Impairment and Compensation in Dexterous Upper-Limb Function After Stroke. From the Direct Consequences of Pyramidal Tract Lesions to Behavioral Involvement of Both Upper-Limbs in Daily Activities
- 1ISIR Institute of Intelligent Systems and Robotics, AGATHE Team, CNRS UMR 7222, INSERM U 1150, Sorbonne University, Paris, France
- 2LINP2-AAPS Laboratoire Interdisciplinaire en Neurosciences, Physiologie et Psychologie: Activité Physique, Santé et Apprentissages, UPL, Paris Nanterre University, Nanterre, France
Impairments in dexterous upper limb function are a significant cause of disability following stroke. While the physiological basis of movement deficits consequent to a lesion in the pyramidal tract is well demonstrated, specific mechanisms contributing to optimal recovery are less apparent. Various upper limb interventions (motor learning methods, neurostimulation techniques, robotics, virtual reality, and serious games) are associated with improvements in motor performance, but many patients continue to experience significant limitations with object handling in everyday activities. Exactly how we go about consolidating adaptive motor behaviors through the rehabilitation process thus remains a considerable challenge. An important part of this problem is the ability to successfully distinguish the extent to which a given gesture is determined by the neuromotor impairment and that which is determined by a compensatory mechanism. This question is particularly complicated in tasks involving manual dexterity where prehensile movements are contingent upon the task (individual digit movement, grasping, and manipulation…) and its objective (placing, two step actions…), as well as personal factors (motivation, acquired skills, and life habits…) and contextual cues related to the environment (presence of tools or assistive devices…). Presently, there remains a lack of integrative studies which differentiate processes related to structural changes associated with the neurological lesion and those related to behavioral change in response to situational constraints. In this text, we shall question the link between impairments, motor strategies and individual performance in object handling tasks. This scoping review will be based on clinical studies, and discussed in relation to more general findings about hand and upper limb function (manipulation of objects, tool use in daily life activity). We shall discuss how further quantitative studies on human manipulation in ecological contexts may provide greater insight into compensatory motor behavior in patients with a neurological impairment of dexterous upper-limb function.
Introduction
Impairments of dexterous upper-limb function are a significant cause of disability following an acquired brain injury or stroke since they affect approximately one half of the patients in this clinical population (Jorgensen et al., 1995). Recovery of upper-limb function after stroke has been the subject of numerous studies from both fundamental and clinical perspectives. Over the two last decades, various novel therapeutic interventions have been proposed (Winstein et al., 2016a). But despite impressive preclinical advances, patient outcomes in rehabilitation often remain disappointing, “with interesting science ultimately proving difficult to translate to the clinic” (Ward and Carmichael, 2020).
Defining hand dexterity proves somewhat complicated, with different perspectives emphasized across scientific disciplines (neurophysiology, cognitive science, humanities). From an etymological point of view, dexterity concerns mainly the right dominant hand. According to a modern dictionary, dexterity is the “readiness and grace in physical activity, especially skill and ease in using the hands” (Merriam-Webster). Exquisite hand dexterity is relatively specific to the human species which possesses several anatomical attributes (e.g., large moment arms for intrinsic muscles) facilitating independent finger movements and thumb opposition for precision grips (Napier, 1961; Marzke and Marzke, 2000). Obviously, human hand dexterity is equally related to the development of human neural substrates, particularly the monosynaptic cortico-motoneuronal system (Lemon et al., 2004). Lateralization and right-hand preference is also quite specific to humans and great apes (Cochet and Byrne, 2013). In addition, the human hand is remarkably versatile, being able to adopt a great variety of postures (Napier, 1956; Kapandji, 1980; Iberall et al., 1986). The control of such a sophisticated and mobile apparatus is highly cognitive. Dexterous upper-limb function relies on skills acquired during repetitive manipulation of objects or tools spanning decades, according to personal habitus (sport, arts, and work related) (Bril, 2015). Expertise is not only characterized by skillful execution of the tasks with optimal precision and timing, but also by fluidity, adaptability, versatility, and understanding of contextual affordances that are hardly summarized by tests outside the particular domain of expertise [review in Causby et al. (2014)]. According to Bernstein (1996) we shall consider that dexterity does not specifically refer to movement of the hand and fingers but more globally to the ability to engage the whole upper-limb in seamless interaction with tools or other objects appropriated from that person’s environment.
After stroke, the impairment of hand function largely depends on the location and extent of the brain lesion. While the physiological basis of movement deficits consequent to a lesion in the pyramidal tract is well demonstrated, specific mechanisms contributing to optimal recovery are less apparent. Indeed, the condition presented by any patient several months after stroke results from the direct consequences of the lesion alleviated by potential compensatory mechanisms developed by the patient in reaction to the impairment (Roby-Brami et al., 2003a; Levin et al., 2009). Various upper limb interventions (motor learning methods, neurostimulation techniques, robotics, virtual reality and serious games) are associated with improvements in motor performance, but many patients continue to experience significant limitations with object handling in everyday activities. Exactly how we go about consolidating adaptive motor behaviors through the rehabilitation process thus remains a considerable challenge. An important part of this problem is the ability to successfully distinguish the extent to which a given gesture is determined by the neuromotor impairment and by a compensatory mechanism. This question is particularly complicated in tasks involving manual dexterity where prehensile movement is contingent upon the task (individual digit movement, grasping, manipulation…) and its objective (placing, two step actions…), as well as personal factors (motivation, acquired skills, life habits…) and contextual cues related to the environment (presence of tools or assistive devices…).
However, in routine clinical examination, there is still a lack of comprehensive integrative methods capable of distinguishing the direct consequences of neuromotor impairment from compensatory strategies. The clinical evaluations of upper-limb function after stroke is based on several tests, organized according to the International Classification of Functioning (ICF). The global motor impairment is often measured using the Fugl-Meyer assessment (FMA) which examines movement speed, force, and range of motion through the upper limb, as well as the impact of abnormal synergies on voluntary actions (Fugl-Meyer et al., 1975). It is complemented by measures of spasticity (Ashworth). There exist numerous tests to quantify hand and upper-limb activity, most using the manipulation of objects such as Action Research Arm Test (ARAT), Jensen, Wolf Motor Function Test (WMFT) [see references in the reviews (Alt Murphy et al., 2015; Santisteban et al., 2016; Villepinte et al., 2020)]. In general, these tests give a unique score reflecting the level of success across a series of items, with penalties attributed where directives regarding timing or movement quality are not respected. Alterations of upper-limb dexterity is a significant cause of activity limitations that can be evaluated using the Motor Activity Log (MAL) which is a questionnaire investigating how frequently and how well the patient uses his/her affected upper limb at home (Taub et al., 1993). Most available methods for the evaluation of dexterity do not afford the description of compensatory strategies, with the exception of one recent proposition integrating observational kinematics to appreciate the quality of movement coordination (Alouche et al., 2020).
In this text, we shall question the link between consequences of brain lesions, functional impairments, motor strategies and performance in activity, particularly during object handling tasks. The presentation will focus on quantitative kinematic and kinetic studies, since we assume that routine use of quantitative methods should provide greater insight into compensatory motor behavior in stroke patients, in agreement with recent international consensus (Winstein and Varghese, 2018; Kwakkel et al., 2019). On a theoretical level, the perspective provided here draws upon the physiological basis of human motor control and concepts developed in ecological psychology. Following this logic, we consider that motor behavior emerges through interactions and reciprocal constraints defined by the specific task parameters, the individual’s sensorimotor attributes and the configuration of the environment (Newell, 1986). In a clinical context, this suggests that patients gravitate toward certain motor strategies adapted to their own specific condition (Latash and Anson, 1996) through action-perception cycles (enaction). This description of dexterity is consistent with the theoretical framework of Embodied, Embedded, Enactive, and Extended cognition (4E perspective) suggesting that the shape of individual motor strategies is embodied (dependent of bodily constraints), embedded into the environment (e.g., home), enacted (built through interaction) and potentially extended by assistive devices (Rowlands, 2010). According to these approaches, we shall consider that factors influencing human dexterity extend much beyond the ability to move fingers or to handle simple objects. Indeed, daily life activity is both the final outcome of post-stroke rehabilitation and a means in itself as part of a comprehensive rehabilitation program.
In this text, we examine those features of upper limb function and manual dexterity which are direct consequences of the brain lesion and those which result from compensation. Our objective is to explore how these contrasting processes emerge during the course of neurorehabilitation. We shall examine the consequences of brain lesions and the process of compensation at three integrative levels: biological (brain plasticity and vicariance), elementary sensorimotor function (sensorimotor patterns) and activity (acquisition of new motor strategies for object reaching and handling) the two first levels together refer to ICF “body functions and structure.” In particular, we insist on the contribution of quantitative kinematic and kinetic methods for improved understanding of these recovery and compensation dynamics. Thereafter, we shall discuss some clinical implications for rehabilitation and some perspectives, with a specific focus on the use of novel technology in this field.
Biological Change to Upper Limb Motor Pathways Following Stroke
Neural Control of Dexterous Upper-Limb Function
Through the course of the 20th century, clinical studies documented how lesions to a given cerebral hemisphere induced contralateral hemiparesis, suggesting that the descending (pyramidal) tract projected to the opposite side of the body (Dejerine, 1914). Subsequent neuroanatomical studies showed that control of contralateral hand movements in macaque monkeys was directly dependent on the integrity of the descending corticospinal tract (CST) between the primary motor cortex M1 and the spinal level (Lawrence and Kuypers, 1968). Later, direct monosynaptic connections between M1 pyramidal neurons onto the spinal motor neurons were identified in monkeys (Muir and Lemon, 1983), then demonstrated in humans (Lemon et al., 2004). This descending monosynaptic pathway to the distal extremity is specific to primates (particularly humans and apes) capable of precision grip involving fingers and an opposable thumb. In lower mammals, descending motor commands for reaching and grasping are mediated via propriospinal and segmental interneurons [review in Alstermark and Isa (2012)]. While this latter system has also been demonstrated in humans (Pierrot-Deseilligny, 1996), its specific role in human prehension remains to be determined (Giboin et al., 2012). Beyond the monosynaptic CST pathway, there are multiple motor brain areas, in each hemisphere which, have descending projections contributing to motor tasks involving the whole upper-limb [review in Kollias et al. (2001)].
Hand dexterity and individual finger movements are critically dependent on somatosensory feedback provided by the primary somatosensory area or thalamus, as demonstrated in monkeys (Asanuma and Arissian, 1984). More generally, the control of motor behavior is distributed in a wide parieto-frontal network. The cognitive control of action is hierarchically organized in the frontal lobe (Koechlin and Jubault, 2006), with skilled action sequences orchestrated via the premotor cortex (Ohbayashi et al., 2016). Multisensory feedback, including visual and proprioceptive cues are integrated in the parietal lobe during goal directed behavior (Battaglia-Mayer et al., 2003), and ultimately projected back to the premotor cortex to inform the consequent gesture.
In brain injured patients after stroke, lesions generally extend beyond the CST, involving sensory and/or integrative brain areas. Somatosensory impairments linked to thalamic or parietal cortical lesions also significantly impair manual dexterity (Hermsdorfer et al., 2004; Meyer et al., 2016). The occurrence of neuropsychological syndromes such as apraxia or neglect remain outside the scope of the present review.
Role of the Corticospinal Tract in the Impairment and Spontaneous Recovery Post Stroke
In neurological clinics, the pathological condition directly related to M1 or CST lesions is referred to as upper motor neuron syndrome. Typically, this involves negative symptoms through the distal upper extremity with weakness of the hand and fingers resulting in decreased force, speed and range of motion as well as the loss of individuated finger control. At the same time, patterns of abnormal muscle overactivity such as spasticity due to increased excitability of the stretch reflex provoke spasticity (Burke et al., 2013; Levin, 2016) and muscle co-contraction (Gracies, 2005b) that can result in soft tissue contractures (Gracies, 2005a). Many recent studies documented the link between the lesion of the CST and the severity of the hemiparesis using brain imaging with tractography or electrophysiological techniques such as transcranial magnetic stimulation (TMS) (Lindberg et al., 2007; Buetefisch et al., 2018; Birchenall et al., 2019). The ability to make selective finger movements proves to be dependent on CST integrity (Lang and Schieber, 2004; Birchenall et al., 2019).
After stroke, hemiparesis may spontaneously recover during the subacute period, however, this recovery is inconsistent and usually incomplete, plateauing generally after a few months (chronic stage). An influential theory proposes that spontaneous recovery represents a fixed proportion, around 70%, of each patient’s maximum possible improvement (Prabhakaran et al., 2008). This rule has been confirmed in a variety of clinical conditions but its meaning remains disputed (Hope et al., 2019; Senesh and Reinkensmeyer, 2019). In effect, several studies show that certain patients do not necessarily obey this proportional rule for spontaneous recovery. Byblow et al. (2015) demonstrated that these outliers were characterized by severe alterations of the CST, demonstrated by anomalies of the motor potentials evoked by TMS. This suggests that the spontaneous recovery is heavily dependent on the restauration of neural tissue contributing to the ipsilesional corticomotor pathways.
Activity dependent brain plasticity has been demonstrated in animals for the primary motor area (Nudo et al., 1996). In addition, animal studies have demonstrated that after stroke, the plastic reorganization of the descending corticomotor pathways also involved the premotor and supplementary motor areas (Dancause, 2006). This has been confirmed by brain imaging studies in humans showing that recovery was associated with activation in ipsilesional medial-premotor and primary motor cortices [meta-analysis in Favre et al. (2014)].
Thusly, recovery at the biological level, in particular with respect to the ipsilesional CST, is an important vector for the return of upper limb motor control. At the same time, the neurological insult may give rise to a host of other vicariant biological structural processes involving the premotor and supplementary motor areas (Dancause, 2006) which may actively work to compensate for the deleted fibers of the pyramidal tract in order to contribute to the functional recovery of dexterity. Figure 1 provides a schematization of this recovery process, indicated in green.
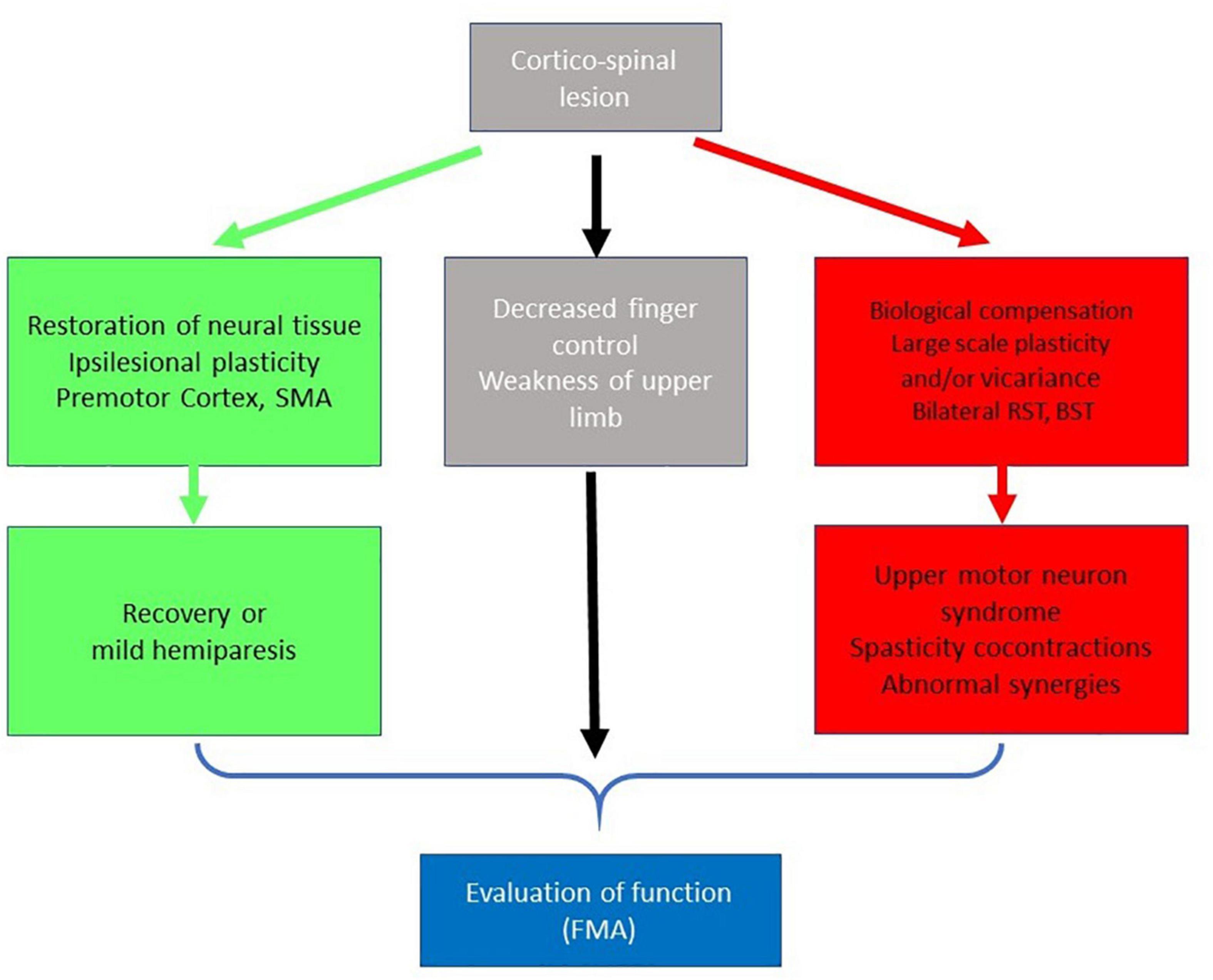
Figure 1. Simplified schema on the role of the Corticospinal tract in recovery. The extent of the CST lesion has direct consequences on dexterous upper limb control following stroke (gray squares). Spontaneous biological recovery can occur (green squares) via restoration of neural tissue or ipsilesional plasticity involving the premotor cortex and/or supplementary motor areas. Following a severe lesion, compensation for diminished CST integrity (red squares) may occur via large scale plasticity and vicariance involving polysynaptic bilateral pathways, including the reticulospinal and bulbospinal tracts. In this event, functional recovery is associated with maladaptive motor symptoms (upper-motor neuron syndrome). The level of impairment resulting from the combined effects of the CST lesion and neuroplastic change can be measured using motor performance tests such as the Fugl Meyer assessment (blue square).
Compensation Through Large Scale Vicariance and Neural Plasticity
After severe lesions of the CST, larger scale plasticity and excitability changes can result in alternative pathways being solicited across sensorimotor networks in both hemispheres (Xerri et al., 2014; Baker et al., 2015; Bundy and Nudo, 2019). This bilateral reorganization may sustain a certain level of upper-limb function, notably via reticulospinal and rubrospinal tracts (Belhaj-Saif and Cheney, 2000; Zaaimi et al., 2012). In clinical observations, a unilateral lesion of the CST induces bilateral impairment of upper-limb function. This is likely due to the presence, and consequent impairment, of non-decussating pathways to the ipsilateral segments (Desrosiers et al., 1996). Similar large scale bilateral reorganizations are also demonstrated in clinical studies (Gerloff et al., 2006; Baker et al., 2015; Buma et al., 2016; Peters et al., 2017). The involvement of alternative ipsilesional and contralesional brain areas relaying at subcortical level in the reticulospinal and rubrospinal tracts has been demonstrated in humans (Honeycutt et al., 2013; McMorland et al., 2015; McPherson et al., 2018). The specific role of the contralesional hemisphere is particularly disputed. On one hand, it could contribute to the recovery of the affected side thanks to ipsilateral cortico-spinal pathways (Dodd et al., 2017). On the other hand, it can have negative effects on the lesioned hemisphere via increased transcallosal inhibition (Murase et al., 2004). The reticulospinal system, which is activated bilaterally, contributes to motor function on the hemiparetic side, as shown by starling reactions, but this phenomenon is negatively correlated with hand dexterity (Choudhury et al., 2019). The alternative descending motor pathways converge on propriospinal relays which appear more heavily engaged in transmission of motor commands after stroke (Mazevet et al., 2003). The rearrangement of neuronal pathways due to plasticity and excitability changes has maladaptive effects. In particular, pathological muscle synergies, spasticity and excessive co-contractions are likely due to the exaggerated involvement of the reticulospinal (Li, 2017; McPherson et al., 2018) and/or bulbospinal tracts (Owen et al., 2017). For example, increases in fractional anisotropy in these pathways appears to be correlated with the severity of pathological upper limb synergies and hand impairment (Owen et al., 2017).
In brief, when the monosynaptic CST is severely lesioned, fine motor control and hand dexterity are compromised but large-scale changes in brain networks with multiple relays may assure a certain level of motor function at the cost of maladaptive phenomena. This process is indicated in red in Figure 1.
Impairments in Dexterous Coordination of the Upper Limb Post Stroke
Broadly speaking, patients with mild to moderate hemiparesis retain the global spatial organization of prehensile movement in the context of goal directed reaching, multi-finger actions during grasp and grip-load force coordination. Nonetheless, the neurological effects of the cerebrovascular accident bring about various irregularities in upper limb kinematics and dexterous control of the hand.
A pathophysiological analysis is necessary in order to better understand the mechanisms of improvement in function, and decipher compensation from true recovery. This means that clinical symptoms should be interpreted in the light of the physiology of the motor system. In the following section, we will focus on quantitative movement analysis (kinematics and kinetics) during well-defined elementary upper-limb tasks (Lemon, 1999; Nowak and Hermsdorfer, 2006; Kwakkel et al., 2019). These experimental paradigms which represent prototypes of naturalistic hand movements have been studied extensively in both healthy subjects and stroke patients.
Kinematics of Reach to Grasp Movements
Physiological Control of Goal Directed Movements
Pointing tasks involve the displacement of the hand, or working point of the upper limb toward an object of interest. In healthy subjects, this movement tends to be predominantly mediated via feed-forward control, as evidenced by the smooth, bell-shaped velocity profile described by the hand (Abend et al., 1982). Prehension tasks couple reach and grasp components, identified by the pioneering works of Jeannerod (1984). The pre-shaping of the finger aperture during reaching and the smooth peak velocity of the reaching hand show that control is anticipated as a function of the object position in space and of its intrinsic characteristics [review in Jeannerod (2009) see Smeets et al. (2019) for an alternative interpretation of the coupling]. In these types of prehensile tasks, the upper-limb may be seen to possess no less than 7 degrees of freedom (DoF) afforded by the rotational axes of the shoulder, elbow and wrist. The pointing task is defined by 6 DoF, corresponding to the 3D position and orientation of the object in space. As a result, there is kinematic redundancy at the level of the upper limb as the 7 DoF all contribute to the displacement of the hand for grasping in the 3D space (Desmurget and Prablanc, 1997). As initially proposed by Bernstein (Bernstein, 1967), control of the upper limb may be based on synergies which share the same spatio-temporal properties, and can be additively combined in a task specific way. There is still no agreement as to whether synergies are coordinated at the joint kinematic (Scholz et al., 2000; Yang et al., 2002) or muscular level (d’Avella and Bizzi, 2005; Ting and McKay, 2007; Bizzi et al., 2008). The Uncontrolled Manifold theory subscribes to the former, suggesting that synergies are flexible and allow automatic compensation between elements in order to stabilize the important task related variables such as the displacement of the endpoint (Yang et al., 2007; Latash, 2008; Martin et al., 2009). As proposed by Feldman, the neural basis of motor control, bridging the gap between physiology and biomechanics, could be the modulation of the stretch reflex threshold by the CST acting on motor neuron membrane potential (Feldman, 2015), consistent with the formation of synergies (Latash et al., 2010). This theory is disputed, and the reduction of redundancy by synergies has also been interpreted in the framework of optimal control (Guigon et al., 2007). However, there is accepted evidence that the primary motor cortex is responsible for the coordination of muscle and joints to generate the spatio-temporal form of goal directed movements (Kalaska, 2009; Capaday et al., 2013).
Movement Features Consecutive to CST Lesion or to Compensation in Stroke Patients
After stroke, patients generally exhibit decreased force and range of motion across joints with alterations in movement coordination, referred to as pathological synergies (Brunnstrom, 1970). Kinematic analysis underscores these direct consequences of the CST lesion and may equally serve to distinguish certain compensatory mechanisms (Figure 2).
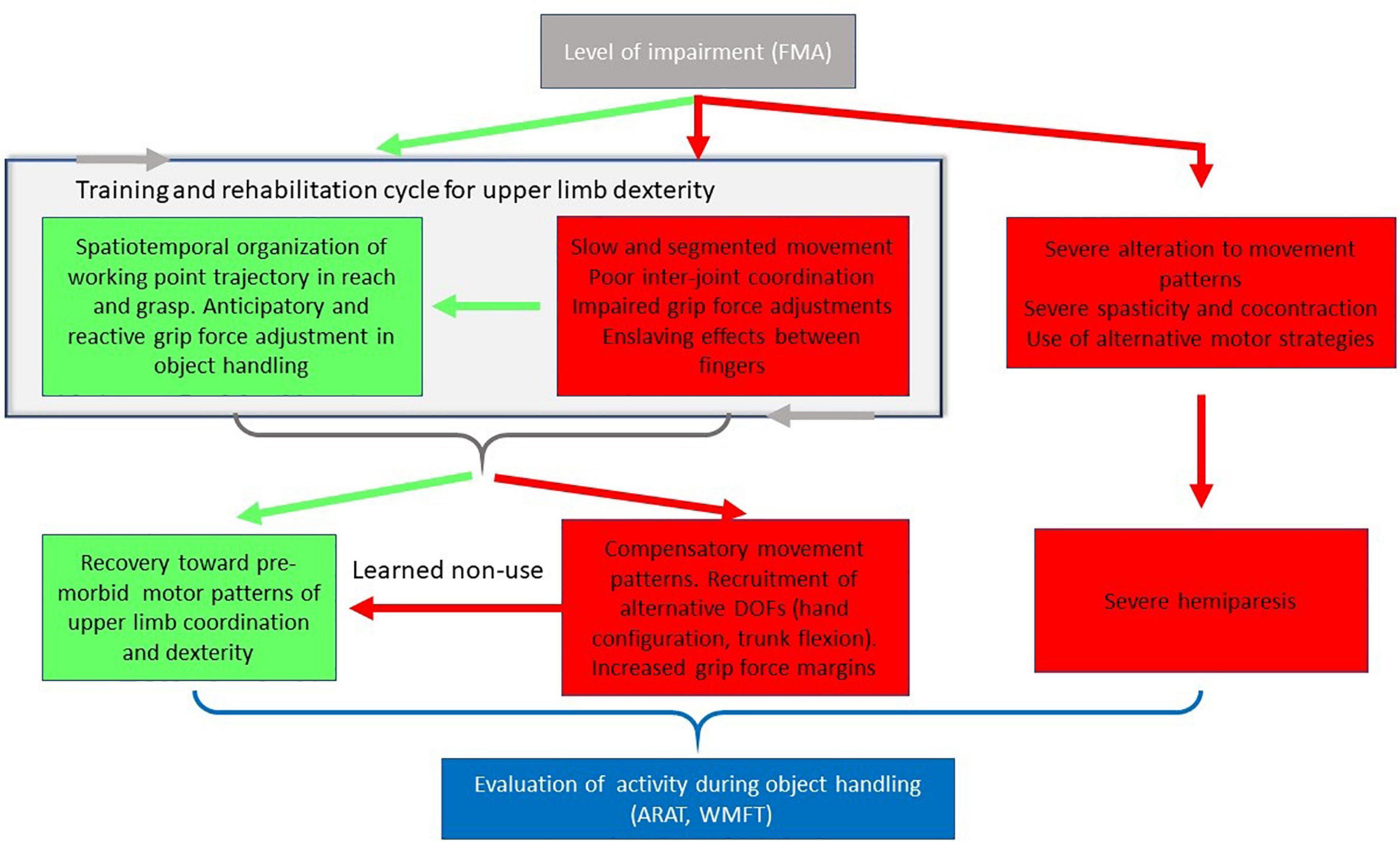
Figure 2. Simplified schema of the consequences of the impairments on movement features. In mild to moderate motor impairments, patients may or may not retain the ability to regulate working point trajectory in reach and grasp, or to effectively adjust grip force during object handling. These spatiotemporal and kinetic parameters evolve through the course of training and rehabilitation (cycle indicated by gray arrows). Inter-joint movement coordination may either progress toward premorbid patterns of motor control (green arrows) or toward compensatory movement patterns involving the recruitment of alternative degrees of freedom to compensate for the impairment (red arrows). Compensatory changes may inhibit progress toward pre-morbid coordination due to the learned non-use phenomenon. In the case of severe impairment, control of spatiotemporal organization of upper limb movement is perturbed, with functional tasks and actions (when they are possible) executed with alternative strategies. Evaluation of activity during object handling may be carried out using clinical tools including the Action Research Arm Test (ARAT) or Wolf Motor Function Test (WMFT).
The most prominent aspect of the CST lesion on kinematics is the duration of upper limb movements, with observable segmentation of the velocity profile (Trombly, 1993; Roby-Brami et al., 1997; van Dokkum et al., 2014; van Kordelaar et al., 2014; Buma et al., 2016). Smoothness metrics, such as the jerk, or spectral arc length, may be used to quantify this particular kinematic feature. This may provide an indication of the level of motor control, or ability to perform efficient movements (Rohrer et al., 2002; Balasubramanian et al., 2015; Buma et al., 2016). Through the course of stroke recovery, reduced smoothness might suggest problems in the central blending of sub-movements (Rohrer et al., 2002). Another hypothesis is that smoothness deficits reflect the suboptimal performance of secondary sensory-motor brain areas (Buma et al., 2016) recruited at the structural level to compensate for the faster and more synchronized CST.
Secondly, the normal flexible shoulder-elbow coordination is disrupted with anomalies in synergistic control at both joint (Levin, 1996; Reisman and Scholz, 2003; Roby-Brami et al., 2003b; Micera et al., 2005) and muscular levels (Beer et al., 2000; Cheung et al., 2012). The normal flexible inter-joint coordination is replaced by stereotypic synergies (Twitchell, 1951; Dewald and Beer, 2001). As a result, hand orientation at the time of grasping is also altered (Roby-Brami et al., 2003b; Sangole and Levin, 2009). It is likely that the disruption of the normally flexible kinematic and muscular synergies, is a direct consequence of the lesion of the primary motor area (Capaday et al., 2013). Abnormal stereotyped synergies are probably due to the reorganization of the neural pathways at the structural level, as described in the Section “Biological Change to Upper Limb Motor Pathways Following Stroke.” Changes in shoulder flexion and elbow extension post stroke limit the upper-limb workspace, and consequently the patient’s reaching abilities (Levin et al., 2002; Reisman and Scholz, 2003; Roby-Brami et al., 2003a). However, hemiparetic patients with mild impairment may retain the ability to use the abundance of DoF to stabilize the trajectory of the hand via automatic compensation of errors between DoF (Reisman and Scholz, 2003). This ability is less flexible than in healthy subjects, particularly when the trunk is involved to reach more distant targets (Reisman and Scholz, 2006).
In effect, coordination between the upper limb and trunk is modified, and stroke patients make excessive use of trunk flexion in forward reaching tasks (Roby-Brami et al., 1997; Cirstea and Levin, 2000; Levin et al., 2002). This is interpreted as a compensatory movement pattern in response to the shortening of the functional length of the limb, arising from impaired elbow extension (Cirstea and Levin, 2000; Roby-Brami et al., 2003a). Indeed, this voluntary control of the trunk remains relatively preserved in most stroke patients due to its bilateral control (Robertson and Roby-Brami, 2011). According to the context, patients may keenly adjust trunk rotation to target direction (Robertson and Roby-Brami, 2011) and to the possible voluntary triggering of pathological synergies (van Kordelaar et al., 2012; Levin et al., 2016). The involvement of the trunk in reaching is interpreted as the spontaneous adaptive use of the redundant and abundant DoF of the body allowing task accomplishment (displace the hand) despite the upper-limb impairment. The use of such compensations may, however, mask a patient’s actual movement abilities. Providing specific instructions to a patient, or use of a mechanical constraint to limit compensatory movement patterns can result in qualitatively better performance. For instance, when the trunk is blocked, a reaching movement within the arm workspace can be successfully executed (at greater effort) with an improved shoulder-elbow coordination (Michaelsen et al., 2001; Levin et al., 2004; Bakhti et al., 2018). Preference for compensatory movement patterns though, do risk becoming highly automated in what is called “learned non-use” (Taub et al., 2006), (Figure 2, also see section “Manipulation and Object Handling”). Once established, these behavioral changes may be difficult to break down, and limit long term clinical outcomes.
Independent Finger Control
The possibility to perform individuated finger control is important for human hand dexterity, with a maximum precision and mobility for the thumb (Marzke and Marzke, 2000). The selectivity of finger control in healthy subjects is not perfect since the action of one finger involuntarily activates others during force production (Zatsiorsky et al., 2000) or kinematic tasks (Teremetz et al., 2015).
Loss of strength and individuated finger control is common following stroke. Generally speaking, both appear to be associated with the integrity of the CST (Wolbrecht et al., 2018; Birchenall et al., 2019). Even after substantial recovery of a pure motor hemiparesis the individuation of finger movements remains limited, particularly in finger abduction (Lang and Schieber, 2003, 2004) due to the lack of selective muscle activation (Schieber et al., 2009; Kamper et al., 2014). Electromyography coupled with grip force measures demonstrate irregular patterns of muscle activation which limit the ability to generate forces and regulate directional control of the fingers and thumb (Cruz et al., 2005; Seo et al., 2010; Triandafilou et al., 2011). Further to this, exaggerated enslaving effects between fingers and the thumb have been observed in studies using both kinematic (Raghavan et al., 2006) and kinetic (Li et al., 2003) measures. This increased involvement of the extra digits is consistent with the fact that stroke patients tend to have relatively important activation of long finger flexors during the generation of fingertip flexion forces compared to healthy control subjects (Cruz et al., 2005).
Nonetheless, individuated finger control appears only weakly correlated with clinical measures of hand dexterity based on grasping actions, suggesting different cortico-spinal control modes of the fingers according to the task (Raghavan et al., 2006).
Hand and Finger Configuration for Grasping
The redundancy of the human hand and fingers with 22 DoF allows to adapt the global hand configuration to the shape of various objects. Principal component analysis of finger joint rotations during grasping and reaching to grasp demonstrated that the DoF were coupled in additively combined synergies: the first component corresponding globally to opening-closing of the hand, with the others contributing to finer adaptation to the shape of the object (Santello et al., 1998). These synergies are progressively formed during reaching, confirming that hand configuration is preshaped as a function of object characteristics (Santello and Soechting, 1998) and task related constraints [reviews in Bicchi et al. (2011) and Santello et al. (2016)]. The curvature of the palmar arch is also preshaped during reaching (Sangole and Levin, 2009). As for proximal upper-limb synergies, hand synergies are probably generated at the cortical level, particularly within the primary motor and somatosensory areas (Leo et al., 2016).
After stroke, the kinematics of the grasping component show impairments and delays in the palmar arch modulation and finger pre-shaping in preparation for grasping (Sangole and Levin, 2009; Tretriluxana et al., 2009). The kinematics of the grasp aperture opening is slow, jerky and less precise with increased delay (Lang et al., 2005; van Kordelaar et al., 2014).
Hand and finger gestures also benefit from compensatory mechanisms. Since performance in precision grip is not completely correlated with the independent control of the fingers, Raghavan et al. (2006) suggested the intervention of compensatory mechanisms within the cerebral sensorimotor networks for grasping actions. This was subsequently demonstrated by a kinematic study of finger joint rotations during reaching to grasp objects with different shapes (concave or convex) (Raghavan et al., 2010). They observed that patients were able to perform different finger coordination to adapt to object shape despite the reduction in finger abduction, PIP (proximal interphalangeal joint) flexion and MCP (metacarpophalangeal joint) extension. The compensatory coordination involved MCP flexion in a later stage of reaching to compensate for the reduction in MCP flexion and to adapt grasp aperture to the shape of the object (Raghavan et al., 2010).
Force Exchanges During Interactions With Objects
A complementary approach for the analysis of manual dexterity is the quantification of force exchanges between the hand and a given object. The physiology of precision grip was pioneered by Johansson and co-workers who used a handheld device equipped with force sensors and an accelerometer to analyze the control of a lifting task (Westling and Johansson, 1984). These, and subsequent studies by other teams demonstrate how grip and load forces increase in parallel prior to lifting the object. In healthy adult subjects, the magnitude of these grip forces is precisely adapted to the anticipated characteristics of both the object (weight, size, shape, and frictional characteristics) and the dynamics of the task. During displacement of the handheld object, grip force is maintained above a safety margin, preventing accidental slippage [reviews in Johansson and Cole (1992) and Flanagan et al. (2009)], and continually adjusted proportional to the load forces associated with the mass and acceleration of that object (Wing, 1996; Hermsdorfer et al., 2003). Each contact event during the performance of the sub-goals of the grasp to lift task is signaled by a distinct sensory event and any perturbing event can be rapidly corrected thanks to short latency cortical loops involving the somatosensory and primary motor areas [reviews in Johansson and Flanagan (2009)].
Hemiparetic patients with moderate disability (Hermsdorfer et al., 2003; Nowak et al., 2003; Quaney et al., 2005; Nowak and Hermsdorfer, 2006) and children with CP (Eliasson et al., 1992; Duque et al., 2003; Bleyenheuft and Thonnard, 2010) tend to exhibit grip force adjustments following the general movement dynamics [review in Bleyenheuft and Gordon (2014)]. This suggests that patients with unilateral brain lesions retain predictive anticipatory motor control in precision grip tasks, which facilitates cyclic movements in particular. During discrete movement (e.g., grasp and place) however, time delays between maximal grip force and load force are often in excess of 200 ms. Temporal irregularities and excessive time delay could be due directly to the CST lesion (Duque et al., 2003) or to delays within transcortical responses. These latter observations suggest that lesions to cerebral sensorimotor networks limit the ability of the patient to reactively modulate grip forces with respect to complex or unexpected movement dynamics (Hermsdorfer et al., 2003).
At the same time, hemiparetic patients typically present with an increased magnitude of grip forces across these different tasks [reviews in Nowak and Hermsdorfer (2009) and Bleyenheuft and Gordon (2014)]. This disruption in grip to load force ratio is similarly observed in healthy individuals immediately following digital anesthesia (Nowak et al., 2001; Monzee et al., 2003). This increased grip force is thus considered to be a highly automated compensation whereby the safety margin is increased to prevent slippage in the context of impaired sensation.
In brief, certain features expressed in most functional movements are likely the direct consequence of the lesion to the CST and/or somatosensory cortex (i) loss of individual finger movement, (ii) general slowness with temporal irregularities and jerkiness, (iii) alterations of the somato-motor reactive control with time delays, (iv) disrupted inter-joint synergies in the upper-limb and the hand. The analysis of hand and finger function in stroke patients illustrate the complex role of the primary motor cortex, both excitatory for the generation of synergies, and inhibitory for the selective activation of muscles. Other pathological features such as abnormal movement synergies are likely due to maladaptive plasticity as described in the Section “Compensation Through Large Scale Vicariance and Neural Plasticity.” Compensation at the level of motor patterns is evidenced by the spontaneous use of extra DoF in the trunk and the finger joints and by the increased level of force to increase the safety margin.
Naturalistic Activity: Compensatory Strategies to Ensure Task Completion
Dexterous upper-limb function is particularly important for the performance of daily activities, which frequently involves the manipulation of objects and tools. The gestures used for naturalistic actions in ecological contexts are more sophisticated than simple prototypic gestures, with a great variability and considerable interindividual differences. When the impairment is too severe and functional sensorimotor adaptations (as described in section “Impairments in Dexterous Coordination of the Upper Limb Post Stroke”) are overwhelmed, a patient may be unable to perform a given action in a habitual or spontaneous manner. In order to carry out said action, they may, however, voluntarily adapt a given motor plan or develop alternative strategies in order to satisfy task objectives. In a simplified and operational way, we shall consider that a motor plan is defined by the trajectory of the working point of the limb. Alternative strategies can thus be executed with alternative working points and/or different trajectories. Consistent with Newell’s ecological framework (Newell, 1986), this can be achieved by adapting the characteristics of the task and/or the disposition of resources in that environment (Figure 3).
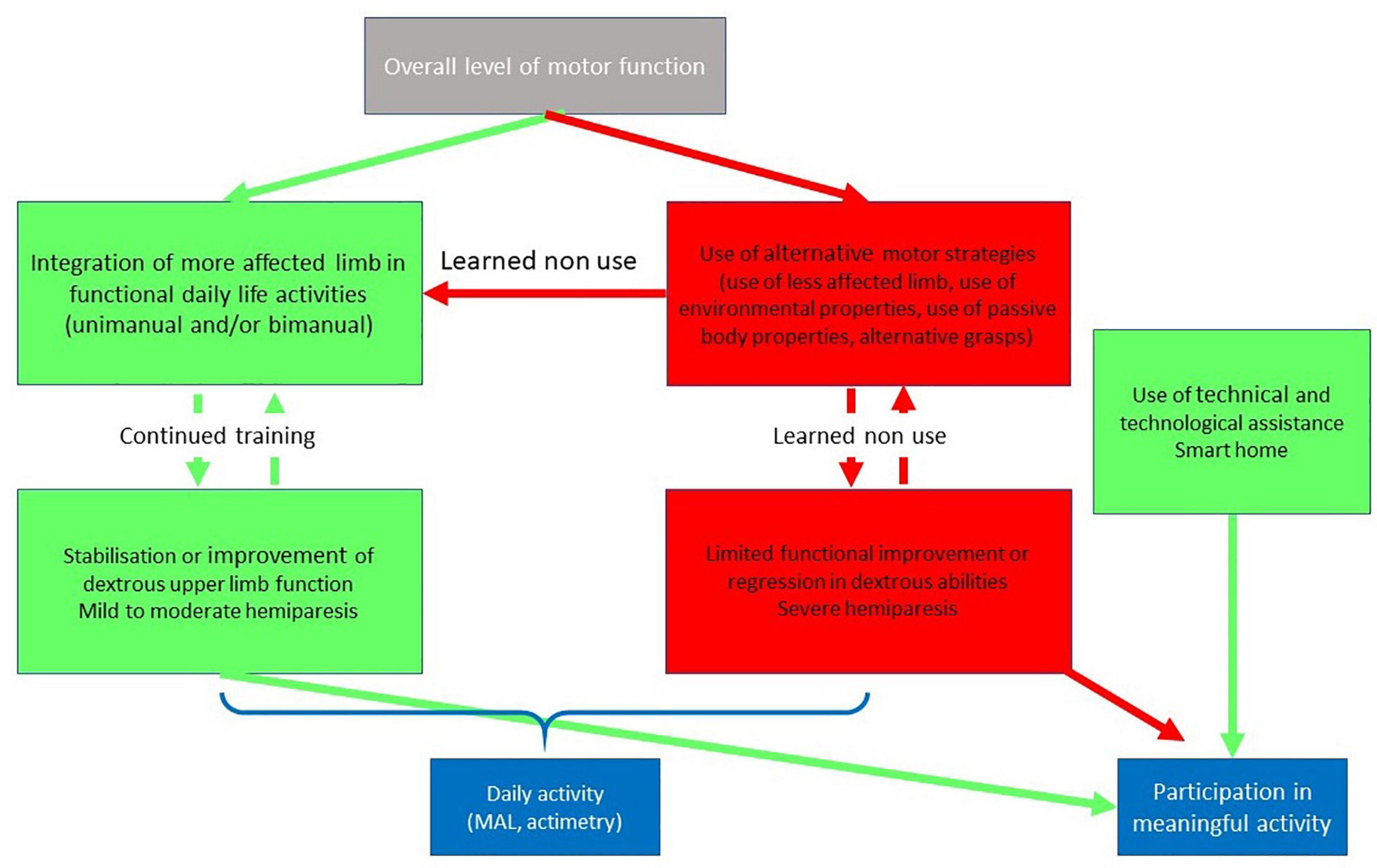
Figure 3. Simplified schema of the relations between the overall level of motor function and daily activity. If the motor function allows the use of the most affected limb in daily life (mild or moderate hemiparesis), the improvement acquired by training is stabilized. If a patient with moderate to severe hemiparesis develops alternatives motor strategies, such as the use of the less affected limb, there is a risk is of functional decline due to the learned-non use phenomenon (i.e., “Use it and improve it or lose it”). Integration of the affected upper limb in functional daily life activities may be measured using questionnaires, such as the Motor Activity Log (MAL), or sensor based actimetry parameters. In case of severe hemiparesis, technical and technological assistance may compensate for upper limb deficits and support participation in meaningful activity.
Task Related Alternative Strategies
The impact of alternative strategies on dexterous upper-limb function will be presented for three representative tasks: unimanual object grasping, manipulation and bimanual activities.
Contrasting Patterns in Reach and Grasp Actions
Analytical studies of hand dexterity focused on precision grip or reach to grasp actions have generally overlooked the great variety of hand configurations adapted to the multiple tasks of everyday life (Bullock et al., 2013). The great flexibility of the human hand to grasp objects (Kapandji, 1980) has been mainly studied using qualitative methods. Napier (Napier, 1956) proposed a dichotomous classification of grasping: “precision grip” and “power grasp.” Later, Iberall et al. (1986) added an intermediate “key grip” and proposed a systematic description of possible finger opposition configurations. This taxonomy of grasping was further developed in the context of anthropomorphic robotics (Feix et al., 2015).
The impairments due to stroke limit the possibilities of action on the environment and, as a consequence, the hemiparetic patient may develop alternative prehension strategies. In order to experimentally investigate this compensation phenomenon, patients must be free to act spontaneously, independent of unnecessary physical constraints or external instruction. Kinematic studies of reach to grasp indicate considerable differences in motor planning. In a study by Roby-Brami et al. (1997), two notable reaching patterns were distinguished. The first involved relatively direct movement of the hand along the sagittal axis toward the object, using the table as a support for the weight of the hand during reaching, this was referred to as a “sliding” strategy. The second pattern observed in this study was characterized by a greater amplitude of vertical movement as patients lifted the hand and descended upon the target object, an action described as “grasping from above” [similar patterns are also observed in tetraplegic patients; (Laffont et al., 2000)]. Importantly, each of these prehensile actions were found to be associated with the severity of hemiparesis, with the “sliding strategy” observed in patients with more proximal weakness and “grasping from above” in those with more distal weakness. These observations illustrate how distinct changes in motor planning emerge in response to the specificity of the motor impairment. Additionally, the ‘sliding strategy’ provides a simple example of how patients may spontaneously exploit features in the environment in order to carry out functional tasks in naturalistic activity.
Significant variation in grasp configuration is also observed in patients post-stroke. When displacing handheld objects, healthy subjects generally use a precision grip or multipulpar grasp including the thumb and a number of fingers according to the size of the object (Cesari and Newell, 1999). Stroke patients, however, appear to use these particular grasp configurations much less frequently when employing objects regularly used in daily life activities (Roby-Brami et al., 1997; Bensmail et al., 2010; Garcia Alvarez et al., 2017). For example, while the majority of healthy subjects used multipulpar grasps to take a spoon, water bottle or ball, the different stroke patients used various combinations of palmar and digito-palmar grasp configurations (Garcia Alvarez et al., 2017). Others still were seen to use a particular “raking” strategy, either with the four fingers and the palm parallel to the table, or with the ulnar aspect of the hand, the palm perpendicular to the table.
Again, the movement variability observed in these works are likely associated with the individual’s impairment and the means by which that person elects to overcome the associated movement limitation. More specifically, we propose that the preservation of precision grasps in some patients might be attributed to less severe lesions of the pyramidal tract or recovery due to cortical plasticity. In contrast, alternative “raking” grasp strategies could be archaic motor acts similar to those of monkeys who lack thumb opposition and have less developed cortico-spinal tracts (Maier et al., 2005). This interpretation is consistent with Jackson’s dissolution concept (York and Steinberg, 1995) and the hierarchical evolutionary organization of dexterity proposed by Bernstein (Bernstein, 1996). Second, we suggest that patients preferentially use standard grasp types if they can. Increased severity of the motor deficit will consequently limit the range of grasp-types possible for that patient, with the ultimate selection of a given hand configuration reflecting the specificity of their impairment (e.g., fine thumb control, spasticity, limitation of the thumb-forefinger opening …).
Finally, we propose that there are causal interactions between impairment and compensation at proximal (shoulder and elbow) and distal (hand and fingers) levels that determine the pose of the hand at the time of grasping. In hemiparetic patients, the hand is oriented more frontally, inclined downward with a variable axial rotation than that which is typical in healthy subjects (Roby-Brami et al., 2003b). Indeed, if a patient uses trunk compensation (flexion and internal axial rotation) or pathological patterns of shoulder and elbow coordination, the hand will be mechanically inclined downward, further constraining potential grasp configurations. Conversely, alternative grasping strategies may impose specific motor planning with atypical hand position and orientation relative to the object and, by consequence, an atypical reaching trajectory inducing deviant kinematic features. These close and complex functional interactions between the proximal and distal parts of the upper-limb may explain that, while cortico-spinal control is mainly distal (Maier et al., 2005), grasping is not more functionally impaired than reaching (Lang et al., 2005). The evolution of these prehensile compensatory strategies may account for the poor correlation between independent finger control and clinical tests of hand function in stroke patients (Raghavan et al., 2006).
Manipulation and Object Handling
In contrast to prehensile tasks aiming at stabilizing the object relative to the hand, manipulation requires the ability to move and rotate the object relative to the body, and supports the use of tools for actions on the environment. Manipulation imposes greater challenges to the sensorimotor system than grasping, including the anticipation of inertial forces and torques in response to variations in the position and orientation of the handheld object (Schneider et al., 2020). In-hand manipulation implies a particularly sophisticated form of manual dexterity where independent finger movements enable the displacement of the object with respect to the hand (Elliott and Connolly, 1984). A classification system has been adopted in order to describe a variety of actions during naturalistic activity in professional or household contexts (Bullock et al., 2013) as well as the use of prosthetic devices by amputees in their homes (Spiers et al., 2017). In daily life activities, performance of object handling tasks is conditioned by the characteristics of the individual and the disposition of the environment. Given the unstructured nature of daily life activity, effective motor solutions may be generated using any number of action sequences and postural configurations. Moreover, certain regularities in grasp transitions (e.g., top grasp to power grip) have been observed in daily life activities (Bullock et al., 2013), suggesting that use of a given hand configuration influences the subsequent prehensile activity. Even for highly repetitive assembly tasks, considerable variability in hand gestures can be observed across different actors (Brunet and Riff, 2009).
Generally speaking, the quantitative analysis of manipulation tasks in stroke patients has received relatively limited attention. Kinematic studies of drinking movements are one exception to this, and recommendations exist on the standardization of this task in order to provide reproducible clinical data (Alt Murphy et al., 2012; Kwakkel et al., 2019). Movement variables examined in these contexts remain, nonetheless, similar to those examined in reaching tasks, including movement duration, hand velocity and smoothness (Alt Murphy et al., 2012). Predictably, stroke patients with poor upper limb function tend to present with segmented velocity profiles and greater total duration for performance of the drinking task (Alt Murphy et al., 2012).
More recently, studies incorporating instrumented objects for measuring force exchanges during tasks involving object rotation have begun to provide additional perspective (Hermsdorfer et al., 2003). Our team designed an instrumented object to be easily manipulated by a person with an upper-limb movement disorder (Jarrassé et al., 2013). This device facilitates the analysis of the sequence of phases involved in object manipulation tasks (Martin-Brevet et al., 2017). Moreover, this method may also serve to highlight micro-errors occurring in action performance at the transitions between sub-goals (Seligman et al., 2014). For example, when using their hemiparetic arm, stroke patients experience greater difficulty with maintaining the vertical orientation of the handheld object, most notably in the transitions to/from a table (i.e. object lifting and object placement) (Parry et al., 2019). In addition, measurable “touch” and “push” errors observed in the form of force variations on lateral load cells prior to establishing grasp as well as increased downward force upon the object following placement. Ongoing research aims at characterizing how stroke patients perform object handling and regulate grip forces during prototypical rotational tasks (e.g., lifting a cup to the mouth, pointing a remote control).
Bimanual Activities
Of course, in everyday life, most activities require some form of bimanual coordination. Moving with cooperative spatiotemporal precision, both hands have differentiated and specialized roles (i.e. the left hand holds and orients an object while the right hand performs an action on it) (Kantak et al., 2017). However, while there is a reasonable body of literature on bimanual organization through infant development (Fagard and Lockman, 2005) and primate evolution (Obhi, 2004), there is a paucity of literature on bimanual cooperative actions during daily life tasks in human adults. Existing studies on the subject have tended to focus most notably on the role of executive functions in movement planning (Gulde et al., 2019).
Despite their clinical interest, bimanual gestures remain largely unexplored in hemiparetic patients (Haaland et al., 2012). It is well known that stroke impairs both sides of the body since the less affected, ipsilesional side, also presents with weakness (Colebatch and Gandevia, 1989) and reduced hand dexterity (Cunha et al., 2017), generally proportional to the severity of the hemiparetic impairment (Maenza et al., 2020). Differential effects upon the ipsilesional hand are observed according to the cerebral hemisphere involved. Most notably, right sided lesions incur problems with visuospatial aspects of coordinated prehensile gestures while left sided lesions have greater effects upon planning and sequencing of the action sequence (Hermsdorfer et al., 1999).
The functional role of the less affected limb of stroke patients is controversial. On one hand, the patients may tend to use their less-affected hand for daily life activity as a compensatory strategy. As stated previously, this preferential use of the less-affected limb may inhibit implication of the hemiparetic counterpart in functional activity and thereby hinder recovery (Taub et al., 2006; Hidaka et al., 2012). It is often assumed that the improvement of the contralesional paretic arm by active rehabilitation will help patients in their (bimanual) daily activities (Johnson et al., 2011). However, some results suggest a limited transfer from unimanual training to bimanual activity (Johnson et al., 2011). In functional assessment of the upper limb during instrumental activities of daily living (IADLs), the use of both arms together favors performance when compared to modal use of the hemiparetic or less affected upper limb (Haaland et al., 2012). As proposed by Haaland et al. (2012) “rehabilitation therapy should focus on the ipsilesional as well as the contralesional arm.”
Taken together, these principles underscore how dexterity after stroke should be broadly understood as a skillful way to perform purposeful actions, either unimanually by using alternative reaching and grasping strategies or bimanually. Beyond the ability to carry out prototypical gestures with the hand and arm, dexterous function is something which engages both upper-limbs and likely the whole body.
Adaptation of the Environment to Support Activity
The ultimate objective of rehabilitation is to favor independence and quality of life for the patients in their living environment, be that in their own home or in a supported care facility. In this context, dexterity does not only represent the gestures of the hand but the individual’s capacity to act on his/her environment. The configuration of the environment may provide affordances that facilitate the behavior, either as hand-held assistive devices or using home automation solutions.
The evaluation of at-home occupational or multidisciplinary interventions is relatively recent [review in Wolf et al. (2015)]. Occupational therapy involves the provision of specialized tools or assistive devices that can support impaired dexterity and improve the functional independence of patients. There exists a variety of low-tech assistive devices (with adapted handles, cuffs, loops, reachers…) for all areas of self-care including dressing, bathing, grooming, cooking, feeding, toileting etc. In addition, readily accessible technology in contemporary home environments may serve as a mediator of actions. Interestingly, recent developments propose technological solutions integrating embedded sensors in various devices, and at various locations in domestic environments to guide and assist daily living activities of patients with diverse neuropsychological impairments (Worthington, 2016; Baber et al., 2017). Smart home systems have a large potential to compensate for limitations in dexterous upper limb function, promote participation and improve quality of life. However, a recent review pinpoints the lack of high-quality evidence supporting the use of such devices. Further to this, ethical concerns (e.g., privacy) and the importance of human contact in personal support packages remain important considerations (Jamwal et al., 2020). Specific methods are needed to better understand how patients with limited dexterity cope with common household tasks. Recent progress in sensor technology, particularly accelerometry, combined with novel signal processing methods are quite promising but remain less developed for applications in upper-limb movement analysis than for the lower limb or gait [review in Dobkin (2017)]. Accelerometry methods have been used to quantify the contribution of both upper-limbs to activity for each time unit during several hours (Bailey et al., 2015). They confirm the expected asymmetry due to hemiparesis and can document non-use of the affected side during bimanual activities (Michielsen et al., 2012). Thus, accelerometry methods afford particularly interesting possibilities for quantifying the effects of rehabilitation techniques on both limbs in ecological contexts (Wang et al., 2017), providing meaningful data on patient activity, which might be complementary to established clinical evaluation techniques (Bailey et al., 2015). However, further progress on the spatiotemporal analysis of gestures using wearable sensors is needed to improve understanding of this link between impairments, functional capacities and task performance in ecological context thanks to behavioral or technological compensations.
Clinical Perspectives
Better Understanding Each Individual Patient’s Dexterity
As described above, hand dexterity after stroke is multifactorial since there is no linear causality between the severity of the brain lesion, in particular that of the CST, and the functional independence of the person. The explanation is likely the possibility of compensation at several integrative levels (Levin et al., 2009). Biological compensation is achieved by plasticity and vicariance at the risk of maladaptive phenomena. Functional sensorimotor compensation is achieved by tuning spatiotemporal motor patterns thanks to kinematic redundancy across the different segments of the body (trunk, upper-limb, hand and fingers) and adaptation of grip forces (adjusted safety margins) (per the training and rehabilitation cycle indicated in Figure 2). As a consequence, patients with moderate recovery from stroke may execute motor plans roughly similar to those of healthy controls (i.e. with similar end-point trajectory) despite the impairment of the fine sensorimotor control. Compensation during naturalistic activity is achieved through the planning and execution of alternative motor strategies according to the context and the environment. The most prominent strategy is the exclusive use of the less affected limb; but patients also frequently use specific reaching and grasping strategies during unimanual or bimanual activities, with or without the assistance of technical devices. Compensation at any given level may have complex reciprocal influences upon any other level, leading to the emergence of a wide variety of upper limb motor behaviors post stroke.
Various clinical evaluation tools are available for testing (Santisteban et al., 2016; Villepinte et al., 2020). However, scores obtained through clinical tests of upper limb function do not precisely distinguish the pathophysiological link between the consequences of brain lesions and the multi-level compensatory mechanisms. Based upon the review presented here, we reiterate previous calls for the development of kinetic and kinematic methods in the clinical settings which may complement clinical scores (Nowak, 2008; Winstein and Varghese, 2018; Kwakkel et al., 2019). At this point though, quantitative analyses of upper limb motor behavior in clinical settings are generally based on highly constrained movement tasks, adapted from those used in experiments on healthy individuals. We advocate further studies to quantify more naturalistic tasks similar to daily life tasks, including manipulation of objects and tools as well as pertinent bimanual activities. The short-term objective would be to distinguish movement characteristics directly consequent to brain lesion from those which emerge through compensation so as to envisage more personalized approaches to patient rehabilitation.
Technology for Assessment and Rehabilitation at Home
In effect, quantitative movement analysis of prototypical gestures in clinical settings may be effective for evaluating functional capacity of the upper limb but provide comparatively less information regarding dexterous use of the hand as it pertains to participation in ecological situations. Activity and independence might conversely be investigated in the patient’s natural environment (home or supported care) where daily life gestures would be facilitated (or impeded) by the physical organization and supports available in those surrounds. The increasing sophistication of technological aids (sensors, wearable devices, and home automation) available for use in the home represent an important means for expanding knowledge on patient movements and strategies and for prolonged rehabilitation in the home (Dobkin, 2017; Maceira-Elvira et al., 2019; Jones et al., 2020). While the majority of consumer wearables are focused are focused on tracking some vital signs (heart rhythms and body temperature) or global activity level, extensive research is currently being conducted on the development of wearable systems relying on different technologies (accelerometers, Inertial Measurement Units-IMUs, wearable robotics or EMG sensors) for monitoring and providing feedback on upper body posture and upper-limbs movements (Wang et al., 2017). However, instrumented clothing designed to monitor activity in daily life is not yet a reality (Maceira-Elvira et al., 2019). Effectively measuring the highly precise movements of the hand using wearables also remains a complex challenge (Lin et al., 2017), with instrumented gloves being complex to install and calibrate (particularly on paretic hands of stroke patients). The availability of relatively inexpensive motion capture systems relying on depth or stereoscopic cameras and without the necessity of worn markers (e.g., Kinect® and Leap Motion®) (Guzsvinecz et al., 2019) may provide new avenues for kinematic analysis of hand function. As shown earlier, the characterization of interaction forces by instrumented objects may be a key to the comprehensive examination of manual dexterity and its recovery. In addition, they may be used as “smart toys” for rehabilitation exercises (Hussain et al., 2015; Borghese et al., 2019). Wearable EMG sensors could also estimate underlying muscular activations in post stroke patients (Mendez et al., 2017). Wearable solutions as reviewed above can be used to provide feedback to the patient to assist and encourage home based rehabilitation exercises. Some commercial products exist, such as the Armeo®Senso by Hocoma, which relies on a set of worn IMUs and a visual interface to guide patients during rehabilitation. Using embedded sensors in smartphones to track upper limb movement could also be a simple, accessible solution to simplify and generalize assessment and monitored home rehabilitation (Ferreira et al., 2014).
Finally, while a growing number of measurement solutions are becoming accessible to assess dexterity, standardized approaches to processing the complex multidimensional datasets which they produce will need to be consolidated (Appelboom et al., 2014). Automated processing and flexible visual analysis tools are essential in order to extract meaningful information which the clinician may use to inform therapeutic interventions.
Therapeutic Indications
The choice of a rehabilitation intervention is based on an overall clinical evaluation of the individual patient, with complementary analysis provided through brain imaging and functional tests. The distinction between recovery and compensation is nonetheless crucial to the matrix of clinical decision making for rehabilitation. The atypical compensatory motor pattern used by the patient may represent a viable adaptation given their physical capacities with respect to the environmental constraints (Latash and Anson, 1996). However, compensatory patterns can inhibit recovery of normal motor behavior due to learned non-use phenomena (Hidaka et al., 2012), or worse, exacerbate physical deformity (e.g., contractures or orthopedic complications). It is thus important to consider individual movement patterns in order to decide whether the therapeutic intervention should limit compensations and attempt to improve the impairment (true recovery), or to proceed with training compensatory patterns with the objective of improving movement safety, supporting functional independence in ADLs and promoting social participation. These options are not necessarily compatible given that therapies which pursue true motor recovery can be quite demanding, necessitating strong motivation over prolonged periods of time (typically several weeks or months). In contrast, favoring compensation may lead to a more immediate benefit. However, such processes are complex to analyze due to (i) intricate causal chains between the state of brain structures, upper-limb function and activity routines, and; (ii) functional discrepancies between behavior (learning) and neurobiology (plasticity). The aim of this section is to underline several crucial elements which might be considered when planning rehabilitation for chronic stroke patients rather than to review rehabilitation methods.
Promoting Recovery by Exercise
Ideally, therapies should induce recovery at the level of the brain via cortical networks, once the extent of the lesion is stabilized following the acute period. Many promising neurobiologically inspired interventions have been proposed (non-invasive brain stimulation and neuro-technologies) but are yet to demonstrate their effectiveness in routine clinical practice (Sandrini and Cohen, 2013; Regenhardt et al., 2020). Regardless, activity-dependent neural plasticity remains the cornerstone of contemporary advances in rehabilitation practice (Nudo et al., 1996). As demonstrated by Nudo et al. (1996), following a lesion to the M1 cortical representation of the hand in monkeys, recovery of dexterous upper limb function occurred only among those monkeys who completed functional exercise by grasping food in feeding activities. The dimensions of the cortical representation for monkeys with no specific training program (spontaneous recovery) were found to diminish. This contraction of cortical maps supports the behavioral concept of learned non-use. Structural plasticity (synaptogenesis, axonal growth and branching) in regions proximal to the lesion (premotor area) was later demonstrated in animal studies (Dancause, 2006). Nudo’s observations are accepted as proof that plasticity of the cortical map is the neurobiological basis of true recovery. However, a video analysis of the same monkeys in a complementary article showed that some of them, in fact, used alternative grasping strategies (Friel and Nudo, 1998). In stroke patients, recovery through the subacute period is associated with changes in brain excitability, functional plasticity of cortical maps, and changes in connectivity in both hemispheres [review in Loubinoux et al. (2017)]. But the clinical consequences of these processes still remain unclear since functional neural plasticity does not necessarily lead to behavioral recovery (Buma et al., 2016; Peters et al., 2017). Structural plasticity probably contributes to improvements during long term rehabilitation but the relationships between the physical intervention and structural plasticity, and between structural plasticity and clinical outcome are still unclear [review in Sampaio-Baptista et al. (2018)].
In healthy subjects, the improvement of performance with repetition during sensory-motor learning is composed of two processes, occurring at different time scales: adaptation and skill learning (Kitago and Krakauer, 2013). Adaptation corresponds to the tuning of sensory-motor parameters to the actual situation after a relatively small number of repetitions; it relies on functional excitability changes and plasticity in the brain and cerebellum sensory-motor networks. Skill learning requires long-term practice, typically in a professional, sporting or artistic context, and can lead to structural changes in the central nervous system, as demonstrated in healthy subjects (Sampaio-Baptista et al., 2018). Contemporary rehabilitation methods inspired by motor learning paradigms are mostly based on the active repetition of meaningful movements, in contrast to classical neuro-developmental methods. The practice should be task specific, goal oriented, and motivating, with intense well-structured practice and provision of adequate sensory-motor feedback (Krakauer, 2006; Maier et al., 2019). Sensory-motor learning is often assisted by technology (e.g., virtual reality, robotics, and adapted video games) to provide more precise and standardized exercises and increased motivation thanks to engaging game design and user experience. However, it is still unclear if patients can truly recover after the acute, 3 month period of spontaneous recovery. Recent Cochrane meta-analyses showed that robotics and electromechanical devices could improve ADL, arm function and strength (Mehrholz et al., 2018) while the benefit of virtual reality was less convincing but significant when used in addition to standard care (Laver et al., 2017). Both studies underline the difficulties involved in evaluating the efficiency of these methods, as considerable variation is observed across interventions from one trial to another, and between the characteristics of the control intervention (in particular, “usual care,” which is still a “black box,” or matched intensity exercises).
Can the Patient Truly Recover Pre-morbid Motor Function?
The possibility of recovery at the impairment level is particularly debated. Negative findings could be due to a dose-effect (Winstein et al., 2019). The cumulated duration of training during usual trials is relatively low [18–36 h according to Ward et al. (2019)] and increasing the dosage up to 60 h improved the MAL but not function (WMFT) (Winstein et al., 2019). A recent study used a particularly intense schedule (300 h in 60 sessions with 5 h/day training) to compare three rehabilitation methods (robotics, functional electrical stimulation, and motor learning). The authors observed some significant and clinically relevant improvement at both impairment (Fugl-Meyer) and activity level, irrespective of the method used. Accordingly, a recent, non-controlled, retrospective study suggested that particularly intensive and prolonged therapies in stroke patients could lead to some improvement in the Fugl-Meyer score (Ward et al., 2019). Other studies suggest that training based on individual finger movements could induce some improvements that generalized to patient performance on Fugl-Meyer, hand function (ARAT) and activity (MAL) testing (Mawase et al., 2020). Similar approaches using highly specific hand training tasks (aiming, tapping, turning…) have also been found to improve manual dexterity when evaluated using the Fugl-Meyer test and hand activity test (TEMPA, Test d’Evaluation des Membres Supérieurs de Personnes âgées) (Platz et al., 2009; Platz and Lotze, 2018).
What Is the Behavioral Effect of Constraint Induced Movement Therapy?
The most studied method to limit compensation is Constraint Induced Movement Therapy (CIMT) and its modified derivatives. The principle, proposed by Taub, is to impede the use of the less affected limb while soliciting the most affected limb with an intensive training program (Taub et al., 1999). This method is indicated only to patients who have already attained a certain functional threshold (clinically determined by taking into consideration factors such as partial recovery of wrist and finger extension) but has nonetheless provided a source of much hope (Sirtori et al., 2009). However, a recent meta-analysis of CIMT in chronic stroke patients reported “limited improvements in motor impairment and motor function, but that these benefits did not convincingly reduce disability” (Corbetta et al., 2015). In particular, a kinematic study showed no improvement in coordination during a 2D pointing task (Kitago et al., 2013). These inconsistencies could be due to individual differences, with some patients improving enough to use their limb, while others regressing following conclusion of the training period (Han et al., 2008). Improvement in daily life activity and self-reported arm use after constraint induced therapy could be attributed to the learning of new behavioral compensations, possibly involving both limbs and not to the recovery of the impairment [review in Kwakkel et al. (2015)].
Movement Quality Is Important
When the use of the less affected limb is blocked during CIMT, people can still use compensatory motor patterns based on body redundancy, in particular the participation of the trunk. This is the equally true of conventional rehabilitation exercises, when the success of the task is only based on the displacement of the end-point. In contrast to classical rehabilitation methods such as Bobath (Levin and Panturin, 2011), these methods based on goal success seldom consider the quality of movement performance. As described in Section “Kinematics of Reach to Grasp Movements,” stroke patients may preferentially involve the trunk instead of exploiting shoulder and elbow rotation to displace the hand toward the target. The involvement of the trunk may be detrimental by inducing non-use phenomena of the upper-limb. Indeed, the limitation of trunk compensation by a physical restraint can improve shoulder-elbow coordination (Michaelsen et al., 2001). Limiting trunk compensation can thus “unmask latent potential recovery of upper extremity movement” (Wee et al., 2014). Training better movement coordination increases the efficiency of training as shown by the initial study (Michaelsen et al., 2006) and a meta-analysis which showed a moderate effect on the impairment and on the kinematics (Wee et al., 2014). These studies show that the quality of movement coordination during training is important and should be closely controlled either by trunk restraint, by Knowledge of Performance (KP) feedback given by the therapist (Cirstea and Levin, 2007) or by specific technology assisted KP, for example auditory feedback (Chen et al., 2016).
Task Related Skill Training
Some rehabilitation methods based on skill training aim at improving daily life activity, rather than reducing impairment through repeated movements (Winstein et al., 2016b). Rehabilitative task-oriented training can induce dosage-dependent improvements in reported motor activity (MAL) (Winstein et al., 2019). However, the effect of skill training is controversial since the interventions are difficult to systematize, and meta-analyses have shown only modest effects (French et al., 2010; Timmermans et al., 2010).
One difficulty is that very few studies have addressed the training of bimanual tasks, which are essential for daily life. A pilot study suggested that intensive training of the ipsilesional, less-affected, limb could improve its dexterity (Jebsen test) and could generalize to functional independence (Sainburg et al., 2016). Several studies developed symmetrical bilateral arm training with the perspective of assisting the paretic limb by the less affected limb thanks to interlimb coupling (Whitall et al., 2011), however, a meta-analysis did not show any clear neural or behavioral effects (Choo et al., 2015). Surprisingly, there are very few studies of bimanual rehabilitation methods with more functional, asymmetric manipulative tasks in hemiparetic patients despite their relevance for daily life activity. A better understanding of the use of both hands during manipulative actions is needed, particularly the effect of laterality and handedness.
Individual behavior during ADL according to the mantra “use it and improve it or lose it” is probably a key to better understanding of why some patients above a certain functional threshold continue to improve during follow-up while other regress (Hidaka et al., 2012).
Conclusion
Interpreting functional movements such as prehension or manipulation in pathological populations implies an inherent dilemma since they represent both the consequences of the lesion in association with the measures taken to overcome those limits. Movements are not pathological in and of themself; but simply the vehicle for intended actions. Broadly speaking, patients with mild to moderate hemiparesis retain the global spatial organization of prehensile movement in the context of goal directed reaching, multi-finger actions during grasp and grip-load force coordination. Nonetheless, the neurological effects of the cerebrovascular accident bring about various irregularities in upper limb kinematics and dexterous control of the hand. These different features may be related to different underlying processes. Certain components of the movement may be directly related to the CST lesion, in particular alteration of the more refined individual finger control and precision grip. While other movement irregularities would be associated with mechanisms implicated in the reorganization of the nervous system (hyperexcitability, plasticity, and vicariance) and of body coordination (e.g., use of body redundancy and setting of force level). In addition to this, alternate strategies of voluntary movement emerge as the patient exploits the abundant motor solutions offered across the brain-body-environment system, leading to behavioral changes in upper limb activities.
While clinical observations attest to the importance of multidisciplinary physical rehabilitation, the precise ingredients required to stimulate and optimize the recovery of upper limb function and manual dexterity post stroke remain elusive. Available international guidelines enumerate recommended rehabilitation methods (e.g., Winstein et al., 2016a). However, as recently pointed out by Bernhardt et al. (2019) “Clinical trials and observational studies have so far failed to distinguish behavioral restitution from behavioral substitution, leaving the association between quality of movement and recovery of upper limb capacity underexplored.” Many studies lack a precise description of the intervention and of the resulting motor behavior. The principles of motor learning involved should be completely described (Maier et al., 2019) with precise dosage, repetition, duration and intensity (Timmermans et al., 2010). The objective of the methods in terms or recovery/compensation at the different levels (brain networks, body function, activity, and participation) should be clarified to facilitate the interpretation of their pathophysiological effects. To this end, standardized instrumented evaluations should be recommended to measure the quality of movements and to distinguish recovery and compensation (Kwakkel et al., 2019; Alouche et al., 2020).
Meta-analyses generally show disappointing negative results but often underline the great heterogeneity of the interventions under review, for example virtual reality or robotics. Randomized controlled trials are the golden standard of evidence-based medicine. However, it is impossible to evaluate separately all the “rehabilitation ingredients” constituting a given intervention without a combinatorial explosion. In addition, it is difficult to account for individual variability beyond simple stratification. A current reflection examines alternative possibilities of “practice-based evidence research” intermediate between randomized controlled trials and retrospective clinical observations (Horn et al., 2012). Future trials could also be inspired by statistical protocols used for precision individualized care (Janiaud et al., 2019).
Many technologies, wearable or not (neurotechnologies, virtual reality, robotics, games, telerehabilitation etc.) are being developed in order to increase the intensity and duration of therapeutic activities in the home environment (Dobkin, 2017; Maceira-Elvira et al., 2019; Jones et al., 2020). Whilst the description of technology assisted rehabilitation methods is beyond the scope of the present article, it is worthy to note that continued fitness exercises as part of home rehabilitation programs are crucial for maintaining and improving motor functioning of both upper limbs in the long term (Ward et al., 2019). Moreover, if a person has reached a sufficient functional level of dexterity to use his/her limb during daily life activities, he/she will have greater opportunities to improve further (Han et al., 2008). This is especially true if the most affected limb is regularly engaged in bimanual actions. There is probably a virtuous circle between prolonged home or community-based rehabilitation and activities of daily life.
Author Contributions
AR-B wrote the first draft of the manuscript. RP and NJ wrote sections of the manuscript. All authors contributed to manuscript revision, read, and approved the submitted version.
Funding
This work was performed within the laboratory of Excellence SMART supported by French state funds managed by the ANR within the “Investissements d’Avenir” program under reference ANR-11-IDEX-0004–02.
Conflict of Interest
The authors declare that the research was conducted in the absence of any commercial or financial relationships that could be construed as a potential conflict of interest.
References
Abend, W., Bizzi, E., and Morasso, P. (1982). Human arm trajectory formation. Brain 105(Pt 2), 331–348.
Alouche, S. R., Molad, R., Demers, M., and Levin, M. F. (2020). Development of a comprehensive outcome measure for motor coordination; Step 1: three-phase content validity process. Neurorehabil. Neural Repair 35, 185–193. doi: 10.1177/1545968320981955
Alstermark, B., and Isa, T. (2012). Circuits for skilled reaching and grasping. Annu. Rev. Neurosci. 35, 559–578. doi: 10.1146/annurev-neuro-062111-150527
Alt Murphy, M., Resteghini, C., Feys, P., and Lamers, I. (2015). An overview of systematic reviews on upper extremity outcome measures after stroke. BMC Neurol. 15:29. doi: 10.1186/s12883-015-0292-6
Alt Murphy, M., Willen, C., and Sunnerhagen, K. S. (2012). Movement kinematics during a drinking task are associated with the activity capacity level after stroke. Neurorehabil. Neural Repair 26, 1106–1115. doi: 10.1177/1545968312448234
Appelboom, G., Yang, A. H., Christophe, B. R., Bruce, E. M., Slomian, J., Bruyere, O., et al. (2014). The promise of wearable activity sensors to define patient recovery. J. Clin. Neurosci. 21, 1089–1093. doi: 10.1016/j.jocn.2013.12.003
Asanuma, H., and Arissian, K. (1984). Experiments on functional role of peripheral input to motor cortex during voluntary movements in the monkey. J. Neurophysiol. 52, 212–227. doi: 10.1152/jn.1984.52.2.212
Baber, C., Khattab, A., Russell, M., Hermsdorfer, J., and Wing, A. (2017). Creating affording situations: coaching through animate objects. Sensors 17:2308. doi: 10.3390/s17102308
Bailey, R. R., Klaesner, J. W., and Lang, C. E. (2015). Quantifying real-world upper-limb activity in nondisabled adults and adults with chronic stroke. Neurorehabil. Neural Repair 29, 969–978. doi: 10.1177/1545968315583720
Baker, S. N., Zaaimi, B., Fisher, K. M., Edgley, S. A., and Soteropoulos, D. S. (2015). Pathways mediating functional recovery. Prog. Brain Res. 218, 389–412. doi: 10.1016/bs.pbr.2014.12.010
Bakhti, K. K. A., Laffont, I., Muthalib, M., Froger, J., and Mottet, D. (2018). Kinect-based assessment of proximal arm non-use after a stroke. J. Neuroeng. Rehabil. 15:104. doi: 10.1186/s12984-018-0451-2
Balasubramanian, S., Melendez-Calderon, A., Roby-Brami, A., and Burdet, E. (2015). On the analysis of movement smoothness. J Neuroeng. Rehabil. 12:112. doi: 10.1186/s12984-015-0090-9
Battaglia-Mayer, A., Caminiti, R., Lacquaniti, F., and Zago, M. (2003). Multiple levels of representation of reaching in the parieto-frontal network. Cereb. Cortex 13, 1009–1022.
Beer, R. F., Dewald, J. P., and Rymer, W. Z. (2000). Deficits in the coordination of multijoint arm movements in patients with hemiparesis: evidence for disturbed control of limb dynamics. Exp. Brain Res. 131, 305–319.
Belhaj-Saif, A., and Cheney, P. D. (2000). Plasticity in the distribution of the red nucleus output to forearm muscles after unilateral lesions of the pyramidal tract. J. Neurophysiol. 83, 3147–3153. doi: 10.1152/jn.2000.83.5.3147
Bensmail, D., Robertson, J., Fermanian, C., and Roby-Brami, A. (2010). Botulinum toxin to treat upper-limb spasticity in hemiparetic patients: grasp strategies and kinematics of reach-to-grasp movements. Neurorehabil. Neural Repair 24, 141–151. doi: 10.1177/1545968309347683
Bernhardt, J., Borschmann, K. N., Kwakkel, G., Burridge, J. H., Eng, J. J., Walker, M. F., et al. (2019). Setting the scene for the second stroke recovery and rehabilitation roundtable. Int. J. Stroke 14, 450–456. doi: 10.1177/1747493019851287
Bicchi, A., Gabiccini, M., and Santello, M. (2011). Modelling natural and artificial hands with synergies. Philos. Trans. R. Soc. Lond. B Biol. Sci. 366, 3153–3161. doi: 10.1098/rstb.2011.0152
Birchenall, J., Teremetz, M., Roca, P., Lamy, J. C., Oppenheim, C., Maier, M. A., et al. (2019). Individual recovery profiles of manual dexterity, and relation to corticospinal lesion load and excitability after stroke -a longitudinal pilot study. Neurophysiol. Clin. 49, 149–164. doi: 10.1016/j.neucli.2018.10.065
Bizzi, E., Cheung, V. C., d’Avella, A., Saltiel, P., and Tresch, M. (2008). Combining modules for movement. Brain Res. Rev. 57, 125–133. doi: 10.1016/j.brainresrev.2007.08.004
Bleyenheuft, Y., and Gordon, A. M. (2014). Precision grip in congenital and acquired hemiparesis: similarities in impairments and implications for neurorehabilitation. Front. Hum. Neurosci. 8:459. doi: 10.3389/fnhum.2014.00459
Bleyenheuft, Y., and Thonnard, J. L. (2010). Predictive and reactive control of precision grip in children with congenital hemiplegia. Neurorehabil. Neural Repair 24, 318–327. doi: 10.1177/1545968309353327
Borghese, N. A., Essenziale, J., Mainetti, R., Mancon, E., Pagliaro, R., and Pajardi, G. (2019). Hand rehabilitation and telemonitoring through smart toys. Sensors 19:5517. doi: 10.3390/s19245517
Bril, B. (2015). “Learning to use tools: a functional approach to action,” in Francophone Perspectives of Learning Through Work: Conceptions, Traditions and Practices, eds L. Filliettaz and S. Billett (Cham: Springer International Publishing), 95–118.
Brunet, M., and Riff, J. (2009). Analyse et exploitation de la variabilité gestuelle dans la prévention des TMS. PISTES Perspectives interdisciplinaires sur le travail et la santé 11, 1–11. doi: 10.4000/pistes.2270
Brunnstrom, S. (1970). Movement Therapy in Hemiplegia: A Neurophysiological Approach. New York, NY: Harper & Row.
Buetefisch, C. M., Revill, K. P., Haut, M. W., Kowalski, G. M., Wischnewski, M., Pifer, M., et al. (2018). Abnormally reduced primary motor cortex output is related to impaired hand function in chronic stroke. J. Neurophysiol. 120, 1680–1694. doi: 10.1152/jn.00715.2017
Bullock, I. M., Zheng, J. Z., De La Rosa, S., Guertler, C., and Dollar, A. M. (2013). Grasp frequency and usage in daily household and machine shop tasks. IEEE Trans. Haptics 6, 296–308. doi: 10.1109/TOH.2013.6
Buma, F. E., van Kordelaar, J., Raemaekers, M., van Wegen, E. E. H., Ramsey, N. F., and Kwakkel, G. (2016). Brain activation is related to smoothness of upper limb movements after stroke. Exp. Brain Res. 234, 2077–2089. doi: 10.1007/s00221-015-4538-8
Bundy, D. T., and Nudo, R. J. (2019). Preclinical studies of neuroplasticity following experimental brain injury. Stroke 50, 2626–2633. doi: 10.1161/STROKEAHA.119.023550
Burke, D., Wissel, J., and Donnan, G. A. (2013). Pathophysiology of spasticity in stroke. Neurology 80(3 Suppl. 2), S20–S26. doi: 10.1212/WNL.0b013e31827624a7
Byblow, W. D., Stinear, C. M., Barber, P. A., Petoe, M. A., and Ackerley, S. J. (2015). Proportional recovery after stroke depends on corticomotor integrity. Ann. Neurol. 78, 848–859. doi: 10.1002/ana.24472
Capaday, C., Ethier, C., Van Vreeswijk, C., and Darling, W. G. (2013). On the functional organization and operational principles of the motor cortex. Front. Neural Circuits 7:66. doi: 10.3389/fncir.2013.00066
Causby, R., Reed, L., McDonnell, M., and Hillier, S. (2014). Use of objective psychomotor tests in health professionals. Percept. Mot. Skills 118, 765–804. doi: 10.2466/25.27.PMS.118k27w2
Cesari, P., and Newell, K. M. (1999). The scaling of human grip configurations. J. Exp. Psychol. Hum. Percept. Perform. 25, 927–935.
Chen, J. L., Fujii, S., and Schlaug, G. (2016). The use of augmented auditory feedback to improve arm reaching in stroke: a case series. Disabil. Rehabil. 38, 1115–1124. doi: 10.3109/09638288.2015.1076530
Cheung, V. C., Turolla, A., Agostini, M., Silvoni, S., Bennis, C., Kasi, P., et al. (2012). Muscle synergy patterns as physiological markers of motor cortical damage. Proc. Natl. Acad. Sci. U.S.A. 109, 14652–14656. doi: 10.1073/pnas.1212056109
Choo, P. L., Gallagher, H. L., Morris, J., Pomeroy, V. M., and van Wijck, F. (2015). Correlations between arm motor behavior and brain function following bilateral arm training after stroke: a systematic review. Brain Behav. 5:e00411. doi: 10.1002/brb3.411
Choudhury, S., Shobhana, A., Singh, R., Sen, D., Anand, S. S., Shubham, S., et al. (2019). The relationship between enhanced reticulospinal outflow and upper limb function in chronic stroke patients. Neurorehabil. Neural Repair 33, 375–383. doi: 10.1177/1545968319836233
Cirstea, M. C., and Levin, M. F. (2000). Compensatory strategies for reaching in stroke. Brain 123, 940–953.
Cirstea, M. C., and Levin, M. F. (2007). Improvement of arm movement patterns and endpoint control depends on type of feedback during practice in stroke survivors. Neurorehabil. Neural Repair 21, 398–411.
Cochet, H., and Byrne, R. W. (2013). Evolutionary origins of human handedness: evaluating contrasting hypotheses. Anim. Cogn. 16, 531–542. doi: 10.1007/s10071-013-0626-y
Colebatch, J. G., and Gandevia, S. C. (1989). The distribution of muscular weakness in upper motor neuron lesions affecting the arm. Brain 112, 749–763.
Corbetta, D., Sirtori, V., Castellini, G., Moja, L., and Gatti, R. (2015). Constraint-induced movement therapy for upper extremities in people with stroke. Cochrane Database Syst. Rev. 2015:CD004433. doi: 10.1002/14651858.CD004433.pub3
Cruz, E. G., Waldinger, H. C., and Kamper, D. G. (2005). Kinetic and kinematic workspaces of the index finger following stroke. Brain 128(Pt 5), 1112–1121.
Cunha, B. P., de Freitas, S., and de Freitas, P. B. (2017). Assessment of the Ipsilesional hand function in stroke survivors: the effect of lesion side. J. Stroke Cerebrovasc. Dis. 26, 1615–1621. doi: 10.1016/j.jstrokecerebrovasdis.2017.02.023
Dancause, N. (2006). Vicarious function of remote cortex following stroke: recent evidence from human and animal studies. Neuroscientist 12, 489–499.
d’Avella, A., and Bizzi, E. (2005). Shared and specific muscle synergies in natural motor behaviors. Proc. Natl. Acad. Sci. U.S.A. 102, 3076–3081.
Dejerine, J. (1914). “Troubles de la motilité,” in Séméiologie des Affections du Système Nerveux (Paris: Masson), 167–392.
Desmurget, M., and Prablanc, C. (1997). Postural control of three-dimensional prehension movements. J. Neurophysiol. 77, 452–464.
Desrosiers, J., Bourbonnais, D., Bravo, G., Roy, P. M., and Guay, M. (1996). Performance of the ‘unaffected’ upper extremity of elderly stroke patients. Stroke 27, 1564–1570.
Dewald, J. P., and Beer, R. F. (2001). Abnormal joint torque patterns in the paretic upper limb of subjects with hemiparesis. Muscle Nerve 24, 273–283.
Dobkin, B. H. (2017). A rehabilitation-internet-of-things in the home to augment motor skills and exercise training. Neurorehabil. Neural Repair 31, 217–227. doi: 10.1177/1545968316680490
Dodd, K. C., Nair, V. A., and Prabhakaran, V. (2017). Role of the Contralesional vs. Ipsilesional hemisphere in stroke recovery. Front. Hum. Neurosci. 11:469. doi: 10.3389/fnhum.2017.00469
Duque, J., Thonnard, J. L., Vandermeeren, Y., Sebire, G., Cosnard, G., and Olivier, E. (2003). Correlation between impaired dexterity and corticospinal tract dysgenesis in congenital hemiplegia. Brain 126(Pt 3), 732–747. doi: 10.1093/brain/awg069
Eliasson, A. C., Gordon, A. M., and Forssberg, H. (1992). Impaired anticipatory control of isometric forces during grasping by children with cerebral palsy. Dev. Med. Child Neurol. 34, 216–225. doi: 10.1111/j.1469-8749.1992.tb14994.x
Elliott, J. M., and Connolly, K. J. (1984). A classification of manipulative hand movements. Dev. Med. Child Neurol. 26, 283–296.
Fagard, J., and Lockman, J. J. (2005). The effect of task constraints on infants’ (bi)manual strategy for grasping and exploring objects. Infant Behav. Dev. 28, 305–315. doi: 10.1016/j.infbeh.2005.05.005
Favre, I., Zeffiro, T. A., Detante, O., Krainik, A., Hommel, M., and Jaillard, A. (2014). Upper limb recovery after stroke is associated with Ipsilesional primary motor cortical activity: a meta-analysis. Stroke 45, 1077–1083. doi: 10.1161/STROKEAHA.113.003168
Feix, T., Romero, J., Schmiedmayer, H. B., Dollar, A. M., and Kragic, D. (2015). “The GRASP taxonomy of human grasp types,” in Proceedings of the IEEE Transactions on Human-Machine Systems (Piscataway, NJ: IEEE), 1–12.
Feldman, A. G. (2015). Referent Control of Action and Perception, Challenging Conventional Theories in Behavioral Neuroscience. New York, NY: Springer-Verlag.
Ferreira, C., Guimarães, V., Santos, A., and Sousa, I. (2014). “Gamification of stroke rehabilitation exercises using a smartphone,” in Proceedings of the 8th International Conference on Pervasive Computing Technologies for Healthcare (PervasiveHealth ’14), Brussels.
Flanagan, R. J., Merritt, K., and Johansson, R. (2009). “Predictive mechanisms and object representations used in object manipulation,” in Sensorimotor Control of Grasping, Physiology and Pathophysiology, eds D. A. Nowak and J. Hermsdörfer (Cambridge: Cambridge University Press), 161–177.
French, B., Thomas, L., Leathley, M., Sutton, C., McAdam, J., Forster, A., et al. (2010). Does repetitive task training improve functional activity after stroke? A Cochrane systematic review and meta-analysis. J. Rehabil. Med. 42, 9–14. doi: 10.2340/16501977-0473
Friel, K. M., and Nudo, R. J. (1998). Recovery of motor function after focal cortical injury in primates: compensatory movement patterns used during rehabilitative training. Somatosens Mot. Res. 15, 173–189.
Fugl-Meyer, A. R., Jaasko, L., Leyman, I., Olsson, S., and Steglind, S. (1975). The post-stroke hemiplegic patient. 1. a method for evaluation of physical performance. Scand J. Rehabil. Med. 7, 13–31.
Garcia Alvarez, A., Roby-Brami, A., Robertson, J., and Roche, N. (2017). Functional classification of grasp strategies used by hemiplegic patients. PLoS One 12:e0187608. doi: 10.1371/journal.pone.0187608
Gerloff, C., Bushara, K., Sailer, A., Wassermann, E. M., Chen, R., Matsuoka, T., et al. (2006). Multimodal imaging of brain reorganization in motor areas of the contralesional hemisphere of well recovered patients after capsular stroke. Brain 129(Pt 3), 791–808. doi: 10.1093/brain/awh713
Giboin, L. S., Lackmy-Vallee, A., Burke, D., and Marchand-Pauvert, V. (2012). Enhanced propriospinal excitation from hand muscles to wrist flexors during reach-to-grasp in humans. J. Neurophysiol. 107, 532–543. doi: 10.1152/jn.00774.2011
Gracies, J. M. (2005a). Pathophysiology of spastic paresis. I: paresis and soft tissue changes. Muscle Nerve 31, 535–551.
Gracies, J. M. (2005b). Pathophysiology of spastic paresis. II: emergence of muscle overactivity. Muscle Nerve 31, 552–571.
Guigon, E., Baraduc, P., and Desmurget, M. (2007). Computational motor control: redundancy and invariance. J. Neurophysiol. 97, 331–347.
Gulde, P., Schmidle, S., Aumuller, A., and Hermsdorfer, J. (2019). The effects of speed of execution on upper-limb kinematics in activities of daily living with respect to age. Exp. Brain Res. 237, 1383–1395. doi: 10.1007/s00221-019-05507-0
Guzsvinecz, T., Szucs, V., and Sik-Lanyi, C. (2019). Suitability of the kinect sensor and leap motion controller-A literature review. Sensors 19:1072. doi: 10.3390/s19051072
Haaland, K. Y., Mutha, P. K., Rinehart, J. K., Daniels, M., Cushnyr, B., and Adair, J. C. (2012). Relationship between arm usage and instrumental activities of daily living after unilateral stroke. Arch. Phys. Med. Rehabil. 93, 1957–1962.
Han, C. E., Arbib, M. A., and Schweighofer, N. (2008). Stroke rehabilitation reaches a threshold. PLoS Comput. Biol. 4:e1000133. doi: 10.1371/journal.pcbi.1000133
Hermsdorfer, J., Hagl, E., and Nowak, D. A. (2004). Deficits of anticipatory grip force control after damage to peripheral and central sensorimotor systems. Hum. Mov. Sci. 23, 643–662.
Hermsdorfer, J., Hagl, E., Nowak, D. A., and Marquardt, C. (2003). Grip force control during object manipulation in cerebral stroke. Clin. Neurophysiol. 114, 915–929.
Hermsdorfer, J., Laimgruber, K., Kerkhoff, G., Mai, N., and Goldenberg, G. (1999). Effects of unilateral brain damage on grip selection, coordination, and kinematics of Ipsilesional prehension. Exp. Brain Res. 128, 41–51.
Hidaka, Y., Han, C. E., Wolf, S. L., Winstein, C. J., and Schweighofer, N. (2012). Use it and improve it or lose it: interactions between arm function and use in humans post-stroke. PLoS Comput. Biol. 8:e1002343.
Honeycutt, C. F., Kharouta, M., and Perreault, E. J. (2013). Evidence for reticulospinal contributions to coordinated finger movements in humans. J. Neurophysiol. 110, 1476–1483. doi: 10.1152/jn.00866.2012
Hope, T. M. H., Friston, K., Price, C. J., Leff, A. P., Rotshtein, P., and Bowman, H. (2019). Recovery after stroke: not so proportional after all? Brain 142, 15–22. doi: 10.1093/brain/awy302
Horn, S. D., DeJong, G., and Deutscher, D. (2012). Practice-based evidence research in rehabilitation: an alternative to randomized controlled trials and traditional observational studies. Arch. Phys. Med. Rehabil. 93(8 Suppl.), S127–S137. doi: 10.1016/j.apmr.2011.10.031
Hussain, A., Dailey, W., Balasubramanian, S., Jarrasse, N., Samuelkamaleshkumar, S., Devasahayam, S., et al. (2015). “Quantitative assessment of motor deficit with an intelligent key object: a pilot study,” in Proceedings of the 14th International Conference on Rehabilitation Robotics (ICORR), Singapore, 247–252.
Iberall, T., Bingham, G., and Arbib, M. A. (1986). “Opposition space as a structuring concept for the analysis of skilled hand movements,” in Generation and Modulation of Action Patterns, eds H. Heuer and C. Fromm (Berlin: Springer), 158–173.
Jamwal, R., Jarman, H. K., Roseingrave, E., Douglas, J., and Winkler, D. (2020). Smart home and communication technology for people with disability: a scoping review. Disabil Rehabil Assist Technol. doi: 10.1080/17483107.2020.1818138 Online ahead of print
Janiaud, P., Serghiou, S., and Ioannidis, J. P. A. (2019). New clinical trial designs in the era of precision medicine: an overview of definitions, strengths, weaknesses, and current use in oncology. Cancer Treat. Rev. 73, 20–30. doi: 10.1016/j.ctrv.2018.12.003
Jarrassé, N., Kuhne, M., Roach, N., Hussain, A., Balasubramanian, S., Burdet, E., et al. (2013). Analysis of grasping strategies and function in hemiparetic patients using an instrumented object. IEEE Int. Conf. Rehabil. Robot. 2013:6650379.
Jeannerod, M. (1984). The timing of natural prehension movements. J. Mot. Behav. 16, 235–254. doi: 10.1080/00222895.1984.10735319
Jeannerod, M. (2009). “The study of hand movements during grasping. A historical perspective,” in Sensorimotor Control of Grasping, Physiology and Pathophysiology, ed. D. A. Nowak and J. Hermsdörfer (Cambridge: Cambridge University press), 127–140.
Johansson, R., and Flanagan, J. (2009). “Sensory control of object manipulation,” in Sensorimotor Control of Grasping, Physiology and Pathophysiology, ed. D. A. Nowak and J. Hermsdorfer (Cambridge: Cambridge University Press).
Johansson, R. S., and Cole, K. J. (1992). Sensory-motor coordination during grasping and manipulative actions. Curr. Opin. Neurobiol. 2, 815–823.
Johnson, M. J., Wang, S., Bai, P., Strachota, E., Tchekanov, G., Melbye, J., et al. (2011). Bilateral assessment of functional tasks for robot-assisted therapy applications. Med. Biol. Eng. Comput. 49, 1157–1171.
Jones, M., Collier, G., Reinkensmeyer, D. J., DeRuyter, F., Dzivak, J., Zondervan, D., et al. (2020). Big data analytics and sensor-enhanced activity management to improve effectiveness and efficiency of outpatient medical rehabilitation. Int. J. Environ. Res. Public Health 17:748. doi: 10.3390/ijerph17030748
Jorgensen, H. S., Nakayama, H., Raaschou, H. O., Vive-Larsen, J., Stoier, M., and Olsen, T. S. (1995). Outcome and time course of recovery in stroke. Part I: outcome. The copenhagen stroke study. Arch. Phys. Med. Rehabil. 76, 399–405.
Kalaska, J. F. (2009). From intention to action: motor cortex and the control of reaching movements. Adv. Exp. Med. Biol. 629, 139–178. doi: 10.1007/978-0-387-77064-2_8
Kamper, D. G., Fischer, H. C., Conrad, M. O., Towles, J. D., Rymer, W. Z., and Triandafilou, K. M. (2014). Finger-thumb coupling contributes to exaggerated thumb flexion in stroke survivors. J. Neurophysiol. 111, 2665–2674. doi: 10.1152/jn.00413.2013
Kantak, S., Jax, S., and Wittenberg, G. (2017). Bimanual coordination: a missing piece of arm rehabilitation after stroke. Restor. Neurol. Neurosci. 35, 347–364. doi: 10.3233/RNN-170737
Kitago, T., and Krakauer, J. W. (2013). Motor learning principles for neurorehabilitation. Handb. Clin. Neurol. 110, 93–103.
Kitago, T., Liang, J., Huang, V. S., Hayes, S., Simon, P., Tenteromano, L., et al. (2013). Improvement after constraint-induced movement therapy: recovery of normal motor control or task-specific compensation? Neurorehabil. Neural Repair 27, 99–109.
Koechlin, E., and Jubault, T. (2006). Broca’s area and the hierarchical organization of human behavior. Neuron 50, 963–974.
Kollias, S. S., Alkadhi, H., Jaermann, T., Crelier, G., and Hepp-Reymond, M. C. (2001). Identification of multiple nonprimary motor cortical areas with simple movements. Brain Res. Brain Res. Rev. 36, 185–195. doi: 10.1016/s0165-0173(01)00094-7
Krakauer, J. W. (2006). Motor learning: its relevance to stroke recovery and neurorehabilitation. Curr. Opin. Neurol. 19, 84–90.
Kwakkel, G., Van Wegen, E., Burridge, J. H., Winstein, C. J., van Dokkum, L., Alt Murphy, M., et al. (2019). Standardized measurement of quality of upper limb movement after stroke: consensus-based core recommendations from the Second Stroke Recovery and Rehabilitation Roundtable. Int. J. Stroke 14, 783–791. doi: 10.1177/1747493019873519
Kwakkel, G., Veerbeek, J. M., van Wegen, E. E., and Wolf, S. L. (2015). Constraint-induced movement therapy after stroke. Lancet Neurol. 14, 224–234. doi: 10.1016/S1474-4422(14)70160-7
Laffont, I., Briand, E., Dizien, O., Combeaud, M., Bussel, B., Revol, M., et al. (2000). Kinematics of prehension and pointing movements in C6 quadriplegic patients. Spinal Cord. 38, 354–362.
Lang, C. E., and Schieber, M. H. (2003). Differential impairment of individuated finger movements in humans after damage to the motor cortex or the corticospinal tract. J. Neurophysiol. 90, 1160–1170.
Lang, C. E., and Schieber, M. H. (2004). Reduced muscle selectivity during individuated finger movements in humans after damage to the motor cortex or corticospinal tract. J. Neurophysiol. 91, 1722–1733.
Lang, C. E., Wagner, J. M., Bastian, A. J., Hu, Q., Edwards, D. F., Sahrmann, S. A., et al. (2005). Deficits in grasp versus reach during acute hemiparesis. Exp. Brain Res. 166, 126–136. doi: 10.1007/s00221-005-2350-6
Latash, M. L., and Anson, J. (1996). What are “normal movements” in atypical populations? Behav. Brain Sci. 19, 55–68. doi: 10.1017/S0140525X00041467
Latash, M. L., Friedman, J., Kim, S. W., Feldman, A. G., and Zatsiorsky, V. M. (2010). Prehension synergies and control with referent hand configurations. Exp. Brain Res. 202, 213–229.
Laver, K. E., Lange, B., George, S., Deutsch, J. E., Saposnik, G., and Crotty, M. (2017). Virtual reality for stroke rehabilitation. Cochrane Database Syst. Rev. 11:CD008349. doi: 10.1002/14651858.CD008349.pub4
Lawrence, D. G., and Kuypers, H. G. (1968). The functional organization of the motor system in the monkey. II The effects of lesions of the descending brain-stem pathways. Brain 91, 15–36.
Lemon, R. N. (1999). Neural control of dexterity: what has been achieved? Exp. Brain Res. 128, 6–12.
Lemon, R. N., Kirkwood, P. A., Maier, M. A., Nakajima, K., and Nathan, P. (2004). Direct and indirect pathways for corticospinal control of upper limb motoneurons in the primate. Prog. Brain Res. 143, 263–279. doi: 10.1016/S0079-6123(03)43026-4
Leo, A., Handjaras, G., Bianchi, M., Marino, H., Gabiccini, M., Guidi, A., et al. (2016). A synergy-based hand control is encoded in human motor cortical areas. Elife 5:e13420. doi: 10.7554/eLife.13420
Levin, M. F. (1996). Interjoint coordination during pointing movements is disrupted in spastic hemiparesis. Brain 119, 281–293.
Levin, M. F. (2016). Principles of motor recovery after neurological injury based on a motor control theory. Adv. Exp. Med. Biol. 957, 121–140. doi: 10.1007/978-3-319-47313-0_7
Levin, M. F., Desrosiers, J., Beauchemin, D., Bergeron, N., and Rochette, A. (2004). Development and validation of a scale for rating motor compensations used for reaching in patients with hemiparesis: the reaching performance scale. Phys. Ther. 84, 8–22.
Levin, M. F., Kleim, J. A., and Wolf, S. L. (2009). What do motor “recovery” and “compensation” mean in patients following stroke? Neurorehabil Neural Repair 23, 313–319.
Levin, M. F., Liebermann, D. G., Parmet, Y., and Berman, S. (2016). Compensatory versus noncompensatory shoulder movements used for reaching in stroke. Neurorehabil. Neural Repair 30, 635–646. doi: 10.1177/1545968315613863
Levin, M. F., Michaelsen, S. M., Cirstea, C. M., and Roby-Brami, A. (2002). Use of the trunk for reaching targets placed within and beyond the reach in adult hemiparesis. Exp. Brain Res. 143, 171–180.
Levin, M. F., and Panturin, E. (2011). Sensorimotor integration for functional recovery and the Bobath approach. Motor Control 15, 285–301.
Li, S. (2017). Spasticity, motor recovery, and neural plasticity after stroke. Front. Neurol. 8:120. doi: 10.3389/fneur.2017.00120
Li, S., Latash, M. L., Yue, G. H., Siemionow, V., and Sahgal, V. (2003). The effects of stroke and age on finger interaction in multi-finger force production tasks. Clin. Neurophysiol. 114, 1646–1655. doi: 10.1016/s1388-2457(03)00164-0
Lin, B. S., Hsiao, P. C., Yang, S. Y., Su, C. S., and Lee, I. J. (2017). Data glove system embedded with inertial measurement units for hand function evaluation in stroke patients. IEEE Trans. Neural. Syst. Rehabil. Eng. 25, 2204–2213. doi: 10.1109/TNSRE.2017.2720727
Lindberg, P. G., Skejo, P. H., Rounis, E., Nagy, Z., Schmitz, C., Wernegren, H., et al. (2007). Wallerian degeneration of the corticofugal tracts in chronic stroke: a pilot study relating diffusion tensor imaging, transcranial magnetic stimulation, and hand function. Neurorehabil. Neural Repair 21, 551–560.
Loubinoux, I., Brihmat, N., Castel-Lacanal, E., and Marque, P. (2017). Cerebral imaging of post-stroke plasticity and tissue repair. Rev. Neurol. 173, 577–583. doi: 10.1016/j.neurol.2017.09.007
Maceira-Elvira, P., Popa, T., Schmid, A. C., and Hummel, F. C. (2019). Wearable technology in stroke rehabilitation: towards improved diagnosis and treatment of upper-limb motor impairment. J. Neuroeng. Rehabil. 16:142. doi: 10.1186/s12984-019-0612-y
Maenza, C., Good, D. C., Winstein, C. J., Wagstaff, D. A., and Sainburg, R. L. (2020). Functional deficits in the less-impaired arm of stroke survivors depend on hemisphere of damage and extent of paretic arm impairment. Neurorehabil. Neural Repair 34, 39–50. doi: 10.1177/1545968319875951
Maier, M., Ballester, B. R., and Verschure, P. (2019). Principles of neurorehabilitation after stroke based on motor learning and brain plasticity mechanisms. Front. Syst. Neurosci. 13:74. doi: 10.3389/fnsys.2019.00074
Maier, M., Kirkwood, P., Nakajima, K., and Lemon, R. (2005). “The importance of direct vs indirect corticospinal connections for dexterity and their evolution,” in Stone Knapping, the Necessary Condition for an Uniquely Hominin Behaviour, eds V. Roux and B. Bril (Cambridge: McDonald Institute), 257–272.
Martin, V., Scholz, J. P., and Schoner, G. (2009). Redundancy, self-motion, and motor control. Neural Comput. 21, 1371–1414. doi: 10.1162/neco.2008.01-08-698
Martin-Brevet, S., Jarrasse, N., Burdet, E., and Roby-Brami, A. (2017). Taxonomy based analysis of force exchanges during object grasping and manipulation. PLoS One 12:e0178185. doi: 10.1371/journal.pone.0178185
Marzke, M. W., and Marzke, R. F. (2000). Evolution of the human hand: approaches to acquiring, analysing and interpreting the anatomical evidence. J. Anat. 197(Pt 1), 121–140. doi: 10.1046/j.1469-7580.2000.19710121.x
Mawase, F., Cherry-Allen, K., Xu, J., Anaya, M., Uehara, S., and Celnik, P. (2020). Pushing the rehabilitation boundaries: hand motor impairment can be reduced in chronic stroke. Neurorehabil. Neural Repair 34, 733–745. doi: 10.1177/1545968320939563
Mazevet, D., Meunier, S., Pradat-Diehl, P., Marchand-Pauvert, V., and Pierrot-Deseilligny, E. (2003). Changes in propriospinally mediated excitation of upper limb motoneurons in stroke patients. Brain 126, 988–1000.
McMorland, A. J., Runnalls, K. D., and Byblow, W. D. (2015). A neuroanatomical framework for upper limb synergies after stroke. Front. Hum. Neurosci. 9:82. doi: 10.3389/fnhum.2015.00082
McPherson, J. G., Chen, A., Ellis, M. D., Yao, J., Heckman, C. J., and Dewald, J. P. A. (2018). Progressive recruitment of contralesional cortico-reticulospinal pathways drives motor impairment post stroke. J. Physiol. 596, 1211–1225. doi: 10.1113/JP274968
Mehrholz, J., Pohl, M., Platz, T., Kugler, J., and Elsner, B. (2018). Electromechanical and robot-assisted arm training for improving activities of daily living, arm function, and arm muscle strength after stroke. Cochrane Database Syst. Rev. 9:CD006876. doi: 10.1002/14651858.CD006876.pub5
Mendez, I., Hansen, B. W., Grabow, C. M., Smedegaard, E. J. L., Skogberg, N. B., Uth, X. J., et al. (2017). Evaluation of the Myo armband for the classification of hand motions. IEEE Int. Conf. Rehabil. Robot. 2017, 1211–1214. doi: 10.1109/ICORR.2017.8009414
Meyer, S., De Bruyn, N., Lafosse, C., Van Dijk, M., Michielsen, M., Thijs, L., et al. (2016). Somatosensory impairments in the upper limb poststroke: distribution and association with motor function and visuospatial neglect. Neurorehabil. Neural Repair 30, 731–742. doi: 10.1177/1545968315624779
Micera, S., Carpaneto, J., Posteraro, F., Cenciotti, L., Popovic, M., and Dario, P. (2005). Characterization of upper arm synergies during reaching tasks in able-bodied and hemiparetic subjects. Clin. Biomech. 20, 939–946.
Michaelsen, S. M., Dannenbaum, R., and Levin, M. F. (2006). Task-specific training with trunk restraint on arm recovery in stroke: randomized control trial. Stroke 37, 186–192.
Michaelsen, S. M., Luta, A., Roby-Brami, A., and Levin, M. F. (2001). Effect of trunk restraint on the recovery of reaching movements in hemiparetic patients. Stroke 32, 1875–1883.
Michielsen, M. E., Selles, R. W., Stam, H. J., Ribbers, G. M., and Bussmann, J. B. (2012). Quantifying nonuse in chronic stroke patients: a study into paretic, nonparetic, and bimanual upper-limb use in daily life. Arch. Phys. Med. Rehabil. 93, 1975–1981. doi: 10.1016/j.apmr.2012.03.016
Monzee, J., Lamarre, Y., and Smith, A. M. (2003). The effects of digital anesthesia on force control using a precision grip. J. Neurophysiol. 89, 672–683. doi: 10.1152/jn.00434.2001
Muir, R. B., and Lemon, R. N. (1983). Corticospinal neurons with a special role in precision grip. Brain Res. 261, 312–316.
Murase, N., Duque, J., Mazzocchio, R., and Cohen, L. G. (2004). Influence of interhemispheric interactions on motor function in chronic stroke. Ann. Neurol. 55, 400–409.
Napier, J. (1961). Prehensility and opposability in the hands of primates. J. Bone Joint Surg. Am. 38B, 902–913.
Napier, J. R. (1956). The prehensile movements of the human hand. J. Bone Joint Surg. 38-B, 902–913.
Newell, K. M. (1986). “Constraints on the development of coordination,” in Motor Development in Children: Aspects of Coordination and Control, eds M. G. Wade and H. T. A. Whiting (Dordrecht: Martinus Nijhoff), 341–360.
Nowak, D. A. (2008). The impact of stroke on the performance of grasping: usefulness of kinetic and kinematic motion analysis. Neurosci. Biobehav. Rev. 32, 1439–1450. doi: 10.1016/j.neubiorev.2008.05.021
Nowak, D. A., and Hermsdorfer, J. (2006). Objective evaluation of manual performance deficits in neurological movement disorders. Brain Res. Rev. 51, 108–124.
Nowak, D. A., and Hermsdorfer, J. (2009). Sensorimotor Control of Grasping, Physiology and Pathophysiology. New York, NY: Cambridge University Press.
Nowak, D. A., Hermsdorfer, J., Glasauer, S., Philipp, J., Meyer, L., and Mai, N. (2001). The effects of digital anaesthesia on predictive grip force adjustments during vertical movements of a grasped object. Eur. J. Neurosci. 14, 756–762. doi: 10.1046/j.0953-816x.2001.01697.x
Nowak, D. A., Hermsdorfer, J., and Topka, H. (2003). Deficits of predictive grip force control during object manipulation in acute stroke. J. Neurol. 250, 850–860.
Nudo, R. J., Milliken, G. W., Jenkins, W. M., and Merzenich, M. M. (1996). Use-dependent alterations of movement representations in primary motor cortex of adult squirrel monkeys. J. Neurosci. 16, 785–807.
Obhi, S. S. (2004). Bimanual coordination: an unbalanced field of research. Motor Control 8, 111–120.
Ohbayashi, M., Picard, N., and Strick, P. L. (2016). Inactivation of the dorsal premotor area disrupts internally generated, but not visually guided, sequential movements. J. Neurosci. 36, 1971–1976. doi: 10.1523/JNEUROSCI.2356-15.2016
Owen, M., Ingo, C., and Dewald, J. P. A. (2017). Upper extremity motor impairments and microstructural changes in bulbospinal pathways in chronic hemiparetic stroke. Front. Neurol. 8:257. doi: 10.3389/fneur.2017.00257
Parry, R., Macias Soria, S., Pradat-Diehl, P., Marchand-Pauvert, V., Jarrasse, N., and Roby-Brami, A. (2019). Effects of hand configuration on the grasping, holding, and placement of an instrumented object in patients with hemiparesis. Front. Neurol. 10:240. doi: 10.3389/fneur.2019.00240
Peters, S., Wadden, K. P., Hayward, K. S., Neva, J. L., Auriat, A. M., and Boyd, L. A. (2017). A structural motor network correlates with motor function and not impairment post stroke. Neurosci. Lett. 658, 155–160. doi: 10.1016/j.neulet.2017.08.036
Pierrot-Deseilligny, E. (1996). Transmission of the cortical command for human voluntary movement through cervical propriospinal premotoneurons. Progress Neurobiol. 48, 489–517.
Platz, T., and Lotze, M. (2018). Arm ability training (AAT) promotes dexterity recovery after a stroke-a review of its design, clinical effectiveness, and the neurobiology of the actions. Front. Neurol. 9:1082. doi: 10.3389/fneur.2018.01082
Platz, T., van Kaick, S., Mehrholz, J., Leidner, O., Eickhof, C., and Pohl, M. (2009). Best conventional therapy versus modular impairment-oriented training for arm paresis after stroke: a single-blind, multicenter randomized controlled trial. Neurorehabil. Neural Repair 23, 706–716. doi: 10.1177/1545968309335974
Prabhakaran, S., Zarahn, E., Riley, C., Speizer, A., Chong, J. Y., Lazar, R. M., et al. (2008). Inter-individual variability in the capacity for motor recovery after ischemic stroke. Neurorehabil. Neural Repair 22, 64–71. doi: 10.1177/1545968307305302
Quaney, B. M., Perera, S., Maletsky, R., Luchies, C. W., and Nudo, R. J. (2005). Impaired grip force modulation in the Ipsilesional hand after unilateral middle cerebral artery stroke. Neurorehabil. Neural Repair 19, 338–349. doi: 10.1177/1545968305282269
Raghavan, P., Petra, E., Krakauer, J. W., and Gordon, A. M. (2006). Patterns of impairment in digit independence after subcortical stroke. J. Neurophysiol. 95, 369–378.
Raghavan, P., Santello, M., Gordon, A. M., and Krakauer, J. W. (2010). Compensatory motor control after stroke: an alternative joint strategy for object-dependent shaping of hand posture. J. Neurophysiol. 103, 3034–3043. doi: 10.1152/jn.00936.2009
Regenhardt, R. W., Takase, H., Lo, E. H., and Lin, D. J. (2020). Translating concepts of neural repair after stroke: structural and functional targets for recovery. Restor. Neurol. Neurosci. 38, 67–92. doi: 10.3233/RNN-190978
Reisman, D. S., and Scholz, J. P. (2003). Aspects of joint coordination are preserved during pointing in persons with post-stroke hemiparesis. Brain 126, 2510–2527.
Reisman, D. S., and Scholz, J. P. (2006). Workspace location influences joint coordination during reaching in post-stroke hemiparesis. Exp. Brain Res. 170, 265–276.
Robertson, J. V., and Roby-Brami, A. (2011). The trunk as a part of the kinematic chain for reaching movements in healthy subjects and hemiparetic patients. Brain Res. 1382, 137–146.
Roby-Brami, A., Feydy, A., Combeaud, M., Biryukova, E. V., Bussel, B., and Levin, M. F. (2003a). Motor compensation and recovery for reaching in stroke patients. Acta Neurol. Scand. 107, 369–381.
Roby-Brami, A., Jacobs, S., Bennis, N., and Levin, M. F. (2003b). Hand orientation for grasping and arm joint rotation patterns in healthy subjects and hemiparetic stroke patients. Brain Res. 969, 217–229. doi: 10.1016/s0006-8993(03)02334-5
Roby-Brami, A., Fuchs, S., Mokhtari, M., and Bussel, B. (1997). Reaching and grasping strategies in hemiparetic patients. Motor Control 1, 72–91.
Rohrer, B., Fasoli, S., Krebs, H. I., Hughes, R., Volpe, B., Frontera, W. R., et al. (2002). Movement smoothness changes during stroke recovery. J. Neurosci. 22, 8297–8304.
Rowlands, M. (2010). The New Science of the Mind: From Extended Mind to Embodied Phenomenology. Cambridge, MA: MIT Press.
Sainburg, R. L., Maenza, C., Winstein, C., and Good, D. (2016). Motor lateralization provides a foundation for predicting and treating non-paretic arm motor deficits in stroke. Adv. Exp. Med. Biol. 957, 257–272. doi: 10.1007/978-3-319-47313-0_14
Sampaio-Baptista, C., Sanders, Z. B., and Johansen-Berg, H. (2018). Structural plasticity in adulthood with motor learning and stroke rehabilitation. Annu. Rev. Neurosci. 41, 25–40. doi: 10.1146/annurev-neuro-080317-62015
Sandrini, M., and Cohen, L. G. (2013). Noninvasive brain stimulation in neurorehabilitation. Handb Clin. Neurol. 116, 499–524. doi: 10.1016/B978-0-444-53497-2.00040-1
Sangole, A. P., and Levin, M. F. (2009). Palmar arch modulation in patients with hemiparesis after a stroke. Exp. Brain Res. 199, 59–70.
Santello, M., Bianchi, M., Gabiccini, M., Ricciardi, E., Salvietti, G., Prattichizzo, D., et al. (2016). Hand synergies: integration of robotics and neuroscience for understanding the control of biological and artificial hands. Phys. Life Rev. 17, 1–23. doi: 10.1016/j.plrev.2016.02.001
Santello, M., Flanders, M., and Soechting, J. F. (1998). Postural hand synergies for tool use. J. Neurosci. 18, 10105–10115.
Santello, M., and Soechting, J. F. (1998). Gradual molding of the hand to object contours. J. Neurophysiol. 79, 1307–1320. doi: 10.1152/jn.1998.79.3.1307
Santisteban, L., Teremetz, M., Bleton, J. P., Baron, J. C., Maier, M. A., and Lindberg, P. G. (2016). Upper limb outcome measures used in stroke rehabilitation studies: a systematic literature review. PLoS One 11:e0154792.
Schieber, M. H., Lang, C. E., Reilly, K. T., McNulty, P., and Sirigu, A. (2009). Selective activation of human finger muscles after stroke or amputation. Adv. Exp. Med. Biol. 629, 559–575. doi: 10.1007/978-0-387-77064-2_30
Schneider, T. R., Buckingham, G., and Hermsdorfer, J. (2020). Visual cues, expectations, and sensorimotor memories in the prediction and perception of object dynamics during manipulation. Exp. Brain Res. 238, 395–409. doi: 10.1007/s00221-019-05711-y
Scholz, J. P., Schöner, G., and Latash, M. L. (2000). Identifying the control structure of multijoint coordination during pistol shooting. Exp. Brain Res. 135, 382–404.
Seligman, S. C., Giovannetti, T., Sestito, J., and Libon, D. J. (2014). A new approach to the characterization of subtle errors in everyday action: implications for mild cognitive impairment. Clin. Neuropsychol. 28, 97–115.
Senesh, M. R., and Reinkensmeyer, D. J. (2019). Breaking proportional recovery after stroke. Neurorehabil. Neural Repair 33, 888–901. doi: 10.1177/1545968319868718
Seo, N. J., Rymer, W. Z., and Kamper, D. G. (2010). Altered digit force direction during pinch grip following stroke. Exp. Brain Res. 202, 891–901.
Sirtori, V., Corbetta, D., Moja, L., and Gatti, R. (2009). Constraint-induced movement therapy for upper extremities in stroke patients. Cochrane Database Syst. Rev. 7:CD004433. doi: 10.1002/14651858.CD004433.pub2
Smeets, J. B. J., van der Kooij, K., and Brenner, E. (2019). A review of grasping as the movements of digits in space. J. Neurophysiol. 122, 1578–1597. doi: 10.1152/jn.00123.2019
Spiers, A. J., Resnik, L., and Dollar, A. M. (2017). Analyzing at-home prosthesis use in unilateral upper-limb amputees to inform treatment & device design. IEEE Int. Conf. Rehabil. Robot. 2017, 1273–1280. doi: 10.1109/ICORR.2017.8009424
Taub, E., Miller, N. E., Novack, T. A., Cook, E. W. III, Fleming, W. C., Nepomuceno, C. S., et al. (1993). Technique to improve chronic motor deficit after stroke. Arch. Phys. Med. Rehabil. 74, 347–354.
Taub, E., Uswatte, G., Mark, V. W., and Morris, D. M. (2006). The learned nonuse phenomenon: implications for rehabilitation. Eura Medicophys. 42, 241–256.
Taub, E., Uswatte, G., and Pidikiti, R. (1999). Constraint-induced movement therapy: a new family of techniques with broad application to physical rehabilitation–a clinical review. J. Rehabil. Res. Dev. 36, 237–251.
Teremetz, M., Colle, F., Hamdoun, S., Maier, M. A., and Lindberg, P. G. (2015). A novel method for the quantification of key components of manual dexterity after stroke. J. Neuroeng. Rehabil. 12:64. doi: 10.1186/s12984-015-0054-0
Timmermans, A. A., Spooren, A. I., Kingma, H., and Seelen, H. A. (2010). Influence of task-oriented training content on skilled arm-hand performance in stroke: a systematic review. Neurorehabil. Neural Repair 24, 858–870. doi: 10.1177/1545968310368963
Ting, L. H., and McKay, J. L. (2007). Neuromechanics of muscle synergies for posture and movement. Curr. Opin. Neurobiol. 17, 622–628.
Tretriluxana, J., Gordon, J., Fisher, B. E., and Winstein, C. J. (2009). Hemisphere specific impairments in reach-to-grasp control after stroke: effects of object size. Neurorehabil. Neural Repair 23, 679–691. doi: 10.1177/1545968309332733
Triandafilou, K. M., Fischer, H. C., Towles, J. D., Kamper, D. G., and Rymer, W. Z. (2011). Diminished capacity to modulate motor activation patterns according to task contributes to thumb deficits following stroke. J. Neurophysiol. 106, 1644–1651. doi: 10.1152/jn.00936.2010
Trombly, C. A. (1993). Observations of improvement of reaching in five subjects with left hemiparesis. J. Neurol. Neurosurg. Psychiatry 56, 40–45.
Twitchell, T. E. (1951). The restoration of motor function following hemiplegia in man. Brain 74, 443–480.
van Dokkum, L., Hauret, I., Mottet, D., Froger, J., Metrot, J., and Laffont, I. (2014). The contribution of kinematics in the assessment of upper limb motor recovery early after stroke. Neurorehabil. Neural Repair 28, 4–12. doi: 10.1177/1545968313498514
van Kordelaar, J., van Wegen, E., and Kwakkel, G. (2014). Impact of time on quality of motor control of the paretic upper limb after stroke. Arch. Phys. Med. Rehabil. 95, 338–344. doi: 10.1016/j.apmr.2013.10.006
van Kordelaar, J., van Wegen, E. E., and Kwakkel, G. (2012). Unraveling the interaction between pathological upper limb synergies and compensatory trunk movements during reach-to-grasp after stroke: a cross-sectional study. Exp. Brain Res. 221, 251–262. doi: 10.1007/s00221-012-3169-6
Villepinte, C., Verma, A., Dimeglio, C., De Boissezon, X., and Gasq, D. (2020). Responsiveness of kinematic and clinical measures of upper-limb motor function after stroke: a systematic review and meta-analysis. Ann. Phys. Rehabil. Med. 64:101366. doi: 10.1016/j.rehab.2020.02.005
Wang, Q., Markopoulos, P., Yu, B., Chen, W., and Timmermans, A. (2017). Interactive wearable systems for upper body rehabilitation: a systematic review. J Neuroeng Rehabil. 14:20. doi: 10.1186/s12984-017-0229-y
Ward, N. S., Brander, F., and Kelly, K. (2019). Intensive upper limb neurorehabilitation in chronic stroke: outcomes from the queen square programme. J. Neurol. Neurosurg. Psychiatry 90, 498–506. doi: 10.1136/jnnp-2018-319954
Ward, N. S., and Carmichael, S. T. (2020). Blowing up neural repair for stroke recovery: preclinical and clinical trial considerations. Stroke 51, 3169–3173. doi: 10.1161/STROKEAHA.120.030486
Wee, S. K., Hughes, A. M., Warner, M., and Burridge, J. H. (2014). Trunk restraint to promote upper extremity recovery in stroke patients: a systematic review and meta-analysis. Neurorehabil. Neural Repair 28, 660–677.
Westling, G., and Johansson, R. S. (1984). Factors influencing the force control during precision grip. Exp. Brain Res. 53, 277–284.
Whitall, J., Waller, S. M., Sorkin, J. D., Forrester, L. W., Macko, R. F., Hanley, D. F., et al. (2011). Bilateral and unilateral arm training improve motor function through differing neuroplastic mechanisms: a single-blinded randomized controlled trial. Neurorehabil. Neural Repair 25, 118–129. doi: 10.1177/1545968310380685
Wing, A. (1996). “Anticipatory control of grip force in rapid arm movement,” in Hand and Brain: Neurophysiology and Psychology of Hand Movement, eds A. M. Wing, P. Haggard, and J. R. Flanagan (Cambridge, MA: Academic press).
Winstein, C., Kim, B., Kim, S., Martinez, C., and Schweighofer, N. (2019). Dosage Matters. Stroke 50, 1831–1837. doi: 10.1161/STROKEAHA.118.023603
Winstein, C., and Varghese, R. (2018). Been there, done that, so what’s next for arm and hand rehabilitation in stroke? NeuroRehabilitation 43, 3–18. doi: 10.3233/NRE-172412
Winstein, C. J., Stein, J., Arena, R., Bates, B., Cherney, L. R., Cramer, S. C., et al. (2016a). Guidelines for adult stroke rehabilitation and recovery: a guideline for healthcare professionals from the american heart association/american stroke association. Stroke 47, e98–e169. doi: 10.1161/STR.0000000000000098
Winstein, C. J., Wolf, S. L., Dromerick, A. W., Lane, C. J., Nelsen, M. A., Lewthwaite, R., et al. (2016b). Effect of a task-oriented rehabilitation program on upper extremity recovery following motor stroke: the ICARE randomized clinical trial. JAMA 315, 571–581. doi: 10.1001/jama.2016.0276
Wolbrecht, E. T., Rowe, J. B., Chan, V., Ingemanson, M. L., Cramer, S. C., and Reinkensmeyer, D. J. (2018). Finger strength, individuation, and their interaction: relationship to hand function and corticospinal tract injury after stroke. Clin. Neurophysiol. 129, 797–808. doi: 10.1016/j.clinph.2018.01.057
Wolf, T. J., Chuh, A., Floyd, T., McInnis, K., and Williams, E. (2015). Effectiveness of occupation-based interventions to improve areas of occupation and social participation after stroke: an evidence-based review. Am. J. Occup. Ther. 69, 6901180060p1–6901180060p11. doi: 10.5014/ajot.2015.012195
Worthington, A. (2016). Treatments and technologies in the rehabilitation of apraxia and action disorganisation syndrome: a review. NeuroRehabilitation 39, 163–174. doi: 10.3233/NRE-161348
Xerri, C., Zennou-Azogui, Y., Sadlaoud, K., and Sauvajon, D. (2014). Interplay between intra- and interhemispheric remodeling of neural networks as a substrate of functional recovery after stroke: adaptive versus maladaptive reorganization. Neuroscience 283, 178–201. doi: 10.1016/j.neuroscience.2014.06.066
Yang, J. F., Scholz, J. P., and Latash, M. L. (2007). The role of kinematic redundancy in adaptation of reaching. Exp. Brain Res. 176, 54–69.
Yang, N., Zhang, M., Huang, C., and Jin, D. (2002). Synergic analysis of upper limb target-reaching movements. J. Biomech. 35, 739–746.
York, G. K., and Steinberg, D. A. (1995). Hughlings Jackson’s theory of recovery. Neurology 45, 834–838.
Zaaimi, B., Edgley, S. A., Soteropoulos, D. S., and Baker, S. N. (2012). Changes in descending motor pathway connectivity after corticospinal tract lesion in macaque monkey. Brain 135(Pt 7), 2277–2289. doi: 10.1093/brain/aws115
Keywords: rehabilitation, recovery, stroke, disability, activity, physiopathologic mechanism
Citation: Roby-Brami A, Jarrassé N and Parry R (2021) Impairment and Compensation in Dexterous Upper-Limb Function After Stroke. From the Direct Consequences of Pyramidal Tract Lesions to Behavioral Involvement of Both Upper-Limbs in Daily Activities. Front. Hum. Neurosci. 15:662006. doi: 10.3389/fnhum.2021.662006
Received: 31 January 2021; Accepted: 27 May 2021;
Published: 21 June 2021.
Edited by:
Sivakumar Balasubramanian, Christian Medical College & Hospital, IndiaReviewed by:
Joon-Ho Shin, National Rehabilitation Center, South KoreaMirko Filippetti, University of Verona, Italy
Augusto Fusco, Fondazione Policlinico Universitario Agostino Gemelli IRCCS, Italy
Copyright © 2021 Roby-Brami, Jarrassé and Parry. This is an open-access article distributed under the terms of the Creative Commons Attribution License (CC BY). The use, distribution or reproduction in other forums is permitted, provided the original author(s) and the copyright owner(s) are credited and that the original publication in this journal is cited, in accordance with accepted academic practice. No use, distribution or reproduction is permitted which does not comply with these terms.
*Correspondence: Agnès Roby-Brami, roby-brami@isir.upmc.fr