- 1Department of Neuroanatomy, Institute of Anatomy and Cell Biology, Faculty of Medicine, University of Freiburg, Freiburg, Germany
- 2Department of Psychiatry and Psychotherapy, Medical Center—Faculty of Medicine, University of Freiburg, Freiburg, Germany
- 3Center for Basics in NeuroModulation (NeuroModulBasics), Faculty of Medicine, University of Freiburg, Freiburg, Germany
- 4Center Brain Links Brain Tools, University of Freiburg, Freiburg, Germany
Single-pulse and repetitive transcranial magnetic stimulation (rTMS) are used in clinical practice for diagnostic and therapeutic purposes. However, rTMS-based therapies that lead to a significant and sustained reduction in neuropsychiatric symptoms remain scarce. While it is generally accepted that the stimulation frequency plays a crucial role in producing the therapeutic effects of rTMS, less attention has been dedicated to determining the role of the electric field strength. Conventional threshold-based intensity selection approaches, such as the resting motor threshold, produce variable stimulation intensities and electric fields across participants and cortical regions. Insufficient standardization of electric field strength may contribute to the variability of rTMS effects and thus therapeutic success. Computational approaches that can prospectively optimize the electric field and standardize it across patients and cortical targets may overcome some of these limitations. Here, we discuss these approaches and propose that electric field standardization will be instrumental for translational science frameworks (e.g., multiscale modeling and basic science approaches) aimed at deciphering the subcellular, cellular, and network mechanisms of rTMS. Advances in understanding these mechanisms will be important for optimizing rTMS-based therapies in psychiatry.
Introduction
Transcranial magnetic stimulation (TMS) is a non-invasive brain stimulation (NIBS) method widely used in neuroscience research and clinical practice (Huang et al., 2017; Bergmann and Hartwigsen, 2020). Based on the physical principle of electromagnetic induction, TMS produces short (~200–500 μs) but strong (>1.5 Tesla) magnetic fields that penetrate the intact skin and skull of patients. Hence, TMS produces its major effects by inducing peak absolute electric fields (~100 mV/mm) in cortical brain regions (Paulus et al., 2013).
When applied repeatedly (i.e., ≧1 Hz), repetitive TMS (rTMS) induces lasting changes in cortical excitability and plasticity, making rTMS a suitable tool for modulating complex brain function in health and disease (Lefaucheur et al., 2014; Huang et al., 2017). Recent research has demonstrated that rTMS is capable of inducing long-lasting plasticity of excitatory and inhibitory neurotransmission in animal models (Gersner et al., 2011; Ma et al., 2013; Lenz et al., 2016, 2020; Tang et al., 2017).
TMS was first introduced in 1985 (Barker et al., 1985), and the first rTMS study was performed in 1991 in epileptic patients (Pascual-Leone et al., 1991). Four years later, the first rTMS study in psychiatric patients suffering from depression was published (George et al., 1995). Since then, rTMS has been used to treat various neuropsychiatric conditions associated with alterations in cortical excitability, including movement disorders, Alzheimer’s disease, depression, anxiety disorders, obsessive-compulsive disorders, and schizophrenia (Lefaucheur et al., 2014).
Two rTMS protocols received approval from the U.S. Food and Drug Administration (FDA) for the treatment of pharmacoresistant depression; the 10 Hz rTMS protocol in 2008 and the intermittent theta-burst stimulation (iTBS) protocol in 2018 (Figure 1). Indeed, a previous meta-analysis including 81 studies (n = 4,233) revealed significant short-term antidepressant effects of rTMS (Brunoni et al., 2017). The most recent meta-analysis including ten studies (six randomized controlled trials, n = 294; four uncontrolled clinical trials, n = 297) on iTBS to the dorsolateral prefrontal cortex (DLPFC) vs. standard rTMS reported overall effect sizes for response and remission rates of 0.38 and 0.20 in depressed patients, respectively (Chu et al., 2020). Finally, a meta-analysis of eighteen studies on the sustainability of rTMS effects in depression showed sustained response rates of about 50% 3, 6, and 12 months after initial treatment (Senova et al., 2019). In sum, these meta-analyses point to a significant efficacy and durability of rTMS in the treatment of depression.
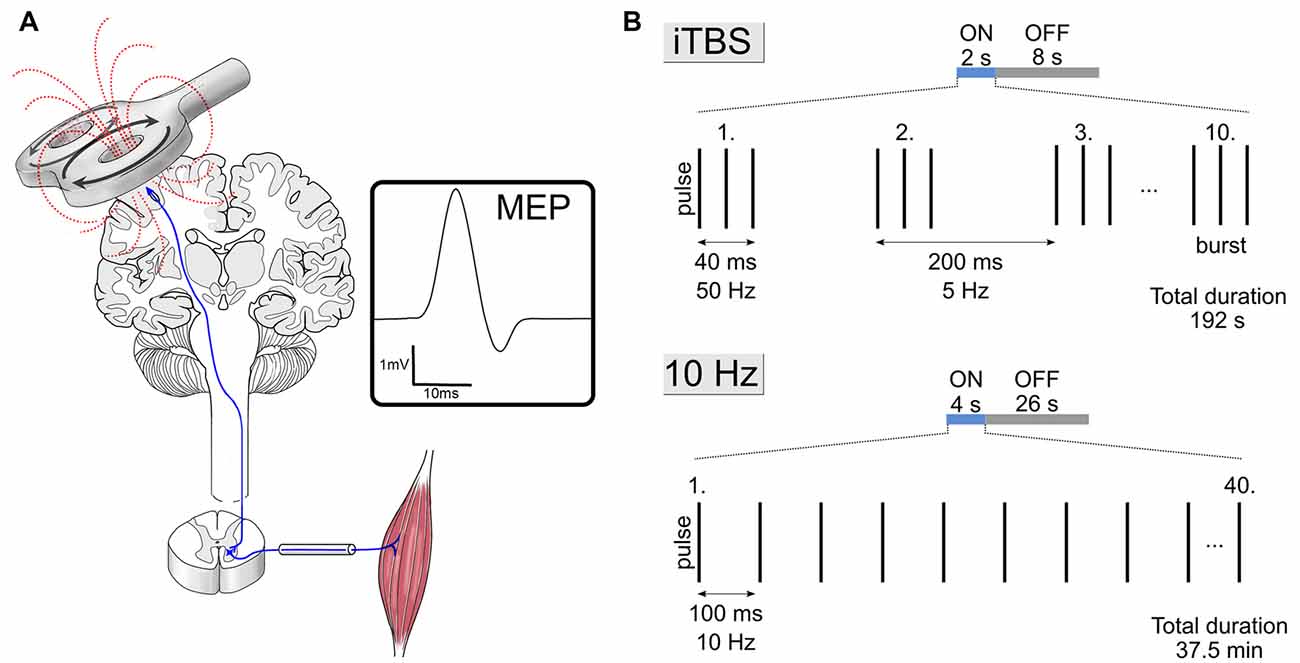
Figure 1. Single-pulse transcranial magnetic stimulation (TMS) and clinically approved stimulation protocols. (A) Visualization of TMS of the motor cortex while recording motor evoked potentials (MEP) of the target muscle. The intensity of TMS is adjusted by recording the amplitude of MEPs elicited from the target muscle while stimulating its motor cortical representation (see Vlachos et al., 2017). (B) Schematic illustration of stimulation parameters of the 10 Hz and intermittent theta-burst stimulation (iTBS) repetitive TMS (rTMS) protocols. Both protocols use stimulation intensities at 120% of the motor threshold.
Still, the clinical relevance of rTMS for depression is debated. Whereas short-term effects in treatment-resistant depression have been repeatedly demonstrated, sustained efficacy regarding clinically relevant outcomes has been questioned (Lepping et al., 2014; Kedzior et al., 2015; Ontario, 2016; Papadimitropoulou et al., 2017). Moreover, a high placebo response, technical difficulties in obtaining a double-blinded sham response, and rather small sample sizes compared to large psychopharmacological trials seem to hamper the validity of several rTMS clinical trials (Razza et al., 2018). Apparently, a more reliable and sustained clinical efficacy of rTMS—based on reproducible neurophysiological effects—is urgently needed.
While it is generally accepted that the stimulation frequency plays the dominant role in producing the therapeutic effects of rTMS, less attention has been dedicated to the role of electric field strength. In this perspective article, we discuss the use of computational modeling to standardize the stimulus intensities by closely matching electric field characteristics in a given cortical target across the study sample. Such an approach could be instrumental for developing suitable translational research frameworks for obtaining insights into the biological and therapeutic mechanisms of rTMS effects in the treatment of neuropsychiatric disorders.
Motor Threshold-Based Intensity Selection for Rtms
In human rTMS-studies, the vast majority of studies use the motor threshold (MT) approach for intensity selection (Turi et al., 2020). This approach adjusts the intensity of TMS by recording the amplitude of motor evoked potentials (MEPs) elicited from the target muscle while stimulating its motor cortical representation (Figure 1A). In research and clinical settings, typical intensities range between 80–120% of the MT for the stimulation of brain regions other than the motor cortex (e.g., the prefrontal cortex; Turi et al., 2020). For example, the FDA-approved 10 Hz and iTBS protocols (Figure 1B) both use 120% of the resting MT intensity for the stimulation of the prefrontal cortex (Blumberger et al., 2018).
One limitation of the MT approach is that the properties of the electric fields, both in the motor cortex and therapeutically targeted brain regions, remain undefined. Moreover, the physiological mechanisms of inducing MEPs by TMS are not completely understood in humans. For example, a crucial question pertains to the mechanisms of TMS activation of layer V pyramidal neurons (Di Lazzaro and Ziemann, 2013). These neurons form the corticospinal tracts, driving the activation of motor neurons in the spinal cord and subsequent muscle activation. It is currently unclear whether layer V pyramidal neurons are depolarized directly by TMS or indirectly via the stimulation of axons terminating onto layer V pyramidal neurons (Di Lazzaro and Ziemann, 2013). Likewise, the role of (in)direct activation of interneurons during TMS remains unclear.
MT intensities can vary substantially across participants, and studies frequently fail to report the stimulation intensity translated into physical parameters, such as percent of the maximum device output (Turi et al., 2020). In this context, it is important to note that the amplitude of MEPs is susceptible to attentional and voluntary mechanisms (Bell et al., 2018; Ruddy et al., 2018). Participants can be trained to significantly decrease or increase the amplitude of their MEPs voluntarily (Ruddy et al., 2018). Thus, intensities corresponding to a given MT are expected to yield distinct electric field strengths in different participants or even in the same participants under certain conditions.
Finally, the stimulation intensities estimated with the MT approach may vary substantially between studies depending on the type of the threshold (e.g., active or resting) and the exact procedure used for detecting the MT (e.g., visual vs. electrophysiological MEP detection). Hence, this conventional intensity selection approach cannot adequately standardize the electric field properties across participants and cortical regions. Thus, a remaining open question is whether the MT-based intensity selection approach can explain, at least in part, the considerable inter- and intra-individual variability of rTMS-induced aftereffects.
Standardization of Stimulus Parameters in Basic Science Experiments
From a translational point of view, the stimulation intensity expressed as a given percentage of the MT (e.g., 120% resting MT), is not informative for basic science experiments aimed at deciphering the mechanisms of rTMS-based therapies. A major advantage of carrying out rTMS experiments in suitable animal models both in vivo and in vitro is the ability to readily standardize electric fields (and other stimulation parameters) across experiments. For example, in our in vitro experimental procedures, all stimulation parameters are kept constant, and the same target volume is stimulated in every experiment, i.e., brain tissue cultures in a standard 35 mm Petri dish filled with artificial cerebrospinal fluid (see Müller-Dahlhaus and Vlachos, 2013). Due to the standardized Petri dish volume, coil-to-tissue culture distance, and tissue culture size, this approach yields closely-matched electric fields in our laboratory.
Indeed, the effects of 10 Hz repetitive magnetic stimulation on synaptic plasticity in vitro are robust and highly reproducible (Vlachos et al., 2012; Lenz et al., 2015, 2016, 2020). It is important to emphasize, however, that in vitro preparations are not comparable to the complex in vivo situation and do not allow for a straightforward translation to treating the diseased human brain. Nevertheless, careful standardization of stimulus parameters—specifically, electric field strength and direction—seems mandatory for a systematic assessment of factors that may affect the outcome of a given (standardized) therapeutic rTMS protocol in suitable animal models (De Risio et al., 2020) as well as human rTMS studies.
Importance of Electric Field Strength Standardization in Clinical Settings
The relevance of careful standardization of electric fields is also supported by studies using transcranial electric stimulation (tES; Antal et al., 2017); another clinically employed NIBS method. The two most frequent tES approaches use either direct (i.e., constant) or alternating (i.e., oscillating) currents for brain stimulation between two or more electrodes that are attached to the skin of the skull.
Studies using tES congruently suggest that the stimulation intensity can have a significant impact on the physiological aftereffects of the intervention. For example, Batsikadze et al. (2013) have shown distinct aftereffects of transcranial direct current stimulation (tDCS) applied at 1 and 2 mA intensities. The corticospinal excitability in humans was decreased by 1 mA cathodal tDCS, whereas 2 mA increased excitability (Batsikadze et al., 2013).
Similarly, Moliadze et al. (2012) demonstrated opposing effects of 140 Hz transcranial alternating current stimulation (tACS) at different intensities. While 0.6 mA decreased the level of corticospinal excitability, 1 mA increased it (corresponding to less than 0.2 mV/mm change in the absolute electric field in the motor cortex). These studies suggest that small changes in the electric field strength play a crucial role in inducing the physiological aftereffects of tES.
The electric fields induced by TMS in the human cortex are several-fold stronger than the electric fields achieved with tES or magnetic stimulation of the rodent brain in vivo and in vitro. Therefore, a systematic analysis of the dose-response effects—the role of the electric field strength in rTMS-induced (therapeutic) aftereffects—seems urgently needed. In this context, computational modeling has the potential to provide a translational framework for the physical input parameters of TMS, such as electric field properties, and neural responses in the human cortex and in suitable animal models.
Computational Modeling for Estimating Electric Fields and Prospective Electric Field Optimization
In recent years, sophisticated computer models have been developed to numerically calculate the induced electric fields in whole-brain volume conductor models (Figure 2). There are several, free toolboxes available for NIBS (Thielscher et al., 2015; Huang et al., 2019). These toolboxes can generate anatomically realistic, multi-compartment head models derived from structural magnetic resonance imaging data of the participants. The three-dimensional head models are generated by finite element or boundary element methods (Saturnino et al., 2019).
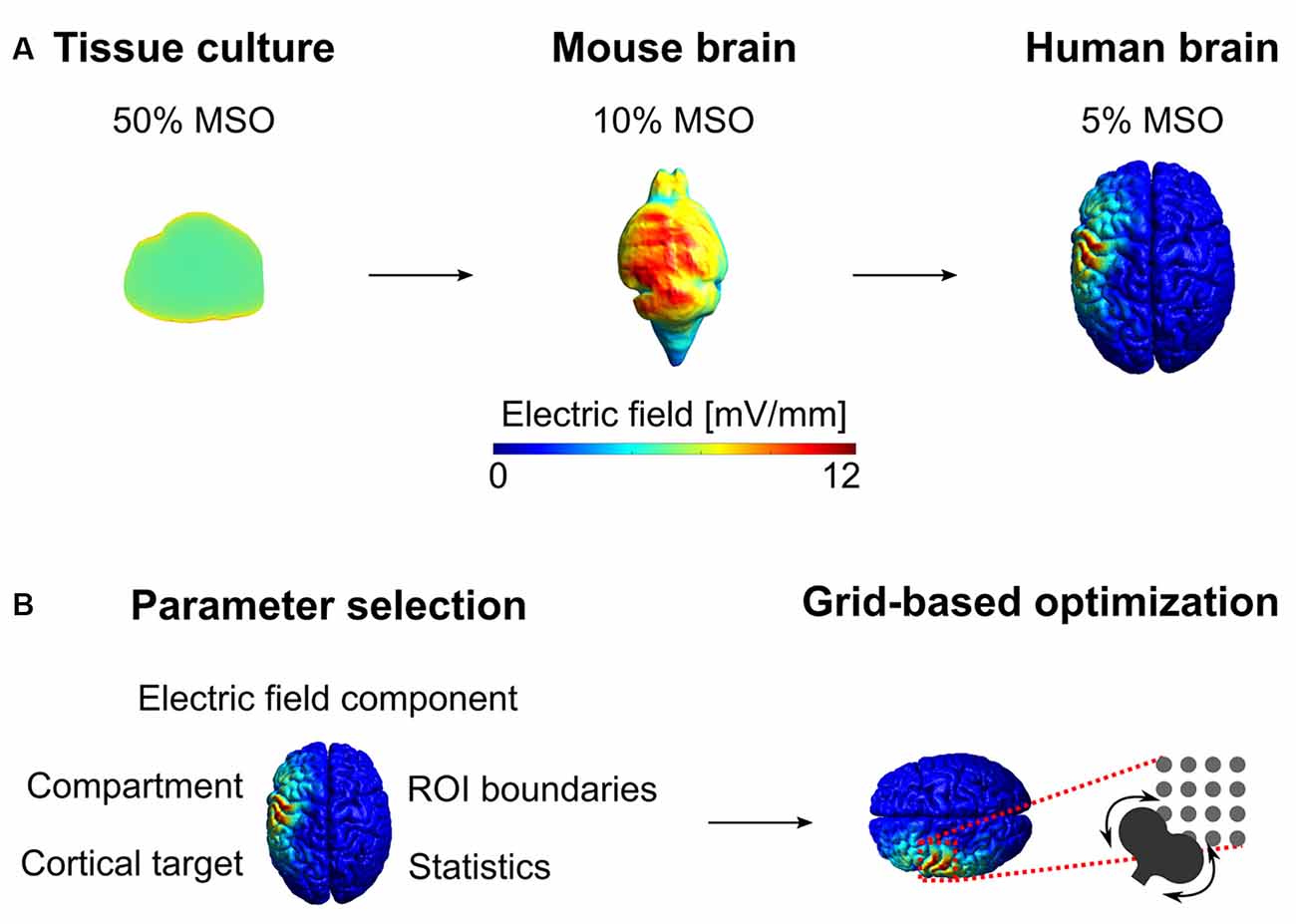
Figure 2. Electric field modeling of in vitro tissue culture and in the mouse and human brain. (A) First, we estimated the electric field in the tissue culture produced by the figure-of-eight TMS coil at 50% of the maximum stimulator output (MSO). Then, we calculated the robust maximum (i.e., 99.9th percentile) of the electric field distribution in the tissue culture and optimized the stimulation intensities to reproduce the same robust maximum in the gray matter volume compartment. Due to the large difference between the brain volumes, the stimulator intensity (expressed in MSO%) is substantially weaker for the mouse and human brain. The spatial distribution of the electric field intensities is more homogenous in the tissue culture with simplified geometry compared to the mouse and human brain. The mesh file for the mouse brain was obtained from Alekseichuk et al. (2019). (B) The choice of simulation parameters influences the characteristics of the electric field and the outcome of the optimization.
Electric field modeling seems to be particularly relevant when determining the stimulation intensity in cortical brain regions, such as DLPFC, that do not produce readily detectable responses, unlike the motor (e.g., MEPs) or visual (e.g., phosphenes) systems. In these cases, computational modeling can be used to adapt and standardize the stimulation intensities based on a prospective electric field optimization approach (Balderston et al., 2020; Beynel et al., 2020; Zmeykina et al., 2020).
After the preparation of the individual head model, several crucial simulation parameters must be considered for prospective electric field optimization (Figure 2B). To begin with, one needs to define the cortical target of the stimulation using one of the several approaches. These include; to define locations relative to the motor cortical representation of a given hand muscle (e.g., the 5 cm rule; Fitzgerald et al., 2009) or a probabilistic map of structural MRI (e.g., Montreal neuroimaging coordinates transformed into subject space; Blumberger et al., 2018). Other methods may use the location where phosphenes can be induced (i.e., occipital lobe; Brückner and Kammer, 2016), functional lesions (e.g., speech arrest; Pascual-Leone et al., 1991), or locations corresponding to electroencephalogram (EEG) electrodes (Zmeykina et al., 2020). Also, locations may be determined by magnetoencephalogram/EEG source analysis or fMRI functional localization at the single-subject or group level (Beynel et al., 2020; Zhang et al., 2020).
The electric field optimization may focus on the cortical surface or the volume compartment (Alekseichuk et al., 2019). Similarly, one may calculate the electric field properties at the entire compartment (e.g., gray matter volume) or on its subset, also called the region of interest (ROI). For ROI-based electric field analysis, one needs to specify its boundaries (e.g., it is surface and volume coordinates) and its shape (e.g., spherical, following the cortical folding pattern, et cetera). The choice of the ROI size will affect certain electric field properties. For example, around the same cortical target, the mean or median electric field strength will be weaker in larger ROIs (e.g., spherical ROI with 20 mm diameter) compared to a smaller ROI that elements are closer to the coil (e.g., 5 mm diameter spherical ROI).
Because the electric field is a complex, three-dimensional vector field characterized by its amplitude and direction, the characteristics of the electric field that will be considered in the electric field optimization process need to be determined. The electric field can be optimized for the absolute electric field strength or its normal (radial) or tangential spatial component for the cortical surface (Alekseichuk et al., 2019). Moreover, there are several ways to characterize the distribution of the electric field strength values in the ROIs, including calculating the robust maximum (usually between the 98th and 99.9th percentiles), mean, median, etc. values (Alekseichuk et al., 2019; Zmeykina et al., 2020).
The resulting electric field characteristics strongly depend on the coil center and its exact orientation. Therefore, a common procedure is to perform grid simulations on a series of predetermined coil locations while systematically manipulating the coil’s rotation angle around the coil’s center axis at each grid location. The outcome of this process is the most optimal coil location, orientation, and device intensity that produces the prospectively determined electric field characteristics in the ROI.
Conclusion and Outlook
Despite careful standardization of the electric field strength, it is conceivable to assume that there is no single, universally effective electric field strength value. Instead, different electric field strengths likely induce different neural effects, perhaps even concurrently in a given cortical target (Liu et al., 2018). For example, weaker electric fields may only induce immediate effects, such as temporal shifts in neural spike timing, without inducing long-lasting aftereffects (Zmeykina et al., 2020). Protocols with stronger electric fields may reach the threshold for the induction of plasticity of excitatory or inhibitory neurotransmission (see Lenz and Vlachos, 2016).
Reverse translational approaches combining basic science methods with computational modeling can facilitate the identification of effective electric field strengths for different neuronal mechanisms. For example, one interesting approach may use standardized electric field values in a human neocortical target that has been demonstrated to exert specific aftereffects in an animal model. However, we have to concede that the link between the rTMS-induced cellular aftereffects and their therapeutic potentials in psychiatry remains unclear.
It is important to also emphasize that electric field estimation can provide only approximate values of the de facto electric field produced. Tissue segmentation inaccuracies, especially between the skull and cerebrospinal fluid can have a substantial effect on the estimated values. Computational modeling toolboxes require additional validation of the induced electric field for TMS. Therefore, one should interpret the exact electric field values with caution.
In summary, we propose that a more careful standardization of electric field strength in rTMS is instrumental for the optimization of current rTMS-based therapies in neuropsychiatric phenotypes. Prospective electric field simulations have the potential to provide a translational framework across distinct scales and experimental settings. Multiscale neuronal modeling of realistic rodent and human neurons provides a promising tool in rapidly screening distinct stimulus intensities, orientations, frequencies, and pulse numbers that can be validated in a translational approach for the optimization of rTMS-based therapies in psychiatry (Shirinpour et al., 2020).
Data Availability Statement
The original contributions presented in the study are included in the article, further inquiries can be directed to the corresponding author.
Author Contributions
ZT, CN, KD, and AV wrote the manuscript. ZT and AV prepared figures. All authors contributed to the article and approved the submitted version.
Funding
The work was supported by the Federal Ministry of Education and Research Germany (Bundesministerium für Bildung und Forschung, BMBF, 01GQ1804A) and the National Institutes of Health USA (R01NS109498).
Conflict of Interest
The authors declare that the research was conducted in the absence of any commercial or financial relationships that could be construed as a potential conflict of interest.
References
Alekseichuk, I., Mantell, K., Shirinpour, S., and Opitz, A. (2019). Comparative modeling of transcranial magnetic and electric stimulation in mouse, monkey and human. NeuroImage 194, 136–148. doi: 10.1016/j.neuroimage.2019.03.044
Antal, A., Alekseichuk, I., Bikson, M., Brockmöller, J., Brunoni, A. R., Chen, R., et al. (2017). Low intensity transcranial electric stimulation: safety, ethical, legal regulatory and application guidelines. Clin. Neurophysiol. 128, 1774–1809. doi: 10.1016/j.clinph.2017.06.001
Balderston, N. L., Roberts, C., Beydler, E. M., Deng, Z.-D., Radman, T., Luber, B., et al. (2020). A generalized workflow for conducting electric field-optimized, fMRI-guided, transcranial magnetic stimulation. Nat. Protoc. 15, 3595–3614. doi: 10.1038/s41596-020-0387-4
Barker, A. T., Jalinous, R., and Freeston, I. L. (1985). Non-invasive magnetic stimulation of human motor cortex. Lancet 1, 1106–1107. doi: 10.1016/s0140-6736(85)92413-4
Batsikadze, G., Moliadze, V., Paulus, W., Kuo, M. F., and Nitsche, M. A. (2013). Partially non-linear stimulation intensity-dependent effects of direct current stimulation on motor cortex excitability in humans. J. Physiol. 591, 1987–2000. doi: 10.1113/jphysiol.2012.249730
Bell, S. J., Lauer, A., Lench, D. H., and Hanlon, C. A. (2018). Visual attention affects the amplitude of the transcranial magnetic stimulation-associated motor-evoked potential: a preliminary study with clinical utility. J. Psychiatr. Pract. 24, 220–229. doi: 10.1097/PRA.0000000000000321
Bergmann, T. O., and Hartwigsen, G. (2020). Inferring causality from noninvasive brain stimulation in cognitive neuroscience. J. Cogn. Neurosci. 33, 1–29. doi: 10.1162/jocn_a_01591
Beynel, L., Davis, S. W., Crowell, C. A., Dannhauer, M., Lim, W., Palmer, H., et al. (2020). Site-Specific effects of online rTMS during a working memory task in healthy older adults. Brain Sci. 10:255. doi: 10.3390/brainsci10050255
Blumberger, D. M., Vila-Rodriguez, F., Thorpe, K. E., Feffer, K., Noda, Y., Giacobbe, P., et al. (2018). Effectiveness of theta burst versus high-frequency repetitive transcranial magnetic stimulation in patients with depression (THREE-D): a randomized non-inferiority trial. Lancet 391, 1683–1692. doi: 10.1016/S0140-6736(18)30295-2
Brückner, S., and Kammer, T. (2016). Modulation of visual cortex excitability by continuous theta burst stimulation depends on coil type. PLoS One 11:e0159743. doi: 10.1088/1361-648X/abe6e0
Brunoni, A. R., Chaimani, A., Moffa, A. H., Razza, L. B., Gattaz, W. F., Daskalakis, Z. J., et al. (2017). Repetitive transcranial magnetic stimulation for the acute treatment of major depressive episodes: a systematic review with network meta-analysis. JAMA Psychiatry 74, 143–152. doi: 10.1001/jamapsychiatry.2016.3644
Chu, H.-T., Cheng, C.-M., Liang, C.-S., Chang, W.-H., Juan, C.-H., Huang, Y.-Z., et al. (2020). Efficacy and tolerability of theta-burst stimulation for major depression: a systematic review and meta-analysis. Prog. Neuropsychopharmacol. Biol. Psychiatry 106:110168. doi: 10.1016/j.pnpbp.2020.110168
De Risio, L., Borgi, M., Pettorruso, M., Miuli, A., Ottomana, A. M., Sociali, A., et al. (2020). Recovering from depression with repetitive transcranial magnetic stimulation (rTMS): a systematic review and meta-analysis of preclinical studies. Transl. Psychiatry 10:393. doi: 10.1038/s41398-020-01055-2
Di Lazzaro, V., and Ziemann, U. (2013). The contribution of transcranial magnetic stimulation in the functional evaluation of microcircuits in human motor cortex. Front. Neural Circuits 7:18. doi: 10.3389/fncir.2013.00018
Fitzgerald, P. B., Maller, J. J., Hoy, K. E., Thomson, R., and Daskalakis, Z. J. (2009). Exploring the optimal site for the localization of dorsolateral prefrontal cortex in brain stimulation experiments. Brain Stimul. 2, 234–237. doi: 10.1016/j.brs.2009.03.002
George, M. S., Wassermann, E. M., Williams, W. A., Callahan, A., Ketter, T. A., Basser, P., et al. (1995). Daily repetitive transcranial magnetic stimulation (rTMS) improves mood in depression. Neuroreport 6, 1853–1856. doi: 10.1097/00001756-199510020-00008
Gersner, R., Kravetz, E., Feil, J., Pell, G., and Zangen, A. (2011). Long-term effects of repetitive transcranial magnetic stimulation on markers for neuroplasticity: differential outcomes in anesthetized and awake animals. J. Neurosci. 31, 7521–7526. doi: 10.1523/JNEUROSCI.6751-10.2011
Huang, Y., Datta, A., Bikson, M., and Parra, L. C. (2019). Realistic volumetric-approach to simulate transcranial electric stimulation-ROAST-a fully automated open-source pipeline. J. Neural Eng. 16:56006. doi: 10.1088/1741-2552/ab208d
Huang, Y.-Z., Lu, M.-K., Antal, A., Classen, J., Nitsche, M., Ziemann, U., et al. (2017). Plasticity induced by non-invasive transcranial brain stimulation : a position article. Clin. Neurophysiol. 128, 2318–2329. doi: 10.1016/j.clinph.2017.09.007
Kedzior, K. K., Reitz, S. K., Azorina, V., and Loo, C. (2015). Durability of the antidepressant effect of the high-frequency repetitive transcranial magnetic stimulation (rTMS) In the absence of maintenance treatment in major depression: a systematic review and meta-analysis of 16 double-blind, randomized, sham-contr. Depress. Anxiety 32, 193–203. doi: 10.1002/da.22339
Lefaucheur, J. P., André-Obadia, N., Antal, A., Ayache, S. S., Baeken, C., Benninger, D. H., et al. (2014). Evidence-based guidelines on the therapeutic use of repetitive transcranial magnetic stimulation (rTMS). Clin. Neurophysiol. 125, 2150–2206. doi: 10.1016/j.clinph.2014.05.021
Lenz, M., and Vlachos, A. (2016). Releasing the Cortical Brake by Non-Invasive Electromagnetic Stimulation’s? rTMS Induces LTD of GABAergic Neurotransmission. Front. Neural Circuits 10:96. doi: 10.3389/fncir.2016.00096
Lenz, M., Eichler, A., Kruse, P., Strehl, A., Rodriguez-Rozada, S., Goren, I., et al. (2020). Neural inflammation alters synaptic plasticity probed by 10 Hz repetitive magnetic stimulation. BioRxiv [Preprint]. doi: 10.1101/2020.10.16.336065
Lenz, M., Galanis, C., Mu, F., Opitz, A., and Wierenga, C. J. (2016). Repetitive magnetic stimulation induces plasticity of inhibitory synapses. Nat. Commun. 7:10020. doi: 10.1038/ncomms10020
Lenz, M., Platschek, S., Priesemann, V., Becker, D., Willems, L. M., Ziemann, U., et al. (2015). Repetitive magnetic stimulation induces plasticity of excitatory postsynapses on proximal dendrites of cultured mouse CA1 pyramidal neurons. Brain Struct. Funct. 220, 3323–3337. doi: 10.1007/s00429-014-0859-9
Lepping, P., Schönfeldt-Lecuona, C., Sambhi, R. S., Lanka, S. V. N., Lane, S., Whittington, R., et al. (2014). A systematic review of the clinical relevance of repetitive transcranial magnetic stimulation. Acta Psychiatr. Scand. 130, 326–341. doi: 10.1111/acps.12276
Liu, A., Vöröslakos, M., Kronberg, G., Henin, S., Krause, M. R., Huang, Y., et al. (2018). Immediate neurophysiological effects of transcranial electrical stimulation. Nat. Commun. 9:5092. doi: 10.1038/s41467-018-07233-7
Ma, J., Zhang, Z., Su, Y., Kang, L., Geng, D., Wang, Y., et al. (2013). Magnetic stimulation modulates structural synaptic plasticity and regulates BDNF-TrkB signal pathway in cultured hippocampal neurons. Neurochem. Int. 62, 84–91. doi: 10.1016/j.neuint.2012.11.010
Moliadze, V., Atalay, D., Antal, A., and Paulus, W. (2012). Close to threshold transcranial electrical stimulation preferentially activates inhibitory networks before switching to excitation with higher intensities. Brain Stimul. 5, 505–511. doi: 10.1016/j.brs.2011.11.004
Müller-Dahlhaus, F., and Vlachos, A. (2013). Unraveling the cellular and molecular mechanisms of repetitive magnetic stimulation. Front. Mol. Neurosci. 6:50. doi: 10.1055/a-1328-8468
Ontario, H. Q. (2016). Repetitive transcranial magnetic stimulation for treatment-resistant depression: a systematic review and meta-analysis of randomized controlled trials. Ont. Health Technol. Assess. Ser. 16, 1–66.
Papadimitropoulou, K., Vossen, C., Karabis, A., Donatti, C., and Kubitz, N. (2017). Comparative efficacy and tolerability of pharmacological and somatic interventions in adult patients with treatment-resistant depression: a systematic review and network meta-analysis. Curr. Med. Res. Opin. 33, 701–711. doi: 10.1080/03007995.2016.1277201
Pascual-Leone, A., Gates, J. R., and Dhuna, A. (1991). Induction of speech arrest and counting errors with rapid-rate transcranial magnetic stimulation. Neurology 41, 697–702. doi: 10.1212/wnl.41.5.697
Paulus, W., Peterchev, A. V., and Ridding, M. (2013). Transcranial electric and magnetic stimulation: technique and paradigms. Handb. Clin. Neurol. 116, 329–342. doi: 10.1016/B978-0-444-53497-2.00027-9
Razza, L. B., Moffa, A. H., Moreno, M. L., Carvalho, A. F., Padberg, F., Fregni, F., et al. (2018). A systematic review and meta-analysis on placebo response to repetitive transcranial magnetic stimulation for depression trials. Prog. Neuropsychopharmacol. Biol. Psychiatry 81, 105–113. doi: 10.1016/j.pnpbp.2017.10.016
Ruddy, K., Balsters, J., Mantini, D., Liu, Q., Kassraian-fard, P., Enz, N., et al. (2018). Neural activity related to volitional regulation of cortical excitability. eLife 7:e40843. doi: 10.7554/eLife.40843
Saturnino, G. B., Puonti, O., Nielsen, J. D., Antonenko, D., Madsen, K. H., and Thielscher, A. (2019). “SimNIBS 2.1: a comprehensive pipeline for individualized electric field modeling for transcranial brain stimulation” in Brain and Human Body Modeling: Computational Human Modeling at EMBC 2018, eds S. Makarov M. Horner and G. Noetscher (Cham, Switzerland: Springer), 3–25.
Senova, S., Cotovio, G., Pascual-Leone, A., and Oliveira-Maia, A. J. (2019). Durability of antidepressant response to repetitive transcranial magnetic stimulation: Systematic review and meta-analysis. Brain Stimul. 12, 119–128. doi: 10.1016/j.brs.2018.10.001
Shirinpour, S., Hananeia, N., Rosado, J., Galanis, C., Vlachos, A., Jedlicka, P., et al. (2020). Multi-scale modeling toolbox for single neuron and subcellular activity under (repetitive) transcranial magnetic stimulation. BioRxiv [Preprint]. doi: 10.1101/2020.09.23.310219
Tang, A., Thickbroom, G., and Rodger, J. (2017). Repetitive transcranial magnetic stimulation of the brain: mechanisms from animal and experimental models. The Neuroscientist 23, 82–94. doi: 10.1177/1073858415618897
Thielscher, A., Antunes, A., and Saturnino, G. B. (2015). Field modeling for transcranial magnetic stimulation: a useful tool to understand the physiological effects of TMS? Proc. Annu. Int. Conf. IEEE Eng. Med. Biol. Soc. EMBS 2015, 222–225. doi: 10.1109/EMBC.2015.7318340
Turi, Z., Lenz, M., Paulus, W., Mittner, M., and Vlachos, A. (2020). Selecting stimulation intensity in repetitive transcranial magnetic stimulation studies: a systematic review between 1991 and 2020. BioRxiv [Preprint]. doi: 10.1101/2020.09.28.316190
Vlachos, A., Funke, K., and Ziemann, U. (2017). Assessment and modulation of cortical inhibition using transcranial magnetic stimulation. Neuroforum 23, 9–17. doi: 10.1515/nf-2016-A103
Vlachos, A., Müller-Dahlhaus, F., Rosskopp, J., Lenz, M., Ziemann, U., and Deller, T. (2012). Repetitive magnetic stimulation induces functional and structural plasticity of excitatory postsynapses in mouse organotypic hippocampal slice cultures. J. Neurosci. 32, 17514–17523. doi: 10.1523/JNEUROSCI.0409-12.2012
Zhang, B., Liu, J., Bao, T., Wilson, G., Park, J., Zhao, B., et al. (2020). Locations for noninvasive brain stimulation in treating depressive disorders: a combination of meta-analysis and resting-state functional connectivity analysis. Aust. N. Z. J. Psychiatry 54, 582–590. doi: 10.1177/0004867420920372
Keywords: non-invasive brain stimulation, repetitive transcranial magnetic stimulation, motor threshold, electric field modeling, depression
Citation: Turi Z, Normann C, Domschke K and Vlachos A (2021) Transcranial Magnetic Stimulation in Psychiatry: Is There a Need for Electric Field Standardization? Front. Hum. Neurosci. 15:639640. doi: 10.3389/fnhum.2021.639640
Received: 09 December 2020; Accepted: 16 February 2021;
Published: 09 March 2021.
Edited by:
Abbas Z. Kouzani, Deakin University, AustraliaReviewed by:
Lekshmy Sudha Kumari, Deakin University, AustraliaDean Corva, Deakin University, Australia
Copyright © 2021 Turi, Normann, Domschke and Vlachos. This is an open-access article distributed under the terms of the Creative Commons Attribution License (CC BY). The use, distribution or reproduction in other forums is permitted, provided the original author(s) and the copyright owner(s) are credited and that the original publication in this journal is cited, in accordance with accepted academic practice. No use, distribution or reproduction is permitted which does not comply with these terms.
*Correspondence: Andreas Vlachos, YW5kcmVhcy52bGFjaG9zQGFuYXQudW5pLWZyZWlidXJnLmRl