- 1Department of Neurosciences, University of Calgary, Calgary, AB, Canada
- 2Alberta Children's Hospital Research Institute (ACHRI), Calgary, AB, Canada
- 3Hotchkiss Brain Institute (HBI), University of Calgary, Calgary, AB, Canada
- 4Department of Pediatrics, University of Calgary, Calgary, AB, Canada
- 5Department of Community Health Sciences, University of Calgary, Calgary, AB, Canada
Background and objectives: Developmental coordination disorder (DCD) is a neurodevelopmental motor disorder occurring in 5-6% of school-aged children. It is suggested that children with DCD show deficits in motor learning. Transcranial direct current stimulation (tDCS) enhances motor learning in adults and children but is unstudied in DCD. We aimed to investigate if tDCS, paired with motor skill training, facilitates motor learning in a pediatric sample with DCD.
Methods: Twenty-eight children with diagnosed DCD (22 males, mean age: 10.62 ± 1.44 years) were randomized and placed into a treatment or sham group. Anodal tDCS was applied (1 mA, 20 min) in conjunction with fine manual training over 5 consecutive days. Children's motor functioning was assessed with the Purdue Pegboard Test and Jebsen-Taylor Hand Function Test at baseline, post-intervention and 6 weeks following intervention. Group differences in rates of motor learning and skill transfer/retention were examined using linear mixed modeling and repeated measures ANOVAs, respectively.
Results: There were no serious adverse events or drop-outs and procedures were well-tolerated. Independent of group, all participants demonstrated improved motor scores over the 5 training days [F(69.280), p < 0.001, 95% CI (0.152, 0.376)], with no skill decay observed at retention. There was no interaction between intervention group and day [F(2.998), p = 0.086, 95% CI (−0.020, 0.297)].
Conclusion: Children with DCD demonstrate motor learning with long-term retention of acquired skill. Motor cortex tDCS did not enhance motor learning as seen in other populations. Before conclusions of tDCS efficacy can be drawn, additional carefully designed trials with reproducible results are required.
Clinical Trial Registration: ClinicalTrials.gov: NCT03453983
Introduction
Developmental coordination disorder (DCD) affects 5–6% of school-aged children and is characterized by early onset of motor impairment, manifesting as clumsy, slow and inaccurate performance of motor tasks (American Psychiatric Association, 2013). Reduced motor competency interferes with activities of daily living (American Psychiatric Association, 2013), posing a threat to the physical literacy and mental health of affected children (Castelli et al., 2014; Harrowell et al., 2018; Blank et al., 2019). In addition to deficits in motor execution, children with DCD may also display difficulties learning new motor skills and/or tasks (Bo and Lee, 2013; Gomez and Sirigu, 2015; Biotteau et al., 2016a).
Children with DCD are encouraged to practice tasks that they find difficult, in the hope that movement repetition will improve performance (Levac et al., 2009; Smits-Engelsman et al., 2013; Blank et al., 2019). Although successes have be reported with practice, improvements are often variable and more commonly observed with intensive practice (Smits-Engelsman et al., 2013; Jane et al., 2018). The need for high doses of repetition could be attributed to a slower rate of motor learning among children with DCD (Biotteau et al., 2016b; Jane et al., 2018). However, such training may be an anathema to most children, highlighting the need for more efficient therapies.
The use of transcranial direct current stimulation (tDCS), a form of non-invasive brain stimulation, in motor rehabilitation is rapidly expanding (Bikson et al., 2016; Palm et al., 2016). TDCS, through the application of a subthreshold electrical current (1–2 milliamps), alters neuronal excitability and spontaneity, facilitating the brain's endogenous mechanisms of neuroplasticity (Stagg and Nitsche, 2011; Kronberg et al., 2017). When paired with motor skill training, multi-session tDCS is shown to augment motor learning in adults (Reis et al., 2009), typically developing children (Ciechanski and Kirton, 2017; Cole et al., 2018) and children with motor impairment such as cerebral palsy (Finisguerra et al., 2019; Grohs et al., 2019; Saleem et al., 2019). Recent reviews highlight the growing body of research investigating the therapeutic potential of tDCS in children with neurodevelopmental disorders (Finisguerra et al., 2019; Grohs et al., 2019; Saleem et al., 2019); preliminary evidence has supported tDCS enhanced motor functioning in balance, gait, hand function, reaction time and in inhibitory control. Safety and tolerability of tDCS is well-established in adults (Bikson et al., 2016) and is growing in children (Zewdie et al., 2020).
Given support for the safety, feasibility and efficacy of tDCS in pediatric populations with motor impairment, tDCS may provide an avenue to modulate motor learning and strengthen the effects of current therapies in children with DCD. However, the application of tDCS in a pediatric population with DCD has yet to be examined. Here, we present results of the first randomized controlled trial (NCT03453983) investigating the effects of multi-session motor cortex tDCS on motor learning in children with DCD; the primary motor cortex (M1) is a logistical initial target given its direct role in movement production (Sanes and Donoghue, 2000) and evidence showing that plastic changes within M1 are associated with early phases of motor learning (Dayan and Cohen, 2011). Based on previous evidence, it was hypothesized that enhanced rates of motor learning would be observed in children with DCD when fine manual skill training was paired with tDCS.
Materials and Methods
Enrollment
This study was carried out between July 2018 and November 2019 at the Alberta Children's Hospital, Calgary, Canada. Participants were recruited through developmental and community pediatricians, psychologists, physical/occupational therapists and via social media. Written informed consent from participants' legal guardians and child assent were obtained at enrollment. The University of Calgary Conjoint Health Research Ethics Board approved this study (REB18-0183).
Eligibility
Inclusion criteria were: (1) age 8 to 12 years, (2) current diagnoses of DCD by a registered health care provider and (3) right-handed (i.e., hand used for writing). Children 8–12 years were recruited as DCD is commonly diagnosed in elementary school. Those with pre-term birth (<36 weeks' gestation) or any neuropsychiatric, neurological and/or chronic disorders were excluded. Children with a diagnosis of attention deficit/hyperactivity disorder (ADHD), learning disorder (LD), or generalized anxiety disorder (GAD) were included given the high co-occurrence with DCD (Dewey et al., 2002; Dewey, 2018).
Participants were screened to ensure they met clinical criteria for DCD outlined in the Diagnostic and Statistical Manual of Mental Disorders (DSM-5) (American Psychiatric Association, 2013). Children demonstrated motor deficits (criterion A) with Total Test scores below the 16th percentile on the Movement Assessment Battery for Children 2nd Edition (MABC-II) (Barnett et al., 2007). Motor deficits interfered with daily functioning (criterion B), began early in development (criterion C), and were not better explained by an intellectual disability, visual impairment or neurological condition (criterion D). Diagnostic criteria B and C were confirmed by a parent questionnaire developed by the investigators, which included questions about difficulties experienced in three domains, (1) motor (i.e., handwriting, riding a bike, self-care tasks, motor planning, learning new motor tasks, etc.), (2) social (play and social skills, physically tired, lack of energy, etc.), and (3) academic (reading, writing, math skills, etc.), as well as the age at which motor difficulties were first observed. Criterion D was confirmed by questions on the parent questionnaire regarding all prior and current diagnoses as well as visual impairments, and children obtaining a Full-Scale IQ score >79 on the Wechsler Abbreviated Scale of Intelligence 2nd Edition (WASI-II) (Wechsler, 2011).
Study Design
A randomized, double-blind, sham-controlled trial was conducted in accordance with CONSORT guidelines (Schulz et al., 2010), including pediatric-specific considerations (Saint-Raymond et al., 2010). After screening, children were randomly assigned without stratification to one of two parallel intervention groups: (1) active tDCS or (2) sham. A simple randomization procedure was used. Allocation (1:1) was concealed in sequentially numbered, opaque, sealed envelopes. Corresponding envelopes were opened by the investigator (MNG) immediately before intervention. Participants were blinded to their assigned group throughout the study and completed a post-intervention questionnaire that asked them to guess which intervention they received and why. Investigators were blinded at data analysis.
Sample Size
Previous evidence of tDCS enhanced motor learning in typically developing children and adolescents, using a similar protocol, reported a moderate-to-large effect size (Cohens d > 0.65) (Cole et al., 2018). To estimate the sample size required for the primary linear mixed model analysis in the current study, the smpsize_lmm command in RStudio was used with the above effect size and a two-sided type 1 error of 0.05. It was estimated that 16 participants per group would have 90% power to detect group differences in the primary outcome measure.
tDCS Intervention
The right M1 was localized using the 10–20 electroencephalography method (Steinmetz et al., 1989). A saline soaked 25cm2 sponge electrode was placed over the right M1 (active anode electrode), with a second identical electrode placed on the contralateral supraorbital region (reference cathode electrode), held in place by adjustable head straps consistent with established methods (Ciechanski and Kirton, 2017).
Electrodes were attached to a conventional 1x1 tDCS system (Soterix, NY). Current was ramped up to 1 mA over 30 seconds (s). After 120 s, the current was maintained for 20 min (active tDCS group) or ramped back down to 0 mA over 30 s (sham group). The initial ramp-up produces transient scalp sensations and has been established as a valid sham technique (Ambrus et al., 2012). Following each stimulation session, participants completed a safety, and tolerability questionnaire (Garvey et al., 2001), documenting symptoms (i.e., headaches, burning, itching, tingling, and nausea), their severity and duration, as well as tolerability compared to seven common childhood experiences.
Outcome Measures
The primary outcome was left hand Purdue Pegboard Test (PPT) performance, a validated assessment of fine motor coordination and hand dexterity (Tiffin and Asher, 1948). The PPT consists of four subtests: left hand [PPTL], right hand [PPTR], bimanual [PPTLR] peg placement, and bimanual assembly [PPTA]. The peg placement tasks involved placing as many pins as possible into a pegboard in 30 s. The assembly task involved building as many copies of a demonstration structure using pins, collars and washers in 60 s. Scores were the highest total number of placed pegs or assembled items. The PPTL (non-dominant left-hand performance) was used for motor skill training and as the primary outcome measure of motor learning, as it is a challenging fine manual task for children to learn without reaching a learning “ceiling” effect.
Motor skills may be acquired by two modes of learning: online and offline. Online learning refers to skill learning that occurs within a training period. Offline learning refers to skill learning that occurs after the training session has ended and is often referred to as consolidation. TDCS may differentially affect online and offline learning (Reis et al., 2009). In the current study, online effects (within-day training) were determined by comparing baseline to final PPTL scores each day. Offline effects (between-day consolidation) were quantified by comparing baseline PPTL scores each day to final PPTL scores from the previous day. Daily effects were summed to obtain total online and offline effects.
Secondary outcomes included PPTR, PPTLR, and PPTA performance, to examine intervention effects on the untrained hand and bimanual skills, as well as Jebsen-Taylor Test of Hand Function (JTT) performance (Jebsen, 1969), an upper extremity test of unimanual motor skills. The JTT included five subtests: card turning, picking up/placing small objects, stacking checkers, moving light objects, and moving heavy objects. Left and right hands were tested independently. An overall score for each hand was obtained by summing the completion times for each subtest [JTTR, JTTL].
Motor Training
A schematic of the study protocol is shown in Figure 1. On day 1, baseline motor tests (PPT, JTT) were administered, followed by tDCS intervention (active or sham). During the intervention, three PPTL trials were performed at 5, 10, and 15 min as well as after intervention. Participants repeated this protocol for 4 consecutive days (days 2–5). Following training on day 5, all motor tests were repeated. Participants returned 6 ± 1 weeks later to repeat all motor tests. Assessments were video recorded and blindly scored offline.
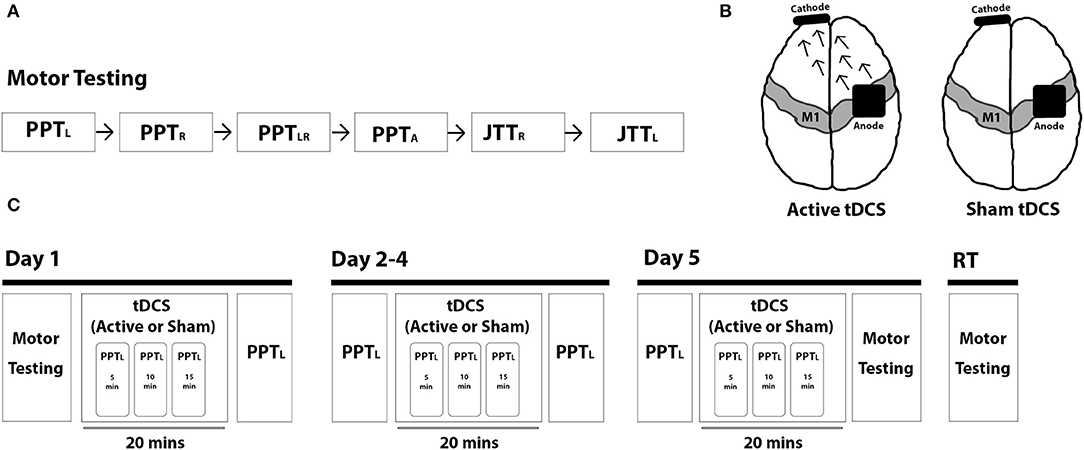
Figure 1. Trial protocol. (A) Motor skill testing included the Purdue Pegboard Test (PPT) (left-handed: PPTL, right-handed: PPTR, bimanual: PPTLR, assembly: PPTA) and the Jebsen-Taylor Test of Hand Function (JTT) (right-handed: JTTR, left-handed: JJTL). (B) Intervention groups included 1 mA anodal tDCS (left; arrows represent the direction of current flow from anode to cathode) and sham tDCS (right). (C) Study protocol is shown broken down by each intervention day (day 1–5) and for retention testing (RT) at 6-weeks post-intervention.
Analysis
Statistical analysis was performed in RStudio (RStudio Team, V1.3.1093) (R Core Team, 2017) and SPSS (IBM SPSS Software, V25) (SPSS Inc., 2017). Shapiro-Wilk tests assessed normality of each measure. As appropriate, independent samples t-tests, Mann-Whitney U-tests or chi-square tests compared participant characteristics, clinical and motor scores at baseline and tolerability ratings between intervention groups. The primary analysis was intention-to-treat and involved all participants.
Our statistical approach was based on previously established methods (Cole et al., 2018). A linear mixed effects model was chosen for the primary analysis as this approach offers advantages for longitudinal data sets with more data points and non-linear outcomes (Gibbons et al., 2010); our primary outcome parameter (change in PPTL score) was measured at six timepoints and previous findings from studies using similar protocols (Ciechanski and Kirton, 2017; Cole et al., 2018) showed non-linear changes in PPTL performance over multiple training days. The linear mixed effects model examined changes in the primary outcome (PPTL) between groups from pre- to post-intervention with fixed effects for Group, Day, the interaction of Group and Day, and random effects for participants including the intercept to account for repeated measures.
As secondary motor outcomes were measured at fewer timepoints and motor learning curves were not being generated, two-way repeated measures ANOVAs were used to investigate changes in secondary outcomes between intervention groups. Independent samples t-tests examined between group differences in performance at each time point. Paired samples t-tests examined within group differences in PPT (PPTL, PPTR, PPTLR, and PPTA) and JTT (JTTL, JTTR) performance between timepoints (i.e., baseline to post-intervention, baseline to retention) and potential skill decay between the final training block (day 5) and retention testing. Online and offline learning effects were explored within and between groups using paired and independent samples t-tests.
Results
Population
Twenty-eight children with DCD [10.62 ± 1.44 years; 22 (79%) male] were randomized (14 active, 14 sham). All participants completed baseline motor skill testing, the 5 consecutive intervention days and post-intervention motor skill testing. Six participants (3 active, 3 sham) did not complete retention motor skill testing due to travel or family factors (Figure 2, CONSORT recruitment flow diagram). Group demographics, clinical scores and baseline motor scores are shown in Table 1. No group differences were observed for age [t(26) = 0.637, p = 0.530], sex [x2(1) = 0.848, p = 0.357] or clinical scores (MABC-II: U = 79, p = 0.374; WASI-II: t(26) = −0.586, p = 0.563). No group differences in baseline motor scores were observed (all p > 0.7). Fifteen participants had ADHD [n = 6 (43%) active, n = 9 (64%) sham], 11 had a LD [n = 6 (43%) active, n = 5 (36%) sham], and 5 had GAD [n = 3 (21%) active, n = 2 (14%) sham] (Table 2). Proportions did not differ between groups (all p > 0.2). Thirteen of the 28 participants were taking medications for ADHD (i.e., Vyvanse, Biphentin, and Clonidine) and/or anxiety (i.e., Prozac, Zoloft, and Citalopram) (Table 2). Proportions did not differ between groups (p > 0.7).
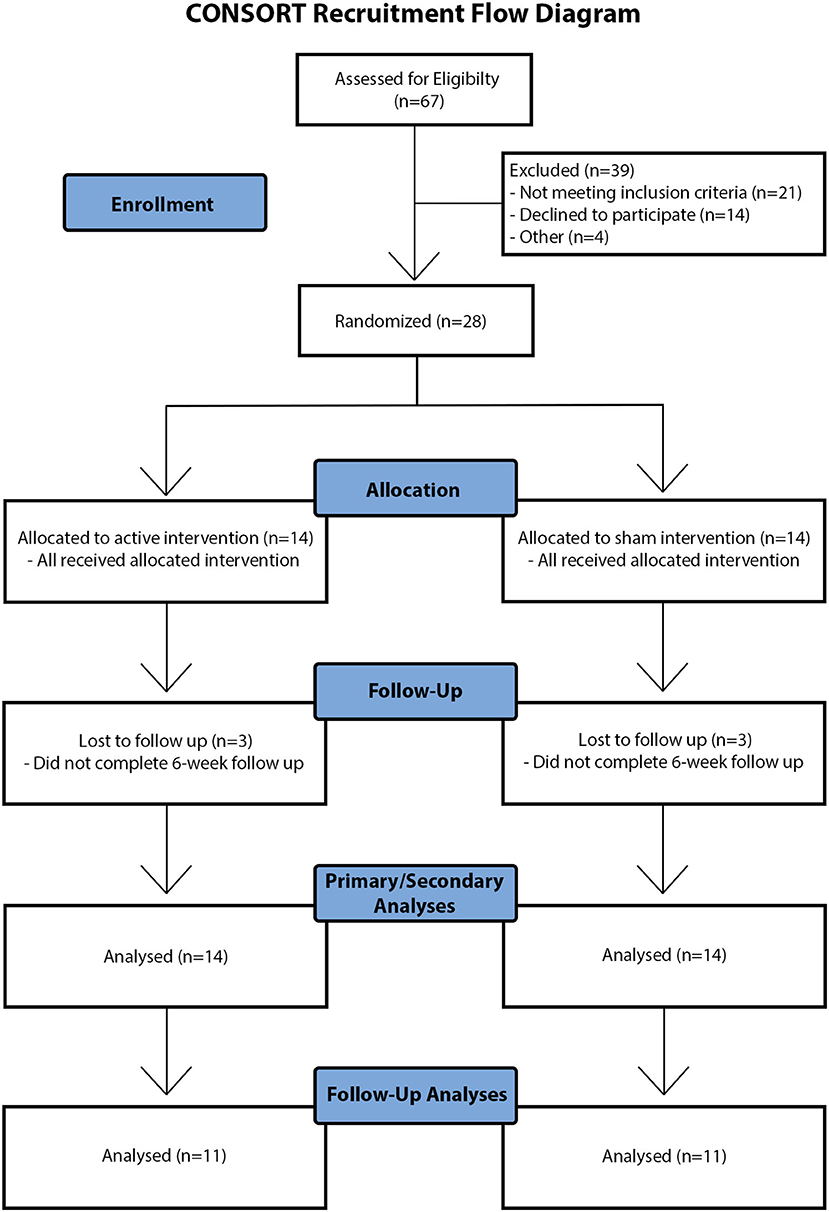
Figure 2. CONSORT recruitment flow diagram. Visual schematic of participant recruitment, screening, data collection, and analysis. Note that “other reasons” for exclusion of children at eligibility screening and follow-up included travel and family factors.
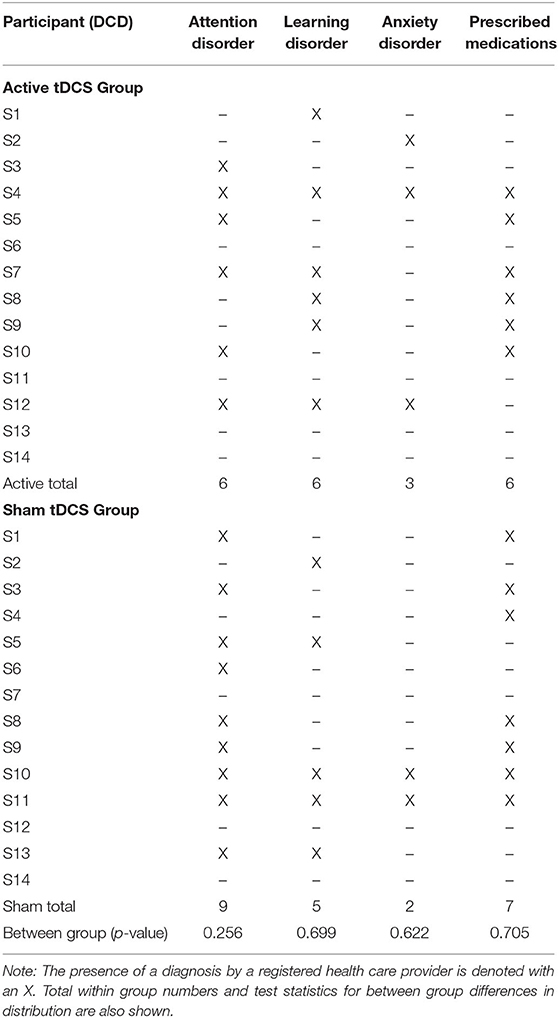
Table 2. Distribution of co-occurring attention, learning and anxiety disorders by participant and group.
Motor Learning
PPTL learning curves by group are shown in Figure 3. Curves were generated by plotting mean change in score from baseline to each training point. Linear mixed effects modeling showed that, independent of intervention, all participants demonstrated motor learning over 5 training days [p < 0.001, 95% CI (0.25–0.41), Table 3]. No interaction effect of Day and Group on rate of motor learning was seen; therefore, the interaction term was removed from the final mixed model [PPTL ~ group + day + (1|subjects)]. Average PPTL performance was higher on post-intervention day 5 compared to baseline in both groups [active: t(13) = −5.824, p < 0.001, Cohen's d = 1.557; sham: t(13) = −2.820, p = 0.014, Cohen's d = 0.754]. No group differences were observed in average PPTL performance at any time point (all p > 0.1).
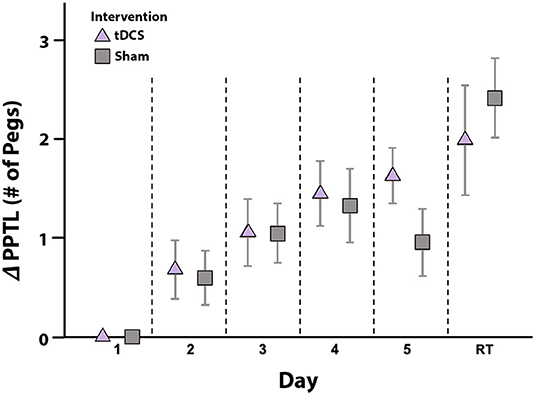
Figure 3. Motor learning by intervention group. Mean daily change in PPTL scores from baseline (y-axis) are shown for the active (triangle) and sham (squares) intervention groups. Error bars represent standard error. In both groups, scores improved from baseline to post-intervention testing on day 5, with no skill decay at retention testing (RT) 6-weeks post-intervention. No between group differences in PPTL scores were noted at any timepoint. ΔPPTL: change in left-hand Purdue Pegboard Test scores from baseline.
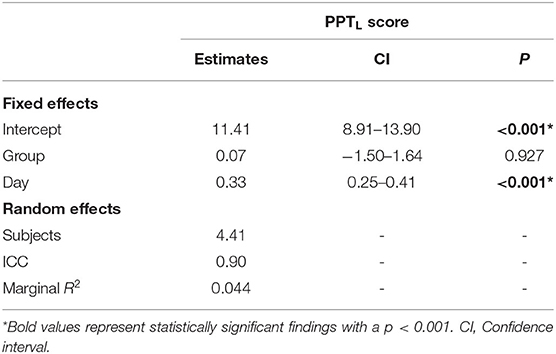
Table 3. Results of linear mixed effects model examining motor learning over 5 days of skill training.
Retention
Learning effects were retained in both groups, with no skill decay in PPTL scores between post-intervention day 5 and retention testing at 6-weeks (Figure 3). In the active group, PPTL scores at retention did not differ from post-intervention day 5 [t(10) = −1.966, p = 0.078, Cohen's d = 0.593]. Within the sham group, higher PPTL scores were observed at retention compared to post-intervention day 5 [t(10) = −4.989, p = 0.001, Cohen's d = 1.504]. This difference may relate to lower scores in the sham group on day 5 (see above). In both groups, PPTL scores at retention were higher than baseline [active: t(10) = −3.585, p = 0.005, Cohen's d = 1.080; sham: t(10) = −6.037, p < 0.001, Cohen's d = 1.820], with no group differences [t(20) = −1.025, p = 0.321].
Online and Offline Learning Effects
There was more online learning compared to offline learning in both the active [t(13) = 2.545, p = 0.024] and sham [t(13) = 5.488, p < 0.001] groups (Figure 4). No group differences in online [t(26) = −0.669, p = 0.509] or offline [t(26) = 0.866, p = 0.395] learning were observed.
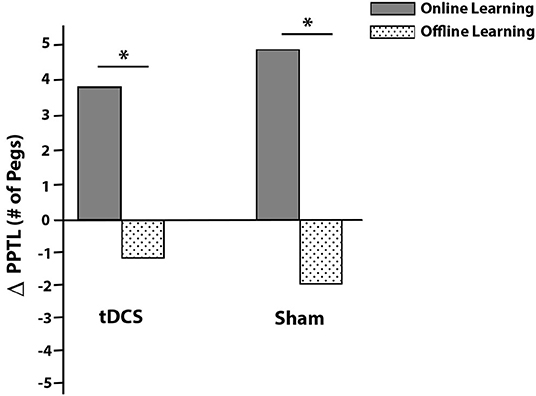
Figure 4. Average PPTL online (solid gray) and offline (dotted) learning effects by intervention group. Online effects are within session improvements while offline effects are improvements that occur between sessions (consolidation). Daily online and offline effects were summed to obtain total online and offline changes in scores (y-axis ΔPPTL). *p < 0.05.
Secondary Motor Outcomes
Effects of intervention on secondary untrained PPT and JTT measures are shown in Figure 5. Learning effects were observed for PPTR (F = 32.346, p < 0.001, partial eta2 = 0.554), PPTLR (F = 32.795, p < 0.001, partial eta2 = 0.558) and PPTA (F = 28.041, p < 0.001, partial eta2 = 0.519). These were independent of group, with no interaction effects of Time and Group (all p > 0.2). In both groups, compared to baseline, PPTR scores were higher on post-intervention day 5 [active: t(13) = −4.535, p = 0.001, Cohen's d = 1.212; sham: t(13) = −3.863, p = 0.002, Cohen's d = 1.032] and at retention [active: t(10) = −6.297, p < 0.001, Cohen's d = 1.899; sham: t(10) = −4.856, p = 0.001, Cohen's d = 1.464]. PPTLR scores were higher by day 5 [active: t(13) = 4.436, p = 0.001, Cohen's d = 1.186; sham: t(13) = 3.721, p = 0.003, Cohen's d = 0.994] and at retention [active: t(10) = −4.730, p = 0.001, Cohen's d = 1.426; sham: t(10) = −4.351, p = 0.001, Cohen's d = 1.312] for both groups. PPTA scores demonstrated a similar pattern at day 5 [active: t(13) = −4.200, p = 0.001, Cohen's d = 1.122; sham: t(13) = −3.727, p = 0.003, Cohen's d = 0.996] and retention [active: t(10) = −4.139, p = 0.002, Cohen's d = 1.248; sham: t(10) = −4.967, p = 0.001, Cohen's d = 1.498]. Finally, no skill decay from post-intervention day 5 to retention testing was observed in either group for PPTR [active: t(10) = −2.015, p = 0.072, Cohen's d = 0.608; sham: t(10) = −0.586, p = 0.571, Cohen's d = 0.177], PPTLR [active: t(10) = 0.379, p = 0.712, Cohen's d = 0.114; sham: t(10) = −2.036, p = 0.069, Cohen's d = 0.614], or PPTA [active: t(10) = −1.919, p = 0.084, Cohen's d = 0.579; sham: t(10) = −0.576, p = 0.578, Cohen's d = 0.174].
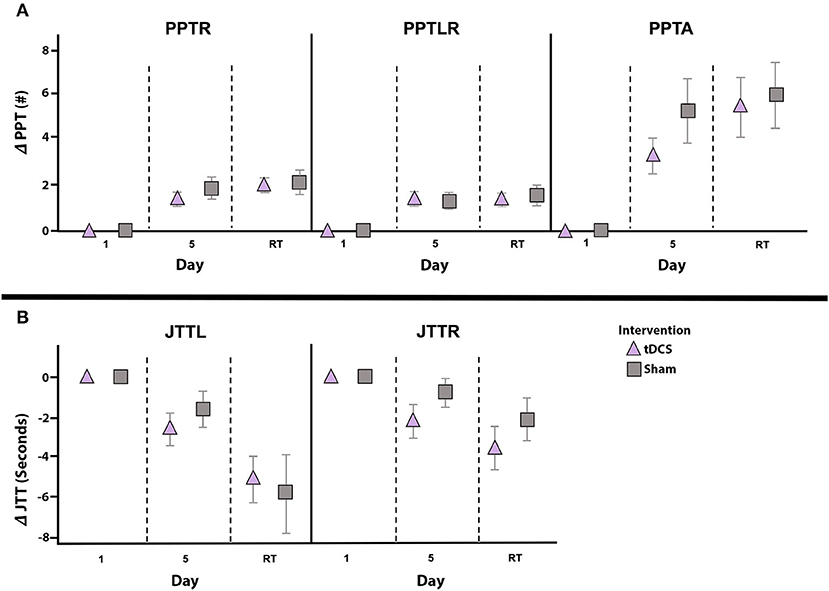
Figure 5. Change in secondary outcomes measures by intervention group. Mean daily score change from baseline (y-axes) for PPTR, PPTLR, and PPTA (A) as well as JTTL and JTTR (B), shown for the active (triangle) and sham (squares) groups. Error bars represent standard error. Independent of intervention group all scores significantly improved from baseline (day 1) to post-intervention testing on day 5, and from baseline to retention testing (RT) 6-weeks post-intervention. No between group differences in performance on any secondary measures were noted at any timepoint. Note that negative JTT scores indicate improved performance (i.e., reduction in completion time) and positive JTT scores indicate worse performance (i.e., increased time required to complete tasks). PPTL, Purdue Pegboard Test left-hand; PPTR, Purdue Pegboard Test right-hand; PPTLR, Purdue Pegboard Test bimanual; PPTA, Purdue Pegboard Test assembly; JTTL, Jebsen-Taylor Test left-hand; JTTR, Jebsen-Taylor Test right-hand.
Independent of intervention, learning effects were observed for JTTL (F = 11.476, p = 0.002, partial eta2 = 0.306) and JTTR (F = 7.887, p = 0.009, partial eta2 = 0.233). In the active group, JTTL and JTTR performance was faster on post-intervention day 5 [JTTL t(13) = 3.150, p = 0.008, Cohen's d = 0.842; JTTR t(13) = 2.700, p = 0.018, Cohen's d = 0.722] and at retention [JTTL t(10) = 4.397, p = 0.001, Cohen's d = 1.326; JTTR t(10) = 3.348, p = 0.007, Cohen's d = 1.009] compared to baseline. In the sham group, improved JTTL performance was not seen on day 5 [t(13) = 1.722, p = 0.109] but was present at retention testing [t(9) = 3.769, p = 0.004, Cohen's d = 1.136]. JTTR scores at day 5 [t(13) = 1.164, p = 0.265, Cohen's d = 0.311] and retention [t(9) = 2.033, p = 0.073, Cohen's d = 0.613] did not differ from baseline in the sham group. There was no evidence of skill decay from day 5 to retention testing on the JTTL [active: t(10) = 2.093, p = 0.063, Cohen's d = 0.631; sham: t(9) = 1.589, p = 0.147, Cohen's d = 0.479] or JTTR [active: t(10) = 1.521, p = 0.159, Cohen's d = 0.459; sham: t(9) = 0.301, p = 0.770, Cohen's d = 0.091].
Safety, Tolerability, and Blinding
In total, 140 tDCS sessions were performed with no serious adverse events and sessions were well-tolerated. Reported sensations included itching (89%; 44% mild, 48% moderate, 8% severe), tingling (68%; 79% mild, 5% moderate, 16% severe), and burning (54%; 73% mild, 27% moderate), which did not differ by group. Seven participants reported a mild headache lasting for the first few minutes of stimulation and five participants felt mildly nauseated in a single session. TDCS tolerability rankings, on an 8-point scale, were similar for the active (4.1 ± 1.1) and sham groups (4.1 ± 1.2; p = 0.974) and were comparable to watching TV (2.6 ± 0.9) or a long car ride (5.1 ± 1.3). Participants were unable to predict their treatment group (44% accuracy, 50% indicates chance).
Discussion
The current trial is the first to examine the therapeutic efficacy of tDCS on motor learning in children with DCD. Independent of intervention, all children's motor performance improved over the 5 training days and skill improvements were retained for 6 weeks. Contrary to our hypothesis, excitatory stimulation of the right primary motor cortex did not enhance motor learning.
The research literature suggests that poor motor performance in children with DCD may be associated with deficits in motor learning (Bo and Lee, 2013; Biotteau et al., 2016a). However, research concerning the presence of motor learning deficits in DCD is inconsistent, with some studies reporting limited skill improvement following practice (Kagerer et al., 2004; Gheysen et al., 2011; Zwicker et al., 2011) and others reporting positive effects of practice (Ferguson et al., 2013; Lejeune et al., 2013; Mombarg et al., 2013; Smits-Engelsman et al., 2015). Studies supporting the latter emphasize that children with DCD are able to acquire motor skills, though they may display slower rates of motor learning, requiring more intensive practice to reach desired levels of motor competence. In the current trial, fine motor performance of the non-dominant limb improved significantly with practice, independent of intervention. This finding supports the capacity of children with DCD to learn novel motor skills.
Motor learning involves both online and offline processes. Online learning includes skill gains obtained during active training, whereas offline learning includes gains occurring between training sessions (i.e., consolidation). Within both groups, the majority of motor learning took place online. This suggests that children with DCD may show less efficient offline motor learning, or consolidation, which has been previously suggested in the DCD literature (Zwicker et al., 2011) and warrants further study.
Motor skill retention and transfer to untrained tasks are also features of successful motor learning (Muratori et al., 2013). We show no evidence of skill decay in either group between the final training day and retention testing at 6-weeks. Moreover, motor skill improvements were not restricted to the trained hand or task as improvements on all secondary motor outcomes were observed. Learning effects were generalized to the untrained dominant hand. Taken together, these results suggest that children with DCD display intact motor skill acquisition, adaptation, retention, and transfer following practice.
Contrary to previous evidence of tDCS enhanced motor learning in typically developing children (Ciechanski and Kirton, 2017; Cole et al., 2018) and children with motor impairment (i.e., cerebral palsy) (Grohs et al., 2019), tDCS did not enhance the rate of motor learning in children with DCD relative to practice alone. The limited efficacy of tDCS could be reflective of stimulation parameters including cortical target (Thibaut et al., 2017) or montage (i.e., anode and cathode arrangement) (Woods and Martin, 2016). Although M1 is a common target to modulate motor learning due its direct role in motor production (Todorov, 2003), other structures may be better suited to the DCD population. For instance, dysfunction in cerebellar networks has commonly been identified in DCD (Biotteau et al., 2016a). Given the role of the cerebellum in motor control and learning (Manto et al., 2012), as well as positive findings from trials implementing cerebellar tDCS for motor impairment (Celnik, 2015), it may be a promising target in DCD.
Regarding montage, different anode/cathode placement uniquely modulates cortical excitability. Anodal tDCS involves placement of the anode over a target region and generally produces excitatory effects within the cortex, whereas in cathodal tDCS the cathode is placed over the target region producing an overall inhibitory effect. Although anodal stimulation was chosen here based on previous evidence (Cole et al., 2018), cathodal stimulation has also been shown to enhance motor learning in children (Ciechanski and Kirton, 2017). Neurophysiological research has reported reduced interhemispheric inhibition of M1 activity in DCD (He et al., 2018). It is, therefore, possible that inhibiting cortical activity via cathodal stimulation may produce favorable outcomes in children with DCD. Future studies that characterize baseline cortical excitability and neurometabolites, using techniques such as transcranial magnetic stimulation (TMS) and magnetic resonance spectroscopy (MRS), could help in refining application (i.e., stimulation intensity, montage, and target).
It is also possible that there are no effects of tDCS in children with DCD. However, given this is the first study to examine effects of neuromodulation in children with DCD, future studies using well-supported protocols that target different cortical regions and/or examine different montages are highly encouraged. Finally, given that tDCS enhances motor learning via facilitating endogenous neuroplastic mechanisms, the absence of response to tDCS observed here could also suggest disordered neuroplastic mechanisms in individuals with DCD. Future studies utilizing techniques such as TMS could help to elucidate plasticity mechanisms in DCD.
Limitations
Our sample size calculation estimated that 16 participants per group would provide us with 90% power to detect group differences; however, our final groups consisted of 14 participants. As a result, our sample size may have decreased our ability to detect potential group differences, or efficacy, and may have limited the generalizability of our findings. There was also a high degree of variability in performance on our outcome measures, which may have decreased our ability to detect group differences given the sample size. Thirteen participants were on medications that influence neurotransmitter systems and could have impacted tDCS efficacy (McLaren et al., 2018). Another limitation was the demanding nature of the trial, which required children to maintain their attention and motivation over 5 consecutive days. This may have been difficult, particularly for our sample with co-occurring attention, learning and anxiety disorders, and may have contributed to performance variability. Co-morbidities and the fact that children with DCD are a heterogeneous group who display many different types of motor skill deficits, constitutes a significant challenge for future trials.
Conclusion
Children with DCD demonstrated motor learning as measured by the PPT with retention of acquired skill at 6-weeks. The addition of motor cortex tDCS during training did not enhance motor learning, as seen in other populations. Procedures were well-tolerated and appear safe. Before conclusions can be made regarding the efficacy of tDCS in DCD, additional carefully designed trials with reproducible results are required. Establishment of an optimal tDCS protocol in DCD is essential, including stimulation target and montage.
Data Availability Statement
The raw data supporting the conclusions of this article will be made available by the authors, without undue reservation.
Ethics Statement
This study, involving human participants, was reviewed and approved by the University of Calgary Conjoint Health Research Ethics Board (Ethics ID: REB10-0183). Written informed consent to participate in this study was provided by the participants' legal guardian/next of kin. Written informed consent was obtained from the minor(s)' legal guardian/next of kin for the publication of any potentially identifiable images or data included in this article.
Author Contributions
MG, AK, and DD conceptualized and designed the study. MG collected, analyzed and interpreted the data, and took the lead in writing the manuscript. BC assisted with the data analysis. DD and AK secured grant funding for this study, provided, administrative, technical, material support, and supervised the project. BC, AK, and DD provided critical feedback and helped to shape the final manuscript. All authors contributed to the article and approved the submitted version.
Funding
This work was supported by a grant from the Robertson Fund for Cerebral Palsy Research (UCP01-10018297) to DD and AK, and a grant from the Alberta Children's Hospital Research Institute (ACHRI; UCP01-10024123) to DD. MG was funded through an Alberta Children's Hospital Research Institute (ACHRI) Graduate Studentship and a University of Calgary Department of Pediatrics Graduate Studentship. BC was supported through a Vanier Canada Graduate Scholarship and a Cumming School of Medicine Graduate Scholarship.
Conflict of Interest
The authors declare that the research was conducted in the absence of any commercial or financial relationships that could be construed as a potential conflict of interest.
Acknowledgments
We would like to acknowledge all the families who took part in this research study, as well as Lauran Cole and Dr. Helen Carlson for statistical guidance. We thank Dr. Ephrem Zewdie and the Non-invasive Neurostimulation Network (N3) for providing the training and equipment essential to this study.
References
Ambrus, G. G., Al-Moyed, H., Chaieb, L., Sharp, L., Antal, A., and Paulus, W. (2012). The fade-in–short stimulation–fade out approach to sham tDCS–reliable at 1 mA for naive and experienced subjects, but not investigators. Brain Stimul. 5, 499–504. doi: 10.1016/j.brs.2011.12.001
American Psychiatric Association (2013). Diagnostic and Statistical Manual of Mental Disorders (DSM-5®). Arlington, VA.
Barnett, A., Henderson, S., and Sugden, D. (2007). Movement Assessment Battery for Children. San Antonio, TX: Pearson Education Limited. doi: 10.1037/t55281-000
Bikson, M., Grossman, P., Thomas, C., Zannou, A. L., Jiang, J., Adnan, T., et al. (2016). Safety of transcranial direct current stimulation: evidence based update 2016. Brain Stimul. 9, 641–661. doi: 10.1016/j.brs.2016.06.004
Biotteau, M., Chaix, Y., and Albaret, J. M. (2016a). What do we really know about motor learning in children with developmental coordination disorder? Curr. Dev. Disord. Rep. 3, 152–160. doi: 10.1007/s40474-016-0084-8
Biotteau, M., Chaix, Y., Blais, M., Tallet, J., Peran, P., and Albaret, J. M. (2016b). Neural signature of DCD: a critical review of MRI neuroimaging studies. Front. Neurol. 7:227. doi: 10.3389/fneur.2016.00227
Blank, R., Barnett, A. L., Cairney, J., Green, D., Kirby, A., Polatajko, H., et al. (2019). International clinical practice recommendations on the definition, diagnosis, assessment, intervention, and psychosocial aspects of developmental coordination disorder. Dev. Med. Child Neurol. 61, 242–285. doi: 10.1111/dmcn.14132
Bo, J., and Lee, C. M. (2013). Motor skill learning in children with developmental coordination disorder. Res. Dev. Disabil. 34, 2047–2055. doi: 10.1016/j.ridd.2013.03.012
Castelli, D. M., Centeio, E. E., Beighle, A. E., Carson, R. L., and Nicksic, H. M. (2014). Physical literacy and comprehensive school physical activity programs. Prev. Med. 66, 95–100. doi: 10.1016/j.ypmed.2014.06.007
Celnik, P. (2015). Understanding and modulating motor learning with cerebellar stimulation. Cerebellum 14, 171–174. doi: 10.1007/s12311-014-0607-y
Ciechanski, P., and Kirton, A. (2017). Transcranial direct-current stimulation can enhance motor learning in children. Cereb. Cortex 27, 2758–2767. doi: 10.1093/cercor/bhw114
Cole, L., Giuffre, A., Ciechanski, P., Carlson, H. L., Zewdie, E., Kuo, H. C., et al. (2018). Effects of high-definition and conventional transcranial direct-current stimulation on motor learning in children. Front. Neurosci. 12:787. doi: 10.3389/fnins.2018.00787
Dayan, E., and Cohen, L. G. (2011). Neuroplasticity subserving motor skill learning. Neuron 72, 443–454. doi: 10.1016/j.neuron.2011.10.008
Dewey, D. (2018). What is comorbidity and why does it matter in neurodevelopmental disorders? Curr. Dev. Disord. Rep. 5, 235–242. doi: 10.1007/s40474-018-0152-3
Dewey, D., Kaplan, B. J., Crawford, S. G., and Wilson, B. N. (2002). Developmental coordination disorder: associated problems in attention, learning, and psychosocial adjustment. Hum. Mov. Sci. 21, 905–918. doi: 10.1016/S0167-9457(02)00163-X
Ferguson, G., Jelsma, D., Jelsma, J., and Smits-Engelsman, B. (2013). The efficacy of two task-orientated interventions for children with developmental coordination disorder: neuromotor task training and nintendo wii fit training. Res. Dev. Disabil. 34, 2449–2461. doi: 10.1016/j.ridd.2013.05.007
Finisguerra, A., Borgatti, R., and Urgesi, C. (2019). Non-invasive brain stimulation for the rehabilitation of children and adolescents with neurodevelopmental disorders: a systematic review. Front. Psychol. 10:135. doi: 10.3389/fpsyg.2019.00135
Garvey, M. A., Kaczynski, K. J., Becker, D. A., and Bartko, J. J. (2001). Subjective reactions of children to single-pulse transcranial magnetic stimulation. J. Child Neurol. 16, 891–894. doi: 10.1177/088307380101601205
Gheysen, F., Van Waelvelde, H., and Fias, W. (2011). Impaired visuo-motor sequence learning in developmental coordination disorder. Res. Dev. Disabil. 32, 749–756. doi: 10.1016/j.ridd.2010.11.005
Gibbons, R. D., Hedeker, D., and DuToit, S. (2010). Advances in analysis of longitudinal data. Annu. Rev. Clin. Psychol. 6, 79–107. doi: 10.1146/annurev.clinpsy.032408.153550
Gomez, A., and Sirigu, A. (2015). Developmental coordination disorder: core sensori-motor deficits, neurobiology and etiology. Neuropsychologia 79, 272–87. doi: 10.1016/j.neuropsychologia.2015.09.032
Grohs, M. N., Hilderley, A., and Kirton, A. (2019). The therapeutic potential of non-invasive neurostimulation for motor skill learning in children with neurodevelopmental disorders. Curr. Dev. Disord. Rep. 6, 19–28. doi: 10.1007/s40474-019-0155-8
Harrowell, I., Hollen, L., Lingam, R., and Emond, A. (2018). The impact of developmental coordination disorder on educational achievement in secondary school. Res. Dev. Disabil. 72, 13–22. doi: 10.1016/j.ridd.2017.10.014
He, J. L., Fuelscher, I., Enticott, P. G., Teo, W. P., Barhoun, P., and Hyde, C. (2018). Interhemispheric cortical inhibition is reduced in young adults with developmental coordination disorder. Front. Neurol. 9:179. doi: 10.3389/fneur.2018.00179
Jane, J. Y., Burnett, A. F., and Sit, C. H. (2018). Motor skill interventions in children with developmental coordination disorder: a systematic review and meta-analysis. Arch. Phys. Med. Rehabil. 99, 2076–2099. doi: 10.1016/j.apmr.2017.12.009
Jebsen, R. H. (1969). An objective and standardized test of hand function. Arch. Phys. Med. Rehabil. 50, 311–319.
Kagerer, F. A., Bo, J., Contreras-Vidal, J. L., and Clark, J. E. (2004). Visuomotor adaptation in children with developmental coordination disorder. Motor Control 8, 450–460. doi: 10.1123/mcj.8.4.450
Kronberg, G., Bridi, M., Abel, T., Bikson, M., and Parra, L. C. (2017). Direct current stimulation modulates LTP and LTD: activity dependence and dendritic effects. Brain Stimul. 10, 51–58. doi: 10.1016/j.brs.2016.10.001
Lejeune, C., Catale, C., Wilems, S., and Meulemans, T. (2013). Intact procedural motor sequence learning in developmental coordination disorder. Res. Dev. Disabil. 34, 1974–1981. doi: 10.1016/j.ridd.2013.03.017
Levac, D., Wishart, L., Missiuna, C., and Wright, V. (2009). The application of motor learning strategies within functionally based interventions for children with neuromotor conditions. Pediatr. Phys. Ther. 21, 345–355. doi: 10.1097/PEP.0b013e3181beb09d
Manto, M., Bower, J. M., Conforto, A. B., Delgado-Garcia, J. M., Da Guarda, S., Gerwig, M., et al. (2012). Consensus paper: roles of the cerebellum in motor control–the diversity of ideas on cerebellar involvement in movement. Cerebellum 11, 457–487. doi: 10.1007/s12311-011-0331-9
McLaren, M. E., Nissim, N. R., and Woods, A. J. (2018). The effects of medication use in transcranial direct current stimulation: a brief review. Brain Stimul. 11, 52–58. doi: 10.1016/j.brs.2017.10.006
Mombarg, R., Jelsma, D., and Hartman, E. (2013). Effect of Wii-intervention on balance of children with poor motor performance. Res. Dev. Disabil. 34, 2996–3003. doi: 10.1016/j.ridd.2013.06.008
Muratori, L. M., Lamberg, E. M., Quinn, L., and Duff, S. V. (2013). Applying principles of motor learning and control to upper extremity rehabilitation. J. Hand Ther. 26, 94–103. doi: 10.1016/j.jht.2012.12.007
Palm, U., Segmiller, F. M., Epple, A. N., Freisleder, F. J., Koutsouleris, N., Schulte-Korne, G., et al. (2016). Transcranial direct current stimulation in children and adolescents: a comprehensive review. J. Neural Transmission 123, 1219–1234. doi: 10.1007/s00702-016-1572-z
R Core Team (2017). R: A Language and Environment for Statistical Computing. Vienna, Austria: R Foundation for Statistical Computing.
Reis, J., Schambra, H. M., Cohen, L. G., Buch, E. R., Fristch, B., Zarahn, E., et al. (2009). Noninvasive cortical stimulation enhances motor skill acquisition over multiple days through an effect on consolidation. Proc. Natl. Acad. Sci. U. S. A. 106, 1590–1595. doi: 10.1073/pnas.0805413106
Saint-Raymond, A., Hill, S., Martines, J., Bahl, R., Fontaine, O., and Bero, L. (2010). CONSORT 2010. Lancet 376, 229–230. doi: 10.1016/S0140-6736(10)61134-8
Saleem, G. T., Crasta, J. E., Solmine, B. S., Cantarero, G. L., and Suskauer, S. J. (2019). Transcranial direct current stimulation in pediatric motor disorders: a systematic review and meta-analysis. Arch. Phys. Med. Rehabil. 100, 724–738. doi: 10.1016/j.apmr.2018.10.011
Sanes, J. N., and Donoghue, J. P. (2000). Plasticity and primary motor cortex. Annu. Rev. Neurosci. 23, 393–415. doi: 10.1146/annurev.neuro.23.1.393
Schulz, K. F., Altman, D. G., Moher, D., and Consort Group. (2010). CONSORT 2010 statement: updated guidelines for reporting parallel group randomised trials. Trials 11:32. doi: 10.1186/1745-6215-11-32
Smits-Engelsman, B. C., Blank, R., Van Der Kaay, A. C., Mosterd-Van Der Meijs, R., Vlugt-Van Den Brand, E., Polatajko, H. J., et al. (2013). Efficacy of interventions to improve motor performance in children with developmental coordination disorder: a combined systematic review and meta-analysis. Dev. Med. Child Neurol. 55, 229–237. doi: 10.1111/dmcn.12008
Smits-Engelsman, B. C., Bouwien, C. M., Jelsma, L. D., Ferguson, G. D., and Geuze, R. H. (2015). Motor learning: an analysis of 100 trials of a ski slalom game in children with and without developmental coordination disorder. PLoS ONE 10:e0140470. doi: 10.1371/journal.pone.0140470
Stagg, C. J., and Nitsche, M. A. (2011). Physiological basis of transcranial direct current stimulation. Neuroscientist 17, 37–53. doi: 10.1177/1073858410386614
Steinmetz, H., Furst, G., and Meyer, B. U. (1989). Craniocerebral topography within the international 10-20 system. Electroencephalogr. Clin. Neurophysiol. 72, 499–506. doi: 10.1016/0013-4694(89)90227-7
Thibaut, A., Zafonte, R., Morse, L. R., and Fregni, F. (2017). Understanding negative results in tDCS research: the importance of neural targeting and cortical engagement. Front. Neurosci. 11:707. doi: 10.3389/fnins.2017.00707
Tiffin, J., and Asher, E. J. (1948). The purdue pegboard; norms and studies of reliability and validity. J. Appl. Psychol. 32, 234–247. doi: 10.1037/h0061266
Todorov, E. (2003). “On the role of primary motor cortex in arm movement control,” in Progress in Motor Control: Vol. 3, eds M. L. Latash and M. F. Levin (Champaign, IL: Human Kinetics), 125–166.
Wechsler, D. (2011). Wechsler Abbreviated Scales of Intelligence-Second Edition (WASI-II). San Antonio, TX: NCS Pearson.
Woods, A. J., and Martin, D. M. (2016). “Clinical research and methodological aspects for tDCS research,” in Transcranial Direct Current Stimulation in Neuropsychiatric Disorders, eds A. Brunoni, M. Nitsche, and C. Loo (New York, NY; Cham: Springer), 393–404. doi: 10.1007/978-3-319-33967-2_26
Zewdie, E., Ciechanski, P., Kuo, H. C., Giuffre, A., Kahl, C., King, R., et al. (2020). Safety and tolerability of transcranial magnetic and direct current stimulation in children: prospective single center evidence from 3.5 million stimulations. Brain Stimul. 13, 565–575. doi: 10.1016/j.brs.2019.12.025
Keywords: neuromodulation, transcranial direct current stimulation, motor learning, developmental coordination disorder, randomized controlled trial
Citation: Grohs MN, Craig BT, Kirton A and Dewey D (2020) Effects of Transcranial Direct Current Stimulation on Motor Function in Children 8–12 Years With Developmental Coordination Disorder: A Randomized Controlled Trial. Front. Hum. Neurosci. 14:608131. doi: 10.3389/fnhum.2020.608131
Received: 19 September 2020; Accepted: 09 November 2020;
Published: 11 December 2020.
Edited by:
Kate Wilmut, Oxford Brookes University, United KingdomReviewed by:
Ian Fuelscher, Deakin University, AustraliaGreg Wood, Manchester Metropolitan University, United Kingdom
Copyright © 2020 Grohs, Craig, Kirton and Dewey. This is an open-access article distributed under the terms of the Creative Commons Attribution License (CC BY). The use, distribution or reproduction in other forums is permitted, provided the original author(s) and the copyright owner(s) are credited and that the original publication in this journal is cited, in accordance with accepted academic practice. No use, distribution or reproduction is permitted which does not comply with these terms.
*Correspondence: Deborah Dewey, ZG1kZXdleUB1Y2FsZ2FyeS5jYQ==