et al. (2022a). A distinct astrocyte subtype in the aging mouse brain characterized by impaired protein homeostasis. Nat. Aging 2 (8), 726–741. doi:10.1038/s43587-022-00257-1
Lee, H.-J., Suk, J.-E., Patrick, C., Bae, E.-J., Cho, J.-H., Rho, S., et al. (2010a). Direct transfer of alpha-synuclein from neuron to astroglia causes inflammatory responses in synucleinopathies. J. Biol. Chem. 285 (12), 9262–9272. doi:10.1074/jbc.M109.081125
Lee, H. S., Ghetti, A., Pinto-Duarte, A., Wang, X., Dziewczapolski, G., Galimi, F., et al. (2014). Astrocytes contribute to gamma oscillations and recognition memory. Proc. Natl. Acad. Sci. 111 (32), E3343–E3352. doi:10.1073/pnas.1410893111
Lee, J.-H., Kim, J.-y., Noh, S., Lee, H., Lee, S. Y., Mun, J. Y., et al. (2021). Astrocytes phagocytose adult hippocampal synapses for circuit homeostasis. Nature 590 (7847), 612–617. doi:10.1038/s41586-020-03060-3
Lee, J.-H., Kim, J., Kim, M., Shin, C.-H., and Chung, W.-S. (2022b). Astrocytic synapse elimination controls ocular dominance plasticity. bioRxiv 2022, .509193. doi:10.1101/2022.09.23.509193
Lee, J. M., Sa, M., An, H., Kim, J. M. J., Kwon, J., Yoon, B.-E., et al. (2022c). Generation of astrocyte-specific MAOB conditional knockout mouse with minimal tonic GABA inhibition. Exp. Neurobiol. 31 (3), 158–172. doi:10.5607/en22016
Lee, S., Yoon, B.-E., Berglund, K., Oh, S.-J., Park, H., Shin, H.-S., et al. (2010b). Channel-mediated tonic GABA release from glia. Science 330 (6005), 790–796. doi:10.1126/science.1184334
Lee, W., Reyes, R. C., Gottipati, M. K., Lewis, K., Lesort, M., Parpura, V., et al. (2013). Enhanced Ca2+-dependent glutamate release from astrocytes of the BACHD Huntington's disease mouse model. Neurobiol. Dis. 58, 192–199. doi:10.1016/j.nbd.2013.06.002
Lezmy, J., Arancibia-Cárcamo, I. L., Quintela-López, T., Sherman, D. L., Brophy, P. J., and Attwell, D. (2021). Astrocyte Ca2+-evoked ATP release regulates myelinated axon excitability and conduction speed. Science 374 (6565), eabh2858. doi:10.1126/science.abh2858
Li, B., Xia, M., Zorec, R., Parpura, V., and Verkhratsky, A. (2021). Astrocytes in heavy metal neurotoxicity and neurodegeneration. Brain Res. 1752, 147234. doi:10.1016/j.brainres.2020.147234
Li, J., Tang, J., Ma, J., Du, M., Wang, R., and Wu, Y. (2016a). Dynamic transition of neuronal firing induced by abnormal astrocytic glutamate oscillation. Sci. Rep. 6 (1), 32343–32410. doi:10.1038/srep32343
Li, J., Wang, R., Du, M., Tang, J., and Wu, Y. (2016b). Dynamic transition on the seizure-like neuronal activity by astrocytic calcium channel block. Chaos, Solit. Fractals 91, 702–708. doi:10.1016/j.chaos.2016.08.009
Li, T., Chen, X., Zhang, C., Zhang, Y., and Yao, W. (2019). An update on reactive astrocytes in chronic pain. J. neuroinflammation 16, 140–213. doi:10.1186/s12974-019-1524-2
Li, W.-P., Su, X.-H., Hu, N.-Y., Hu, J., Li, X.-W., Yang, J.-M., et al. (2022). Astrocytes mediate cholinergic regulation of adult hippocampal neurogenesis and memory through m1 muscarinic receptor. Biol. Psychiatry 92 (12), 984–998. doi:10.1016/j.biopsych.2022.04.019
Lievens, J.-C., Woodman, B., Mahal, A., Spasic-Boscovic, O., Samuel, D., Kerkerian-Le Goff, L., et al. (2001). Impaired glutamate uptake in the R6 Huntington's disease transgenic mice. Neurobiol. Dis. 8 (5), 807–821. doi:10.1006/nbdi.2001.0430
Limbad, C., Oron, T. R., Alimirah, F., Davalos, A. R., Tracy, T. E., Gan, L., et al. (2020). Astrocyte senescence promotes glutamate toxicity in cortical neurons. PloS one 15 (1), e0227887. doi:10.1371/journal.pone.0227887
Lines, J., Martin, E. D., Kofuji, P., Aguilar, J., and Araque, A. (2020). Astrocytes modulate sensory-evoked neuronal network activity. Nat. Commun. 11 (1), 3689–3712. doi:10.1038/s41467-020-17536-3
Liu, C., Han, T., Xu, Z., Liu, J., Zhang, M., Du, J., et al. (2022). Modulating gamma oscillations promotes brain connectivity to improve cognitive impairment. Cereb. Cortex 32 (12), 2644–2656. doi:10.1093/cercor/bhab371
Longuemare, M., Rose, C., Farrell, K., Ransom, B., Waxman, S., and Swanson, R. (1999). K+-induced reversal of astrocyte glutamate uptake is limited by compensatory changes in intracellular Na+. Neuroscience 93 (1), 285–292. doi:10.1016/s0306-4522(99)00152-9
Luo, T., and Gao, T.-M. (2021). A novel phagocytic role of astrocytes in activity-dependent elimination of mature excitatory synapses. Neurosci. Bull. 37 (8), 1256–1259. doi:10.1007/s12264-021-00690-z
Ma, B., Buckalew, R., Du, Y., Kiyoshi, C. M., Alford, C. C., Wang, W., et al. (2016). Gap junction coupling confers isopotentiality on astrocyte syncytium. Glia 64 (2), 214–226. doi:10.1002/glia.22924
MacDonald, M. E., Ambrose, C. M., Duyao, M. P., Myers, R. H., Lin, C., Srinidhi, L., et al. (1993). A novel gene containing a trinucleotide repeat that is expanded and unstable on Huntington's disease chromosomes. The Huntington's Disease Collaborative Research Group. Cell 72 (6), 971–983. doi:10.1016/0092-8674(93)90585-e
Madill, M., McDonagh, K., Ma, J., Vajda, A., McLoughlin, P., O’Brien, T., et al. (2017). Amyotrophic lateral sclerosis patient iPSC-derived astrocytes impair autophagy via non-cell autonomous mechanisms. Mol. Brain 10 (1), 22–12. doi:10.1186/s13041-017-0300-4
Madji Hounoum, B., Mavel, S., Coque, E., Patin, F., Vourc'h, P., Marouillat, S., et al. (2017). Wildtype motoneurons, ALS-Linked SOD1 mutation and glutamate profoundly modify astrocyte metabolism and lactate shuttling. Glia 65 (4), 592–605. doi:10.1002/glia.23114
Magi, S., Piccirillo, S., Amoroso, S., and Lariccia, V. (2019). Excitatory amino acid transporters (EAATs): Glutamate transport and beyond. Int. J. Mol. Sci. 20 (22), 5674. doi:10.3390/ijms20225674
Makovkin, S. Y., Shkerin, I., Gordleeva, S. Y., and Ivanchenko, M. (2020). Astrocyte-induced intermittent synchronization of neurons in a minimal network. Chaos, Solit. Fractals 138, 109951. doi:10.1016/j.chaos.2020.109951
Mariotti, L., Losi, G., Sessolo, M., Marcon, I., and Carmignoto, G. (2016). The inhibitory neurotransmitter GABA evokes long-lasting C a2+ oscillations in cortical astrocytes. Glia 64 (3), 363–373. doi:10.1002/glia.22933
Matos, M., Bosson, A., Riebe, I., Reynell, C., Vallée, J., Laplante, I., et al. (2018). Astrocytes detect and upregulate transmission at inhibitory synapses of somatostatin interneurons onto pyramidal cells. Nat. Commun. 9 (1), 4254–4315. doi:10.1038/s41467-018-06731-y
Mazzetti, S., Barichella, M., Giampietro, F., Giana, A., Calogero, A. M., Amadeo, A., et al. (2022). Astrocytes expressing Vitamin D-activating enzyme identify Parkinson’s disease. CNS Neurosci. Ther. 28 (5), 703–713. doi:10.1111/cns.13801
Mederos, S., Sanchez-Puelles, C., Esparza, J., Valero, M., Ponomarenko, A., and Perea, G. (2021). GABAergic signaling to astrocytes in the prefrontal cortex sustains goal-directed behaviors. Nat. Neurosci. 24 (1), 82–92. doi:10.1038/s41593-020-00752-x
Meier, S. D., Kafitz, K. W., and Rose, C. R. (2008). Developmental profile and mechanisms of GABA-induced calcium signaling in hippocampal astrocytes. Glia 56 (10), 1127–1137. doi:10.1002/glia.20684
Miyazaki, T., Yamasaki, M., Hashimoto, K., Kohda, K., Yuzaki, M., Shimamoto, K., et al. (2017). Glutamate transporter GLAST controls synaptic wrapping by Bergmann glia and ensures proper wiring of Purkinje cells. Proc. Natl. Acad. Sci. 114 (28), 7438–7443. doi:10.1073/pnas.1617330114
Morales, I., Sanchez, A., Rodriguez-Sabate, C., and Rodriguez, M. (2017). Striatal astrocytes engulf dopaminergic debris in Parkinson's disease: A study in an animal model. PloS one 12 (10), e0185989. doi:10.1371/journal.pone.0185989
Morizawa, Y. M., Matsumoto, M., Nakashima, Y., Endo, N., Aida, T., Ishikane, H., et al. (2022). Synaptic pruning through glial synapse engulfment upon motor learning. Nat. Neurosci. 25, 1458–1469. doi:10.1038/s41593-022-01184-5
Morley, J. E., Farr, S. A., and Nguyen, A. D. (2018). Alzheimer disease. Clin. Geriatr. Med. 34 (4), 591–601. doi:10.1016/j.cger.2018.06.006
Moshrefi-Ravasdjani, B., Hammel, E. L., Kafitz, K. W., and Rose, C. R. (2017). Astrocyte sodium signalling and panglial spread of sodium signals in brain white matter. Neurochem. Res. 42 (9), 2505–2518. doi:10.1007/s11064-017-2197-9
Nagai, J., Bellafard, A., Qu, Z., Yu, X., Ollivier, M., Gangwani, M. R., et al. (2021). Specific and behaviorally consequential astrocyte Gq GPCR signaling attenuation in vivo with iβARK. Neuron 109 (14), 2256–2274.e9. doi:10.1016/j.neuron.2021.05.023
Nagai, M., Re, D. B., Nagata, T., Chalazonitis, A., Jessell, T. M., Wichterle, H., et al. (2007). Astrocytes expressing ALS-linked mutated SOD1 release factors selectively toxic to motor neurons. Nat. Neurosci. 10 (5), 615–622. doi:10.1038/nn1876
Nanclares, C., Poynter, J., Martell-Martinez, H. A., Vermilyea, S., Araque, A., Kofuji, P., et al. (2023). Dysregulation of astrocytic Ca2+ signaling and gliotransmitter release in mouse models of α-synucleinopathies. Acta Neuropathol. 145, 597–610. doi:10.1007/s00401-023-02547-3
Nedergaard, M. (1994). Direct signaling from astrocytes to neurons in cultures of mammalian brain cells. Science 263 (5154), 1768–1771. doi:10.1126/science.8134839
Newman, E. A. (2003). New roles for astrocytes: Regulation of synaptic transmission. Trends Neurosci. 26 (10), 536–542. doi:10.1016/S0166-2236(03)00237-6
Octeau, J. C., Chai, H., Jiang, R., Bonanno, S. L., Martin, K. C., and Khakh, B. S. (2018). “An optical neuron-astrocyte proximity assay at synaptic distance scales,” in Neuron (USA: Elsevier Inc.).
Oh, S.-J., Han, K.-S., Park, H., Kim, H. Y., Traynelis, S. F., Lee, C. J., et al. (2012). Protease activated receptor 1-induced glutamate release in cultured astrocytes is mediated by Bestrophin-1 channel but not by vesicular exocytosis. Mol. Brain 5 (1), 38–39. doi:10.1186/1756-6606-5-38
Oka, M., Wada, M., Wu, Q., Yamamoto, A., and Fujita, T. (2006). Functional expression of metabotropic GABAB receptors in primary cultures of astrocytes from rat cerebral cortex. Biochem. biophysical Res. Commun. 341 (3), 874–881. doi:10.1016/j.bbrc.2006.01.039
Orkand, R., Nicholls, J., and Kuffler, S. (1966). Effect of nerve impulses on the membrane potential of glial cells in the central nervous system of amphibia. J. neurophysiology 29 (4), 788–806. doi:10.1152/jn.1966.29.4.788
Ortinski, P. I., Dong, J., Mungenast, A., Yue, C., Takano, H., Watson, D. J., et al. (2010). Selective induction of astrocytic gliosis generates deficits in neuronal inhibition. Nat. Neurosci. 13 (5), 584–591. doi:10.1038/nn.2535
Ouali Alami, N., Schurr, C., Olde Heuvel, F., Tang, L., Li, Q., Tasdogan, A., et al. (2018). NF-κB activation in astrocytes drives a stage-specific beneficial neuroimmunological response in ALS. EMBO J. 37 (16), e98697. doi:10.15252/embj.201798697
Padmashri, R., Suresh, A., Boska, M. D., and Dunaevsky, A. (2015). Motor-skill learning is dependent on astrocytic activity. Neural plast. 2015, 1. doi:10.1155/2015/938023
Panatier, A., Vallée, J., Haber, M., Murai, K. K., Lacaille, J.-C., and Robitaille, R. (2011). Astrocytes are endogenous regulators of basal transmission at central synapses. Cell 146 (5), 785–798. doi:10.1016/j.cell.2011.07.022
Pankratova, E. V., Kalyakulina, A. I., Stasenko, S. V., Gordleeva, S. Y., Lazarevich, I. A., and Kazantsev, V. B. (2019). Neuronal synchronization enhanced by neuron–astrocyte interaction. Nonlinear Dyn. 97, 647–662. doi:10.1007/s11071-019-05004-7
Papouin, T., Dunphy, J., Tolman, M., Foley, J. C., and Haydon, P. G. (2017). Astrocytic control of synaptic function. Philosophical Trans. R. Soc. B Biol. Sci. 372 (1715), 20160154. doi:10.1098/rstb.2016.0154
Park, H., Han, K.-S., Seo, J., Lee, J., Dravid, S. M., Woo, J., et al. (2015). Channel-mediated astrocytic glutamate modulates hippocampal synaptic plasticity by activating postsynaptic NMDA receptors. Mol. Brain 8 (1), 7–16. doi:10.1186/s13041-015-0097-y
Parpura, V., Basarsky, T. A., Liu, F., Jeftinija, K., Jeftinija, S., and Haydon, P. G. (1994). Glutamate-mediated astrocyte–neuron signalling. Nature 369 (6483), 744–747. doi:10.1038/369744a0
Parpura, V., and Verkhratsky, A. (2012). Homeostatic function of astrocytes: Ca2+ and Na+ signalling. Transl. Neurosci. 3 (4), 334–344. doi:10.2478/s13380-012-0040-y
Pascual, O., Casper, K. B., Kubera, C., Zhang, J., Revilla-Sanchez, R., Sul, J.-Y., et al. (2005). Astrocytic purinergic signaling coordinates synaptic networks. Science 310 (5745), 113–116. doi:10.1126/science.1116916
Patel, M. R., and Weaver, A. M. (2021). Astrocyte-derived small extracellular vesicles promote synapse formation via fibulin-2-mediated TGF-β signaling. Cell Rep. 34 (10), 108829. doi:10.1016/j.celrep.2021.108829
Patton, A. P., Smyllie, N. J., Chesham, J. E., and Hastings, M. H. (2022). Astrocytes sustain circadian oscillation and bidirectionally determine circadian period, but do not regulate circadian phase in the suprachiasmatic nucleus. J. Neurosci. 42 (28), 5522–5537. doi:10.1523/JNEUROSCI.2337-21.2022
Paulsen, J. S., Ready, R., Hamilton, J., Mega, M., and Cummings, J. (2001). Neuropsychiatric aspects of Huntington's disease. J. Neurology, Neurosurg. Psychiatry 71 (3), 310–314. doi:10.1136/jnnp.71.3.310
Perea, G., Gómez, R., Mederos, S., Covelo, A., Ballesteros, J. J., Schlosser, L., et al. (2016). Activity-dependent switch of GABAergic inhibition into glutamatergic excitation in astrocyte-neuron networks. Elife 5, e20362. doi:10.7554/eLife.20362
Petravicz, J., Fiacco, T. A., and McCarthy, K. D. (2008). Loss of IP3 receptor-dependent Ca2+ increases in hippocampal astrocytes does not affect baseline CA1 pyramidal neuron synaptic activity. J. Neurosci. 28 (19), 4967–4973. doi:10.1523/JNEUROSCI.5572-07.2008
Pirttimaki, T. M., Sims, R. E., Saunders, G., Antonio, S. A., Codadu, N. K., and Parri, H. R. (2017). Astrocyte-mediated neuronal synchronization properties revealed by false gliotransmitter release. J. Neurosci. 37 (41), 9859–9870. doi:10.1523/JNEUROSCI.2761-16.2017
Pita-Almenar, J. D., Zou, S., Colbert, C. M., and Eskin, A. (2012). Relationship between increase in astrocytic GLT-1 glutamate transport and late-LTP. Learn. Mem. 19 (12), 615–626. doi:10.1101/lm.023259.111
Polykretis, I., Ivanov, V., and Michmizos, K. P. (2018). “A Neural-Astrocytic Network Architecture: Astrocytic calcium waves modulate synchronous neuronal activity,” in Proceedings of the International Conference on Neuromorphic Systems, New York, July 2018 (IEEE), 1–8.
Poskanzer, K. E., and Yuste, R. (2016). Astrocytes regulate cortical state switching in vivo. Proc. Natl. Acad. Sci. 113 (19), E2675–E2684. doi:10.1073/pnas.1520759113
Poskanzer, K. E., and Yuste, R. (2011). Astrocytic regulation of cortical UP states. Proc. Natl. Acad. Sci. 108 (45), 18453–18458. doi:10.1073/pnas.1112378108
Ramos-Gonzalez, P., Mato, S., Chara, J. C., Verkhratsky, A., Matute, C., and Cavaliere, F. (2021). Astrocytic atrophy as a pathological feature of Parkinson’s disease with LRRK2 mutation. npj Parkinson's Dis. 7 (1), 31. doi:10.1038/s41531-021-00175-w
Rannikko, E. H., Weber, S. S., and Kahle, P. J. (2015). Exogenous α-synuclein induces toll-like receptor 4 dependent inflammatory responses in astrocytes. BMC Neurosci. 16 (1), 57–11. doi:10.1186/s12868-015-0192-0
Ransom, B. (1996). “Do glial gap junctions play a role in extracellular ion homeostasis?,” in Gap Junctions in the nervous system. Editors D. C. Spray, R. Dermietzel, and Tx Austin (R.G. Landes Company), USA, 159–173.
Rasmussen, R., Nicholas, E., Petersen, N. C., Dietz, A. G., Xu, Q., Sun, Q., et al. (2019). Cortex-wide changes in extracellular potassium ions parallel brain state transitions in awake behaving mice. Cell Rep. 28 (5), 1182–1194. doi:10.1016/j.celrep.2019.06.082
Reichenbach, N., Delekate, A., Plescher, M., Schmitt, F., Krauss, S., Blank, N., et al. (2019). Inhibition of Stat3-mediated astrogliosis ameliorates pathology in an Alzheimer's disease model. EMBO Mol. Med. 11 (2), e9665. doi:10.15252/emmm.201809665
Ren, C., He, K.-J., Hu, H., Zhang, J.-B., Dong, L.-G., Li, D., et al. (2022). Induction of Parkinsonian-like changes via targeted downregulation of astrocytic glutamate transporter GLT-1 in the striatum. J. Parkinson's Dis. 12 (1), 295–314. doi:10.3233/JPD-212640
Reyes, R. C., Verkhratsky, A., and Parpura, V. (2012). Plasmalemmal Na+/Ca2+ exchanger modulates Ca2+-dependent exocytotic release of glutamate from rat cortical astrocytes. ASN neuro 4 (1), e00075. doi:10.1042/AN20110059
Ribak, C. E., Tong, W. M., and Brecha, N. C. (1996). GABA plasma membrane transporters, GAT-1 and GAT-3, display different distributions in the rat hippocampus. J. Comp. Neurology 367 (4), 595–606. doi:10.1002/(SICI)1096-9861(19960415)367:4<595::AID-CNE9>3.0.CO;2-#
Rimmele, T. S., Rocher, A.-B., Wellbourne-Wood, J., and Chatton, J.-Y. (2017). Control of glutamate transport by extracellular potassium: Basis for a negative feedback on synaptic transmission. Cereb. Cortex 27 (6), 3272–3283. doi:10.1093/cercor/bhx078
Risher, W. C., Patel, S., Kim, I. H., Uezu, A., Bhagat, S., Wilton, D. K., et al. (2014). Astrocytes refine cortical connectivity at dendritic spines. Elife 3, e04047. doi:10.7554/eLife.04047
Romanos, J., Benke, D., Saab, A. S., Zeilhofer, H. U., and Santello, M. (2019). Differences in glutamate uptake between cortical regions impact neuronal NMDA receptor activation. Commun. Biol. 2 (1), 127–215. doi:10.1038/s42003-019-0367-9
Rose, C. R., and Karus, C. (2013). Two sides of the same coin: Sodium homeostasis and signaling in astrocytes under physiological and pathophysiological conditions. Glia 61 (8), 1191–1205. doi:10.1002/glia.22492
Rose, C. R., Ziemens, D., and Verkhratsky, A. (2020a). On the special role of NCX in astrocytes: Translating Na+-transients into intracellular Ca2+ signals. Cell Calcium 86, 102154. doi:10.1016/j.ceca.2019.102154
Rose, J., Brian, C., Pappa, A., Panayiotidis, M. I., and Franco, R. (2020b). Mitochondrial metabolism in astrocytes regulates brain bioenergetics, neurotransmission and redox balance. Front. Neurosci. 14, 536682. doi:10.3389/fnins.2020.536682
Rothstein, J. D., Dykes-Hoberg, M., Pardo, C. A., Bristol, L. A., Jin, L., Kuncl, R. W., et al. (1996). Knockout of glutamate transporters reveals a major role for astroglial transport in excitotoxicity and clearance of glutamate. Neuron 16 (3), 675–686. doi:10.1016/s0896-6273(00)80086-0
Rothstein, J. D., Van Kammen, M., Levey, A. I., Martin, L. J., and Kuncl, R. W. (1995). Selective loss of glial glutamate transporter GLT-1 in amyotrophic lateral sclerosis. Ann. Neurology Official J. Am. Neurological Assoc. Child Neurology Soc. 38 (1), 73–84. doi:10.1002/ana.410380114
Sakatani, S., Seto-Ohshima, A., Shinohara, Y., Yamamoto, Y., Yamamoto, H., Itohara, S., et al. (2008). Neural-activity-dependent release of S100B from astrocytes enhances kainate-induced gamma oscillations In Vivo. J. Neurosci. 28 (43), 10928–10936.
Santello, M., Toni, N., and Volterra, A. (2019). Astrocyte function from information processing to cognition and cognitive impairment. Nat. Neurosci. 22 (2), 154–166. doi:10.1038/s41593-018-0325-8
Santello, M., and Volterra, A. (2009). Synaptic modulation by astrocytes via Ca2+-dependent glutamate release. Neuroscience 158 (1), 253–259. doi:10.1016/j.neuroscience.2008.03.039
Sarantis, M., and Attwell, D. (1990). Glutamate uptake in mammalian retinal glia is voltage-and potassium-dependent. Brain Res. 516 (2), 322–325. doi:10.1016/0006-8993(90)90935-5
Sardinha, V. M., Guerra-Gomes, S., Caetano, I., Tavares, G., Martins, M., Reis, J. S., et al. (2017). Astrocytic signaling supports hippocampal–prefrontal theta synchronization and cognitive function. Glia 65 (12), 1944–1960. doi:10.1002/glia.23205
Sasaki, T., Ishikawa, T., Abe, R., Nakayama, R., Asada, A., Matsuki, N., et al. (2014). Astrocyte calcium signalling orchestrates neuronal synchronization in organotypic hippocampal slices. J. physiology 592 (13), 2771–2783. doi:10.1113/jphysiol.2014.272864
Sasaki, T., Matsuki, N., and Ikegaya, Y. (2011). Action-potential modulation during axonal conduction. Science 331 (6017), 599–601. doi:10.1126/science.1197598
Schousboe, A., Hertz, L., and Svenneby, G. (1977). Uptake and metabolism of GABA in astrocytes cultured from dissociated mouse brain hemispheres. Neurochem. Res. 2 (2), 217–229. doi:10.1007/BF00964098
Shen, W., Li, Z., Tang, Y., Han, P., Zhu, F., Dong, J., et al. (2022). Somatostatin interneurons inhibit excitatory transmission mediated by astrocytic GABAB and presynaptic GABAB and adenosine A1 receptors in the hippocampus. J. Neurochem. 163 (4), 310–326. doi:10.1111/jnc.15662
Shen, Y., Wu, S. Y., Rancic, V., Aggarwal, A., Qian, Y., Miyashita, S. I., et al. (2019). Genetically encoded fluorescent indicators for imaging intracellular potassium ion concentration. Commun. Biol. 2, 18. doi:10.1038/s42003-018-0269-2
Sherwood, M. W., Arizono, M., Hisatsune, C., Bannai, H., Ebisui, E., Sherwood, J. L., et al. (2017). Astrocytic IP3Rs: Contribution to Ca2+ signalling and hippocampal LTP. Glia 65 (3), 502–513. doi:10.1002/glia.23107
Shibasaki, K., Ikenaka, K., Tamalu, F., Tominaga, M., and Ishizaki, Y. (2014). A novel subtype of astrocytes expressing TRPV4 (transient receptor potential vanilloid 4) regulates neuronal excitability via release of gliotransmitters. J. Biol. Chem. 289 (21), 14470–14480. doi:10.1074/jbc.M114.557132
Shigetomi, E., Bowser, D. N., Sofroniew, M. V., and Khakh, B. S. (2008). Two forms of astrocyte calcium excitability have distinct effects on NMDA receptor-mediated slow inward currents in pyramidal neurons. J. Neurosci. 28 (26), 6659–6663. doi:10.1523/JNEUROSCI.1717-08.2008
Shigetomi, E., Saito, K., Sano, F., and Koizumi, S. (2019). Aberrant calcium signals in reactive astrocytes: A key process in neurological disorders. Int. J. Mol. Sci. 20 (4), 996. doi:10.3390/ijms20040996
Shim, H. S., Park, H. J., Woo, J., Lee, C. J., and Shim, I. (2019). Role of astrocytic GABAergic system on inflammatory cytokine-induced anxiety-like behavior. Neuropharmacology 160, 107776. doi:10.1016/j.neuropharm.2019.107776
Shimizu, H., Watanabe, E., Hiyama, T. Y., Nagakura, A., Fujikawa, A., Okado, H., et al. (2007). Glial Nax channels control lactate signaling to neurons for brain [Na+] sensing. Neuron 54 (1), 59–72. doi:10.1016/j.neuron.2007.03.014
Shin, J.-Y., Fang, Z.-H., Yu, Z.-X., Wang, C.-E., Li, S.-H., and Li, X.-J. (2005). Expression of mutant huntingtin in glial cells contributes to neuronal excitotoxicity. J. Cell Biol. 171 (6), 1001–1012. doi:10.1083/jcb.200508072
Shlosberg, D., Buskila, Y., Abu-Ghanem, Y., and Amitai, Y. (2012). Spatiotemporal alterations of cortical network activity by selective loss of NOS-expressing interneurons. Front. Neural Circuits 6, 3. doi:10.3389/fncir.2012.00003
Shlosberg, D., Patrick, S. L., Buskila, Y., and Amitai, Y. (2003). Inhibitory effect of mouse neocortex layer I on the underlying cellular network. Eur. J. Neurosci. 18 (10), 2751–2759. doi:10.1111/j.1460-9568.2003.03016.x
Sibille, J., Dao Duc, K., Holcman, D., and Rouach, N. (2015). The neuroglial potassium cycle during neurotransmission: Role of Kir4. 1 channels. PLoS Comput. Biol. 11 (3), e1004137. doi:10.1371/journal.pcbi.1004137
Sibille, J., Pannasch, U., and Rouach, N. (2014). Astroglial potassium clearance contributes to short-term plasticity of synaptically evoked currents at the tripartite synapse. J. physiology 592 (1), 87–102. doi:10.1113/jphysiol.2013.261735
Sicot, G., Servais, L., Dinca, D. M., Leroy, A., Prigogine, C., Medja, F., et al. (2017). Downregulation of the glial GLT1 glutamate transporter and purkinje cell dysfunction in a mouse model of myotonic dystrophy. Cell Rep. 19 (13), 2718–2729. doi:10.1016/j.celrep.2017.06.006
Simard, M., and Nedergaard, M. (2004). The neurobiology of glia in the context of water and ion homeostasis. Neuroscience 129 (4), 877–896. doi:10.1016/j.neuroscience.2004.09.053
Simpson, J., Ince, P., Lace, G., Forster, G., Shaw, P., Matthews, F., et al. (2010). Astrocyte phenotype in relation to Alzheimer-type pathology in the ageing brain. Neurobiol. aging 31 (4), 578–590. doi:10.1016/j.neurobiolaging.2008.05.015
Sobieski, C., Jiang, X., Crawford, D. C., and Mennerick, S. (2015). Loss of local astrocyte support disrupts action potential propagation and glutamate release synchrony from unmyelinated hippocampal axon terminals in vitro. J. Neurosci. 35 (31), 11105–11117. doi:10.1523/JNEUROSCI.1289-15.2015
Sonninen, T.-M., Hämäläinen, R. H., Koskuvi, M., Oksanen, M., Shakirzyanova, A., Wojciechowski, S., et al. (2020). Metabolic alterations in Parkinson’s disease astrocytes. Sci. Rep. 10 (1), 14474. doi:10.1038/s41598-020-71329-8
Stevenson, R., Samokhina, E., Rossetti, I., Morley, J. W., and Buskila, Y. (2020). Neuromodulation of glial function during neurodegeneration. Front. Cell Neurosci. 14, 278. doi:10.3389/fncel.2020.00278
Sveinbjornsdottir, S. (2016). The clinical symptoms of Parkinson's disease. J. Neurochem. 139, 318–324. doi:10.1111/jnc.13691
Szabó, Z., Héja, L., Szalay, G., Kékesi, O., Füredi, A., Szebényi, K., et al. (2017). Extensive astrocyte synchronization advances neuronal coupling in slow wave activity in vivo. Sci. Rep. 7 (1), 6018–18. doi:10.1038/s41598-017-06073-7
Tabrizi, S. J., Scahill, R. I., Owen, G., Durr, A., Leavitt, B. R., Roos, R. A., et al. (2013). Predictors of phenotypic progression and disease onset in premanifest and early-stage Huntington's disease in the TRACK-HD study: Analysis of 36-month observational data. Lancet Neurology 12 (7), 637–649. doi:10.1016/S1474-4422(13)70088-7
Takata, N., Mishima, T., Hisatsune, C., Nagai, T., Ebisui, E., Mikoshiba, K., et al. (2011). Astrocyte calcium signaling transforms cholinergic modulation to cortical plasticity in vivo. J. Neurosci. 31 (49), 18155–18165. doi:10.1523/JNEUROSCI.5289-11.2011
Takeda, I., Yoshihara, K., Cheung, D. L., Kobayashi, T., Agetsuma, M., Tsuda, M., et al. (2022). Controlled activation of cortical astrocytes modulates neuropathic pain-like behaviour. Nat. Commun. 13 (1), 4100. doi:10.1038/s41467-022-31773-8
Tanaka, M., Shih, P.-Y., Gomi, H., Yoshida, T., Nakai, J., Ando, R., et al. (2013). Astrocytic Ca2+ signals are required for the functional integrity of tripartite synapses. Mol. Brain 6 (1), 6–13. doi:10.1186/1756-6606-6-6
Tertil, M., Skupio, U., Barut, J., Dubovyk, V., Wawrzczak-Bargiela, A., Soltys, Z., et al. (2018). Glucocorticoid receptor signaling in astrocytes is required for aversive memory formation. Transl. Psychiatry 8 (1), 255. doi:10.1038/s41398-018-0300-x
Thompson, L., Khuc, J., Saccani, M. S., Zokaei, N., and Cappelletti, M. (2021). Gamma oscillations modulate working memory recall precision. Exp. Brain Res. 239, 2711–2724. doi:10.1007/s00221-021-06051-6
Timofeev, I., Bazhenov, M., Seigneur, J., and Sejnowski, T. (2012). Neuronal synchronization and thalamocortical rhythms in sleep, wake and epilepsy. Jasper's Basic Mechanisms of the Epilepsies. [Internet]. 4th edition. Bethesda (MD): National Center for Biotechnology Information (US). PMID: 22787672.
Todd, A. C., Marx, M. C., Hulme, S. R., Bröer, S., and Billups, B. (2017). SNAT3-mediated glutamine transport in perisynaptic astrocytes in situ is regulated by intracellular sodium. Glia 65 (6), 900–916. doi:10.1002/glia.23133
Tong, X., Ao, Y., Faas, G. C., Nwaobi, S. E., Xu, J., Haustein, M. D., et al. (2014). Astrocyte Kir4. 1 ion channel deficits contribute to neuronal dysfunction in Huntington's disease model mice. Nat. Neurosci. 17 (5), 694–703. doi:10.1038/nn.3691
Trabelsi, Y., Amri, M., Becq, H., Molinari, F., and Aniksztejn, L. (2017). The conversion of glutamate by glutamine synthase in neocortical astrocytes from juvenile rat is important to limit glutamate spillover and peri/extrasynaptic activation of NMDA receptors. Glia 65 (2), 401–415. doi:10.1002/glia.23099
Trudler, D., Sanz-Blasco, S., Eisele, Y. S., Ghatak, S., Bodhinathan, K., Akhtar, M. W., et al. (2021). α-Synuclein oligomers induce glutamate release from astrocytes and excessive extrasynaptic NMDAR activity in neurons, thus contributing to synapse loss. J. Neurosci. 41 (10), 2264–2273. doi:10.1523/JNEUROSCI.1871-20.2020
Tyurikova, O., Shih, P. Y., Dembitskaya, Y., Savtchenko, L. P., McHugh, T. J., Rusakov, D. A., et al. (2022). K+ efflux through postsynaptic NMDA receptors suppresses local astrocytic glutamate uptake. Glia 70 (5), 961–974. doi:10.1002/glia.24150
Unichenko, P., Myakhar, O., and Kirischuk, S. (2012). Intracellular Na+ concentration influences short-term plasticity of glutamate transporter-mediated currents in neocortical astrocytes. Glia 60 (4), 605–614. doi:10.1002/glia.22294
Uwechue, N. M., Marx, M. C., Chevy, Q., and Billups, B. (2012). Activation of glutamate transport evokes rapid glutamine release from perisynaptic astrocytes. J. physiology 590 (10), 2317–2331. doi:10.1113/jphysiol.2011.226605
Vaidyanathan, T. V., Collard, M., Yokoyama, S., Reitman, M. E., and Poskanzer, K. E. (2021). Cortical astrocytes independently regulate sleep depth and duration via separate GPCR pathways. Elife 10, e63329. doi:10.7554/eLife.63329
Valenza, M., Leoni, V., Karasinska, J. M., Petricca, L., Fan, J., Carroll, J., et al. (2010). Cholesterol defect is marked across multiple rodent models of Huntington's disease and is manifest in astrocytes. J. Neurosci. 30 (32), 10844–10850. doi:10.1523/JNEUROSCI.0917-10.2010
Valenza, M., Marullo, M., Di Paolo, E., Cesana, E., Zuccato, C., Biella, G., et al. (2015). Disruption of astrocyte-neuron cholesterol cross talk affects neuronal function in Huntington’s disease. Cell Death Differ. 22 (4), 690–702. doi:10.1038/cdd.2014.162
van Deijk, A. L. F., Camargo, N., Timmerman, J., Heistek, T., Brouwers, J. F., Mogavero, F., et al. (2017). Astrocyte lipid metabolism is critical for synapse development and function in vivo. Glia 65 (4), 670–682. doi:10.1002/glia.23120
Van Horn, M. R., and Ruthazer, E. S. (2019). Glial regulation of synapse maturation and stabilization in the developing nervous system. Curr. Opin. Neurobiol. 54, 113–119. doi:10.1016/j.conb.2018.10.002
Verkhratsky, A., and Steinhäuser, C. (2000). Ion channels in glial cells. Brain Res. Rev. 32 (2-3), 380–412. doi:10.1016/s0165-0173(99)00093-4
Verveyko, D. V., Verisokin, A. Y., Postnov, D. E., and Brazhe, A. R. (2019). "When Na modulates Ca: Nonlinear interplay between Na/Ca-exchanger and IP3-mediated Ca oscillations in astrocytes", in: Saratov fall meeting 2018: Computations and data analysis: From nanoscale Tools to brain functions: SPIE, America, 114–120.
Vezzoli, E., Cali, C., De Roo, M., Ponzoni, L., Sogne, E., Gagnon, N., et al. (2020). Ultrastructural evidence for a role of astrocytes and glycogen-derived lactate in learning-dependent synaptic stabilization. Cereb. Cortex 30 (4), 2114–2127. doi:10.1093/cercor/bhz226
Voutsinos-Porche, B., Bonvento, G., Tanaka, K., Steiner, P., Welker, E., Chatton, J.-Y., et al. (2003). Glial glutamate transporters mediate a functional metabolic crosstalk between neurons and astrocytes in the mouse developing cortex. Neuron 37 (2), 275–286. doi:10.1016/s0896-6273(02)01170-4
Wade, J. J., Breslin, K., Wong-Lin, K., Harkin, J., Flanagan, B., Van Zalinge, H., et al. (2019). Calcium microdomain formation at the perisynaptic cradle due to NCX reversal: A computational study. Front. Cell. Neurosci. 13, 185. doi:10.3389/fncel.2019.00185
Walls, A. B., Nilsen, L. H., Eyjolfsson, E. M., Vestergaard, H. T., Hansen, S. L., Schousboe, A., et al. (2010). GAD65 is essential for synthesis of GABA destined for tonic inhibition regulating epileptiform activity. J. Neurochem. 115 (6), 1398–1408. doi:10.1111/j.1471-4159.2010.07043.x
Walz, W. (2000). Role of astrocytes in the clearance of excess extracellular potassium. Neurochem. Int. 36 (4-5), 291–300. doi:10.1016/s0197-0186(99)00137-0
Wang, H., Kulas, J. A., Wang, C., Holtzman, D. M., Ferris, H. A., and Hansen, S. B. (2021). Regulation of beta-amyloid production in neurons by astrocyte-derived cholesterol. Proc. Natl. Acad. Sci. 118 (33), e2102191118. doi:10.1073/pnas.2102191118
Wang, J., Tu, J., Cao, B., Mu, L., Yang, X., Cong, M., et al. (2017). Astrocytic l-lactate signaling facilitates amygdala-anterior cingulate cortex synchrony and decision making in rats. Cell Rep. 21 (9), 2407–2418. doi:10.1016/j.celrep.2017.11.012
Wiktorowska, L., Bilecki, W., Tertil, M., Kudla, L., Szumiec, L., Mackowiak, M., et al. (2021). Knockdown of the astrocytic glucocorticoid receptor in the central nucleus of the amygdala diminishes conditioned fear expression and anxiety. Behav. Brain Res. 402, 113095. doi:10.1016/j.bbr.2020.113095
Wood, T. E., Barry, J., Yang, Z., Cepeda, C., Levine, M. S., and Gray, M. (2019). Mutant huntingtin reduction in astrocytes slows disease progression in the BACHD conditional Huntington’s disease mouse model. Hum. Mol. Genet. 28 (3), 487–500. doi:10.1093/hmg/ddy363
Yan, X.-X., and Ribak, C. E. (1998). Increased expression of GABA transporters, GAT-1 and GAT-3, in the deafferented superior colliculus of the rat. Brain Res. 783 (1), 63–76. doi:10.1016/s0006-8993(97)01157-8
Yang, Y., Song, J.-J., Choi, Y. R., Kim, S.-h., Seok, M.-J., Wulansari, N., et al. (2022). Therapeutic functions of astrocytes to treat α-synuclein pathology in Parkinson’s disease. Proc. Natl. Acad. Sci. 119 (29), e2110746119. doi:10.1073/pnas.2110746119
Yarom, Y., Grossman, Y., Gutnick, M., and Spira, M. (1982). Transient extracellular potassium accumulation produced prolonged depolarizations during synchronized bursts in picrotoxin-treated cockroach CNS. J. Neurophysiology 48 (5), 1089–1097. doi:10.1152/jn.1982.48.5.1089
Yoon, B.-E., Jo, S., Woo, J., Lee, J.-H., Kim, T., Kim, D., et al. (2011). The amount of astrocytic GABA positively correlates with the degree of tonic inhibition in hippocampal CA1 and cerebellum. Mol. Brain 4 (1), 42–47. doi:10.1186/1756-6606-4-42
Yoon, B. E., Woo, J., Chun, Y. E., Chun, H., Jo, S., Bae, J. Y., et al. (2014). Glial GABA, synthesized by monoamine oxidase B, mediates tonic inhibition. J. physiology 592 (22), 4951–4968. doi:10.1113/jphysiol.2014.278754
Yu, X., Nagai, J., and Khakh, B. S. (2020). Improved tools to study astrocytes. Nat. Rev. Neurosci. 21 (3), 121–138. doi:10.1038/s41583-020-0264-8
Zehnder, T., Petrelli, F., Romanos, J., Figueiredo, E. C. D. O., Lewis, T. L., Déglon, N., et al. (2021). Mitochondrial biogenesis in developing astrocytes regulates astrocyte maturation and synapse formation. Cell Rep. 35 (2), 108952. doi:10.1016/j.celrep.2021.108952
Zhang, Y., Meng, X., Jiao, Z., Liu, Y., Zhang, X., and Qu, S. (2020). Generation of a novel mouse model of Parkinson’s disease via targeted knockdown of glutamate transporter GLT-1 in the substantia nigra. ACS Chem. Neurosci. 11 (3), 406–417. doi:10.1021/acschemneuro.9b00609
Zhang, Y. V., Ormerod, K. G., and Littleton, J. T. (2017). Astrocyte Ca2+ influx negatively regulates neuronal activity. Eneuro 4 (2), ENEURO.0340–16.2017. doi:10.1523/ENEURO.0340-16.2017
Ziemens, D., Oschmann, F., Gerkau, N. J., and Rose, C. R. (2019). Heterogeneity of activity-induced sodium transients between astrocytes of the mouse hippocampus and neocortex: Mechanisms and consequences. J. Neurosci. 39 (14), 2620–2634. doi:10.1523/JNEUROSCI.2029-18.2019
Keywords: astrocytes, excitability, synapse, neuromodulation, oscillations
Citation: Purushotham SS and Buskila Y (2023) Astrocytic modulation of neuronal signalling. Front. Netw. Physiol. 3:1205544. doi: 10.3389/fnetp.2023.1205544
Received: 14 April 2023; Accepted: 18 May 2023;
Published: 01 June 2023.
Edited by:
Anja Scheller, Saarland University, GermanyCopyright © 2023 Purushotham and Buskila. This is an open-access article distributed under the terms of the Creative Commons Attribution License (CC BY). The use, distribution or reproduction in other forums is permitted, provided the original author(s) and the copyright owner(s) are credited and that the original publication in this journal is cited, in accordance with accepted academic practice. No use, distribution or reproduction is permitted which does not comply with these terms.
*Correspondence: Yossi Buskila, eS5idXNraWxhQHdlc3Rlcm5zeWRuZXkuZWR1LmF1