- Department of Neurobiology and Center for Neuroscience, University of Pittsburgh School of Medicine, Pittsburgh, PA, USA
Interactions between nicotinic excitatory postsynaptic potentials (EPSPs) critically determine whether paravertebral sympathetic ganglia behave as simple synaptic relays or as integrative centers that amplify preganglionic activity. Synaptic connectivity in this system is characterized by an n + 1 pattern of convergence, where each ganglion cell receives one very strong primary input and a variable number (n) of weak secondary inputs that are subthreshold in strength. To test whether pairs of secondary nicotinic EPSPs can summate to fire action potentials (APs) and thus mediate ganglionic gain in the rat superior cervical ganglion, we recorded intracellularly at 34°C and used graded presynaptic stimulation to isolate individual secondary synapses. Weak EPSPs in 40 of 53 neurons had amplitudes of 0.5–7 mV (mean 3.5 ± 0.3 mV). EPSPs evoked by paired pulse stimulation were either depressing (n = 10), facilitating (n = 9), or borderline (n = 10). In 15 of 29 cells, pairs of weak secondary EPSPs initiated spikes when elicited within a temporal window <20 ms, irrespective of EPSP amplitude or paired pulse response type. In six other neurons, we observed novel secondary EPSPs that were strong enough to straddle spike threshold without summation. At stimulus rates <1 Hz straddling EPSPs appeared suprathreshold in strength. However, their limited ability to drive firing could be blocked by the afterhyperpolarization following an AP. When viewed in a computational context, these findings support the concept that weak and straddling secondary nicotinic synapses enable mammalian sympathetic ganglia to behave as use-dependent amplifiers of preganglionic activity.
Introduction
Convergence is a cardinal feature of synaptic organization in sympathetic ganglia, with each neuron typically receiving preganglionic input from 2 to 15 nicotinic synapses (Purves et al., 1986; Wang et al., 1995). The sparse nature of ganglionic convergence resembles that of glutamatergic driver inputs to first order thalamic relay nuclei (Turner and Salt, 1998; Chen and Regehr, 2000; Guillery and Sherman, 2002; Deschenes et al., 2003). This suggests that summation between small numbers of excitatory postsynaptic potentials (EPSPs) may have similar physiological consequences in both circuits. In the present study, we assessed the ability of individual nicotinic synapses to drive activity in the rat superior cervical ganglion (SCG) at the rostral end of the paravertebral sympathetic chain. The results are discussed in terms of their implications for ganglionic integration and the analogy with thalamic relay function.
The impact of nicotinic synapses on paravertebral sympathetic neurons depends on their strength, which falls into two categories described as primary and secondary. All ganglion cells have one primary nicotinic synapse, where EPSP magnitude always exceed spike threshold, and a variable number (n) of secondary nicotinic synapses that are generally subthreshold in strength. Karila and Horn (2000) proposed that this n + 1 pattern of convergence can result in the amplification of preganglionic activity. When the gain of synaptic amplification is >1, postganglionic neurons fire faster than preganglionic neurons. A central tenet of the gain hypothesis is that summation between pairs of secondary nicotinic EPSPs can be sufficient to initiate action potentials (APs; Horn and Kullmann, 2007).
Several lines of support for coincidence detection and the gain hypothesis come from the analysis of secondary synapses in bullfrog sympathetic ganglia. First, in vivo intracellular recordings have shown that naturally occurring secondary EPSPs sometimes summate to drive APs (Ivanoff and Smith, 1995). Second, paired pulse stimulation in isolated ganglia has revealed that coincidence detection of secondary EPSPs can be effective in driving APs (Karila and Horn, 2000). A third line of evidence comes from computational simulations of a conductance-based model and dynamic clamp experiments on dissociated bullfrog neurons (Wheeler et al., 2004; Kullmann and Horn, 2006, 2010). Both approaches show that when neurons are stimulated with noisy temporal patterns of EPSPs, to mimic activity in vivo, pairs of secondary EPSPs arising through convergence of nicotinic synapses can drive postganglionic firing and thereby amplify preganglionic activity by as much as three-fold.
In this paper, we turn attention to mammalian sympathetic ganglia, where the evidence for coincidence detection of nicotinic EPSPs remains less clear. In vivo intracellular recording from the rabbit SCG (Skok and Ivanov, 1983) and rat SCG (McLachlan et al., 1997, 1998; Habler et al., 1999) has shown that naturally occurring subthreshold nicotinic EPSPs may contribute to postganglionic firing. Although consistent with the gain hypothesis, an alternative interpretation has been advanced. In this view, secondary EPSPs are too weak to produce consistent coincidence detection and therefore do not make a significant contribution to the overall level of ganglionic firing (Maslov et al., 1996; McLachlan, 2003; Janig, 2006). However, the methodology employed with in vivo recording did not allow for nerve stimulation and the direct evaluation of secondary synapses, but instead relied upon analysis of AP waveforms to distinguish spikes driven by primary EPSPs from those arising through summation of secondary EPSPs. The present experiments were done to further resolve this issue by examining the strength of secondary EPSPs evoked by minimal presynaptic stimulation of the isolated rat SCG. Our results identify conditions where pairs of secondary EPSPs can drive APs and they reveal the existence of previously unknown straddling secondary synapses that can drive firing at low rates without the need for summation.
Materials and Methods
Preparation of Ganglia
The methods for intracellular and extracellular recording from the rat SCG were as previously described (Li and Horn, 2006). Data in the present study were obtained from 32 male adult Sprague Dawley rats (180–250 g). In brief, animals were killed by CO2 inhalation, following procedures approved by the Institutional Animal Care and Use Committee at the University of Pittsburgh. Both SCGs were removed and the better specimen was desheathed and pinned out using the remaining connective tissue in a Sylgard-lined recording chamber (1.0 ml volume). For nerve stimulation and extracellular recording, suction electrodes were attached to the preganglionic cervical sympathetic trunk (CST) and the postganglionic external carotid nerve (ECN) and internal carotid nerve (ICN). After mounting the preparation and chamber on a fixed stage Zeiss WL microscope with 40× water immersion objective and Nomarski DIC, the ganglion was superfused with bicarbonate-buffered Ringer solution at a rate of 2 ml min−1 using a peristaltic pump. The preparation was maintained at 33–35°C using an inline heater (Warner Instruments, Hamden, CT, USA). Ringer solution contained (in mM): 124 NaCl, 4 KCl, 25.7 NaHCO3, 1.25 NaH2PO4, 2.45 CaCl2, 1.2 MgSO4, 11 glucose, 0.15 ascorbic acid and equilibrated with carbogen gas.
Electrophysiological Recordings and Data Analysis
Intracellular recordings were made with glass microelectrodes filled with 3 M K acetate (80–120 MΩ) and an Axoclamp 2B conventional bridge amplifier with filtering set to 10 kHz (Molecular Devices, Sunnyvale, CA, USA). Extracellular compound APs (CAPs) were recorded from the ECN and ICN using AC coupled differential amplifiers (Grass P55, West Warwick, RI, USA). Intracellular and extracellular records were digitized at 10 kHz using a Digidata 1440A interface with pClamp 10.1 software (Molecular Devices) and a personal computer.
Only neurons with resting membrane potentials more negative than −45 mV and overshooting (>0 mV) APs were included in the analysis. The preganglionic CST was stimulated with brief voltage pulses (100 μs, 5–50 V). After recording the supramaximal synaptic response, stimulus amplitude was decreased incrementally to ascertain the threshold level for synaptic activation and to isolate weak EPSPs.
All grouped data are expressed as the mean ± SE. Statistical comparisons were done using a one-way ANOVA followed by tests for multiple comparisons and P < 0.05 as the criterion for significance (Instat, Graphpad, San Diego, CA, USA).
Results
Isolation of Primary and Secondary EPSPs
Weak EPSPs arising from secondary synapses were isolated in 40 of 53 sympathetic neurons by applying minimal stimulation to the presynaptic CST. All recordings were done at 33–35°C to approximate conditions in vivo. Figure 1A illustrates an example in which graded stimuli of increasing amplitude recruited four inputs to a cell – two secondary synapses followed by the primary synapse and finally a third secondary synapse. Each input had a distinct stimulus threshold and latency. In this example, all of the synaptic inputs were recruited in concert with high threshold components of the postsynaptic extracellular CAPs, which were recorded simultaneously from the ICN and the ECN. Using the classification scheme of Li and Horn (2006), this cell would therefore be identified as a high threshold rat SCG neuron and likely to be either vasomotor or pilomotor in function. Note also that synapse 4 (Figure 1A) introduced a large depolarizing component into the spike afterhyperpolarization (AHP) and that this EPSP was strong enough to shunt the AP amplitude.
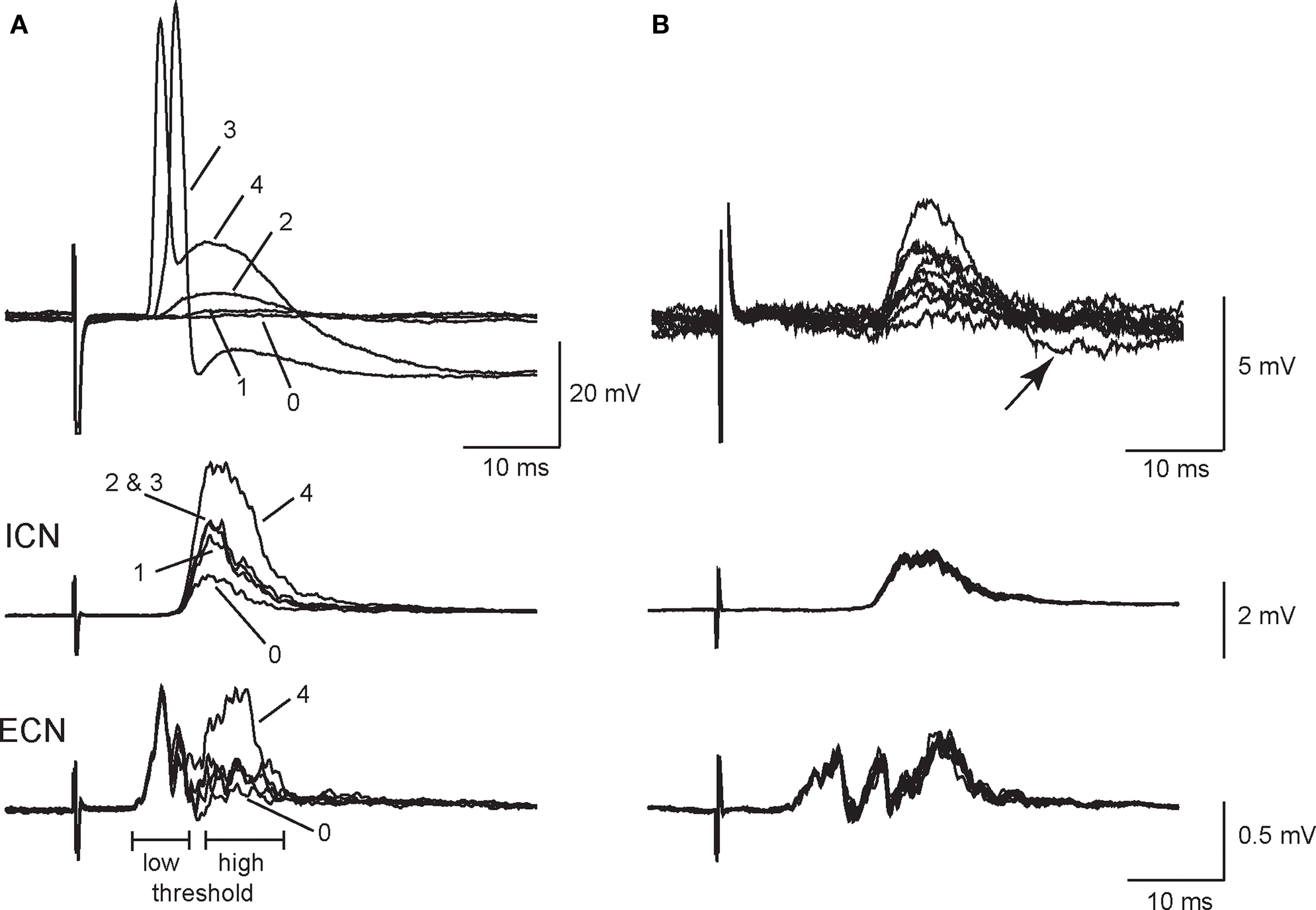
Figure 1. Primary and secondary synapses on SCG neurons have distinct presynaptic stimulus thresholds. (A) Intracellular recording from an SCG neuronal cell body (top) together with simultaneous extracellular recordings from the internal carotid nerve (middle), and the external carotid nerve (bottom) show that the selective recruitment of four synaptic components (1–4) correlated with activation of high threshold components in postsynaptic compound action potentials (CAPs). Responses were evoked with graded presynaptic stimuli of increasing strength. (B) Additional intracellular recordings (top) from synapse number 2 in the same cell after reversing stimulus polarity to improve isolation show that EPSP amplitudes at synapse 2 fluctuated during repeated stimulation. Note, however, that the corresponding population responses recorded from the ICN (middle) and ECN (bottom) remained stable. In addition, one can see an undershoot (arrow) activated by the largest intracellular EPSP (B, top).
When evoked at frequencies below 0.5 Hz, secondary EPSP amplitudes fluctuated from trial to trial with occasional failures (Figure 1B). In this particular example, we inverted the stimulus polarity to give better isolation of the synapse labeled 2 in Figure 1A. This also caused an increase in the latency of the response. However, it also shows that the extracellular ICN and ECN recordings were very stable, which indicates the intracellular EPSP fluctuations were synaptic in origin and not due to changes in the efficacy of stimulation. In 34 neurons, secondary EPSPs were 3.5 ± 0.3 mV in amplitude (range 0.5–7 mV) and the resting potential (Vrest) was −58.6 ± 2.2 mV. Larger EPSPs often had undershoots (Figure 1B), which indicates they activated a voltage-dependent K+ conductance and/or a Ca2+-dependent K+ conductance (Tokimasa et al., 1983; Kullmann et al., 2004).
As in previous work (Skok and Ivanov, 1983; Hirst and McLachlan, 1984; McLachlan et al., 1998; Karila and Horn, 2000), the disparity in strength between primary and secondary synapses was evident from the relative magnitudes of their associated EPSPs and the high safety factor for AP initiation by primary EPSPs. Injecting current to hyperpolarize the resting potential increased EPSP amplitude at primary and secondary synapses (Figure 2) and in some cases blocked AP generation by primary EPSPs (Figure 2B).
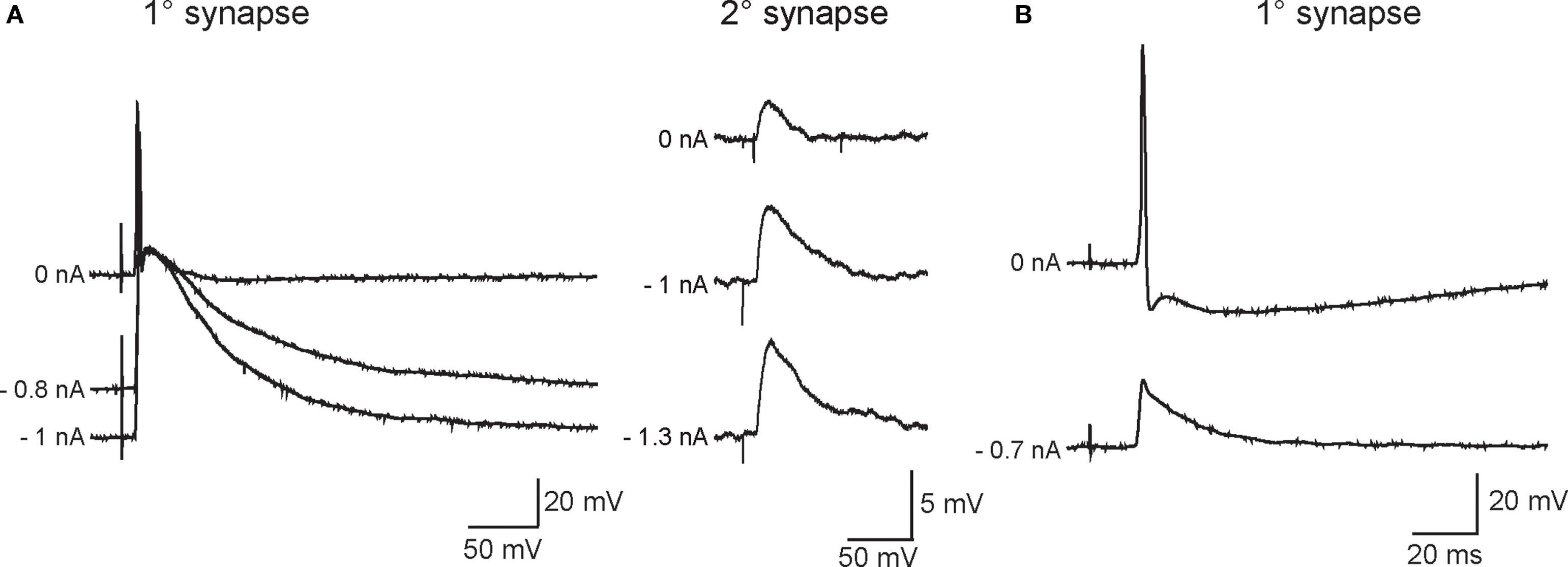
Figure 2. Varying the membrane potential reveals that primary and secondary EPSPs differ in magnitude and strength. (A) Example of a neuron in which primary (left) and secondary (right) EPSP amplitudes increased as the driving force on nicotinic currents was increased by injecting hyperpolarizing current through the recording electrode (noted at the left of each trace). This cell was typical of most recordings in that strong hyperpolarization was unable to block firing of an action potential by the primary EPSP. (B) Example of a neuron where the primary synaptic response was recruited with relatively low stimulus strength and obscured the ability to resolve secondary EPSPs with stronger stimuli. In this cell, hyperpolarization was able to block firing and revealed a 20-mV primary EPSP.
In cells where the primary synapse had a higher stimulus threshold than secondary synapses (Figure 1), the strength of the primary synapse was augmented by concomitant activation of secondary synapses. This effect leads to an overestimate of primary synaptic strength. However, in 13 cells where secondary synapses could not be isolated, we believe this was because the primary synapse had a lower presynaptic stimulus threshold than secondary synapses. Such cells afforded the opportunity to study primary synapses in isolation. Although hyperpolarizing the resting potential blocked spike initiation in the example shown in Figure 2B, this was often not the case in cells where graded stimulation recruited the primary synapse before secondary synapses. The observations of relative EPSP amplitudes support the idea that primary and secondary synapses differ greatly in strength due to a large disparity in their underlying synaptic currents.
The Homosynaptic Window of Summation Between Pairs of Weak Secondary EPSPs
When pairs of secondary EPSPs were evoked at intervals between 10 and 20 ms they were sufficient to drive APs in 15 of 29 neurons tested. Figure 3A illustrates a cell in which suprathreshold summation occurred at 10, 15, and 20 ms, but not 30 ms. One can also see from the repeated trials in these records that paired pulse stimulation became more effective in generating spikes at shorter intervals. At the 10 ms interval, over half the trials led to postsynaptic firing. Although the response profiles of individual cells varied (Figure 3B), the proportion of suprathreshold responses was consistently higher at the shorter interpulse intervals and fell to 0 at 30 ms. Analysis of the averaged data showed the proportion of suprathreshold summation was significantly higher at the 10 ms interval than at 15 or 20 ms. These data indicate the window of summation for spike generation by pairs of secondary EPSPs is between 10 and 20 ms.
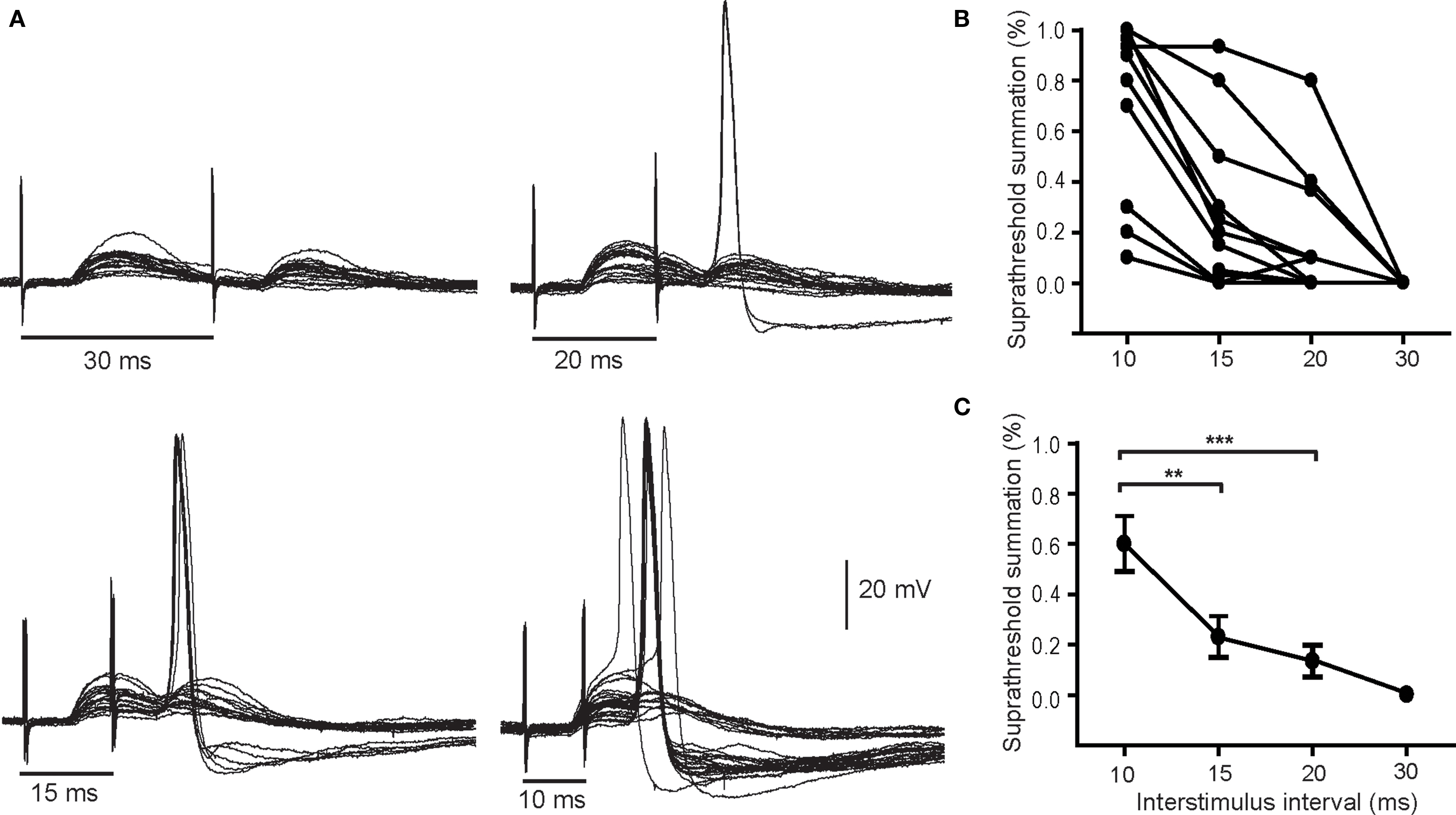
Figure 3. Pairs of secondary EPSPs can drive action potentials. (A) Example of a neuron where the paired pulse interval was varied from 30 to 10 ms shows that stimulation became more effective in stimulating spikes as the interval was shortened. The percentage of suprathreshold trials was calculated and plotted for individual cells (B) and as an average of 14 cells (C). Although the efficacy of paired pulse stimulation varied it was greatest at the briefest interval (10 ms). **P < 0.01, ***P < 0.001.
Interaction Between Secondary Synaptic Summation and Short-Term Synaptic Plasticity
Previous studies have reported both facilitation and depression of nicotinic transmission in sympathetic ganglia using different preparations and stimulus paradigms (Larrabee and Bronk, 1947; McLachlan, 1975; Zengel et al., 1980; Birks and Isacoff, 1988; Karila and Horn, 2000; Lin et al., 2001). To determine whether either form of short-term plasticity strongly influenced spike generation by secondary synapses, we began by classifying synapses using averages of paired pulse trials, with suprathreshold responses removed (Figure 4A). The trials employed intervals within the temporal window of suprathreshold summation (i.e., ≤20 ms) that were collected at 0.2 Hz. In 10 neurons with a depressing secondary synapse, the paired pulse ratio (P2/P1) of EPSP amplitudes was consistently <1 at all time intervals: 0.54 ± 0.06, 0.39 ± 0.1, and 0.55 ± 0.2 (±SE) for interstimulus intervals of 20, 15, and 10 ms. In nine other neurons with a facilitating secondary synapse, the P2/P1 values were 1.70 ± 0.3, 1.87 ± 0.3, and 2.10 ± 0.5 at the same three intervals. Secondary synapses in the remaining group of 10 neurons gave inconsistent responses and were termed borderline. Four borderline neurons had P2/P1 values close to 1, while the remaining six borderline neurons fluctuated between depressing and facilitating responses at different intervals. The average P2/P1 ratios for all 10 borderline cells were 0.83 ± 0.2, 0.83 ± 0.1, and 1.0 ± 0.2 ms at 20, 15, and 10 ms. Examples of individual 20 ms paired pulse responses for each of the three behaviors are shown in Figure 4Ai, together with the corresponding averaged traces (Figure 4Aii). The paired pulse data were not corrected for effects of non-linear summation because secondary EPSP amplitudes were small in comparison to the driving force on synaptic currents (McLachlan, 1975).
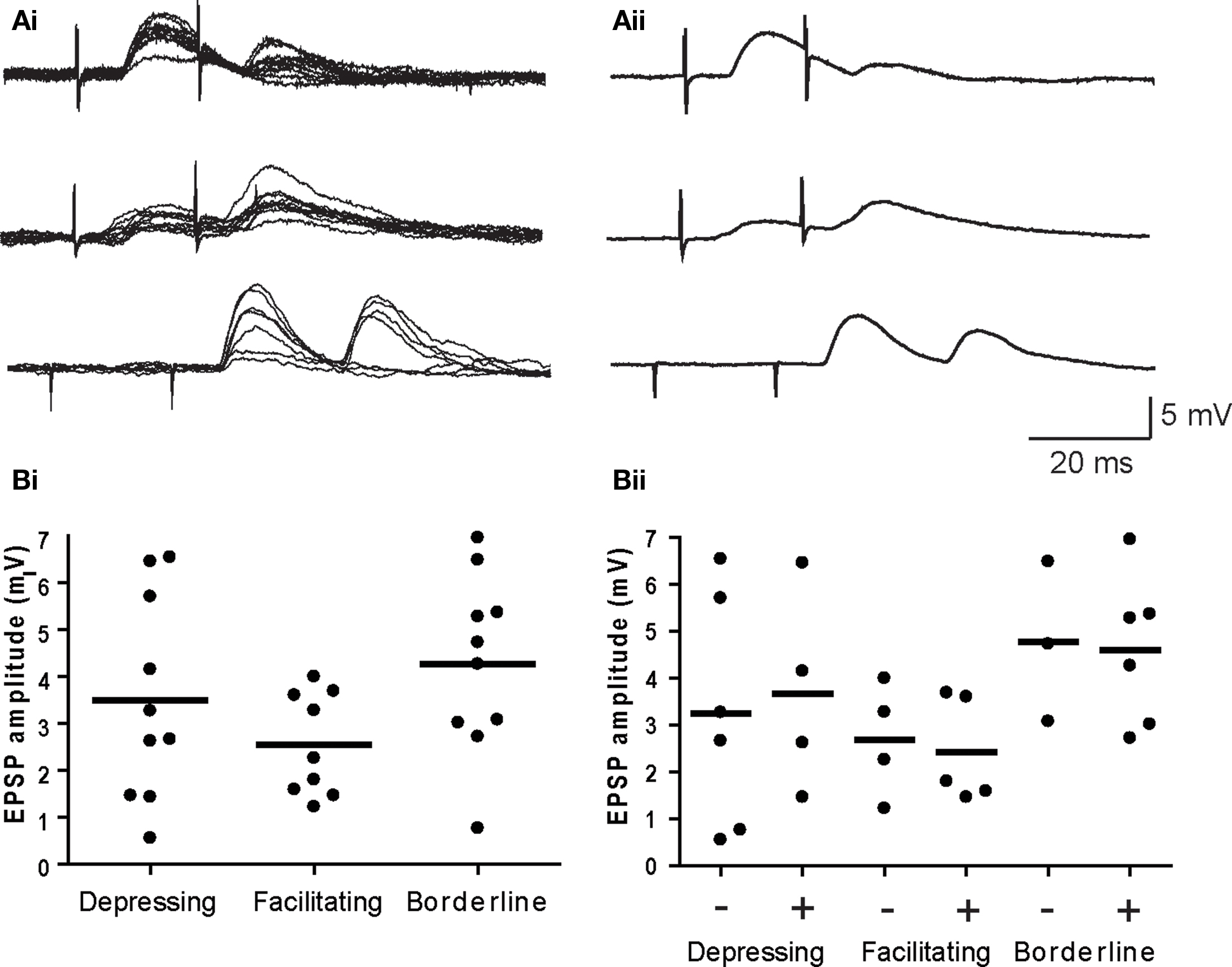
Figure 4. EPSP amplitude does not predict short-term plasticity or suprathreshold summation. (A) Examples of depressing (top), facilitating (middle), and borderline (bottom) paired pulse responses – (Ai) individual trials and (Aii) averaged EPSPs. (Bi) Plots of the average EPSP amplitude (first response in the pair) for individual synapses show no difference for the three types of plasticity. (Bii) Further subdivision of each response type into cells where paired EPSPs did (+) and did not (−) summate to action potential threshold revealed no differences in EPSP amplitudes. Horizontal lines denote the mean EPSP amplitude for each cell group in both graphs.
There was no significant difference (P > 0.13, ANOVA) in the average EPSP amplitude at depressing (3.49 ± 0.68 mV), facilitating (2.54 ± 0.36 mV), and borderline (4.27 ± 0.60 mV) secondary synapses (Figure 4Bi). Similar proportions of synapses belonging to all three groups were effective in producing suprathreshold summation and within each group there was no difference in EPSP amplitudes at synapses that yielded suprathreshold summation, when compared to those that did not (Figure 4Bii). It therefore appears that the expression of facilitation is not essential for coincidence detection by sympathetic neurons and that expression of depression does not eliminate coincidence detection.
Straddling Inputs are a Novel form of Secondary Synapses
In six other neurons, we found nicotinic synapses whose strength straddled threshold. At slow rates of stimulation, typically <1 Hz, these synapses reliably generated suprathreshold EPSPs. However, in response to paired stimuli at intervals between 30 and 300 ms, the second EPSP fluctuated around threshold during repeated trials. As the intervals between pulse pairs was shortened the second straddling response eventually failed completely to elicit spikes. This behavior is quite different than that seen with primary synapses where the safety factor for triggering a postsynaptic AP was very high and difficult to block even with large hyperpolarizing current injections. Figure 5A illustrates a particularly robust example of straddling behavior in which the second EPSP in the pair began to fall below spike threshold when delivered at a 350-ms interval, well after the spike afterpotential of the first response had recovered. The second response become progressively weaker at shorter intervals and became totally subthreshold at a 100 ms interval. In five other cases (Figure 5Bi), similar straddling behavior was evident, but at shorter intervals between 30 and 100 ms. When averaged, these data (Figure 5Bii) showed that straddling synapses were largely subthreshold in strength at stimulus intervals less than 200 ms. Unlike primary synapses, the strength of these synapses straddled threshold depending upon the recent history of previous activity. The average amplitude of the straddling EPSPs was 3.4 ± 2.2 mV (n = 6) and Vrest in these cells was −59.3 ± 2.7 mV. In cells with straddling synapses, we also observed that large primary synaptic responses were recruited at higher stimulus strengths. Unlike straddling EPSPs, the primary synaptic responses always elicited APs during repetitive stimulation at rates where straddling inputs failed to remain suprathreshold.
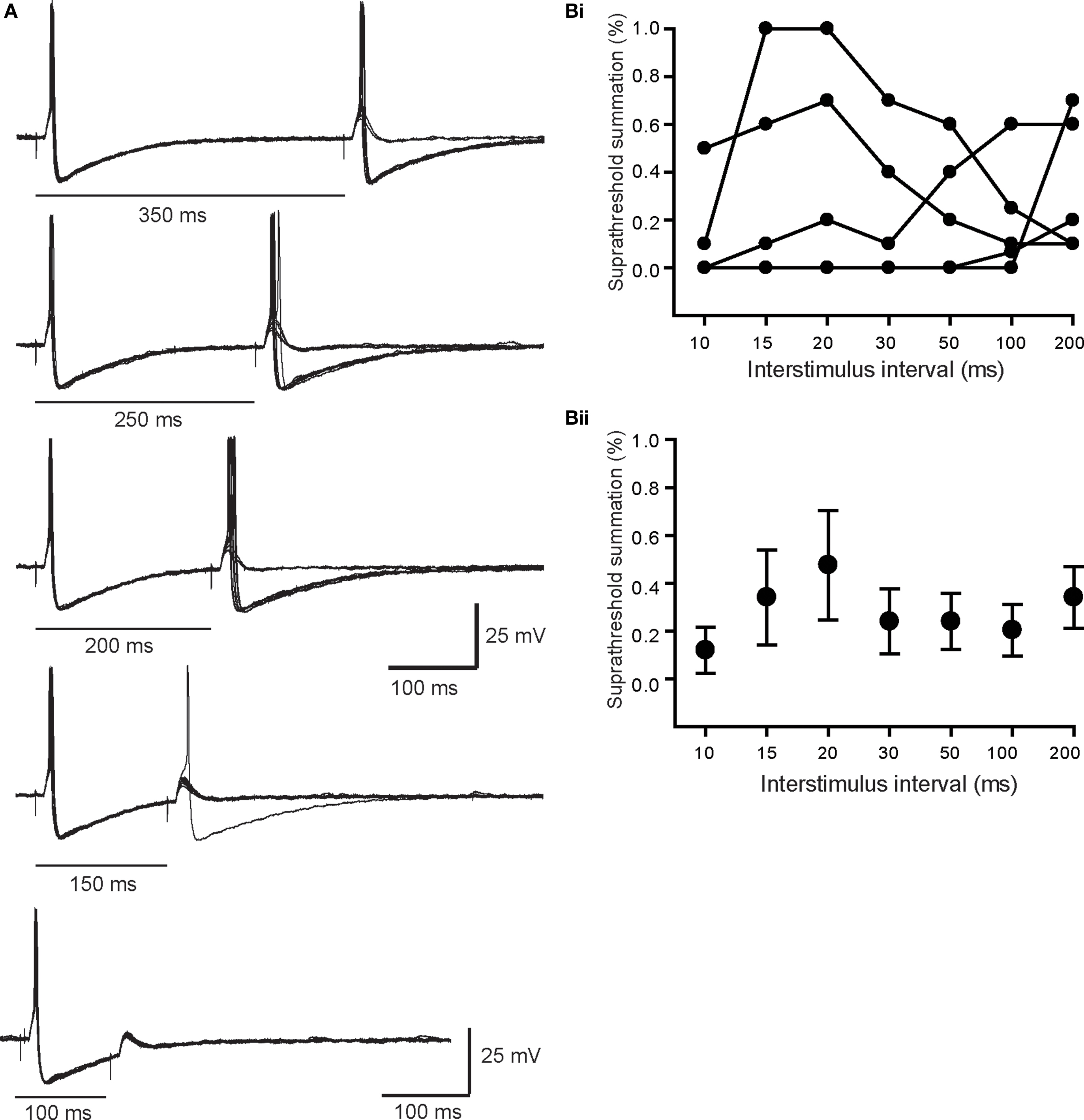
Figure 5. Properties of straddling EPSPs. (A) Superimposed paired pulse trials at intervals between 100 and 350 ms. Note that at in all cases the first response was always suprathreshold in strength. By contrast the second response in the pair straddled threshold, even at an interval of 350 ms, which was after full recovery of the first spike AHP. At shorter intervals where the second EPSP was evoked during the AHP, it became much less effective in driving spikes. (B) The efficacy of six straddling synapses, plotted for each cell (Bi) and as the average (Bii), shows that they become substantially subthreshold in strength at paired pulse intervals <200 ms.
Double Spike Responses
In three cells, we observed that a single presynaptic stimulus elicited a large EPSP and a doublet of APs in which the second spike was sometimes smaller than the first and could be eliminated by paired pulse stimulation (Figure 6). Given the rarity of these responses we were unable to determine whether double spike responses resulted from the convergence of two primary nicotinic synapses or from the summation of a primary synapse and multiple secondary EPSPs. Similar responses have been reported in vivo (McLachlan et al., 1998).
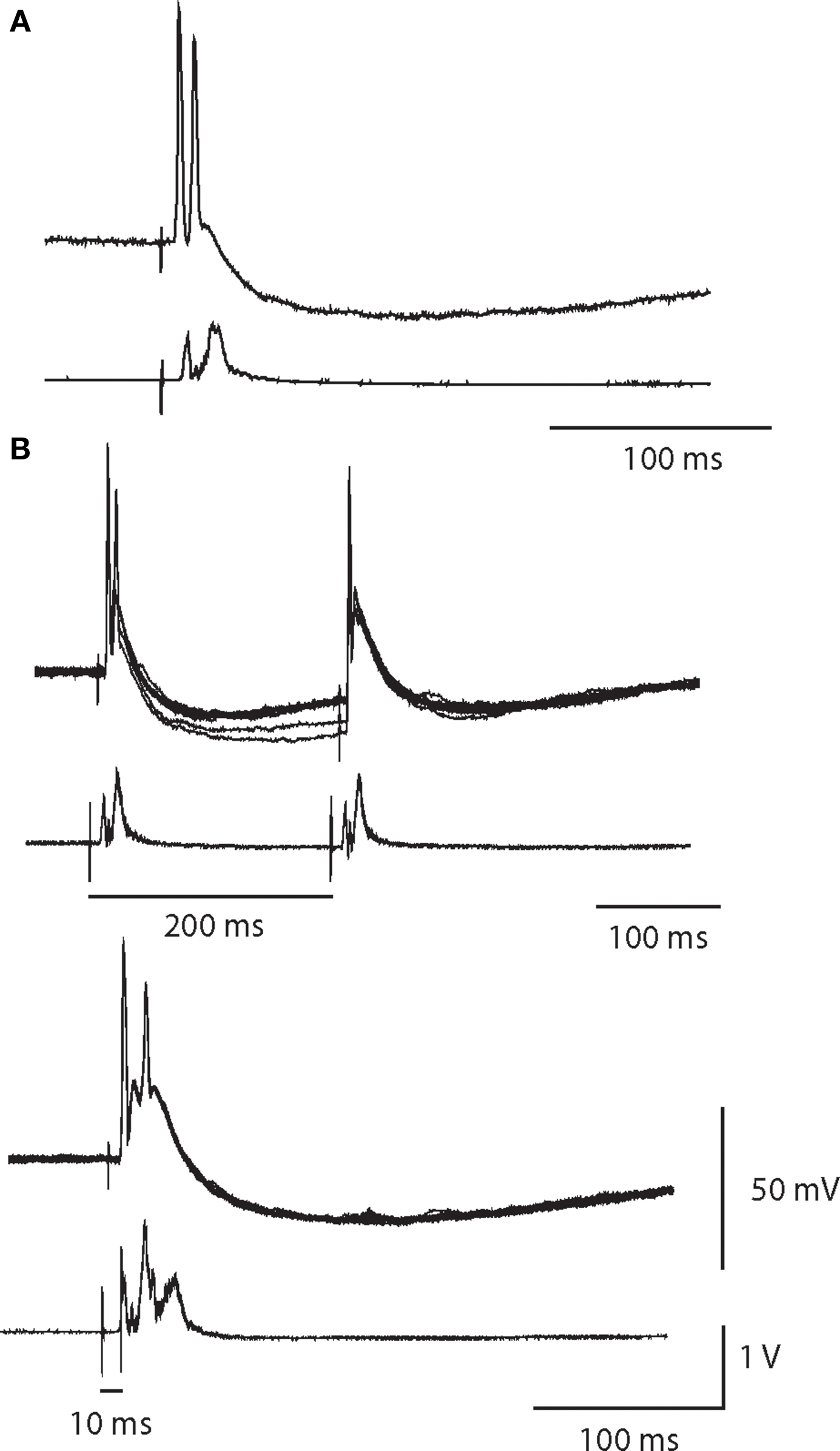
Figure 6. A single synaptic volley can drive double spikes. (A) Intracellular recording of a double spike neuron with a partially attenuated second spike. Simultaneous extracellular recording (below) indicates the cell was a high threshold neuron. (B) In response to paired pulse stimulation of the same cell at a 200-ms interval, the second spike failed. Ten superimposed trials showed that the double firing was robust after the first stimulus, but was completely absent after the second stimulus. Reducing the paired pulse interval to 10 ms obliterated the double spike response.
Discussion
In this study, weak secondary nicotinic synapses were evident in three quarters (40/53) of sympathetic neurons in the rat SCG when tested with graded presynaptic stimulation of the CST (Figures 1 and 2). Paired pulse stimulation of secondary synapses was capable of generating APs in half of the cells tested at intervals of 10–20 ms (Figure 3). The efficacy of paired pulse stimulation was greater at shorter paired pulse intervals. Although secondary synapses appeared heterogeneous in terms of their short-term plasticity, there was no correlation between the expression of depression or facilitation and the ability of paired pulse stimulation to drive postsynaptic APs (Figure 4). Together these observations, which were made at physiological temperature in acutely isolated ganglion preparations, suggest that coincidence detection of weak nicotinic EPSPs can contribute to the postsynaptic spike output of mammalian sympathetic neurons.
Is Coincidence Detection of Secondary Nicotinic EPSPs Sufficient to Generate Synaptic Gain?
Our results are consistent with in vivo intracellular evidence that weak nicotinic synapses can drive firing in sympathetic neurons (Skok and Ivanov, 1983; McLachlan et al., 1997, 1998; Bratton et al., 2010). However, the larger and more difficult question is whether such firing contributes in a major way to ganglionic output under physiological conditions. One way to examine the problem in quantitative terms uses the coincidence detection theory (Karila and Horn, 2000). Based on the observation that time intervals between naturally occurring synaptic events in sympathetic neurons appear to be exponentially distributed (McLachlan et al., 1998), it becomes possible to calculate the probability of coincidences between EPSPS, if one knows the average rate of activity. One can then estimate how many coincidences contribute to postsynaptic firing by knowing the temporal window required for suprathreshold summation of weak EPSPs. Our present results indicate the window of summation in rat SCG neurons is 10–20 ms.
The overall average rate of synaptic activity in rat SCG neurons depends upon the number of preganglionic neurons that converge on each cell and their average rate of firing at each synapse. Earlier measurements of synaptic convergence suggested that each cell receives one primary synapse and around nine secondary inputs (Purves et al., 1986), which is considerably higher than in our experiments. If one makes a much more conservative estimate that the number of secondary synapses was around 3 and that presynaptic firing rates are on the order of 1–3 Hz, then Karila and Horn’s theory predicts the synaptic gain will be on the order of 1.2–1.6 for a 20-ms window of summation (see Figure 9 in Karila and Horn, 2000). In other words, up to a third or more of postganglionic activity may arise from secondary EPSPs and this contribution will vary with the overall level of sympathetic tone. This is especially interesting given recent in vivo evidence that secondary nicotinic synapses may drive as much as two-thirds of postganglionic activity in lumbar sympathetic ganglia of the rat (Bratton et al., 2010).
Although the levels of synaptic convergence detected in our experiments were not as high as previously reported (Purves et al., 1986), we cannot claim to disagree with the earlier work. The focus of the work by Purves et al. (1986) was to count systematically the numbers of inputs to cells, while our goal was to isolate individual weak synapses and test their ability to drive firing.
Two considerations argue that our approach underestimates ganglionic synaptic gain. The first problem arises from microelectrode damage and the second from blunted autonomic activity in anesthetized animals. Recent preliminary experiments using whole cell patch electrode recordings from the SCG suggest that the window of summation may actually be 5–10 times greater than estimated with microelectrodes (Rimmer et al., 2009). The most informative estimates of in vivo preganglionic activity have come from intracellular recordings in anesthetized animals (Skok and Ivanov, 1983; Ivanoff and Smith, 1995; McLachlan et al., 1997, 1998; Bratton et al., 2010) and from extracellular single unit recordings from postganglionic nerves in awake human subjects (Macefield et al., 1994, 1999, 2002). Although these approaches provide insight into basal activity, it remains less clear how individual preganglionic and postganglionic neurons fire during intense aerobic exercise and other conditions where one might imagine that ganglionic amplification could prove most significant. Nonetheless, studies of total postganglionic sympathetic activity indicate that huge increases occur during strenuous exercise in humans (Fadel, 2008; Ichinose et al., 2008; Joyner et al., 2008).
A Handful of Synapses can be Very Influential – Lessons from the Thalamus
Although the thalamic relay nuclei were originally seen simply as relays, subsequent analysis has revealed they do much more in processing and directing activity to and from the cerebral cortex and between cortical areas (Sherman, 2007). One very important lesson from this work is that the size of a neuronal projection can belie its physiological significance. Indeed, Sherman and colleagues point out that while many have inferred the importance of neuronal projections from their magnitudes, this need not be the case (Sherman and Guillery, 1996, 2002; Sherman, 2007). The most important input to the lateral geniculate nucleus (LGN) comes from retinal ganglion cells and defines this circuit’s function. Yet these so-called “driver” connections constitute only a small minority of synapses (<5%) on geniculate neurons, with the remainder serving to modulate and shape the retinogeniculate stream of information. Other studies show that the number of glutamatergic synaptic connections between retinal ganglion cells and individual LGN cells is sparse and probably quite similar to the number and strength of nicotinic synapses on sympathetic neurons (Turner and Salt, 1998; Chen and Regehr, 2000; Deschenes et al., 2003). Although few in number nicotinic synapses may therefore play a key role in enabling autonomic ganglia to function as more than simple motor relays.
Straddling Synapses Raise New Questions
The presence of straddling nicotinic synapses in the SCG (Figure 5) suggests that coincidence detection may not fully explain how secondary synapses contribute to ganglionic gain. Such a possibility seems even more likely in view of the very recent report of straddling synapses on vasoconstrictor neurons in lumbar paravertebral sympathetic ganglia of the rat (Bratton et al., 2010). This study, which employed intracellular in vivo recordings suggests that as many as two-thirds of postganglionic APs in lumbar vasoconstrictors may be driven by straddling synapses and that the strength of nicotinic synapses may lie on a continuum, rather than fall into the dichotomy suggested by analysis of primary and secondary synapses in bullfrog sympathetic ganglia. It remains for future studies to resolve these issues by determining the precise strengths of nicotinic synapses in mammalian sympathetic ganglia from different segmental levels and from subsets of sympathetic neurons that control different peripheral target types.
Implications for Autonomic Transmission and Modulation
We have shown here that weak secondary EPSPs have the ability to drive postsynaptic firing in the rat SCG. The data provide further evidence that autonomic ganglia have the potential to amplify and modulate presynaptic activity. Weak secondary EPSPs and straddling secondary EPSPs each possess the latent ability to influence synaptic gain and to serve as the substrate for numerous forms of modulation through G protein coupled receptors that regulate presynaptic acetylcholine release and postsynaptic excitability.
Conflict of Interest Statement
The authors declare that the research was conducted in the absence of any commercial or financial relationships that could be construed as a potential conflict of interest.
Acknowledgment
This work was supported by NIH grant NS21065.
References
Birks, R. I., and Isacoff, E. Y. (1988). Burst-patterned stimulation promotes nicotinic transmission in isolated perfused rat sympathetic ganglia. J. Physiol. 402, 515–532.
Bratton, B., Davies, P., Janig, W., and McAllen, R. (2010). Ganglionic transmission in a vasomotor pathway studied in vivo. J. Physiol. 588, 1647–1659.
Chen, C., and Regehr, W. G. (2000). Developmental remodeling of the retinogeniculate synapse. Neuron 28, 955–966.
Deschenes, M., Timofeeva, E., and Lavallee, P. (2003). The relay of high-frequency sensory signals in the Whisker-to-barreloid pathway. J. Neurosci. 23, 6778–6787.
Fadel, P. J. (2008). Dynamic arterial baroreflex function during high intensity exercise in humans: insights into sympathetic control. J. Physiol. 586, 2667–2668.
Guillery, R. W., and Sherman, S. M. (2002). Thalamic relay functions and their role in corticocortical communication: generalizations from the visual system. Neuron 33, 163–175.
Habler, H. J., McLachlan, E. M., Jamieson, J., and Davies, P. J. (1999). Synaptic responses evoked by lower urinary tract stimulation in superior cervical ganglion cells in the rat. J. Urol. 161, 1666–1671.
Hirst, G. D., and McLachlan, E. M. (1984). Post-natal development of ganglia in the lower lumbar sympathetic chain of the rat. J. Physiol. 349, 119–134.
Horn, J. P., and Kullmann, P. H. (2007). Dynamic clamp analysis of synaptic integration in sympathetic ganglia. Neirofiziologiia 39, 423–429.
Ichinose, M., Saito, M., Fujii, N., Ogawa, T., Hayashi, K., Kondo, N., and Nishiyasu, T. (2008). Modulation of the control of muscle sympathetic nerve activity during incremental leg cycling. J. Physiol. 586, 2753–2766.
Ivanoff, A. Y., and Smith, P. A. (1995). In vivo activity of B- and C-neurones in the paravertebral sympathetic ganglia of the bullfrog. J. Physiol. 485, 797–815.
Janig, W. (2006). The Integrative Action of the Autonomic Nervous System, Cambridge University Press, Cambridge.
Joyner, M. J., Charkoudian, N., and Wallin, B. G. (2008). A sympathetic view of the sympathetic nervous system and human blood pressure regulation. Exp. Physiol. 93, 715–724.
Karila, P., and Horn, J. P. (2000). Secondary nicotinic synapses on sympathetic B neurons and their putative role in ganglionic amplification of activity. J. Neurosci. 20, 908–918.
Kullmann, P. H., and Horn, J. P. (2006). Excitatory muscarinic modulation strengthens virtual nicotinic synapses on sympathetic neurons and thereby enhances synaptic gain. J. Neurophysiol. 96, 3104–3113.
Kullmann, P. H., and Horn, J. P. (2010). Homeostatic regulation of M-current modulates synaptic integration in secretomotor, but not vasomotor, sympathetic neurons in the bullfrog. J. Physiol. 588, 923–938.
Kullmann, P. H., Wheeler, D. W., Beacom, J., and Horn, J. P. (2004). Implementation of a fast 16-Bit dynamic clamp using LabVIEW-RT. J. Neurophysiol. 91, 542–554.
Larrabee, M. G., and Bronk, D. W. (1947). Prolonged facilitation of synaptic excitation in sympathetic ganglia. J. Neurophysiol. 10, 139–154.
Li, C., and Horn, J. P. (2006). Physiological classification of sympathetic neurons in the rat superior cervical ganglion. J. Neurophysiol. 95, 187–195.
Lin, Y. Q., Graham, K., and Bennett, M. R. (2001). Depression of transmitter release at synapses in the rat superior cervical ganglion: the role of transmitter depletion. Auton. Neurosci. 88, 16–24.
Macefield, V. G., Elam, M., and Wallin, B. G. (2002). Firing properties of single postganglionic sympathetic neurones recorded in awake human subjects. Auton. Neurosci. 95, 146–159.
Macefield, V. G., Rundqvist, B., Sverrisdottir, Y. B., Wallin, B. G., and Elam, M. (1999). Firing properties of single muscle vasoconstrictor neurons in the sympathoexcitation associated with congestive heart failure. Circulation 100, 1708–1713.
Macefield, V. G., Wallin, B. G., and Vallbo, A. B. (1994). The discharge behaviour of single vasoconstrictor motoneurones in human muscle nerves. J. Physiol. 481, 799–809.
Maslov, V. Y., Ivanov, A. Y., and Skok, V. I. (1996). Background activity of the rabbit superior cervical ganglion neurons: soundness of random hypothesis of its formation. Neirofiziologiia. 39, 486–492.
McLachlan, E. M. (1975). An analysis of the release of acetylcholine from preganglionic nerve terminals. J. Physiol. 245, 447–466.
McLachlan, E. M. (2003). Transmission of signals through sympathetic ganglia – modulation, integration or simply distribution? Acta Physiol. Scand. 177, 227–235.
McLachlan, E. M., Davies, P. J., Habler, H. J., and Jamieson, J. (1997). On-going and reflex synaptic events in rat superior cervical ganglion cells. J. Physiol. 501, 165–181.
McLachlan, E. M., Habler, H. J., Jamieson, J., and Davies, P. J. (1998). Analysis of the periodicity of synaptic events in neurones in the superior cervical ganglion of anaesthetized rats. J. Physiol. 511, 461–478.
Purves, D., Rubin, E., Snider, W. D., and Lichtman, J. (1986). Relation of animal size to convergence, divergence, and neuronal number in peripheral sympathetic pathways. J. Neurosci. 6, 158–163.
Rimmer, K., Kullmann, P. H., and Horn, J. P. (2009). A robust method for whole-cell recording in the rat superior cervical ganglion – comparison of patch and microelectrode data helps resolve the n + 1 rule. Soc. Neurosci. Abstr. Abstract No. 226.25.
Sherman, S. M., and Guillery, R. W. (1996). Functional organization of thalamocortical relays. J. Neurophysiol. 76, 1367–1395.
Sherman, S. M., and Guillery, R. W. (2002). The role of the thalamus in the flow of information to the cortex. Philos. Trans. R. Soc. Lond., B, Biol. Sci. 357, 1695–1708.
Skok, V. I., and Ivanov, A. Y. (1983). What is the ongoing activity of sympathetic neurons? J. Auton. Nerv. Syst. 7, 263–270.
Tokimasa, T., Cherubini, E., and North, R. A. (1983). Nicotinic depolarization activates calcium dependent gK in myenteric neurons. Brain Res. 263, 57–62.
Turner, J. P., and Salt, T. E. (1998). Characterization of sensory and corticothalamic excitatory inputs to rat thalamocortical neurones in vitro. J. Physiol. 510, 829–843.
Wang, F. B., Holst, M. C., and Powley, T. L. (1995). The ratio of pre- to postganglionic neurons and related issues in the autonomic nervous system. Brain Res. Brain Res. Rev. 21, 93–115.
Wheeler, D. W., Kullmann, P. H., and Horn, J. P. (2004). Estimating use-dependent synaptic gain in autonomic ganglia by computational simulation and dynamic-clamp analysis. J. Neurophysiol. 92, 2659–2671.
Keywords: superior cervical ganglion, synaptic gain, summation, facilitation, depression
Citation: Rimmer K and Horn JP (2010) Weak and straddling secondary nicotinic synapses can drive firing in rat sympathetic neurons and thereby contribute to ganglionic amplification. Front. Neur. 1:130. doi: 10.3389/fneur.2010.00130
Received: 12 July 2010;
Paper pending published: 06 August 2010;
Accepted: 10 September 2010;
Published online: 23 September 2010
Edited by:
Simon J. H. Brookes, Flinders University, AustraliaReviewed by:
Simon J. H. Brookes, Flinders University, AustraliaVladimir Zagorodnyuk, Flinders University, Australia
Copyright: © 2010 Rimmer and Horn. This is an open-access article subject to an exclusive license agreement between the authors and the Frontiers Research Foundation, which permits unrestricted use, distribution, and reproduction in any medium, provided the original authors and source are credited.
*Correspondence: John P. Horn, Department of Neurobiology, University of Pittsburgh School of Medicine, E1440 Starzl Biomedical Science Tower, Pittsburgh, PA 15261, USA. e-mail: jph@pitt.edu