- 1Department of Rehabilitation Medicine, Center for Brain Plasticity and Recovery, Georgetown University and MedStar National Rehabilitation Hospital, Washington, DC, USA
- 2Department of Neurology, Georgetown University, Washington, DC, USA
- 3Department of Kinesiology and Occupational Therapy, University of Wisconsin, Madison, WI, USA
- 4Department of Biostatistics, Georgetown University, Washington, DC, USA
- 5Department of Neuroscience, Georgetown University, Washington, DC, USA
- 6Departments of Oncology and Biochemistry, Georgetown University, Washington, DC, USA
- 7Department of Neurology, University of Rochester, Rochester, NY, USA
Introduction: Seven hundred ninety-five thousand Americans will have a stroke this year, and half will have a chronic hemiparesis. Substantial animal literature suggests that the mammalian brain has much potential to recover from acute injury using mechanisms of neuroplasticity, and that these mechanisms can be accessed using training paradigms and neurotransmitter manipulation. However, most of these findings have not been tested or confirmed in the rehabilitation setting, in large part because of the challenges in translating a conceptually straightforward laboratory experiment into a meaningful and rigorous clinical trial in humans. Through presentation of methods for a Phase II trial, we discuss these issues and describe our approach.
Methods: In rodents there is compelling evidence for timing effects in rehabilitation; motor training delivered at certain times after stroke may be more effective than the same training delivered earlier or later, suggesting that there is a critical or sensitive period for strongest rehabilitation training effects. If analogous critical/sensitive periods can be identified after human stroke, then existing clinical resources can be better utilized to promote recovery. The Critical Periods after Stroke Study (CPASS) is a phase II randomized, controlled trial designed to explore whether such a sensitive period exists. We will randomize 64 persons to receive an additional 20 h of upper extremity therapy either immediately upon rehab admission, 2–3 months after stroke onset, 6 months after onset, or to an observation-only control group. The primary outcome measure will be the Action Research Arm Test (ARAT) at 1 year. Blood will be drawn at up to 3 time points for later biomarker studies.
Conclusion: CPASS is an example of the translation of rodent motor recovery experiments into the clinical setting; data obtained from this single site randomized controlled trial will be used to finalize the design of a Phase III trial.
Background
Using animal models of stroke, substantial scientific progress has been made in the understanding of the neural substrates of recovery after brain injury. Experimental studies of motor training after injury show that motor function can be improved significantly when a number of recovery and training variables are controlled. The experiment of Biernaskie et al. (2004) has been particularly intriguing given the finding of a sensitive period after experimental stroke in which rodents are most responsive to motor training in a specific time window soon after stroke. This finding has provoked much discussion in the stoke rehabilitation research community, since of course one wants to rehabilitate stroke patients at the time after stroke when therapies can be most effective. In this paper, we discuss the challenges faced by clinical trialists in translating a conceptually straightforward rodent experiment into a stroke rehabilitation clinical trial. We present our methods for the Critical Periods after Stroke Study (CPASS) as one example of the choices that can be made in testing whether promising findings in rodents have relevance in rehabilitation of patients with stroke.
The CPASS trial is designed to translate important findings from the rodent motor recovery literature into the human clinical trial setting. Adapting the critical elements of the rodent studies to the stroke rehabilitation setting requires a series of decisions and accommodations. In this paper, we review and discuss these considerations and how we have addressed them. Where possible we have retained essential elements of the rodent studies, including manipulation of intervention timing, randomization, standardized motor training paradigm based on a highly salient reward, and the use of motor performance measures. Data obtained from this randomized controlled trial will be used to formulate more effective treatments to better focus on the needs of individuals with stroke.
Approaching the Translation of Animal Experiments into Clinical Trials
Table 1 displays the many advantages of rodent experiments; these advantages allow exacting study of the biology of mammalian brain recovery, and the most unequivocal demonstration of the impact of putative motor training interventions. The rodents can be healthy young animals predictably available through breeding or purchase, eliminating confounds of differences in rearing, medical conditions and post-injury mortality. The ability to test a group of subjects simultaneously eliminates any drift in study or training procedures. Heterogeneity across animal subjects can be limited by the use of a single gender and a genetically homogenous strain. Brain lesions can be standardized and made in a brain that is otherwise pristine. Motor training protocols can be uniform and timed exactly. Food can be used as a highly motivating reward, and subjects are not lost to follow-up. Biological mechanisms can be studied using tissue and molecular techniques requiring sacrifice of the animals.
Designing a human stroke motor recovery trial tightly linked to the methods used in rodent motor recovery experiments involves a series of adaptations. These adaptations attempt to minimize the real-world limitations of clinical research and to maximize the clinical and scientific utility. The middle column of Table 1 displays some of the challenges faced by clinical trialists as they adapt these experiments to the clinical setting. A simple direct translation of rodent methods into humans can result in a trial that would be straightforward to design, but impractical to execute. For example, an investigator may want to insist that a single, specific lesion type be present for an individual to enroll in a clinical trial. This insistence might be scientifically justifiable, but impossible to execute because of the difficulty of finding sufficient numbers of individuals who suffered the needed infarct, meet other inclusion criteria, and are willing and able to participate in a trial. Similarly, challenges exist in enforcing exact timing of treatments, the content of treatments, obtaining motivated participation in training, and simply locating the individual to collect outcome measures. Approaching the biology of brain recovery in humans is also more challenging because of the infeasibility of recovering brain tissue; even lumbar punctures limit large scale participation in trials.
Clinical trial methods can mitigate the limitations of the stroke rehabilitation clinical setting; many examples are listed in the third column of Table 1. For example, the problem of identifying large numbers of participants can be limited through the use of adaptive trial designs, ensuring that participants will be randomized only to study arms that are promising. Less stringent inclusion/exclusion criteria can increase participant accrual, and the accompanying increase in heterogeneity across subjects can be managed using adaptive randomization strategies to minimize differences between study groups. Treatments that begin on a single preselected day in animals are not realistic in the fluid and unpredictable clinical setting, but can be replaced by treatment initiation intervals, allowing flexibility to the participant and research team. In other cases, investigators must simply make choices based on knowledge of the population, clinical setting, or treatment techniques. The number of choices can be quite large, and often the importance of individual choices is visible only in retrospect at the end of an expensive multiyear effort to answer what to all initial appearances is a straightforward question.
Laboratory-based Work in Critical Periods after Stroke
In current practice, as it becomes possible for the patient to participate after stroke, rehabilitation begins. This rehabilitation is initially superimposed on a background of resolving brain edema, inflammation and apoptosis, which are not thought to be materially influenced by experiences such as motor training (Carmichael, 2006; Cramer, 2008).
In contrast, rehabilitation itself is a mixture of compensation and learning. New learning, particularly that obtained via activity-based therapies (ABT's) (Dromerick et al., 2006), is thought to be accomplished by experience driven neuroplasticity (Kleim and Jones, 2008; Carter et al., 2010). The patient relearns prior methods of accomplishing everyday tasks and when necessary, learns new ways to accomplish goals through a combination of newly acquired compensatory strategies (Nakayama et al., 1994) and restoration of motor, sensory, and cognitive function in uninjured tissues (Lum et al., 2004, 2009; Levin et al., 2009). Since these processes often do not return the patient back to pre-stroke levels of function, understanding and exploiting animal findings of critical or sensitive periods in rehabilitation is an important approach to improving treatment.
These putative periods of greatest responsiveness after stroke have been hypothesized to be analogous to the “critical periods” in normal development (Murphy and Corbett, 2009). In the developing brain, critical periods are defined as times of greatest sensitivity to exogenous influences or experiences. Critical periods for the effect of experience on the formation of neural circuits and on the behaviors they control have been demonstrated, for example, in the establishment of ocular dominance columns and stereopsis in the visual system (Hubel and Wiesel, 1970), in the formation of attachment and species identification in a variety of avian species (Hess, 1973), and in vocal learning in songbirds (Marler, 1970) and in humans (Johnson and Newport, 1989; Newport, 1990). The molecular mechanisms underlying the opening and closing of developmental critical periods are beginning to be well understood (Hensch, 2005), and there are now even examples of “reopening” early critical periods during adulthood (Bavelier et al., 2010; Zhou et al., 2011).
The work of Biernaskie et al. (2004) suggests that certain periods after stroke may constitute a period of enhanced plasticity, analogous to a critical period during which the recovering brain is most sensitive to exogenous stimuli and experience. Thus, there may be an optimal time when stroke patients might show the largest improvement from therapy; and, should stroke patients not receive this optimally timed therapy, it is possible that the opportunity for optimal recovery could be irrevocably lost. Given the discontinuities in US health care, it is common for patients' therapy to be delayed for personal, medical or insurance reasons (Ostwald et al., 2009); even inpatient rehabilitation admission does not guarantee substantial amounts of motor training (Lang et al., 2009). Carefully executed studies demonstrating the optimal timing of therapies will help clinicians and policymakers ensure delivery of effective rehabilitation.
Most of the evidence regarding the timing effects of post-stroke motor training focuses on the behavioral, cellular, and molecular mechanisms of neuroplasticity. More recently, animal models demonstrate that genes involved in normal development (and that are quiescent in adulthood) are expressed at high levels in the first weeks after stroke and then decline, with distinct temporal patterns of gene expression after injury (Carmichael, 2003, 2006). This pattern of gene expression is consistent with the notion of an injury-induced recapitulation of development-like processes which occur during a period of enhanced plasticity. Most of these findings focus on the first weeks after stroke; our study design has three relevant time points (early/acute, subacute, and chronic), in order to best assess and locate such an effect, if indeed it occurs in human patients.
There are two major findings regarding treatment timing in animal models of stroke. First is the work of Schallert (Kozlowski et al., 1996; Humm et al., 1998) and others (Bland et al., 2000) showing that very early and intensive training can reduce recovery after experimental stroke and enlarge lesions. This may have been confirmed in humans in our own work (Dromerick et al., 2009), when we found that very intense motor training early after stroke led to worse outcomes. Second, and more optimistic is the work of Biernaskie et al. (2004) where the question of timing effects was directly addressed. They randomized lesioned animals to receive focused motor training at 5, 14, or 30 days after lesioning. They found that the best response to training started at 5 days after lesioning; an intermediate response was present when training was initiated at 14 days; and therapy beginning at 30 days resulted in the same motor outcome as controls who were not trained at all. This powerful pattern of results suggests that critical periods in stroke recovery do exist in adult mammals (Murphy and Corbett, 2009).
Human and Clinical Data regarding Timing Effects in Rehabilitation Treatment
Whether and how the results of Biernaskie et al. translate to human stroke patients is unknown. Few prospective human studies directly address optimal timing of rehabilitation. Natural history studies show that recovery after stroke in humans is fastest in the first weeks (Wade and Hewer, 1987; Jorgensen et al., 1995a,b); this period coincides with both the onset of rehabilitation treatment and the time that homeostasis is re-established, as described above. Clinicians have written for decades regarding the features of motor recovery that seem to resemble patterns of normal motor development (Cramer and Chopp, 2000; Pollock et al., 2007; Kollen et al., 2009). Retrospective data from clinical populations suggest that early initiation of rehabilitation is associated with better outcome (Wylie, 1970; Feigenson et al., 1977; Kotila et al., 1984; Rossi et al., 1997). However, these studies are confounded because patients who present late to rehabilitation are generally sicker and more severely affected, and thus less likely to improve regardless of timing of care (Ween et al., 1996). Some, but not all (Gagnon et al., 2006) newer studies using case control methods (Paolucci et al., 2000) or large multicenter cohorts (Maulden et al., 2005) have also found better responses early.
Secondary analyses of existing clinical trials are mixed. The EXCITE trial (Wolf et al., 2006) evaluated whether constraint therapy was superior to an uncharacterized “usual and customary care” (UCC) control in improving UE motor impairment; secondary analyses suggested that the participants treated earlier had a better motor outcome than those treated later (Wolf et al., 2010). The LEAPS trial (Duncan et al., 2011) of body-weight supported treadmill training for gait did not confirm a timing effect. LEAPS found that there were persistent treatment responses at both time points tested (2 and 6 months), but there were no significant outcome differences between the earlier and later groups. VECTORS, a single center Phase II trial (Dromerick et al., 2009) of constraint therapy early after stroke addressed dosing and therapy content rather than timing, but the results at this earlier time period suggested an inverse dose response relationship (at high doses, more therapy led to less motor recovery). A more recent study testing additional rehabilitation therapy early after stroke did not confirm this inverse dose phenomenon and suggested greater ipsilesional cortical activation on functional MRI in those randomized to extra therapy (Hubbard et al., 2014). A recent trial in ICH patients suggested a possible mortality benefit with early therapy (Liu et al., 2014). Preliminary data from AVERT (Bernhardt et al., 2008), an early mobilization RCT, are promising but enrollment is still ongoing.
Study Rationales and Hypotheses
The overall goal for the CPASS trial is to identify a critical period after stroke in which patients are particularly responsive to motor training interventions. We hope simply to elicit a signal that a critical period exists; optimization of dosing or treatment strategy would come in subsequent studies.
Our approach is to use a bolus of standardized motor therapy to elicit a motor improvement during a specific time period indicative of a critical period. Our hypothesis for the CPASS Phase II trial is that, compared to individuals randomized to the control condition or to the subacute (2–3 months after onset) or chronic (6–9 months after onset) time points, persons randomized to early intensive motor training will show greater UE motor improvement measured at 1 year. In addition, we will use the opportunity presented to collect peripheral blood to perform a proof of principle study exploring molecular signals associated with response to treatment and overall motor recovery. See Figure 1 for a diagram of study design.
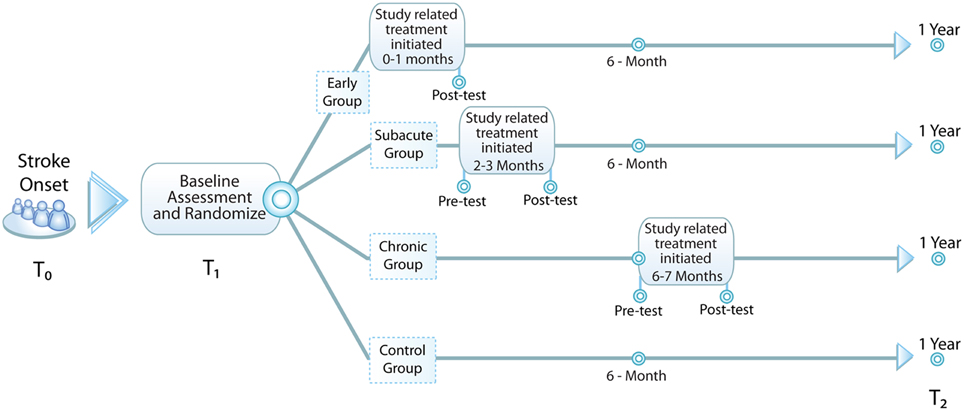
Figure 1. Study design. Baseline assessment (T1) occurs within the first month following stroke and subjects are randomized to one of four groups: early—additional 20 h of occupational therapy (OT) initiated <1 mo. from stroke onset, subacute—additional 20 h of OT initiated 2-3 mo. from stroke onset, chronic—additional 20 h of OT initiated 6-7 mo. from stroke onset, and control—no additional OT. All subjects are reassessed at 1 year (T2) to evaluate for durable change in study-related outcome measures.
In order to adapt the rodent experimental design to the clinical delivery patterns in the United States, we made two major decisions. First was the choice of time periods in which study-related treatment would be delivered. Precisely how post-stroke days compare between humans and rodents is unknown, and we attempted to balance fidelity to the Biernaskie et al. design with the pragmatics of accommodating existing treatment venues. These venues are not under the control of the research team. Choosing the exact time points and a single day window to initiate therapies such as was used in the rodent study meant that participants would need to be consented within 72 h of stroke onset so that baseline measures could be collected and the participant randomized with the possibility of treatment beginning exactly on post-stroke Day 5. Though conceptually not impossible, this choice would lead to several complexities including unavailability of patients to undergo study related therapy during a time when diagnostic testing must take first priority, medical complications and fatigue preventing therapy participation, uncertainty about the trajectory of motor recovery, and uncertainty as to whether and where the patient might be referred for inpatient rehabilitation. We chose instead three more flexible windows of time for study related treatments: early (<30 days, corresponding to the inpatient rehabilitation period), subacute (60–90 days, corresponding to typical outpatient therapy delivery), and chronic (6 months, by which time most US stroke patients will have been discharged from therapy). These times were chosen as analogous to the 5, 14, and 30 day times used in the Biernaskie et al. study. By using those existing clinical treatment venues, any improvements in efficacy that results from this line of work can improve the effectiveness of those venues without requiring a major change in how care is delivered. Thus, the translation to actual treatment would not be hindered by the need for policy and reimbursement changes.
The second major decision was that of how much study related treatment was necessary to observe a detectable effect of a critical period. Dose-response data for motor training are particularly lacking in the first few weeks after stroke onset. We chose 20 h of additional motor therapy because our previous work has shown that a difference of 10 h of treatment is sufficient to alter motor outcomes; this amount of additional therapy should thus provide an adequate signal indicating a critical period, if there is one (Dromerick et al., 2009). Moreover, should we find a large difference in outcomes in one group, it seems feasible to deliver 20 h more training to stroke patients in the current healthcare environment. Several studies document persistent motor improvements post-stroke with treatments of similar or even less intensity (Sivenius et al., 1985; Sunderland et al., 1992; Whitall et al., 2000; Page et al., 2001; Michaelsen and Levin, 2004; Michaelsen et al., 2006; Woldag et al., 2010; Han et al., 2013).
Methods
Patient Population
Sixty-four participants will be recruited primarily from the inpatient stroke service at MedStar National Rehabilitation Hospital (MedStar NRH). MedStar NRH hosts the Washington DC site for the NINDS StrokeNet, and recruitment from other DC area hospitals can be used if needed. Study participants will include adults with neuroimaging confirmed diagnosis of recent ischemic stroke or intraparenchymal hemorrhage. See Table 2 for a comprehensive list of inclusion/exclusion criteria.
Study Measures
The primary outcome measure, secondary outcome measures, and covariates are listed in Table 3 along with the time periods at which they will be assessed. Pre-treatment, 6 mo, and 1 yr assessments will be performed by study therapists and the assessments for the motor scales will be videotaped. A blinded therapist will view the videotapes to provide the formal assessments for each subject at the end of the study, including the ARAT primary outcome measure.
Further information on study outcome measures and covariates are provided below:
• Action Research Arm Test (ARAT): The ARAT, adapted from the Fugl-Meyer (Fugl-Meyer et al., 1975), assesses functional limitations of the UE and is the primary outcome measure for the study. It uses a 4-point ordinal scale on 19 items, where 0 indicates no movement and 3 indicates normative movement. The scale has 4 subscale scores- gross motor (9 point maximum), grasp (18 point maximum), grip (12 point maximum), and pinch (18 point maximum)- and a total scale score with a maximum of 57. The ARAT has been shown to be reliable, valid, and responsive to change across a variety of time points post-stroke (Deweert and Harrison, 1985; Hsieh et al., 1998; Van der Lee et al., 2001, 2002; Lang et al., 2006).
• Motor Activity Log (MAL): The MAL is a 28-item questionnaire measuring UE use on functional tasks (Uswatte et al., 2006; Taub et al., 2011). It has two subscales: Amount of Movement (AoM) and Quality of Movement (QoM).
• Nine Hole Peg Test: The nine hole peg test measures bimanual dexterity. The test assesses the amount of time required to position and remove nine (9) pegs on a board (Mathiowetz et al., 1992).
• Functional Independence Measure (FIM): The FIM assesses 18 item activities of daily living (Granger et al., 1993). Two subscores are created: a motor score and a cognitive score. Subscales will be analyzed separately.
• Motor Assessment Scale: The Motor Assessment Scale is a test which assesses an individual's supine to side lying, supine to sitting edge of bed, balanced sitting, sitting to standing, walking, upper-arm function, hand movements and advanced hand movements (Carr, 1992). For this study the upper-limb function portion of this test will be utilized.
• Barthel Index: The Barthel Index is the most commonly used measure of functional performance in stroke clinical trials (Shah et al., 1989).
• Upper Extremity Fugl-Meyer (UE-FM): The Fugl-Meyer will be used to assess motor function at the shoulder, elbow, wrist, and fingers (Fugl-Meyer et al., 1975). It scores reflexes and the ability to perform several simple movements and tasks on a 3-point scale. Validity and reliability of the Fugl-Meyer Test have been established (Lin et al., 2009). The Fugl-Meyer was designed for the recovery patterns observed after stroke and is most sensitive to detecting changes in subjects with moderate to severe impairment.
• Manual Muscle Test (MMT): The Manual Muscle Test evaluates strength and function of individual muscles to assess the degree of muscular weakness resulting from injury or disease (Medical Research Council, 1976). The therapist palpates each muscle while the subject voluntarily contracts the muscle against resistance. A score ranging from 0 to 5 indicates the relative strength.
• Motricity Index: The Motricity Index is a short and simple measure of motor loss primarily developed for use after stroke (Collen, 1992). This measure has proven validity and reliability and is sensitive to change in motor function after stroke.
• Stroke Impact Scale (SIS): (SIS Hand Function Subscale and SIS Recovery Scale): The SIS is a self-report scale for evaluating stroke recovery (Duncan et al., 1999). The 5-item Hand Subscale assesses hand strength, dexterity, fine, and gross motor ability associated with the ability to complete functional tasks. In the Recovery Subscale, the patient is asked to assess his or her overall degree of recovery; possible scores range from 0 to 100 with higher scores indicative of better outcomes.
• Modified Rankin Scale (mRS): The mRS measures the degree of disability and level of independence in those who suffer from neurologic injury (van Swieten et al., 1988). In addition to serving as a secondary outcome measure, the mRS will be used to gauge pre-stroke level of disability.
• Activity Card Sort (ACS): The ACS is a self-report measure of activity in four domains: instrumental activities, high and low physical-demand leisure activities, and social activities (Baum and Edwards, 2008).
• Reintegration to Normal Living: This 10 item Likert scale evaluates life satisfaction and quality of life (Stark et al., 2005).
• Geriatric Depression Scale: The 5 items version of the Geriatric Depression Scale will be used to screen for depression (Yesavage, 1988).
• NIH Stroke Scale (NIHSS): The NIHSS assesses cognitive, sensory, and motor impairments as an indicator of overall stroke severity (Brott et al., 1989).
• Short Blessed Orientation Memory Concentration Test: This test screens for dementia by assessing orientation, reasoning and short-term memory (Katzman et al., 1983).
• Mesulam Symbol Cancellation Test- This paper and pencil measure uses a cancelation task to assess visuospatial neglect (Mesulam, 1985). More than 3 omissions between the left and right visuospatial field indicates neglect.
• Pain Scale: A scale with cartoon faces that represent varying degrees of pain. The subject is asked which face reflects their current pain level (Wong et al., 2001).
• Charlson Comorbidity Index: An index that factors in a subject's medical comorbidities and age to derive a probability of 10 year survival (Charlson et al., 1987).
• Oxfordshire Classification: Classifies stroke based on neuroimaging/clinical data according to 4 possible stroke locations/severities: total anterior circulation stroke, partial anterior circulation stroke, posterior circulation stroke, and lacunar stroke (Mead et al., 2000).
• Edinburgh Inventory: A questionnaire that determines the degree to which a subject is left- or right-handed (Oldfield, 1971).
Procedures
The trial was approved by the Institutional Review Board at MedStar National Rehabilitation Hospital/MedStar Health Research Institute (protocol #2014-065). All study subjects will provide informed consent prior to engaging in study procedures. Based on recruitment rates for the ICARE trial (Winstein et al., 2013), which was conducted at the same hospital and had somewhat similar inclusion/exclusion criteria, we anticipate all subjects for CPASS will be recruited by the end of year 2 and final outcome measures will be collected by the end of year 3.
Randomization
The approach to randomization offers an opportunity to mitigate some of the limitations of stroke rehabilitation clinical trials. Specifically, randomization allows the investigator to decrease the impact of heterogeneity of important personal characteristics among subjects that could affect the overall study results. Ideally, all important and measurable characteristics could be balanced across the study, but there are limits to the number of variables that can be balanced, short of enrolling a sample far larger than circumstances permit. To avoid groups that are unbalanced with respect to potentially important secondary variables, we use an adaptive randomization strategy based on methods described by Atkinson (1982), Meinert (1986), and Signorini et al. (1993). The adaptive randomization scheme (Yuan et al., 2014) that we have developed increases to six the number of variables that can be balanced in this sample of 64 subjects. We have chosen to balance these variables that we have judged to be most important: age, number of days from stroke onset to baseline evaluation, ischemic vs. hemorrhagic stroke type, baseline ARAT score, concordance (dominant vs. non-dominant UE affected) and overall stroke severity as indicated by NIHSS score at baseline evaluation. We were limited to six variables to balance; we prioritized these over other potentially important variables such as the presence of a fully recovered prior stroke in the randomization. Cortical involvement was another potential randomization variable that we chose not to address because neuroimages are not always available at our center, and resources did not allow for study-related imaging. By balancing days from stroke onset to baseline evaluation, we minimize the impact of variability of time from onset to rehabilitation admission.
Interventions
In designing the motor training intervention for CPASS, we addressed several goals for mitigating the limitations of clinical trials. These included motivating subjects as well as developing a standard treatment approach that is theory driven, measurable, and reproducible. Ideally, the treatment should be engaging and meet the clinical goals and interests of the individual participant.
One important advantage of rodent studies over human trials is the ability of the investigator to motivate maximal engagement and participation by study subjects. Rodent and human motor training paradigms are based on the work of Thorndike who proposed the “law of effect” (Thorndike, 1898). The law of effect suggests that responses closely followed by satisfaction will become firmly attached to the situation and therefore will be more likely to recur when the situation is repeated. This principle forms the basis of operant conditioning (Skinner, 1938). Operant conditioning experiments of motor training in rodents have shown that food is a powerful form of reinforcement in motor learning studies. Studies demonstrated that food pellets as positive reinforcers produced more rapid motor learning and better retention of learned responses than foot shocks as negative reinforcers in rodents (Lawson and Watson, 1963). Animals receiving highly desirable food pellets learned faster and retained more than those who were reinforced with minimally acceptable pellets. These principles form the foundation of the translation of rodent models of stroke recovery to the present treatment protocol: the use of highly salient reinforcement procedures to reinforce motor training activities.
In a human clinical trial, the use of delayed feeding and food rewards to increase motivation is not feasible. Instead, we developed an individualized treatment protocol based on the UE functional activity preferences of study participants. To standardize the elicitation of an individual participant's preferred activities, we used a widely accepted measure of leisure and functional activities. Sixty-five functional activities requiring upper extremity performance assessed by the Activity Card Sort (ACS) (Baum and Edwards, 2008) minus 24 of those activities that do not involve upper extremity involvement are presented during the treatment-planning phase in order to identify pre-stroke social, instrumental, high and low demand leisure UE activities. Study participants are asked to identify and rank the 10 most important activities that require arm and hand skills that they want to focus on during their treatment. Study participants are also able to add activities not included in the ACS. Participants rank the importance of each activity. Study therapists use these activities to develop a specific treatment plan based on a series of shaping tasks that are described below. These tasks are used for the treatment of all participants. Thus, there is customization for the goals and priorities of individual participants, but uniformity of actual motor training using the library of shaping tasks. All study participants will receive their usual and customary care (UCC) treatment as prescribed by the clinical team and approved by their insurance company. Data are collected on the number and type of UCC therapy sessions, but no attempt will be made to standardize UCC. Study related therapies will be delivered by research-employed certified physical or occupational therapists; the study team meets twice weekly to review treatment related issues and insure reproducibility.
Persons randomized to the early group (<30 days) have study-related treatments started as quickly as possible; those randomized to a later time point will have their treatment started as close as possible to the first day of their treatment window (2 mo. or 6 mo. post-stroke for the subacute and chronic groups respectively). By concentrating therapy for the early group as much as feasible, overlap with the subacute group will be minimized or eliminated. Study related treatments will be delivered in addition to UCC, and will consist of 20 h of therapy delivered in 10 two-hour sessions 2-3x/week. In the United States, stroke patients admitted to the acute inpatient rehabilitation setting are generally required to receive 3 h of therapy daily; however a multisite study (Lang et al., 2009) demonstrated a paucity of time devoted to restorative motor training that was remarkably consistent at less than 10 min daily. Thus, the additional study-related training overwhelms any differences in the amount of UCC training delivered to individual study participants. To avoid reproducing the High Intensity condition used in the VECTORS trial, individuals randomized to the early/acute group will only receive 1 additional hour of treatment per session until discharge from in-patient rehab, and then will receive 2 h of treatment per session similar to the subacute and chronic groups. This training will focus exclusively on UE motor training, and the use of the more affected UE in the activities identified by the baseline. This treatment will be delivered in the inpatient rehab setting (for those randomized to the early group) or outpatient clinic settings (for subacute and chronic groups) whenever possible. If needed, the study therapy will be provided in the home to achieve the requisite 20 h of treatment.
Balancing the need to customize study-related treatment to the goals and preferences of individual participants (thereby maximizing motivation) while at the same time delivering a measurable, reproducible, and theory driven motor training program is important. The shaping treatment developed for use in VECTORS, an NINDS-funded Phase II trial (Dromerick et al., 2009) will be included in the 20 h of therapy. Constraint will not be used. Shaping is a process used in both human and rodent motor training after stroke. There are many theories of why shaping produces lasting changes in motor performance (Taub et al., 1994; Ingvaldsen, 1997; Winstein et al., 2013). The selection of tasks is very important since the participant must be committed to achieving the goal despite the difficulty of task performance. The therapist grades the tasks to provide enough challenge to encourage progress while guarding against frustration and failure which act as negative reinforcers and decrease the probability of full participation in the treatment program. The therapist offers positive reinforcement in the forms of verbal and visual feedback. Task difficulty is increased when the participant completes two sets of 10 correct repetitions of task performance on two consecutive days of treatment.
Statistical Analysis
Power analysis estimated that a sample size of 64 (16 per study arm) is sufficient to test the study hypotheses based on the standard deviations and treatment differences observed in the VECTORS trial. The study sample size is determined based on demonstrating a moderate effect size (0.425 as observed in the preliminary data) in the primary endpoint, the ARAT at 1 year in an ANOVA model, with 80% power at a significance level of 5%. The specific comparison of the treatment at each time point will be conducted using contrasts. One interim analysis is planned when the number of patients with 1 year ARAT reaches 32. This analysis following the adaptive group sequential procedure allows us the opportunity to spot treatment time points with pronounced improvement in ARAT or the lack thereof while maintaining the rigor (e.g., Type I error) of the trial.
The general linear model (ANOVA) will be used for group comparisons on the primary endpoint ARAT using intent to treat principle. All subjects recruited into the study will be included in this primary analysis except the run-in participants. Additional per protocol secondary analyses will be performed. The timing of treatment hypothesis will be addressed by contrasts between the four treatment groups at 1 year after stroke. The magnitude of these effects will be computed. The analysis on secondary endpoints will be mostly descriptive in nature given the limited sample size in this pilot trial.
We will also examine additional covariates to ascertain which, if any, of these covariates are correlated with treatment response, we will carefully examine the data for indications of interactions between covariates and treatment effects. Cross validation for the selected covariates predictive of patient outcome will be performed. Such covariates will then be considered as potential exclusion criteria for future trials of therapy dosing during identified sensitive periods after stroke.
Analysis of the motor function measures and their correlation with clinical scales of UE motor function will allow us to select the particular parameters to be retained in future trials should a sensitive period be found. If the effects are large, a subsequent trial can be designed in a more economical fashion.
Data obtained from the skill and activity rankings will be analyzed using Spearman rank coefficients. Skill preference scores per category will be calculated by summing the total preference scores belonging to a specific skill category. Overall satisfaction scores will be computed for the selected skills following the standard scoring procedures established for the Motor Activity Log. These summed scores will be analyzed using appropriate parametric or non-parametric repeated measures analyses based on the distribution of the scores. The correlative analysis with multi-omic assessments is more descriptive given the limited sample size and is described further in the next section.
Peripheral Blood Draws for Multi-omic Assessments
The CPASS trial also presents an opportunity to determine whether the same molecular processes that occur in experimental animals post-stroke also take place in the human. The last decade has produced an explosion in the analytic methods used to assess various clinical specimens. Blood biomarker analysis in human stroke is not new, but most of the focus up to this point has remained in the hyperacute phase—finding biomarkers to diagnose acute stroke (Tang et al., 2006). Blood biomarker analysis is viable because many brain-derived molecules cross the blood-brain-barrier, including micro-RNA's, lipids, short peptide chains, and exosomes. Based on similar analyses from relevant conditions such as TBI (Diaz-Arrastia et al., 2014) and Alzheimer's Disease (Mapstone et al., 2014), we believe there is a reasonable expectation of useful molecular signals. Additionally, it seems possible that peripheral metabolism might also affect recovery.
In order to limit sample variability in the CPASS trial, whenever possible, blood draws will be performed on fully awake subjects, between 8 a.m. and 10 a.m., who are fasting at least since midnight, and have not yet taken their morning medications. Multi-omic analyses requires collection of blood into three lavender top (EDTA) tubes (7 ml) and three PAXgene tubes (2.5 ml), which are processed and stored for later analysis at study conclusion. To minimize costs and subject burden, we will limit blood draws to baseline, pre-treatment, and post-treatment assessments.
Multi-omics Analysis from Peripheral Blood Specimens
We plan to use a variety of methods in analyzing human blood specimens from subjects in the CPASS trial. In this article we will use the term multi-omics to represent what some call panomics or “pan-omics,” terms used to identify a group of molecular biological methods and technologies, including metabolomics, proteomics, transcriptomics, epigenomics, genomics, and exosomics. While each of these methods has the potential to provide important insights, integration of their combined analytic results may further elucidate the biological basis of human stroke recovery.
To our knowledge, there are no completed studies testing multi-omics approaches during the recovery phase of human stroke. We know of two studies currently underway: the START trial (Carey et al., 2013), which seeks to identify a gene profile for post-stroke depression; and BAPTISe (Nave et al., 2013), which looks for changes in specific lipids and proteins related to post-stroke cardiovascular training. Our approach to molecular analysis in CPASS differs in many ways. In CPASS, we seek to identify profiles of differentially expressed genes, lipids, and proteins in the baseline blood draw that will distinguish those with good recovery from those with poor recovery at 1 year. In addition, we will compare blood samples drawn immediately before and after the 20 h of additional OT in the subacute and chronic groups with the hope of identifying molecular changes associated with OT. Given the small number of subjects in each group (16), omics findings for therapy-related changes will largely be descriptive in nature. Similar to START, but unlike BAPTISe, we will take an untargeted approach to multi-omics. In other words, we will not identify particular genes, lipids, and proteins a priori, but rather will use advanced bioinformatic techniques to identify candidate biomarkers. Our past experience suggests this untargeted approach is an effective method to identify novel biomarkers in neurologic disease (Mapstone et al., 2014). Another important way in which our analysis differs is that it will include exosomics. Exosomes are small vesicles released by almost all cell types including neurons. Central nervous system (CNS) exosomes appear to exit to the periphery and comprise at least 10–20% of plasma exosomes (Fiandaca et al., 2014). We anticipate that the analysis of protein, lipid, and RNA cargos from the CNS-derived exosomes in plasma may allow us to differentiate biological changes taking place in the CNS from those occurring in the periphery during stroke recovery.
Discussion: Challenges of Translating an Animal Experimental Paradigm into a Human Clinical Trial
Laboratory experiments using rodents have many advantages that allow the most exacting study of the biology of mammalian brain recovery, and the most unequivocal demonstration of the impact of putative motor training interventions. At the same time, the application of the scientific findings uncovered in the laboratory is motivated in large part by improving brain injury recovery in humans. For the translation of rodent findings to human disease, demonstration that the findings in rodents generalize to humans is essential, and requires clinical trials. We have designed a human stroke motor recovery trial tightly linked to the methods used in rodent motor recovery experiments done in the laboratory. Our design decisions have been made with the goals of minimizing the real-world limitations of rehabilitation trials and maximizing the clinical and scientific utility of our efforts and those of our study participants. A single center trial allows for maximal control of experimental conditions, but that control cannot approach that of a rodent laboratory.
The CPASS trial lays the groundwork for determining whether critical periods truly exist following human stroke. This phase II trial will provide the necessary data to conceive a well-planned definitive multicenter trial. The CPASS trial will navigate the challenges of delivering study-based occupational therapy in three different settings: during inpatient rehabilitation, outpatient rehabilitation, and in the patient's home. By measuring the primary endpoint at 1 year, we ensure that any treatment related difference between groups is durable—a key feature if we are to prove the existence of a critical period. Elucidation of molecular markers for recovery might lead to lab tests that could identify sensitive periods, when persons with stroke can best use treatment resources to achieve their goals. This could also someday lead to drug development that could prolong or restore sensitive periods during stroke recovery, giving persons with stroke a better recovery and thus more opportunity to choose goals and more fully participate in society. We hope that the advances explored by CPASS will lead to new discoveries in the field and ultimately better outcomes for stroke patients.
Author Contributions
All authors participated in experimental design and preparation of the manuscript. DE, MG, JB, KB, and EC were primarily responsible for Sections Study Measures and Interventions. MT was primarily responsible for Sections Randomization and Statistical Analysis. Members of the Biomarker Laboratory and Biorepository at Georgetown University Medical Center (IT, RC, MO, RP, AC, MM, MF, and HF) were primarily responsible for Sections Peripheral Blood Draws for Multi-omics Analysis and Multi-omics Analysis from Peripheral Blood Specimens.
Conflict of Interest Statement
Drs. Federoff, Fiandaca, Cheema, and Mapstone are named as co-inventors on provisional patent applications that have been filed by Georgetown University and the University of Rochester related to specific biomarker technologies described in this article. The authors declare that the research was conducted in the absence of any commercial or financial relationships that could be construed as a potential conflict of interest.
Acknowledgments
This work is supported by the Center for Brain Plasticity and Recovery at Georgetown University and MedStar National Rehabilitation Hospital, the National Institute for Neurological Diseases and Stroke (1U10NS086513) and the National Institute for Deafness and Communicative Disorders (K18 DC014558).
References
Atkinson, A. C. (1982). Optimal biased coin designs for sequential clinical trials with prognostic factors. Biometrika 69, 61–67. doi: 10.1093/biomet/69.1.61
Bavelier, D., Levi, D. M., Li, R. W., Dan, Y., and Hensch, T. K. (2010). Removing brakes on adult brain plasticity: from molecular to behavioral interventions. J. Neurosci. 30, 14964–14971. doi: 10.1523/JNEUROSCI.4812-10.2010
PubMed Abstract | Full Text | CrossRef Full Text | Google Scholar
Bernhardt, J., Dewey, H., Thrift, A., Collier, J., and Donnan, G. (2008). A very early rehabilitation trial for stroke (AVERT): phase II safety and feasibility. Stroke 39, 390–396. doi: 10.1161/STROKEAHA.107.492363
PubMed Abstract | Full Text | CrossRef Full Text | Google Scholar
Biernaskie, J., Chernenko, G., and Corbett, D. (2004). Efficacy of rehabilitative experience declines with time after focal ischemic brain injury. J. Neurosci. 24, 1245–1254. doi: 10.1523/JNEUROSCI.3834-03.2004
PubMed Abstract | Full Text | CrossRef Full Text | Google Scholar
Bland, S. T., Schallert, T., Strong, R., Aronowski, J., Grotta, J. C., and Feeney, D. M. (2000). Early exclusive use of the affected forelimb after moderate transient focal ischemia in rats: functional and anatomic outcome. Stroke 31, 1144–1152. doi: 10.1161/01.STR.31.5.1144
PubMed Abstract | Full Text | CrossRef Full Text | Google Scholar
Brott, T., Adams, H. P. Jr., Olinger, C. P., Marler, J. R., Barsan, W. G., Biller, J., et al. (1989). Measurements of acute cerebral infarction: a clinical examination scale. Stroke 20, 864–870. doi: 10.1161/01.STR.20.7.864
PubMed Abstract | Full Text | CrossRef Full Text | Google Scholar
Carey, L. M., Crewther, S., Salvado, O., Linden, T., Connelly, A., Wilson, W., et al. (2013). STroke imAging pRevention and treatment (START): a longitudinal stroke cohort study: clinical trials protocol. Int. J. Stroke doi: 10.1111/ijs.12190. [Epub ahead of print].
PubMed Abstract | Full Text | CrossRef Full Text | Google Scholar
Carmichael, S. T. (2003). Gene expression changes after focal stroke, traumatic brain and spinal cord injuries. Curr. Opin. Neurol. 16, 699–704. doi: 10.1097/00019052-200312000-00009
PubMed Abstract | Full Text | CrossRef Full Text | Google Scholar
Carmichael, S. T. (2006). Cellular and molecular mechanisms of neural repair after stroke: making waves. Ann. Neurol. 59, 735–742. doi: 10.1002/ana.20845
PubMed Abstract | Full Text | CrossRef Full Text | Google Scholar
Carr. (1992). “Motor Assesment Scale,” in Measurements in Neurological Rehabilitation, ed D. T. Wade (New York, NY: Oxford University Press), 155–157.
Carter, A. R., Connor, L. T., and Dromerick, A. W. (2010). Rehabilitation after stroke: current state of the science. Curr. Neurol. Neurosci. Rep. 10, 158–166. doi: 10.1007/s11910-010-0091-9
PubMed Abstract | Full Text | CrossRef Full Text | Google Scholar
Charlson, M. E., Pompei, P., Ales, K. L., and MacKenzie, C. R. (1987). A new method of classifying prognostic comorbidity in longitudinal studies: development and validation. J. Chronic Dis. 40, 373–383. doi: 10.1016/0021-9681(87)90171-8
PubMed Abstract | Full Text | CrossRef Full Text | Google Scholar
Collen. (1992). “The Motricity Index,” in Measurements in Neurological Rehabilitation, ed D. T. Wade (New York, NY: Oxford University Press), 154–157.
Cramer, S. C. (2008). Repairing the human brain after stroke: I. Mechanisms of spontaneous recovery. Ann. Neurol. 63, 272–287. doi: 10.1002/ana.21393
PubMed Abstract | Full Text | CrossRef Full Text | Google Scholar
Cramer, S. C., and Chopp, M. (2000). Recovery recapitulates ontogeny. Trends Neurosci. 23, 265–271. doi: 10.1016/S0166-2236(00)01562-9
PubMed Abstract | Full Text | CrossRef Full Text | Google Scholar
Deweert, W., and Harrison, M. A. (1985). Measuring recovery of arm-hand function in stroke patients: a comparison of the Brunnstrom-Fugl-Meyer Test and the Action Research Arm Test. Physiother. Can. 37, 65–70. doi: 10.3138/ptc.37.2.065
Diaz-Arrastia, R., Wang, K. K., Papa, L., Sorani, M. D., Yue, J. K., Puccio, A. M., et al. (2014). Acute biomarkers of traumatic brain injury: relationship between plasma levels of ubiquitin C-terminal hydrolase-L1 and glial fibrillary acidic protein. J. Neurotrauma 31, 19–25. doi: 10.1089/neu.2013.3040
PubMed Abstract | Full Text | CrossRef Full Text | Google Scholar
Dromerick, A. W., Lang, C. E., Birkenmeier, R. L., Wagner, J. M., Miller, J. P., Videen, T. O., et al. (2009). Very early constraint-induced movement during stroke rehabilitation (VECTORS): a single-center RCT. Neurology 73, 195–201. doi: 10.1212/WNL.0b013e3181ab2b27
PubMed Abstract | Full Text | CrossRef Full Text | Google Scholar
Dromerick, A. W., Lum, P. S., and Hidler, J. (2006). Activity-based therapies. NeuroRx 3, 428–438. doi: 10.1016/j.nurx.2006.07.004
PubMed Abstract | Full Text | CrossRef Full Text | Google Scholar
Duncan, P. W., Sullivan, K. J., Behrman, A. L., Azen, S. P., Wu, S. S., Nadeau, S. E., et al. (2011). Body-weight-supported treadmill rehabilitation after stroke. N. Engl. J. Med. 364, 2026–2036. doi: 10.1056/NEJMoa1010790
PubMed Abstract | Full Text | CrossRef Full Text | Google Scholar
Duncan, P. W., Wallace, D., Lai, S. M., Johnson, D., Embretson, S., and Laster, L. J. (1999). The stroke impact scale version 2.0. Evaluation of reliability, validity, and sensitivity to change. Stroke 30, 2131–2140. doi: 10.1161/01.STR.30.10.2131
PubMed Abstract | Full Text | CrossRef Full Text | Google Scholar
Feigenson, J. S., McDowell, F. H., Meese, P., McCarthy, M. L., and Greenberg, S. D. (1977). Factors influencing outcome and length of stay in a stroke rehabilitation unit. Part 1. Analysis of 248 unscreened patients—medical and functional prognostic indicators. Stroke 8, 651–656. doi: 10.1161/01.STR.8.6.651
PubMed Abstract | Full Text | CrossRef Full Text | Google Scholar
Fiandaca, M. S., Kapogiannis, D., Mapstone, M., Boxer, A., Eitan, E., Schwartz, J. B., et al. (2014). Identification of preclinical Alzheimer's disease by a profile of pathogenic proteins in neurally derived blood exosomes: a case-control study. Alzheimers Dement. doi: 10.1016/j.jalz.2014.06.008. [Epub ahead of print].
PubMed Abstract | Full Text | CrossRef Full Text | Google Scholar
Fugl-Meyer, A. R., Jaasko, L., Leyman, I., Olsson, S., and Steglind, S. (1975). The post-stroke hemiplegic patient. 1. a method for evaluation of physical performance. Scand. J. Rehabil. Med. 7, 13–31.
Gagnon, D., Nadeau, S., and Tam, V. (2006). Ideal timing to transfer from an acute care hospital to an interdisciplinary inpatient rehabilitation program following a stroke: an exploratory study. BMC Health Serv. Res. 6:151. doi: 10.1186/1472-6963-6-151
PubMed Abstract | Full Text | CrossRef Full Text | Google Scholar
Granger, C. V., Hamilton, B. B., Linacre, J. M., Heinemann, A. W., and Wright, B. D. (1993). Performance profiles of the functional independence measure. Am. J. Phys. Med. Rehabil. 72, 84–89. doi: 10.1097/00002060-199304000-00005
PubMed Abstract | Full Text | CrossRef Full Text | Google Scholar
Han, C., Wang, Q., Meng, P. P., and Qi, M. Z. (2013). Effects of intensity of arm training on hemiplegic upper extremity motor recovery in stroke patients: a randomized controlled trial. Clin. Rehabil. 27, 75–81. doi: 10.1177/0269215512447223
PubMed Abstract | Full Text | CrossRef Full Text | Google Scholar
Hensch, T. K. (2005). Critical period mechanisms in developing visual cortex. Curr. Top. Dev. Biol. 69, 215–237. doi: 10.1016/S0070-2153(05)69008-4
PubMed Abstract | Full Text | CrossRef Full Text | Google Scholar
Hess, E. H. (1973). Imprinting: Early experience and the Developmental Psychobiology of Attachment. New York, NY: Van Nostrand Reinhold.
Hsieh, C. L., Hsueh, I. P., Chiang, F. M., and Lin, P. H. (1998). Inter-rater reliability and validity of the action research arm test in stroke patients. Age Ageing 27, 107–113. doi: 10.1093/ageing/27.2.107
PubMed Abstract | Full Text | CrossRef Full Text | Google Scholar
Hubbard, I. J., Carey, L. M., Budd, T. W., Levi, C., McElduff, P., Hudson, S., et al. (2014). A randomized controlled trial of the effect of early upper-limb training on stroke recovery and brain activation. Neurorehabil. Neural Repair. doi: 10.1177/1545968314562647. [Epub ahead of print].
PubMed Abstract | Full Text | CrossRef Full Text | Google Scholar
Hubel, D. H., and Wiesel, T. N. (1970). The period of susceptibility to the physiological effects of unilateral eye closure in kittens. J. Physiol. 206, 419–436. doi: 10.1113/jphysiol.1970.sp009022
PubMed Abstract | Full Text | CrossRef Full Text | Google Scholar
Humm, J. L., Kozlowski, D. A., James, D. C., Gotts, J. E., and Schallert, T. (1998). Use-dependent exacerbation of brain damage occurs during an early post-lesion vulnerable period. Brain Res. 783, 286–292. doi: 10.1016/S0006-8993(97)01356-5
PubMed Abstract | Full Text | CrossRef Full Text | Google Scholar
Ingvaldsen, R. P. (1997). Modern views on motor skill learning are not “representative”. Hum. Mov. Sci. 16, 705–732. doi: 10.1016/S0167-9457(97)00022-5
Johnson, J. S., and Newport, E. L. (1989). Critical period effects in second language learning: the influence of maturational state on the acquisition of English as a second language. Cogn. Psychol. 21, 60–99. doi: 10.1016/0010-0285(89)90003-0
PubMed Abstract | Full Text | CrossRef Full Text | Google Scholar
Jorgensen, H. S., Nakayama, H., Raaschou, H. O., Vive-Larsen, J., Stoier, M., and Olsen, T. S. (1995a). Outcome and time course of recovery in stroke. Part I: Outcome. The Copenhagen Stroke Study. Arch. Phys. Med. Rehabil. 76, 399–405. doi: 10.1016/S0003-9993(95)80567-2
PubMed Abstract | Full Text | CrossRef Full Text | Google Scholar
Jorgensen, H. S., Nakayama, H., Raaschou, H. O., Vive-Larsen, J., Stoier, M., and Olsen, T. S. (1995b). Outcome and time course of recovery in stroke. Part II: time course of recovery. The copenhagen stroke study. Arch. Phys. Med. Rehabil. 76, 406–412. doi: 10.1016/S0003-9993(95)80568-0
PubMed Abstract | Full Text | CrossRef Full Text | Google Scholar
Katzman, R., Brown, T., Fuld, P., Peck, A., Schechter, R., and Schimmel, H. (1983). Validation of a short orientation-memory-concentration test of cognitive impairment. Am. J. Psychiatry 140, 734–739. doi: 10.1176/ajp.140.6.734
PubMed Abstract | Full Text | CrossRef Full Text | Google Scholar
Kleim, J. A., and Jones, T. A. (2008). Principles of experience-dependent neural plasticity: implications for rehabilitation after brain damage. J. Speech Lang. Hear. Res. 51, S225–S239. doi: 10.1044/1092-4388(2008/018)
PubMed Abstract | Full Text | CrossRef Full Text | Google Scholar
Kollen, B. J., Lennon, S., Lyons, B., Wheatley-Smith, L., Scheper, M., Buurke, J. H., et al. (2009). The effectiveness of the Bobath concept in stroke rehabilitation: what is the evidence? Stroke 40, e89–e97. doi: 10.1161/STROKEAHA.108.533828
PubMed Abstract | Full Text | CrossRef Full Text | Google Scholar
Kotila, M., Waltimo, O., Niemi, M. L., Laaksonen, R., and Lempinen, M. (1984). The profile of recovery from stroke and factors influencing outcome. Stroke 15, 1039–1044. doi: 10.1161/01.STR.15.6.1039
PubMed Abstract | Full Text | CrossRef Full Text | Google Scholar
Kozlowski, D. A., James, D. C., and Schallert, T. (1996). Use-dependent exaggeration of neuronal injury after unilateral sensorimotor cortex lesions. J. Neurosci. 16, 4776–4786.
Lang, C. E., Macdonald, J. R., Reisman, D. S., Boyd, L., Jacobson Kimberley, T., Schindler-Ivens, S. M., et al. (2009). Observation of amounts of movement practice provided during stroke rehabilitation. Arch. Phys. Med. Rehabil. 90, 1692–1698. doi: 10.1016/j.apmr.2009.04.005
PubMed Abstract | Full Text | CrossRef Full Text | Google Scholar
Lang, C. E., Wagner, J. M., Dromerick, A. W., and Edwards, D. F. (2006). Measurement of upper-extremity function early after stroke: properties of the action research arm test. Arch. Phys. Med. Rehabil. 87, 1605–1610. doi: 10.1016/j.apmr.2006.09.003
PubMed Abstract | Full Text | CrossRef Full Text | Google Scholar
Lawson, R., and Watson, L. (1963). Learning in the rat (Rattus Norvegicus) under positive vs. negative reinforcement with incentive conditions controlled. Ohio J. Sci. 63, 87–91.
Levin, M. F., Kleim, J. A., and Wolf, S. L. (2009). What do motor “recovery” and “compensation” mean in patients following stroke? Neurorehabil. Neural Repair 23, 313–319. doi: 10.1177/1545968308328727
PubMed Abstract | Full Text | CrossRef Full Text | Google Scholar
Lin, J. H., Hsu, M. J., Sheu, C. F., Wu, T. S., Lin, R. T., Chen, C. H., et al. (2009). Psychometric comparisons of 4 measures for assessing upper-extremity function in people with stroke. Phys. Ther. 89, 840–850. doi: 10.2522/ptj.20080285
PubMed Abstract | Full Text | CrossRef Full Text | Google Scholar
Liu, N., Cadilhac, D. A., Andrew, N. E., Zeng, L., Li, Z., Li, J., et al. (2014). Randomized controlled trial of early rehabilitation after intracerebral hemorrhage stroke: difference in outcomes within 6 months of stroke. Stroke 45, 3502–3507. doi: 10.1161/STROKEAHA.114.005661
PubMed Abstract | Full Text | CrossRef Full Text | Google Scholar
Lum, P. S., Burgar, C. G., and Shor, P. C. (2004). Evidence for improved muscle activation patterns after retraining of reaching movements with the MIME robotic system in subjects with post-stroke hemiparesis. IEEE Trans. Neural Syst. Rehabil. Eng. 12, 186–194. doi: 10.1109/TNSRE.2004.827225
PubMed Abstract | Full Text | CrossRef Full Text | Google Scholar
Lum, P. S., Mulroy, S., Amdur, R. L., Requejo, P., Prilutsky, B. I., and Dromerick, A. W. (2009). Gains in upper extremity function after stroke via recovery or compensation: potential differential effects on amount of real-world limb use. Top. Stroke Rehabil. 16, 237–253. doi: 10.1310/tsr1604-237
Mapstone, M., Cheema, A. K., Fiandaca, M. S., Zhong, X., Mhyre, T. R., Macarthur, L. H., et al. (2014). Plasma phospholipids identify antecedent memory impairment in older adults. Nat. Med. 20, 415–418. doi: 10.1038/nm.3466
PubMed Abstract | Full Text | CrossRef Full Text | Google Scholar
Mathiowetz, V., Volland, G., Kashman, N., and Weber, K. (1992). “The nine-hole peg test,” in Measurement in Neurological Rehabilitation, ed D. T. Wade (New York, NY: Oxford University Press), 171.
Maulden, S. A., Gassaway, J., Horn, S. D., Smout, R. J., and Dejong, G. (2005). Timing of initiation of rehabilitation after stroke. Arch. Phys. Med. Rehabil. 86, S34–S40. doi: 10.1016/j.apmr.2005.08.119
PubMed Abstract | Full Text | CrossRef Full Text | Google Scholar
Mead, G. E., Lewis, S. C., Wardlaw, J. M., Dennis, M. S., and Warlow, C. P. (2000). How well does the Oxfordshire community stroke project classification predict the site and size of the infarct on brain imaging? J. Neurol. Neurosurg. Psychiatr. 68, 558–562. doi: 10.1136/jnnp.68.5.558
PubMed Abstract | Full Text | CrossRef Full Text | Google Scholar
Medical Research Council. (1976). Aids to Examination of the Peripheral Nervous System. Memorandum no. 45. London: Her Majesty's Stationary Office.
Michaelsen, S. M., Dannenbaum, R., and Levin, M. F. (2006). Task-specific training with trunk restraint on arm recovery in stroke: randomized control trial. Stroke 37, 186–192. doi: 10.1161/01.STR.0000196940.20446.c9
PubMed Abstract | Full Text | CrossRef Full Text | Google Scholar
Michaelsen, S. M., and Levin, M. F. (2004). Short-term effects of practice with trunk restraint on reaching movements in patients with chronic stroke: a controlled trial. Stroke 35, 1914–1919. doi: 10.1161/01.STR.0000132569.33572.75
PubMed Abstract | Full Text | CrossRef Full Text | Google Scholar
Murphy, T. H., and Corbett, D. (2009). Plasticity during stroke recovery: from synapse to behaviour. Nat. Rev. Neurosci. 10, 861–872. doi: 10.1038/nrn2735
PubMed Abstract | Full Text | CrossRef Full Text | Google Scholar
Nakayama, H., Jorgensen, H. S., Raaschou, H. O., and Olsen, T. S. (1994). Compensation in recovery of upper extremity function after stroke: the Copenhagen Stroke Study. Arch. Phys. Med. Rehabil. 75, 852–857. doi: 10.1016/0003-9993(94)90108-2
Nave, A. H., Krober, J. M., Brunecker, P., Fiebach, J. B., List, J., Grittner, U., et al. (2013). Biomarkers and perfusion—training-induced changes after stroke (BAPTISe): protocol of an observational study accompanying a randomized controlled trial. BMC Neurol. 13:197. doi: 10.1186/1471-2377-13-197
PubMed Abstract | Full Text | CrossRef Full Text | Google Scholar
Newport, E. L. (1990). Maturational constraints on language learning. Cogn. Sci. 14, 11–28. doi: 10.1207/s15516709cog1401_2
Oldfield, R. C. (1971). The assessment and analysis of handedness: the Edinburgh inventory. Neuropsychologia 9, 97–113. doi: 10.1016/0028-3932(71)90067-4
PubMed Abstract | Full Text | CrossRef Full Text | Google Scholar
Ostwald, S. K., Godwin, K. M., Cheong, H., and Cron, S. G. (2009). Predictors of resuming therapy within four weeks after discharge from inpatient rehabilitation. Top. Stroke Rehabil. 16, 80–91. doi: 10.1310/tsr1601-80
Page, S. J., Sisto, S. A., Levine, P., Johnston, M. V., and Hughes, M. (2001). Modified constraint induced therapy: a randomized feasibility and efficacy study. J. Rehabil. Res. Dev. 38, 583–590.
Paolucci, S., Antonucci, G., Grasso, M. G., Morelli, D., Troisi, E., Coiro, P., et al. (2000). Early versus delayed inpatient stroke rehabilitation: a matched comparison conducted in Italy. Arch. Phys. Med. Rehabil. 81, 695–700. doi: 10.1016/S0003-9993(00)90095-9
PubMed Abstract | Full Text | CrossRef Full Text | Google Scholar
Pollock, A., Baer, G., Pomeroy, V., and Langhorne, P. (2007). Physiotherapy treatment approaches for the recovery of postural control and lower limb function following stroke. Cochrane Database Syst. Rev. CD001920. doi: 10.1002/14651858.CD001920.pub2
Rossi, P. W., Forer, S., and Wiechers, D. (1997). Effective rehabilitation for patients with stroke: analysis of entry, functional gain, and discharge to community. J. Neurol. Rehabil. 11, 27–33.
Shah, S., Vanclay, F., and Cooper, B. (1989). Improving the sensitivity of the Barthel Index for stroke rehabilitation. J. Clin. Epidemiol. 42, 703–709. doi: 10.1016/0895-4356(89)90065-6
PubMed Abstract | Full Text | CrossRef Full Text | Google Scholar
Signorini, D. F., Leung, O., Simes, R. J., Beller, E., Gebski, V. J., and Callaghan, T. (1993). Dynamic balanced randomization for clinical trials. Stat. Med. 12, 2343–2350. doi: 10.1002/sim.4780122410
PubMed Abstract | Full Text | CrossRef Full Text | Google Scholar
Sivenius, J., Pyorala, K., Heinonen, O. P., Salonen, J. T., and Riekkinen, P. (1985). The significance of intensity of rehabilitation of stroke—a controlled trial. Stroke 16, 928–931. doi: 10.1161/01.STR.16.6.928
PubMed Abstract | Full Text | CrossRef Full Text | Google Scholar
Skinner, B. F. (1938). The Behavior of Organisms: An Experimental Analysis. New York, NY: Appleton-Century-Crofts.
Stark, S. L., Edwards, D. F., Hollingsworth, H., and Gray, D. B. (2005). Validation of the reintegration to normal living index in a population of community-dwelling people with mobility limitations. Arch. Phys. Med. Rehabil. 86, 344–345. doi: 10.1016/j.apmr.2004.03.020
PubMed Abstract | Full Text | CrossRef Full Text | Google Scholar
Sunderland, A., Tinson, D. J., Bradley, E. L., Fletcher, D., Langton Hewer, R., and Wade, D. T. (1992). Enhanced physical therapy improves recovery of arm function after stroke. A randomised controlled trial. J. Neurol. Neurosurg. Psychiatry 55, 530–535. doi: 10.1136/jnnp.55.7.530
PubMed Abstract | Full Text | CrossRef Full Text | Google Scholar
Tang, Y., Xu, H., Du, X., Lit, L., Walker, W., Lu, A., et al. (2006). Gene expression in blood changes rapidly in neutrophils and monocytes after ischemic stroke in humans: a microarray study. J. Cereb. Blood Flow Metab. 26, 1089–1102. doi: 10.1038/sj.jcbfm.9600264
PubMed Abstract | Full Text | CrossRef Full Text | Google Scholar
Taub, E., Crago, J. E., Burgio, L. D., Groomes, T. E., Cook, E. W. III, Deluca, S. C., et al. (1994). An operant approach to rehabilitation medicine: overcoming learned nonuse by shaping. J. Exp. Anal. Behav. 61, 281–293. doi: 10.1901/jeab.1994.61-281
PubMed Abstract | Full Text | CrossRef Full Text | Google Scholar
Taub, E., McCoullock, K., Uswatte, G., and Morris, D. (eds.). (2011). Motor Activity Log (MAL) Manual. Birmingham: U.T.R. Group.
Thorndike, E. L. (1898). Animal intelligence: an experimental study of the associative processes in animals. Psychol. Monogr. 2, 1–109. doi: 10.1037/h0092987
Uswatte, G., Taub, E., Morris, D., Light, K., and Thompson, P. A. (2006). The Motor Activity Log-28: assessing daily use of the hemiparetic arm after stroke. Neurology 67, 1189–1194. doi: 10.1212/01.wnl.0000238164.90657.c2
PubMed Abstract | Full Text | CrossRef Full Text | Google Scholar
Van der Lee, J. H., De Groot, V., Beckerman, H., Wagenaar, R. C., Lankhorst, G. J., and Bouter, L. M. (2001). The intra- and interrater reliability of the action research arm test: a practical test of upper extremity function in patients with stroke. Arch. Phys. Med. Rehabil. 82, 14–19. doi: 10.1053/apmr.2001.18668
PubMed Abstract | Full Text | CrossRef Full Text | Google Scholar
Van der Lee, J. H., Roorda, L. D., Beckerman, H., Lankhorst, G. J., and Bouter, L. M. (2002). Improving the Action Research Arm test: a unidimensional hierarchical scale. Clin. Rehabil. 16, 646–653. doi: 10.1191/0269215502cr534oa
PubMed Abstract | Full Text | CrossRef Full Text | Google Scholar
van Swieten, J. C., Koudstaal, P. J., Visser, M. C., Schouten, H. J., and van Gijn, J. (1988). Interobserver agreement for the assessment of handicap in stroke patients. Stroke 19, 604–607. doi: 10.1161/01.STR.19.5.604
PubMed Abstract | Full Text | CrossRef Full Text | Google Scholar
Wade, D. T., and Hewer, R. L. (1987). Functional abilities after stroke: measurement, natural history and prognosis. J. Neurol. Neurosurg. Psychiatr. 50, 177–182. doi: 10.1136/jnnp.50.2.177
PubMed Abstract | Full Text | CrossRef Full Text | Google Scholar
Ween, J. E., Alexander, M. P., D'Esposito, M., and Roberts, M. (1996). Factors predictive of stroke outcome in a rehabilitation setting. Neurology 47, 388–392. doi: 10.1212/WNL.47.2.388
PubMed Abstract | Full Text | CrossRef Full Text | Google Scholar
Whitall, J., McCombe Waller, S., Silver, K. H., and Macko, R. F. (2000). Repetitive bilateral arm training with rhythmic auditory cueing improves motor function in chronic hemiparetic stroke. Stroke 31, 2390–2395. doi: 10.1161/01.STR.31.10.2390
PubMed Abstract | Full Text | CrossRef Full Text | Google Scholar
Winstein, C. J., Wolf, S. L., Dromerick, A. W., Lane, C. J., Nelsen, M. A., Lewthwaite, R., et al. (2013). Interdisciplinary comprehensive arm rehabilitation evaluation (ICARE): a randomized controlled trial protocol. BMC Neurol. 13:5. doi: 10.1186/1471-2377-13-5
PubMed Abstract | Full Text | CrossRef Full Text | Google Scholar
Woldag, H., Stupka, K., and Hummelsheim, H. (2010). Repetitive training of complex hand and arm movements with shaping is beneficial for motor improvement in patients after stroke. J. Rehabil. Med. 42, 582–587. doi: 10.2340/16501977-0558
PubMed Abstract | Full Text | CrossRef Full Text | Google Scholar
Wolf, S. L., Thompson, P. A., Winstein, C. J., Miller, J. P., Blanton, S. R., Nichols-Larsen, D. S., et al. (2010). The EXCITE stroke trial: comparing early and delayed constraint-induced movement therapy. Stroke 41, 2309–2315. doi: 10.1161/STROKEAHA.110.588723
PubMed Abstract | Full Text | CrossRef Full Text | Google Scholar
Wolf, S. L., Winstein, C. J., Miller, J. P., Taub, E., Uswatte, G., Morris, D., et al. (2006). Effect of constraint-induced movement therapy on upper extremity function 3 to 9 months after stroke: the EXCITE randomized clinical trial. JAMA 296, 2095–2104. doi: 10.1001/jama.296.17.2095
PubMed Abstract | Full Text | CrossRef Full Text | Google Scholar
Wong, D. L., Hockenberg-Eaton, M., Wilson, D., Winklestein, M. L., and Schwarz, P. (2001). Wong's Essentials of Pediatric Nursing. St. Louis, MO: Mosby.
Wylie, C. M. (1970). The value of early rehabilitation in stroke. Geriatrics 25, 107–113. doi: 10.1097/00006199-197011000-00106
PubMed Abstract | Full Text | CrossRef Full Text | Google Scholar
Yuan, A., Dromerick, A., and Tan, M. T. (2014). Phase II multi-arm clinical trial designs and randomization with covariates via empirical weighting. J.Clin. Trials 4:181. doi: 10.4172/2167-0870.1000181
Zhou, X., Panizzutti, R., de Villers-Sidani, E., Madeira, C., and Merzenich, M. M. (2011). Natural restoration of critical period plasticity in the juvenile and adult primary auditory cortex. J. Neurosci. 31, 5625–5634. doi: 10.1523/JNEUROSCI.6470-10.2011
PubMed Abstract | Full Text | CrossRef Full Text | Google Scholar
Keywords: stroke rehabilitation, cerebrovascular disorders, critical period, motor recovery, multi-omics, adaptive randomization
Citation: Dromerick AW, Edwardson MA, Edwards DF, Giannetti ML, Barth J, Brady KP, Chan E, Tan MT, Tamboli I, Chia R, Orquiza M, Padilla RM, Cheema AK, Mapstone ME, Fiandaca MS, Federoff HJ and Newport EL (2015) Critical periods after stroke study: translating animal stroke recovery experiments into a clinical trial. Front. Hum. Neurosci. 9:231. doi: 10.3389/fnhum.2015.00231
Received: 30 January 2015; Accepted: 10 April 2015;
Published: 29 April 2015.
Edited by:
Tobias Loetscher, University of South Australia, AustraliaReviewed by:
Sophie Jacquin-Courtois, Hospices Civils de Lyon, FranceAnna-Sophia Wahl, University of Zurich, Switzerland
Copyright © 2015 Dromerick, Edwardson, Edwards, Giannetti, Barth, Brady, Chan, Tan, Tamboli, Chia, Orquiza, Padilla, Cheema, Mapstone, Fiandaca, Federoff and Newport. This is an open-access article distributed under the terms of the Creative Commons Attribution License (CC BY). The use, distribution or reproduction in other forums is permitted, provided the original author(s) or licensor are credited and that the original publication in this journal is cited, in accordance with accepted academic practice. No use, distribution or reproduction is permitted which does not comply with these terms.
*Correspondence: Alexander W. Dromerick, Department of Rehabilitation Medicine, Center for Brain Plasticity and Research, MedStar National Rehabilitation Hospital, 102 Irving St., NW Washington, DC 01801 USA,YWxleGFuZGVyLncuZHJvbWVyaWNrQGd1bmV0Lmdlb3JnZXRvd24uZWR1