- 1 CNRS UMR6149, Laboratory of Integrative and Adaptive Neurobiology, University of Provence Marseille, Marseille, France
- 2 INSERM U1028, CNRS UMR5292, Lyon Neuroscience Research Center, Bron, France
- 3 CNRS, University Claude Bernard Lyon 1, Lyon, France
- 4 CNRS UMR 6155, Laboratory of Neurobiology and Cognition, University of Provence, Marseille, France
Anticipatory postural adjustments (APAs) compensate in advance for the destabilizing effect of a movement. This study investigated the specific involvement of each primary motor cortex (M1) during a bimanual load-lifting task in which subjects were required to maintain a stable forearm position during voluntary unloading. Kinematics, electromyographic, and electroencephalographic (EEG) data were recorded in eight right-handed healthy subjects lifting a load placed on their left forearm. Two EEG analyses were performed: a time–frequency (TF) analysis and an event-related potential (ERP) analysis. The TF analysis revealed a mean power decrease in the mu rhythm over the left and right M1 concomitant with lifting onset. Each decrease showed specific features: over the right M1, contralateral to the postural forearm, there was a steeper slope and a greater amplitude than over the left M1. Although a mu rhythm desynchronization has until now been the signature of cortical activity related to a motor component, we show that it can also be related to postural stabilization. We discuss the involvement of the mu rhythm desynchronization over the postural M1 in the high temporal precision enabling efficient APAs. ERP analysis showed a negative wave over the left M1 and a concomitant positive wave over the right M1. While the negative wave classically reflects M1 recruitment related to the forthcoming lifting, the novelty here is that the positive wave reflects the transmission of inhibitory commands toward the postural forearm.
Introduction
The execution of a voluntary movement triggers postural perturbations, which can be predicted and cancelled by the central nervous system. In order to minimize the destabilizing effects of the movement on the posture, the anticipatory postural adjustments (APAs) occur before the onset of movement and prevent the forthcoming disturbance of posture (Bouisset and Zattara, 1981; Massion et al., 1999). Spatial and temporal coordination between movements and APAs is crucial for the efficiency of a gesture, but up to now the underlying central organization has not been fully understood.
The coordination between a movement and its postural counterpart has been extensively studied using the bimanual load-lifting task, derived from the so-called “barman test.” When a barman lifts up a bottle from the tray he is holding, the hand supporting the tray stays stabilized in a horizontal position, although unloading the tray should provoke an upward forward movement. This is due to the use of APAs. In the experimental context, the subjects use their right (motor) hand to voluntarily lift a load placed on their left (postural) forearm (Hugon et al., 1982). The advantage of this paradigm is that it enables a clear anatomical separation of the movement and its associated APAs. Here, APAs are characterized by a stabilization of the postural forearm due to an inhibition of the flexor muscles before the onset of unloading (Hugon et al., 1982).
Models of brain lesions (see Massion et al., 1999 for a review), EEG (Martineau et al., 2004), magnetoencephalography (Ng et al., 2011) and fMRI (Schmitz et al., 2005) recordings during the bimanual load-lifting task have shown the involvement of the sensorimotor cortex, the supplementary motor area (SMA), and the basal ganglia within the hemisphere contralateral to the postural arm in the production of APAs. As an alteration of the postural performance was found in patients with a lesion of the primary motor cortex (M1) contralateral to the postural arm only, the specificity of the role of each M1 has been questioned (Viallet et al., 1992). Kazennikov et al. (2005) suggested that the M1 contralateral to the lifting arm actively inhibits the corticospinal neurons of the M1 contralateral to the postural arm through interhemispheric transcallosal connections. This seems contradictory to results showing that patients with a complete resection of the corpus callosum have good postural stability (Viallet et al., 1992; Diedrichsen et al., 2005). In another posture–movement coordination task, transcranial magnetic stimulation (TMS) over each M1 altered the movement but did not affect the posture, suggesting that each command develops independently (Taylor, 2005). The specificity of the postural and the lifting commands arising from each M1 therefore remains unsolved.
Studies with EEG have revealed that during the planning and the execution of movements, the mu rhythm, characterized by frequencies in the 8- to 13-Hz band, is suppressed above the sensorimotor areas. This phenomenon first described as “blocking” of the central mu rhythm in man (Gastaut et al., 1952) has been later referred to as “event-related-desynchronization” (ERD). ERD can be observed in response to a variety of different type of tasks. In general, ERD reflects active information processing in the sense of excitatory brain processes (Klimesch et al., 2007). More specifically, in motor tasks, it has been interpreted to reflect an increased cortical excitability of the M1 contralateral to the moving side (Pfurtscheller and Lopes da Silva, 1999). A previous study in children revealed an ERD in the theta rhythm (equivalent to the mu rhythm in adults, taking into account the maturational process; Cochin et al., 2001) over the M1 contralateral to the postural arm in the bimanual load-lifting task (Martineau et al., 2004). However, due to the small number of trials, the exact timing was not determined. Moreover, in between-hand choice tasks, premovement potentials have revealed a negative wave over one M1 and a positive wave over the other, reflecting a specific activation of the M1 contralateral to the responding hand and an inhibition of the M1 contralateral to the non-responding hand (Vidal et al., 2003).
The aim of this study was to clarify the contribution of each M1 during the coordination between posture and movement that takes place in the bimanual load-lifting task. We assumed that a detailed comparison between EEG activities recorded over each M1 would reveal common and/or distinct features, which should help to disentangle the specificity of the motor and the postural commands. Originally, in order to capture a broad range of EEG features, we performed two types of EEG analysis because of their complementarities: a time–frequency (TF) analysis and an event-related potentials (ERPs) analysis. We used TF analysis based on Gaussian Morlet’s wavelet transform (Tallon-Baudry and Bertrand, 1999) instead of the traditional ERD/ERS quantification, because this approach provides an overview of the activity over broad frequency ranges, which enables a specific frequency band to be chosen for each subject for a detailed analysis (Graimann and Pfurtscheller, 2006). We hypothesized that a TF analysis focused on oscillatory activities would highlight features in the sensorimotor rhythms differentiating the postural and lifting programs, while an ERP analysis would specify the role of each M1.
Materials and Methods
Subjects
Eight healthy subjects [four males, 29.11 ± 4.3 years old (mean ± SD)] participated in this experiment. All participants, chosen in the general population, used they right hand for every day manual activities and did not show any particular motor expertise. All participants gave their informed consent before the trial and local ethics committee approval (Comité de Protection des Personnes Sud Méditerranée 1) was obtained in accordance with the ethical standards of the Declaration of Helsinki.
Task and Experimental Set-Up
The experimental arrangement has been described in previous papers (Massion et al., 1999; Schmitz et al., 2002) and is schematically depicted in Figure 1A.
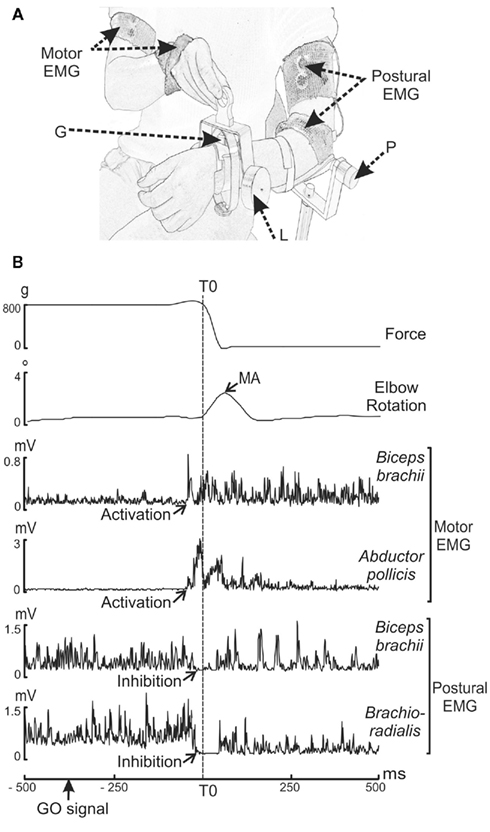
Figure 1. (A,B) Experimental set-up and raw trial recording during voluntary unloading. (A) G: strain gage measuring the force exerted by the load. P: potentiometer measuring the elbow rotation. L: 800 g-load. Motor EMG: EMG recording sites in the motor arm (biceps brachii and abductor pollicis brevis). Postural EMG: EMG recording sites in the postural arm (brachioradialis and biceps brachii). (B) Parameters recorded (from top to bottom) are: force, elbow rotation angle, rectified EMG of the biceps brachii and abductor pollicis brevis on the motor arm, and of the biceps brachii and brachioradialis on the postural forearm. The decrease in force indicated the onset of unloading (vertical dashed line), which was used as a reference time (T0). The maximum angular amplitude (MA) of the upward movement of the postural forearm was measured after unloading. The muscular activity (activations for the motor arm and inhibitions for the postural arm) occurred before T0.
The subjects were comfortably seated on a hardback chair in a Faraday cage. In the bimanual load-lifting task, the postural arm supports the load while the motor arm lifts the load (Dufosse et al., 1985; Massion et al., 1999). The postural arm, chosen as the left arm for all subjects, was fixed vertically just above the elbow. The subjects were asked to maintain the left forearm in a horizontal and semi-prone position throughout the entire session, with no specific instructions. Wrapped around each subject’s wrist was a metallic wristband equipped with a strain gage, enabling a load to be either suspended by means of an electromagnet or placed on top of the forearm. In order to measure the effectiveness of the stabilization during the bimanual load-lifting task, repetitive imposed unloadings were used in a control situation. This consisted of a passive situation during which the suspended load was released by the experimenter by switching off the magnet at unpredictable times. In this situation, no anticipation was observed; a reactive postural correction, expressed by an unloading reflex, was seen at the level of the flexors of the postural forearm (Hugon et al., 1982). In contrast, in the voluntary situation, the load placed on top of the wristband was voluntarily lifted by the subjects with their right hand. The beginning of each active trial was indicated by a green LED located close to the weight placed on the postural arm (GO signal). Subjects were instructed to lift the load as quickly as possible when the green LED appeared. In order to maintain a high level of attention, NO GO trials signaled by a red LED were also presented to the subjects (who were instructed not to lift the load in those trials). Only GO trials were included in the analysis. Moreover, to limit the ocular artifacts in EEG signals, subjects were informed that they could blink or close their eyes only between trials.
Compared to previous studies (Dufosse et al., 1985; Massion et al., 1999), the weight of the load was reduced from 1000 to 800 g in order to avoid any muscular fatigue caused by the high repetition of lifts in the voluntary situation (n = 210). The general procedure was as follows: a series of 10 passive trials in the imposed situation followed by six series of 35 trials in the voluntary situation, making a total of 210 GO trials. A 5-min rest period was proposed between each series. An entire session usually lasted less than 2 h.
Data Acquisition and Analysis
In each trial, the force exerted by the load and the angular elbow displacement signals were recorded on the postural side, digitalized, and stored on a computer disk (Windelest®, TechnoConcept, France). Electromyographic (EMG) signals were recorded with a TELEMG multi-channel electromyograph (BTS). Both kinematics and EMG signals were acquired with a 500-Hz sampling rate. EEG and electrooculographic (EOG) data were continuously monitored with a 512-Hz sampling rate by the BioSemi Active Two system (BioSemi, Amsterdam). Because the EEG and the EMG signals were sampled with independent amplifiers and acquisition cards and also with different sampling rates, a common trigger ensured the time correspondence between the two data sets.
Kinematics, force, and EMG acquisition
The change of force was measured by a strain gage fitted to the metallic wristband supporting the weight. The angular displacement of the forearm was measured by a potentiometer placed along the elbow joint axis. EMG data were acquired using bipolar surface electrodes (surface area: 2.5 mm2) placed over the surface of two flexors (brachioradialis, biceps brachii) on the postural forearm, and one flexor (biceps brachii) and one abductor (abductor pollicis brevis) on the motor arm. The EMG signals were amplified, filtered (5–100 Hz band pass), and rectified using the MatLab software program (The Math-works, Inc.).
Kinematics, force, and EMG analysis
Figure 1B illustrates a single trial recorded during the voluntary situation. Each trial was viewed offline on a monitor screen. Measurements were performed with the MatLab software program (The Math-works, Inc.). The onset of unloading (T0), used as a reference time, was defined as the time of the first maximal value of the second derivative of the force signal transmitted by the strain gage. The upward movement of the postural forearm was quantified both in the imposed and voluntary situations by measuring the maximum angular amplitude (MA) after unloading. In the voluntary situation we also measured the reaction time (RT), defined as the time-interval between the onset of the GO signal and T0. A visual inspection made it possible to characterize identifiable EMG events for each trial. They consisted of activations or inhibitions, for which we measured the EMG latencies, i.e., the time-interval between T0 and the onset of the EMG event, and the EMG duration, i.e., the interval between the onset and the end of EMG activity. The onset of the EMG event was determined visually and marked with a cursor using the computer mouse. Although laborious, this method was preferred to an automated one because it guarantees an accurate and reliable detection (Van Boxtel et al., 1993).
We compared MA obtained in the imposed and voluntary situations to ensure the presence of APAs during the bimanual coordination. Furthermore, in order to detect any possible effects of the muscular strain on APAs effectiveness throughout the entire voluntary situation, we verified that the kinematics and muscular events were not altered. Differences in MA and in the latency and duration of the postural muscle inhibitions were tested by comparing the first 25 and the last 25 trials. Statistical analyses conducted for the kinematics and EMG data were performed using the Wilcoxon test. Differences with a P value <0.05 were considered statistically significant.
EEG data acquisition
During the voluntary situation, EEG was recorded from 64 pre-amplified Ag/AgCl scalp electrodes placed according to the standard 10–20 electrodes placement system. In order to detect ocular artifacts, EOG was recorded using electrodes situated above and below the left eye and both outer canthi. EEG and EOG data were amplified and filtered with a bandwidth between 0.1 and 200 Hz. A selective 50 Hz “notch” filter was used. The data were offline referenced to the left mastoid.
EEG data analysis
The acquired EEG data were subjected to the following preprocessing steps: preprocessing as well as artifact removal were performed in the MATLAB environment program (The Math-works, Inc.) with the EEGLAB toolbox (Delorme and Makeig, 2004) as well as in BrainVision Analyser software (Brain Product Gmbh).
Subsequently, since the bimanual motor task involved the muscles of the shoulder girdle, we used independent component analysis (ICA) to remove muscle artifacts as well as ocular ones. The use of ICA allows direct examination of information components in the data rather than their summed effects at the scalp electrodes. By removing or minimizing the effects of overlapping components, ICA enables a detailed examination of the separate dynamics of electrical brain activity as well as artifacts in order to remove them (Delorme et al., 2007). In addition, if the artifact ICA components were deemed unsatisfactory, the EEG recordings were visually inspected and trials presenting with residual artifacts were rejected. The Laplace transform was applied to the monopolar averages after spherical spline interpolation, with three as the order of spline (Pernier et al., 1988; Perrin et al., 1989). All further statistical analysis were performed on Laplacian transform.
We chose T0 (i.e., the onset of unloading) as the reference time because T0 is the common event indicating accurate coordination between the postural arm and the motor arm. This time reference has been classically used in other behavioral and EEG studies (Viallet et al., 1992; Massion et al., 1999; Schmitz et al., 2002; Martineau et al., 2004). The pre-GO signal period (defined from −1000 to −200 ms with respect to the GO signal) was considered as a baseline level for both the TF and the ERP analyses.
Time–frequency analysis
Each epoch was analyzed in the TF domain by convolution with complex Gaussian Morlet’s wavelets (Tallon-Baudry and Bertrand, 1999). This convolution provided for each trial a TF power map P (t, f) = Iw (t, f) * s (t) I2, where w (t, f) was for each time t and frequency f a complex Morlet’s wavelet , with
and σt = 1/(2πσf), and σf a function of the frequency f: σf = f/7. The investigated frequency range was 5–49 Hz with a step of 1 Hz. After averaging the TF data power across each single trial, the mean TF power data measured during the baseline was subtracted from the TF power data. This correction was performed separately in each frequency band. From the 64 original electrodes, we focused our analyses on C3 and C4 electrodes corresponding to the two motor cortices, M1L for the left M1 and M1R for the right M1 respectively. Visual inspection revealed the most prominent task-related spectral power change, mainly observed around the 10- to 13-Hz frequency band, corresponding to the sensorimotor mu rhythm. Since the upper and the lower limits as well as the frequency bandwidth varied across subjects, we selected for each of them the band power with the most significant variations during the task on the basis of the TF map obtained for M1R. We selected M1R instead of M1L because the most significant power variations occurred over this cortex. For each subject we used the half-height bandwidth to select the subject’s mu frequency band. Further analysis of the time course of spectral power (−1000 to 1000 ms relative to reference time) was performed on the individualized mu frequency band.
Statistical analyses of the time course of spectral power were performed using the Wilcoxon test. Differences with a P value <0.05 were considered statistically significant.
(1) To eliminate the possibility that further differences between M1R and M1L band powers were due to different level of baseline activities, we first compared their absolute powers during a period (F1) defined as a time window extending from −550 to −300 ms with respect to the GO signal.
(2) For each M1 separately, to determine when the mu rhythm differed from the baseline, a comparison between the power values and the baseline was performed.
(3) We then directly compared M1R and M1L band power amplitudes for (F2) defined as a time window extending from T0 to the point at which the forearm was stabilized (250 ms after T0).
(4) To compare the time course of the spectral power related to M1R and to M1L, the slope of the curve was estimated for each electrode (C3 and C4) and for each subject. The slope estimation was based on a linear regression with a period starting at the first maximal value of the second derivative of the spectral power time course and extending to T0. We also compared the initial slopes of the time course of the spectral power obtained over M1L and M1R to a theoretical zero value using the one-sample Wilcoxon test over two time periods. The first (P1) extended from −300 to −250 ms with respect to T0. The second (P2) extended from −240 to −140 ms before T0. Differences with a P value <0.05 were considered statistically significant.
Event-related potential analysis
Separate ERP averages were obtained for C3 and C4 electrodes over each sensorimotor cortex. We compared the initial slopes of the waves obtained over M1L and M1R to a theoretical zero value using the one-sample Wilcoxon test during two time windows: (P1) extending from −300 to −250 ms with respect to T0, and (P2) extending from −240 to −140 ms with respect to T0. Differences with a P value <0.05 were considered statistically significant.
The TF, ERP, and statistical analyses were performed with a software package for electrophysiological analysis (ELAN-Pack) developed at the CRNL (Brain Dynamics and Cognition team, Lyon, France; http://elan.lyon.inserm.fr/).
Results
Kinematics and EMG Analyses
The mean values of MA during the voluntary situation (1.3° ± 3.2°) showed a significant reduction (w = ± 78; p < 0.001) as compared to the mean values during the imposed situation (9.1° ± 3.2°), indicating an effective stabilization of the postural forearm.
During the voluntary situation, the contraction of the muscle of the motor arm appeared before unloading (−55 ± 29 ms for the abductor pollicis brevis and −49 ± 16 ms for the motor biceps brachii). The two flexors of the postural forearm showed a decrease in activity also starting before unloading (−14 ± 11 ms for the postural biceps brachii and −8 ± 8 ms for the brachioradialis), signaling an anticipatory EMG event. The duration of each muscular event was 228 ± 38 ms for the abductor pollicis brevis, 152 ± 35 ms for the motor biceps brachii, 92 ± 23 ms for the postural biceps brachii, and 82 ± 10 ms for the brachioradialis.
The comparison between the first 25 and last 25 trials did not reveal any significant differences for the mean values of MA (1.5 ± 0.4° versus 1.4 ± 0.4°), the latency (−14 ± 14 versus −16 ± 13 ms) or the duration (78 ± 13 versus 68 ± 10 ms) of the biceps brachii inhibition, and the latency (−6 ± 9 versus −8 ± 12 ms) and the duration (84 ± 20 versus 66 ± 7 ms) of the brachioradialis inhibition (p > 0.05).
Moreover, in the voluntary situation the mean RT was 333 ms with a low inter-subject variability (+77 ms) and a low intra-subject variability (around ±60 ms).
Time–Frequency Analysis
Time–frequency maps
The TF maps are presented in Figure 2 for C3 and C4 electrodes over the two motor cortices: M1L and M1R. These maps show the mean power in the 5- to 49-Hz range as a function of time for M1L and M1R during the voluntary situation. The mean power decreased in the 10- to 13-Hz frequency band around T0 over M1L (Figure 2A) and M1R (Figure 2B).
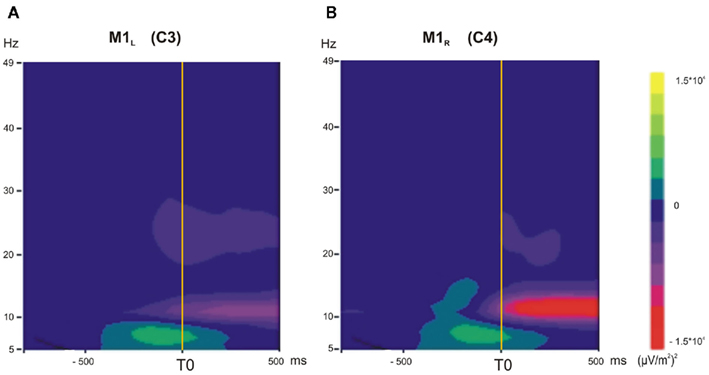
Figure 2. (A,B) Time–frequency (TF) analysis in the 5- to 49-Hz frequency range, grand average across subjects in the voluntary situation (−1000 to +500 ms). (A) M1L, left primary motor cortex. (B) M1R, right primary motor cortex. A transient diminution in the 10- to 13-Hz frequency range occurred for M1L and M1R just before T0 and during unloading.
Topographic maps
To explore the spatial distribution of the mean power decrease in the 10- to 13-Hz frequency band over each M1, the mean power has been mapped over the whole scalp as a function of time (Figure 3). After the presentation of the GO signal, a decrease of the mean power occurred over the occipital areas at about −350 ms before T0. It increased with time and reached its maximum around −150 ms. Over the central areas, a power decrease occurred around −50 ms prior to T0 and reached its maximum about 200 ms after T0. The Laplacian transform enabled to clearly disclose the occipital from the central transient activity over the motor areas in the 10- to 13-Hz frequency band.
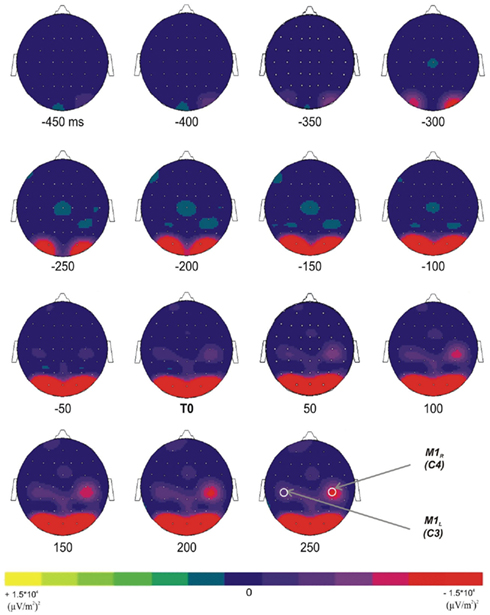
Figure 3. Topographic representations (top view) of the TF energy time course (from −450 ms before T0 to +250 after T0, with a 50-ms step) in the 10- to 13-Hz frequency range; grand average across subjects. Two distinct diminutions occurred over the occipital and the superior central areas.
Time course
For each subject, the individual M1R TF map was used to define the frequency band specific to the mu rhythm. This resulted in a mean frequency band of 10.6 ± 2.1 Hz for the lower limit and of 13.2 ± 2.1 Hz for the upper limit. The time course of each individually defined band power was then averaged and extracted for M1L and M1R (Figure 4).
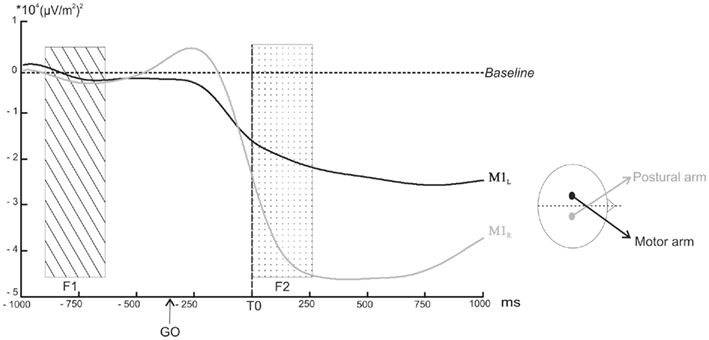
Figure 4. Power profile in selected band power of the TF energy as a function of time over M1L (black line) and M1R (grey line) averaged across subjects. The arrows indicate the point at which the decrease in the selected band power became significantly different from the baseline (p < 0.05). The rectangular areas indicate the two time windows chosen for the comparison of the mean amplitude (dotted rectangular area F1: from −500 to −300 ms before the GO signal, ns; hatched rectangular area F2: from 0 to +250 ms after T0, p < 0.05).
(1) As the tonic muscular activities recorded before T0 on the postural and the motor arms were different, it was first necessary to eliminate the possibility that the baseline activities recorded over M1L and M1R were also different. A direct comparison between the two baselines during F1 indicated no significant differences (p > 0.05).
(2) The power profile averaged across subjects revealed a decrease above M1L starting around −250 ms prior to T0. This decrease became significantly different from the baseline 20 ms after T0 (z = −1.96, p < 0.05). In a similar way, the amplitude of the mu rhythm above M1R decreased around −250 ms but differed significantly from the baseline −7 ms before T0 (z = −1.96, p < 0.05). The mu rhythm remained below the baseline level after the movement.
(3) The comparison between the amplitude of the M1L and M1R mean band powers during F2 indicated that it was significantly higher for M1R than for M1L (z = −24, p = 0.046).
(4) M1L and M1R mean band power slopes differed significantly from each other (z = 36, p = 0.007). The slope comparison performed during (P1) and (P2) confirmed that M1L and M1R slopes were both significantly negative (z = −24, p < 0.05).
Event-related potentials
Figure 5 shows the ERPs time-locked to T0 for C3 and C4 electrodes over the two motor cortices: M1L and M1R. During (P1), the slope of the two signals did not differ from a theoretical zero value (p > 0.05). A negative wave, starting at −240 ms with respect to T0, was observed over M1L with a maximal peak about −40 ms before T0. Over M1R, a positive wave started concomitantly. The slope comparison performed during (P2) confirmed that the M1L slope was significantly negative (z = −55, p = 0.039) whereas the M1R slope was significantly positive (z = 28, p = 0.027).
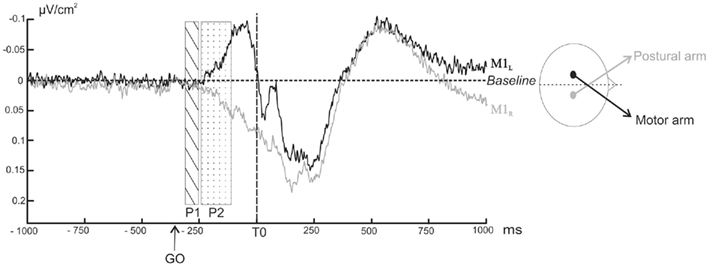
Figure 5. Grand averages of surface Laplacians as a function of time over M1L (black line) and M1R (grey line) averaged across subjects. Negativity is up. The rectangular areas indicate the two time windows chosen for the comparison of the slope to a theoretical zero value (dotted rectangular area P1: from −300 to −250 ms before T0, ns; hatched rectangular area P2: from −240 to −140 ms before T0, p < 0.05).
Discussion
To clarify the specific contribution of each M1 and the temporal organization of the postural and motor commands during the bimanual load-lifting task, we explored the characteristics of APAs using TF and ERP analyses. While models of brain lesions (see Massion et al., 1999 for a review) and fMRI recordings (Schmitz et al., 2005) have identified a broad network of regions involved in the organization and the production of APAs during the bimanual load-lifting task, here EEG analyses enabled to tackle the question of the cortical dynamics and the nature of both M1 recruitment. Beyond this task, this is relevant to the general understanding of the neurophysiological mechanisms yielding the coordination between motor and postural control. The main results can be summarized as follows:
(1) Time–frequency analysis showed a mean power decrease in the mu rhythm over both motor cortices (M1L and M1R) before lifting onset. Distinct features in terms of slope and amplitude characterized mu rhythm variations recorded over each M1.
(2) Event-related potentials showed a negative wave over M1L contralateral to the motor arm, and a positive wave over M1R contralateral to the postural arm.
Reduced maximal elbow rotation during the voluntary unloading situation indicated the use of APAs, characterized by an early inhibition of the postural flexor muscles (Hugon et al., 1982). Since EEG methods require a large number of trials, we needed to ensure that any potential muscular fatigue would not alter the characteristics or effectiveness of the APAs, or their central expression. This is unlikely since the effectiveness of the APAs did not vary during the experimental session, as the stabilization was kept the same, and the latency and the duration of the flexor inhibitions were identical throughout trial repetitions. The EEG activities analyzed here therefore convey stable central processes underlying the organization of the voluntary command on the one side, and the associated anticipatory postural control on the other.
Mu Rhythm Desynchronization Characterizing the Temporal Organization of Each Command
The topographic representation of the 10- to 13-Hz frequency band showed two power decreases: one over the occipital areas and the other one over the motor areas (Figure 3). Their respective topography differentiates them into the occipital alpha rhythm above the visual areas, related to the visual processing of the stimulus (GO signal), and the sensorimotor mu rhythm over the M1 areas, involved in the production of a motor behavior (Pfurtscheller, 2003). Interestingly, the power decreases of the mu rhythm showed a bilateral and symmetrical spatial distribution focussed over M1. Hence, the topographic maps did not enable to distinguish spatial differences related to distinct controls (motor or postural) applied to the lifting hand and the stabilized forearm.
Time–frequency analysis showed that, before lifting onset, a bilateral desynchronization over M1L and M1R occurred in the alpha rhythm. The role of alpha oscillations still remains a matter of debate. The pre-stimulus alpha activity is likely to be involved in the functional architecture of the brain network when preparing for upcoming processing (Palva and Palva, 2011). According to the “inhibition hypothesis,” information is gated through the brain by functional inhibition of task-irrelevant areas, and is reflected by oscillatory activity in the alpha band (Klimesch et al., 2007; Jensen and Mazaheri, 2010). While increased synchronization would reflect inhibition, desynchronization, which translates the fact that the population of neurons no longer oscillate in synchrony, is interpreted as functionally closely related to active cognitive processing (Klimesch et al., 2007). The functional role of alpha oscillations may also vary along the cortical hierarchy (Palva and Palva, 2011). Within the sensorimotor regions, decreased alpha amplitude is classically taken to reflect a state of increased neuronal excitability, or disinhibition, associated with active-processing (Pfurtscheller, 2003). Moreover, one should also consider the variety of alpha rhythms in terms of functional rhythms (Pfurtscheller, 2003). In particular, the rolandic mu rhythm has been associated to sensorimotor processes in motor tasks (Pfurtscheller, 2003). Indeed, during voluntary unimanual finger movements, a mu ERD starts prior to the movement onset over the contralateral sensorimotor areas (see Pfurtscheller and Lopes da Silva, 1999 for a review). Interestingly, the mu ERD becomes bilateral at the onset of the unimanual movement, but not before (Pfurtscheller and Lopes da Silva, 1999). In our study, the mu rhythm desynchronization over M1L that occurred before unloading onset related to the contralateral hand lifting the object. The mu desynchronization over M1R before unloading should therefore have related to the left postural forearm stabilization. Taken together, this indicates that in the bimanual load-lifting task, the mu desynchronization revealed the anticipatory mechanisms monitoring both the motor and the postural commands.
A comparison of the mu rhythm desynchronization over each M1 showed that distinct features characterized them: over M1R, contralateral to the postural forearm, there was a steeper slope and a greater amplitude, whereas over M1L there was a gradual slope and a reduced amplitude. This result emphasizes the specific and distinct electrophysiological signature of M1R involvement in postural stabilization and M1L involvement in the lifting movement. Lastly, while a mu rhythm desynchronization has until now been the signature of increased cortical excitability related to movement, the present study shows that it might also be the signature of cortical activity related to postural stabilization.
Interestingly, this stabilization is characterized by an active muscular inhibition of the postural flexors, which produces an almost total absence of movement. The flexor inhibition of the postural arm occurs with a high temporal precision and a low inter- and intra-subject variability (Schmitz et al., 2002). By contrast, the contraction of the biceps brachii of the lifting arm occurs with more variability. The postural and manual muscular events therefore present with differences in their temporal variability. A steep slope characterized the mu rhythm desynchronization over M1R, corresponding to the postural arm, whereas there was a gradual decrease in M1L mu rhythm, corresponding to the motor arm. Taken together, this suggests that the temporal precision of the muscle events occurring on the postural and the motor sides might relate to the slope of the mu desynchronization. The rapid and strong desynchronization of the M1R mu rhythm might therefore reflect processes involved in the high temporal precision of the postural inhibition, which is essential to the efficiencies of the APAs. The development of APAs in children is characterized by a progressive mastering of muscular temporal adjustments (Schmitz et al., 2002). Further studies using a developmental approach should confirm the link between the mu rhythm desynchronization and the temporal precision of the muscular event. According to our assumption, if the slope of the mu rhythm desynchronization reflects the high temporal muscle inhibition, a steeper slope should reflect improved accuracy of the postural flexor inhibition onset.
An Activation–Inhibition EEG Pattern Mirroring the Activation–Inhibition Muscular Pattern
In between-hand two-choice reaction tasks, the unimanual motor command is expressed bilaterally: a negative wave is recorded over the motor regions contralateral to the responding hand and is accompanied by a positive wave over the motor regions contralateral to the non-responding hand (Vidal et al., 2003). The contralateral negativity/ipsilateral positivity pattern corresponds to an activation/inhibition pattern of contra- and ipsi-sensorimotor cortices respectively (Burle et al., 2004). In our study, ERPs revealed a negative wave over M1L, contralateral to the manual arm, which reached its maximal value at the onset of muscle contraction (i.e., the abductor pollicis brevis). The initial slope of this motor potential arises from the pyramidal neurons of the motor cortex from which the efferent volley is sent to subcortical structures (Arezzo and Vaughan, 1980). Therefore, this activity might reflect the implementation of the motor command involved in the load-lifting.
Starting at the same time, a positive wave was found over M1R, contralateral to the postural arm. Conversely, this positive wave might convey an active inhibition of the motor cortex contralateral to the postural arm. This would correspond well with the fact that the anticipatory postural command consists of inhibiting the postural muscle activity.
A Specific Role for a Postural M1 in Favor of Independent Control of Posture and Movement
Studies focused on the coordination of bimanual movements have revealed the significant role for interhemispheric coupling between the two sensorimotor cortices (Serrien and Brown, 2002; Blum et al., 2007). Interhemispheric connectivity is dynamically modulated during coordinated behavior as a function of the processing demands. Complex bimanual coordination tasks (anti-phase movements) result in increased interhemispheric coupling, reflecting increased information processing requirements (Serrien, 2008). The bimanual load-lifting task involves another type of complex movement–posture coordination. A fundamental and controversial question is whether the postural and the motor commands are generated by common or independent processes (Massion, 1992). According to Kazennikov et al. (2005), the activation of the M1 contralateral to the lifting hand triggers an interhemispheric transcallosal inhibition of the M1 contralateral to the postural hand, therefore suggesting that a unique command arises from the motor M1 and is transmitted to the postural M1. However, in another posture–movement coordination task in which subjects’ arms were linked, causing flexion about one elbow resulting in extension force about the other, TMS over each motor cortex altered the contralateral (movement-related) EMG response but did not affect the ipsilateral (posture-related) EMG response (Taylor, 2005). These results suggest that the postural and the motor commands develop independently and in parallel. Following Kazennikov and collaborators’ assumption, the mu rhythm desynchronization over the two M1 should present with similar features in terms of amplitude and slope but their onset should differ. However, the interhemispheric comparison between each mu rhythm desynchronization disclosed distinct amplitudes and slopes. Because of their different shape and slope we could not specify which one started first. Nevertheless their time course was also distinct as they varied significantly from the baseline at different times, the mu rhythm desynchronization over the postural M1 coming first.
The ERP findings may be in agreement with Kazennikov et al. (2005) hypothesis, as the M1R inhibition (positive wave) might be caused by the opposite M1L activation (negative wave). However, during a unimanual go/no go task, when the inhibition of the response is required (i.e., in a no go trial), a positive wave over the contralateral M1 can be observed in the absence of a negative wave in the ipsilateral M1. This indicates that the inhibition of the non-involved M1 is independent from the activation of the involved M1 (see Burle et al., 2004 for a review). Thus, our results in both TF and ERPs shed light on the independent role played by the postural M1 during the inhibition of the postural muscles. The specific and opposite recruitment of each M1 is therefore more in line with a parallel and distinct control of movement and posture.
To conclude, the two EEG analyses performed in this study highlight a specific mu rhythm desynchronization and a positive wave over the M1 contralateral to the postural arm as the expression of the electrophysiological signature of APAs. While the positive wave might be at the origin of the transmission of the inhibitory commands toward the postural flexor muscles, the mu rhythm desynchronization over the postural M1 might reflect the processes involved in the high temporal precision enabling efficient APAs. The specific and opposite recruitment of each M1, confirmed by the two EEG approaches, seems to be in line with the “parallel control” in which the APAs and the movement are initiated by separate processes and are organized independently over each primary motor cortices (Massion, 1992).
Conflict of Interest Statement
The authors declare that the research was conducted in the absence of any commercial or financial relationships that could be construed as a potential conflict of interest.
Acknowledgments
The authors wish to thank the CNRS for its financial support. They are grateful to all the subjects who participated in this study and to Melanie Cole for revising the English manuscript.
References
Arezzo, J., and Vaughan, J. (1980). Intracortical sources and surface topography of the motor potential and somatosensory evoked potential in the monkey. Prog. Brain Res. 77–83.
Blum, J., Lutz, K., and Jäncke, L. (2007). Coherence and phase locking of intracerebral activation during visuo- and audio-motor learning of continuous tracking movements. Exp. Brain Res. 182, 59–69.
Bouisset, S., and Zattara, M. (1981). A sequence of postural movements precedes voluntary movement. Neurosci. Lett. 22, 263–270.
Burle, B., Vidal, F., Tandonnet, C., and Hasbroucq, T. (2004). Physiological evidence for response inhibition in choice reaction time tasks. Brain Cogn. 56, 153–164.
Cochin, S., Barthelemy, C., Roux, S., and Martineau, J. (2001). Electroencephalographic activity during perception of motion in childhood. Eur. J. Neurosci. 13, 1791–1796.
Delorme, A., and Makeig, S. (2004). EEGLAB: an open source toolbox for analysis of single-trial EEG dynamics including independent component analysis. J. Neurosci. Methods 134, 9–21.
Delorme, A., Sejnowski, T., and Makeig, S. (2007). Enhanced detection of artifacts in EEG data using higher-order statistics and independent component analysis. Neuroimage 34, 1443–1449.
Diedrichsen, J., Verstynen, T., Lehman, S. L., and Ivry, R. B. (2005). Cerebellar involvement in anticipating the consequences of self-produced actions during bimanual movements. J. Neurophysiol. 93, 801–812.
Dufosse, M., Hugon, M., and Massion, J. (1985). Postural forearm changes induced by predictable in time or voluntary triggered unloading in man. Exp. Brain Res. 60, 330–334.
Gastaut, H., Terzian, H., and Gastaut, Y. (1952). Etude d’une activité éléctroencephalographique méconnue: le rythme rolandique en arceau. Mars. Med. 89, 296–310.
Graimann, B., and Pfurtscheller, G. (2006). Quantification and visualization of event-related changes in oscillatory brain activity in the time-frequency domain. Prog. Brain Res. 159, 79–97.
Hugon, M., Massion, J., and Wiesendanger, M. (1982). Anticipatory postural changes induced by active unloading and comparison with passive unloading in man. Pflugers Arch. 393, 292–296.
Jensen, O., and Mazaheri, A. (2010). Shaping functional architecture by oscillatory alpha activity: gating by inhibition. Front. Hum. Neurosci. 4:186. doi:10.3389/fnhum.2010.00186
Kazennikov, O., Solopova, I., Talis, V., Grishin, A., and Ioffe, M. (2005). TMS-responses during anticipatory postural adjustment in bimanual unloading in humans. Neurosci. Lett. 383, 246–250.
Klimesch, W., Sauseng, P., and Hanslmayr, S. (2007). EEG alpha oscillations: the inhibition-timing hypothesis Brain Res. Rev. 53, 63–88.
Martineau, J., Schmitz, C., Assaiante, C., Blanc, R., and Bartélémy, C. (2004). Impairment of a cortical event-related desynchronisation during a bimanual load-lifting task in children with autistic disorder. Neurosci. Lett. 367, 298–303.
Massion, J. (1992). Movement, posture and equilibrium: interaction and coordination. Prog. Neurobiol. 38, 35–56.
Massion, J., Ioffe, M., Schmitz, C., Viallet, F., and Gantcheva, R. (1999). Acquisition of anticipatory postural adjustments in a bimanual load-lifting task: normal and pathological aspects. Exp. Brain Res. 128, 229–235.
Ng, T., Sowman, P. l., Brock, J., and Johnson, B. (2011). Premovement brain activity in a bimanual load-lifting task. Exp. Brain Res. 208, 189–201.
Palva, S., and Palva, J. M. (2011). Functional roles of alpha-band phase synchronization in local and large-scale cortical networks. Front. Psychol. 2:204. doi:10.3389/fpsyg.2011.00204
Pernier, J., Perrin, F., and Bertrand, O. (1988). Scalp current-density fields – concept and properties. Electroencephalogr. Clin. Neurophysiol. 69, 385–389.
Perrin, F., Pernier, J., Bertrand, O., and Echallier, J. F. (1989). Spherical splines for scalp potential and current density mapping. Electroencephalogr. Clin. Neurophysiol. 76, 565–566.
Pfurtscheller, G. (2003). Induced oscillations in the alpha band: functional meaning. Epilepsia 44, 2–8.
Pfurtscheller, G., and Lopes da Silva, F. H. L. (1999). Event-related EEG/MEG synchronization and desynchronization: basic principles. Clin. Neurophysiol. 110, 1842–1857.
Schmitz, C., Jenmalm, P., Westling, G., Ehrsson, H., and Forssberg, H. (2005). Anticipatory postural adjustments in a bimanual load-lifting task: central aspects. Gait Posture 21.
Schmitz, C., Martin, N., and Assaiante, C. (2002). Building anticipatory postural adjustment during childhood: a kinematic and electromyographic analysis of unloading in children from 4 to 8 years of age. Exp. Brain Res. 142, 354–364.
Serrien, D. J. (2008). Coordination constraints during bimanual versus unimanual performance conditions. Neuropsychologia 46, 419–425.
Serrien, D. J., and Brown, P. (2002). The functional role of interhemispheric synchronization in the control of bimanual timing tasks. Exp. Brain Res. 147, 268–272.
Tallon-Baudry, C., and Bertrand, O. (1999). Oscillatory gamma activity in humans and its role in object representation. Trends Cogn. Sci. (Regul. Ed.) 3, 151–162.
Taylor, J. L. (2005). Independent control of voluntary movements and associated anticipatory postural responses in a bimanual task. Clin. Neurophysiol. 116, 2083–2090.
Van Boxtel, G., Geraats, L., Van den Berg-Lenssen, M., and Brunia, C. H. M. (1993). Detection of EMG onset in ERP research. Psychophysiology 30, 405–412.
Viallet, F., Massion, J., Massarino, R., and Khalil, R. (1992). Coordination between posture and movement in a bimanual load lifting task: putative role of a medial frontal region including the supplementary motor area. Exp. Brain Res. 88, 674–684.
Keywords: anticipation, posture, coordination, motor, mu desynchronization, ERP, EMG, kinematics
Citation: Barlaam F, Descoins M, Bertrand O, Hasbroucq T, Vidal F, Assaiante C and Schmitz C (2011) Time–frequency and ERP analyses of EEG to characterize anticipatory postural adjustments in a bimanual load-lifting task. Front. Hum. Neurosci. 5:163. doi: 10.3389/fnhum.2011.00163
Received: 22 July 2011;
Accepted: 22 November 2011;
Published online: 14 December 2011.
Edited by:
Francisco Barcelo, University of Illes Balears, SpainReviewed by:
Juliana Yordanova, Bulgarian Academy of Sciences, BulgariaLutz Jäncke, University of Zurich, Switzerland
Copyright: © 2011 Barlaam, Descoins, Bertrand, Hasbroucq, Vidal, Assaiante and Schmitz. This is an open-access article distributed under the terms of the Creative Commons Attribution Non Commercial License, which permits non-commercial use, distribution, and reproduction in other forums, provided the original authors and source are credited.
*Correspondence: Christina Schmitz, INSERM U1028, CNRS UMR5292, Brain Dynamics and Cognition Team, Lyon Neuroscience Research Center, 69675 Bron Cedex, Marseille, France. e-mail:Y2hyaXN0aW5hLnNjaG1pdHpAaW5zZXJtLmZy