- 1 Department of Physiology and Cell Biology, The Ohio State University, Columbus, OH, USA
- 2 Department of Pharmacological and Pharmaceutical Sciences, University of Houston, Houston, TX, USA
- 3 Department of Anatomy and Cell Biology, Indiana University School of Medicine, Indianapolis, IN, USA
Ca2+ dissociation from the regulatory domain of troponin C may influence the rate of striated muscle relaxation. However, Ca2+ dissociation from troponin C has not been measured within the geometric and stoichiometric constraints of the muscle fiber. Here we report the rates of Ca2+ dissociation from the N-terminal regulatory and C-terminal structural domains of fluorescent troponin C constructs reconstituted into rabbit rigor psoas myofibrils using stopped-flow technology. Chicken skeletal troponin C fluorescently labeled at Cys 101, troponin CIAEDANS, reported Ca2+ dissociation exclusively from the structural domain of troponin C at ∼0.37, 0.06, and 0.07/s in isolation, in the presence of troponin I and in myofibrils at 15°C, respectively. Ca2+ dissociation from the regulatory domain was observed utilizing fluorescently labeled troponin C containing the T54C and C101S mutations. Troponin reported Ca2+ dissociation exclusively from the regulatory domain of troponin C at >1000, 8.8, and 15/s in isolation, in the presence of troponin I and in myofibrils at 15°C, respectively. Interestingly, troponin
reported a biphasic fluorescence change upon Ca2+ dissociation from the N- and C-terminal domains of troponin C with rates that were similar to those reported by troponin
and troponin CIAEDANS at all levels of the troponin C systems. Furthermore, the rate of Ca2+ dissociation from troponin C in the myofibrils was similar to the rate of Ca2+ dissociation measured from the troponin C-troponin I complexes. Since the rate of Ca2+ dissociation from the regulatory domain of TnC in myofibrils is similar to the rate of skeletal muscle relaxation, Ca2+ dissociation from troponin C may influence relaxation.
Introduction
Fast-twitch skeletal muscle troponin C (TnC) contains two N-terminal and two C-terminal EF-hand Ca2+ binding sites that can be easily distinguished according to their Ca2+ dissociation rates (Johnson et al., 1981). Based on data obtained from TnC in solution, only the N-terminal EF-hands release Ca2+ fast enough to be involved in the regulation of muscle relaxation (Johnson et al., 1981; Stephenson and Williams, 1981). However, this hypothesis has not been tested with TnC bound in the myofibril.
Clearly, the mechanisms that determine or modulate the rate of striated muscle relaxation are biochemical and mechanical (Stephenson and Williams, 1981; Wahr et al., 1998; Gordon et al., 2000; Luo et al., 2002; Tesi et al., 2002). Thus, it is important to link the fundamental relationships of muscle biochemistry to that of its physiology. There are at least three biochemical factors that can potentially influence the rate of striated muscle relaxation: (1) the rate of fall in the Ca2+ transient; (2) the rate of cross-bridge detachment; and (3) the rate of deactivation of the thin filament controlled by Ca2+ dissociation from the N-terminal regulatory domain of TnC (Gordon et al., 2000; Luo et al., 2002). Under normal physiological conditions it may be that no one mechanism is the all-inclusive rate-limiting step of relaxation, but that these processes may be working together (Johnson et al., 1997; Luo et al., 2002).
Previously, we demonstrated that TnC mutants with faster or slower regulatory domain Ca2+ dissociation rates in solution increased, but more effectively decreased, the rate of Diazo-2 induced relaxation when reconstituted into rabbit skinned psoas muscle (Luo et al., 2002). The rate of Ca2+ dissociation from TnC in solution is at least 20-times faster than the rate of skeletal muscle relaxation and thus is too rapid to be considered rate-limiting for relaxation (Stephenson and Williams, 1981; Luo et al., 2002). However, isolated TnC is not a good model system for troponin (Tn) in muscle (Davis et al., 2002, 2004). The simplest model system for Ca2+ dissociation from TnC in skeletal muscle may be the rate of Ca2+ dissociation from the regulatory domain of TnC bound to troponin I (TnI) or its peptide fragment, TnI96–148 (Davis et al., 2002, 2004). For instance, the rate of Ca2+ dissociation from the regulatory domain of the TnC–TnI96–148, TnC–TnI and Tn complexes, are all quantitatively similar to the rate of skeletal muscle relaxation at ∼4, 15, and 20°C (Stephenson and Williams, 1981; Johnson et al., 1997; Luo et al., 2002; Davis et al., 2004; Kreutziger et al., 2008; Lee et al., 2010).
In order to ultimately test the hypothesis that the rate of Ca2+ dissociation from the N-terminal regulatory domain of TnC contributes to the rate of skeletal muscle relaxation, one must be able to measure this rate in a muscle fiber and confirm that it is quantitatively similar to the rate of muscle relaxation. To date this has not been accomplished, nor to the best of our knowledge even attempted. However, numerous studies have attempted to simulate the physiological system’s Ca2+ kinetics by studying TnC in isolation, in the TnC–TnI complex, in Tn and in regulated thin filaments (Johnson et al., 1981, 1994; Luo et al., 2002; Davis et al., 2004; Shitaka et al., 2004, 2005). We have taken these studies a step further and closer to the physiological system by utilizing isolated rigor skeletal myofibrils. Skeletal myofibrils contain all the necessary contractile and regulatory proteins in the proper stoichiometry and geometry. Isolated myofibrils have been shown to contract and relax with kinetics similar to skinned and intact muscle fibers (Tesi et al., 2002; Poggesi et al., 2005).
In order to observe the N-terminal regulatory domain of TnC in myofibrils we have engineered a new fluorescent TnC labeled with two different environmentally sensitive fluorescent probes, IAANS or MIANS. Neither the two mutations (T54C and C101S) nor the extrinsic fluorescent labeling of TnC affected its biological activity. Furthermore, it was possible to follow both the N-terminal and C-terminal rates of Ca2+ dissociation from TnC using the different fluorescent TnC moieties in isolation, in the TnC–TnI complex and in myofibrils. Consistent with current thought, only the rate of Ca2+ dissociation from the N-terminal regulatory domain of TnC in rigor myofibrils is kinetically rapid enough to be physiologically relevant. Furthermore, this rate is quantitatively similar to the rate of skeletal muscle relaxation at ∼4 and 15°C and thus may influence the rate of skeletal muscle relaxation (Stephenson and Williams, 1981; Luo et al., 2002).
Materials and Methods
Materials
Phenyl-Sepharose CL-4B and EGTA were purchased from Sigma Chemical Co. (St. Louis, MO, USA). Quin-2 was purchased from Calbiochem (La Jolla, CA, USA). All other chemicals were of analytical grade.
Protein Mutagenesis and Purification
The chicken skeletal fast TnI protein (with native Cys residues mutated to Ser) and TnC plasmid were a generous gift from Dr. Lawrence B. Smillie (University of Alberta). The construction and expression of chicken skeletal TnC in pET3a has been described (Li et al., 1994). The TnCT54C,C101S protein was constructed from the TnC plasmid by primer based site-directed mutagenesis using Stratagene’s (La Jolla, CA, USA) Quik-Change Site-Directed Mutagenesis Kit. The mutations were confirmed by DNA sequence analysis. The plasmid for TnCT54C,C101S was transformed into E. Coli BL21(DE3)pLysS cells (Novagen), expressed and purified as previously described (Tikunova et al., 2002). Aliquots of TnC or TnCT54C,C101S were labeled with the environmentally sensitive thiol-reactive fluorescent probes 5-((((2-iodoacetyl)amino)ethyl)amino) naphthalene-1-sulfonic acid (IAEDANS), 2-(4′-(iodoacetamido)anilino) naphthalene-6-sulfonic acid (IAANS), or 2-(4′-maleimidylanilino)naphthalene-6-sulfonic acid (MIANS). The TnC constructs were reacted with three- to fivefold molar excess of the different fluorescent probes for ∼6 h at room temperature with constant shaking in 50 mM Tris, 90 mM KCl, 1 mM EGTA, 6 M urea, pH 7.5. The labeling reaction was stopped by addition of 2 mM DTT and the labeled proteins were exhaustively dialyzed against 10 mM MOPS, 90 mM KCl, pH 7.0 at 4°C to remove un-reacted label. On average, 71 ± 4% of the different TnC constructs were determined to be labeled with the various fluorescent probes under these conditions.
Determination of Ca2+ Affinities
All steady-state fluorescence measurements were performed using a Perkin-Elmer LS5 Spectrofluorimeter at 15°C. IAANS fluorescence was excited at 330 nm and monitored at 450 nm, whereas MIANS fluorescence was excited at 325 nm and monitored at 435 nm. Ca2+ titrations were performed by adding microliter amounts of CaCl2 to 1 ml of the fluorescent TnC constructs (0.6 (M) ± TnI (1.2 (M) in 200 mM MOPS (to prevent pH changes upon addition of Ca2+), 90 mM KCl, 2 mM EGTA, 1 mM Mg2+, 1 mM DTT, pH 7.0, at 15°C. The [Ca2+]free was calculated using the computer program EGCA02 developed by Robertson and Potter (1984). The Ca2+ affinities are reported as dissociation constants (Kd(Ca)). Each Kd(Ca) represents a mean ± SE of three to five titrations fit with a logistic sigmoid function mathematically equivalent to the Hill equation, as previously described (Tikunova et al., 2002).
Myofibril Preparation
All the animal protocols and procedures were performed in accordance with National Institutes of Health guidelines and approved by the Institutional Laboratory Animal Care and Use Committee at The Ohio State University. Rabbit skeletal psoas myofibrils in rigor were prepared, stored, and handled as previously described (Swartz et al., 1997; Davis et al., 2004). Endogenous TnC was extracted from a sample of the stock myofibrils by first washing the myofibrils three times in a myofibril TnC extraction solution (10 mM MOPS, 5 mM EDTA, 1 mM DTT, 0.02% Tween-20, pH 8.0) to remove any residual glycerol. Tween-20 was utilized as a surfactant to minimize myofibril aggregation. Bovine serum albumin was initially utilized instead of Tween-20, but was found to bind Ca2+ and interfere with the Quin-2 studies mentioned below. The myofibrils were then incubated in the TnC extraction solution for approximately 10 min at room temperature, pelleted and resuspended in fresh TnC extraction solution an additional three times each for 10 min to ensure that TnC was extracted from both the non-overlap and overlap zones of the sarcomere (Swartz et al., 1997). The TnC extracted myofibrils were then washed three times in 10 mM MOPS, 90 mM KCl, 1 mM Mg2+, 1 mM DTT, 0.02% Tween-20 at pH 7.0. The TnC extracted myofibrils were then exposed to 16 (M TnCIAEDANS, , or
for 30 min on ice. Free labeled TnC was removed from the myofibrils by washing the myofibrils three times in 10 mM MOPS, 90 mM KCl, 1 mM Mg2+, 1 mM DTT, 0.02% Tween-20 at pH 7.0. The myofibrils were then diluted in the same buffer to ∼1 mg/ml and filtered through a 100-μm pore diameter mesh to remove large pieces of muscle or myofibril aggregates. Prior to the stopped-flow experiments, 200 μM Ca2+ was added to the myofibrils. Samples of the reconstituted myofibrils were also plated on a glass slide with a cover slip and imaged as previously described (Swartz et al., 1997; Zhang et al., 2000; Davis et al., 2004). Briefly, the images were collected using a Zeiss Axiovert TV (Thornwood, NJ, USA) epifluorescence microscope equipped with a 100× oil-immersion phase contrast lens and a Chroma filter set #11000UV (360 nm broad excitation, 400 nm long pass dichroic, and 420 nm long pass emission). Digital images were obtained using a 12-bit intensity resolution CCD camera (Kodak KAF 1300 chip; Photometrics, Tucson, AZ, USA) controlled by a Matrox board and IPLab Spectrum software (v3.2.3, Signal Analytics, Vienna, VA, USA) run by a PowerMac 8100.
Determination of Ca2+ Dissociation Kinetics
Ca2+ dissociation kinetics were measured using an Applied Photophysics Ltd. (Leatherhead, UK) model SX.18 MV stopped-flow instrument with a dead time of 1.4 ms at 15°C. The fluorescently labeled TnC or TnCT54C,C101S ± TnI samples were excited using a 150-W xenon arc source (at 325, 330, or 340 nm for MIANS, IAANS, or IAEDANS, respectively) with emission monitored through a 420- to 470-nm band-pass filter (Oriel, Stratford, CT, USA). Direct Ca2+ dissociation rates were also measured from unlabeled TnC ± TnI using the fluorescent Ca2+ chelator Quin-2 (Tikunova et al., 2002; Davis et al., 2004). Quin-2 was excited at 330 nm with its emission monitored through a 510-nm broad band-pass interference filter (Oriel, Stratford, CT, USA). The buffer used for the solution stopped-flow experiments was 10 mM MOPS, 90 mM KCl, 1 mM Mg2+, 1 mM DTT, at pH 7.0. Since the myofibrils are a suspension prone to scatter artifacts, approximately one in six shots led to inconsistent fluorescent signals, a phenomenon not experienced with the solution studies. Each Ca2+ dissociation event represents an average of at least five traces collected and repeated 9 times for the isolated TnC and TnC–TnI complexes and 14 times for the myofibril studies. The data were fit with a single or double exponential equation after mixing was complete.
Muscle Fiber Experiments
Single fibers were isolated the day of use from bundles of rabbit psoas muscle that had been stored in a glycerinating solution at −20°C no longer than 1 month. Solutions and the mechanical setup utilized for force measurements were as previously described (Greaser et al., 1988). Briefly, a single fiber was soaked in relaxing solution containing 1% (v/v) Triton X-100 for 5 min to remove any residual sarcolemma and sarcoplasmic reticulum. The fiber was then attached to a servo-controlled DC torque motor (Cambridge Technologies, Watertown, MA, USA) and an isometric force transducer (model 403A, Cambridge Technologies, Watertown, MA, USA) by aluminum T-clips (Wahr et al., 1998). Resting sarcomere length was set between 2.50 and 2.60 (m. The fiber was then activated in a pCa 4.0 solution and rapidly slackened after isometric force reached a plateau. The analog output of the force transducer was digitized using a DaqBoard/2000 and Daqview software (Iotech Inc., Cleveland, OH, USA). The total force was measured between the plateau and baseline levels. The same procedure was utilized to obtain the resting force level of the fiber in a pCa 9.0 solution. The active force generated by the fiber in the various pCa solutions was calculated as the total force minus the resting force. Three active force measurements were performed in pCa 4.0 with the final activation taken as the maximal force generated by the native fiber (i.e., prior to extraction of endogenous TnC) which led to an average force per cross sectional area of 73 ± 7 kN/m2. The fiber was then exposed for 2 min to a TnC extraction solution containing 5 mM EDTA, 10 mM HEPES and 0.5 mM trifluoperazine dihydrochloride (TFP; used to enhance the rate of TnC extraction) at pH 7.0 (Metzger et al., 1989). The fiber was then washed three times in pCa 9.0 solution to remove residual TFP. TFP was not used with the myofibril studies since TFP is fluorescent and we were concerned that trace amounts remaining after washing the myofibrils could compromise the IAANS or MIANS fluorescent signals (Martyn et al., 1999). If the residual force in pCa 4.0 solution was >10% of the maximal force the extraction process was repeated. The fibers were then incubated for 2 min in a pCa 9.0 solution containing 16.7 (M recombinant TnCT54C,C101S or . All of the reconstituted fibers were then exposed to a series of pCa solutions varying from pCa 9.0 to 4.0 and the active force versus pCa was measured. Every fourth activation was performed at pCa 4.0 to which each adjacent and randomized pCa were normalized. Each data point represents the mean ± SE from at least four separate fibers individually normalized and fit with a logistic sigmoid function mathematically equivalent to the Hill equation. The solutions for skinned fiber experiments were prepared as previously described (Norman et al., 2007). Large batches of pCa 9.0 and pCa 4.0 solutions were divided into aliquots and stored at -80°C. These aliquots were thawed and mixed to make intermediate-pCa solutions, which were stored at 4°C and used within a week.
Results
Characterization of the Ca2+ Dissociation Rates from TnCIAEDANS in Isolation, in the Presence of TnI and in Myofibrils
Chicken skeletal TnC contains a single, C-terminal Cys residue at position 101 (Golosinska et al., 1991). Previously we have shown that this residue can be labeled with an environmentally sensitive fluorophore, TnCIAEDANS, and observed in reconstituted rabbit psoas myofibrils (Davis et al., 2004). We hypothesized that it would be possible to take advantage of the fluorescence signal of TnCIAEDANS to follow the rate of Ca2+ dissociation from the C-terminal structural domain of TnC in myofibrils.
As Ca2+ dissociated from isolated TnCIAEDANS, the fluorescent signal decayed at a rate of 0.37 ± 0.03/s at 15°C (Figure 1A), which was similar to the rate of Ca2+ dissociation from the C-terminal sites of TnCF29W (0.48 ± 0.01/s) as measured by the fluorescent Ca2+ chelator Quin-2 (Davis et al., 2002). We have previously shown that addition of TnI to TnCF29W slowed the rate of Ca2+ dissociation from the C-terminal sites of TnCF29W approximately fourfold as measured by Quin-2 at 15°C (Davis et al., 2002). Likewise, the dissociation of Ca2+ from the TnCIAEDANS–TnI complex decreased the rate of fluorescence decay approximately fivefold (Figure 1A). Figure 1A also shows that the rate of fluorescence decay of TnCIAEDANS (0.07 ± 0.02/s) reconstituted into rabbit psoas skeletal myofibrils (in rigor) was identical to that of the TnCIAEDANS–TnI complex (0.07 ± 0.01/s; Figure 1A). Figure 1B demonstrates that TnCIAEDANS incorporated into the myofibrils and that its fluorescence originated from the location of the thin filaments. Based on the phase contrast images, the average sarcomere length of the myofibrils was 2.5 ± 0.1 μm. Thus, Ca2+ dissociation from the C-terminal EF-hands of TnC can be followed by TnCIAEDANS in the myofibrils and consistent with the solution studies, is too slow to be rate-limiting for fast-twitch skeletal muscle relaxation (∼4 and 17/s at ∼4 and 15°C, respectively; Johnson et al., 1981; Stephenson and Williams, 1981; Luo et al., 2002).
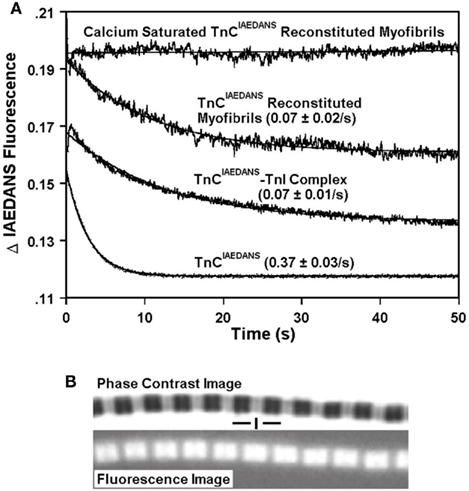
Figure 1. Characterization of the Ca2+ dissociation rates from TnCIAEDANS in isolation, in the presence of TnI and in myofibrils. (A) Shows the time course of decrease in IAEDANS fluorescence as Ca2+ was removed by EGTA from the C-terminal Ca2+-binding sites of TnCIAEDANS ± TnI and in reconstituted myofibrils. TnCIAEDANS (0.6 μM) ± TnI (1.2 μM) in 10 mM MOPS, 90 mM KCl, 1 mM Mg2+, 1 mM DTT at pH 7.0 plus 200 μM Ca2+ was rapidly mixed with an equal volume of the same buffer plus 10 mM EGTA at 15°C. Similar experiments were performed with ∼1 mg/ml reconstituted myofibrils except the buffer also contained 0.02% Tween-20. The flat line represents the fluorescence level of Ca2+ saturated myofibrils in which EGTA in the second syringe was replaced with 200 μM Ca2+. IAEDANS emission fluorescence was monitored through a 420- to 470-nm band-pass filter with excitation at 340 nm. The TnCIAEDANS and TnCIAEDANS–TnI complex traces have been normalized to that of the TnCIAEDANS reconstituted myofibrils trace and staggered for visual clarity. (B) Shows the phase contrast (top panel) and IAEDANS fluorescence (bottom panel) images obtained from a representative rigor psoas myofibril reconstituted with TnCIAEDANS. The vertical line designates the location of the Z-line, and the horizontal lines designate the location of the A bands.
Calcium Binding to
or 
The question still remained if Ca2+ dissociation from the N-terminal Ca2+ binding sites of TnC in myofibrils occurs with a rate that could influence the rate of muscle relaxation. We have previously demonstrated that the rate of Ca2+ dissociation from the N-terminal, regulatory Ca2+ binding sites of the TnCF29W–TnI complex was slow enough (∼11/s) to potentially be rate-limiting for fast-twitch muscle relaxation and may be a good model system for the rate of Ca2+ dissociation from TnC in muscle (Davis et al., 2002, 2004). It was not possible to utilize TnCF29W fluorescence in myofibrils due to contaminating Trp signals from numerous other proteins. Reconstituted myofibrils with TnCF29W(5OH) (a Trp analog with distinct spectral properties; Valencia et al., 2003) gave signals that were too noisy to utilize (data not shown), possibly due to the fact that this fluorescent probe has a small Stoke’s shift, making it more prone to scatter artifacts. Additionally, even though TnCdanz fluorescence has been utilized in skinned fibers (Guth and Potter, 1987; Morano and Ruegg, 1991), the signal was also too noisy and small to reliably measure the Ca2+ dissociation rates from TnCdanz reconstituted myofibrils in the stopped-flow apparatus (data not shown).
We engineered a novel fluorescent TnC that would follow the rate of Ca2+ dissociation from the regulatory sites of TnC with high fidelity and had a fluorescent signal that could be distinguished and measured in the myofibril. Figure 2A shows a cartoon representation of the movement of Thr 54 (T54) as TnC binds Ca2+ ± TnI. T54 is located at the junction of the B and C helices in the N-terminal of TnC and is not involved in direct ligation of Ca2+ nor does it appear to interact with TnI based on the crystal structure of the Ca2+ saturated regulatory domain of the Tn complex (however, in the Ca2+-free N-terminal Tn structure T54 comes in close contact with Arg 112 in TnI; Vinogradova et al., 2005). Thus, we predicted that the conservative mutation of T54 to Cys (with the endogenous Cys 101 mutated to Ser) labeled with an environmentally sensitive fluorophore would create an appropriate fluorescent TnC to measure Ca2+ dissociation in myofibrils.
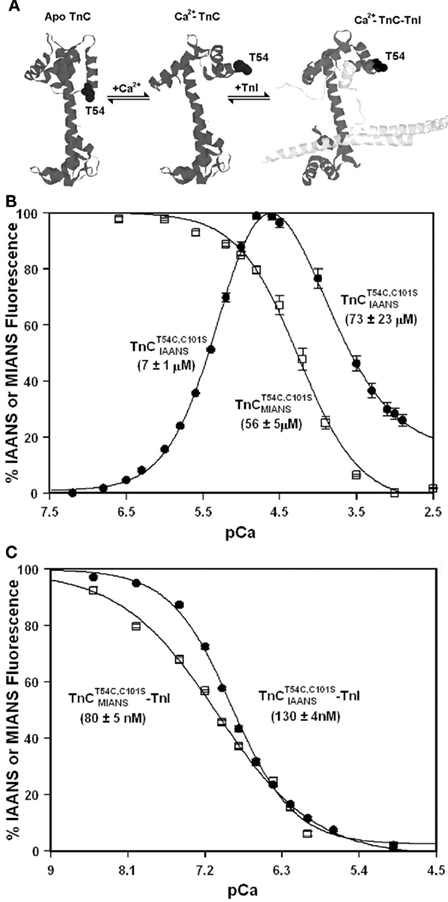
Figure 2. Calcium binding to
(A) Shows a helical representation of apo TnC and Ca2+ saturated TnC ± TnI with T54 depicted in space fill format. The Protein Data Bank files utilized for this figure were 1TOP (apo TnC; Satyshur et al., 1994), 2TN4 (Ca2+–TnC; Houdusse et al., 1997), and 1YTZ (Ca2+–TnC–TnI, TnT is not shown for clarity; Vinogradova et al., 2005) and were rendered using Rasmol (Sayle and Milner-White, 1995). (B) Shows the Ca2+ dependent increase and decrease in IAANS fluorescence for
(●) and the Ca2+ dependent decrease in MIANS fluorescence for
(□) as a function of pCa.
fluorescence was fit with the sum of two independent Hill equations. (C) Shows the Ca2+ dependent decrease in IAANS and MIANS fluorescence for the
–TnI (●) and
–TnI (□) complexes as a function of pCa. Microliter amounts of Ca2+ were added to the different fluorescent TnC constructs (0.6 μM) ± TnI (1.2 μM) in 200 mM MOPS, 90 mM KCl, 2 mM EGTA, 1 mM Mg2+, 1 mM DTT, pH 7.0, at 15°C. IAANS fluorescence emission was monitored at 450 nm with excitation at 330 nm, whereas MIANS fluorescence emission was monitored at 435 nm with excitation at 325 nm. Hundred percentage fluorescence corresponds to the highest fluorescent state whereas 0% fluorescence corresponds to the lowest fluorescent state of the two respective conditions (±TnI).
Figure 2B shows that the fluorescence of increases upon Ca2+ binding with a Kd(Ca) of 7 ± 1 μM, which was close to that of TnCF29W at 15°C (Davis et al., 2002; Tikunova et al., 2002). However, unlike TnCF29W fluorescence, Ca2+ concentrations in excess of 16 μM caused the fluorescence of isolated
to decrease with a Kd(Ca) of 73 ± 23 μM. The Hill coefficients of these fluorescent changes were near unity (1.2 ± 0.2 and 0.9 ± 0.2 for the increase and decrease phases, respectively) potentially implying the binding event of two independent Ca2+ ions to the regulatory domain of TnC. This biphasic pattern of Ca2+ binding to
is consistent with a mechanism of sequential Ca2+ binding to the two N-terminal EF-hands of TnC, as was observed with wild type TnC by NMR, CD spectroscopy, and flow dialysis (Li et al., 1995; Pearlstone et al., 2000; Valencia et al., 2003). The calculated Kd(Ca) values for
have relatively large errors and may under- or over-estimate the actual Kd(Ca) values. The error does not arise from irreproducible data but from the fact that there was no unique solution to the curve fit since we were unable to determine the true amplitudes of the fluorescent changes. Interestingly,
fluorescence only decreased upon the addition of Ca2+ with a Kd(Ca) of 56 ± 5 μM (Figure 2B). This low Kd(Ca) for
falls within the range observed by NMR for the second Ca2+ binding to the N-terminal of TnC, potentially at site I (Li et al., 1995; Pearlstone et al., 2000; Valencia et al., 2003). Thus, IAANS fluorescence was sensitive to both N-terminal Ca2+ binding sites, whereas MIANS fluorescence was only sensitive to the second, low affinity binding site.
Unlike isolated , the
–TnI complex displayed only a monophasic decrease in fluorescence upon Ca2+ binding with a Kd(Ca) of 130 ± 4 nM (Figure 2C), a value similar to that of the TnCF29W–TnI complex (Ramos, 1999; Davis et al., 2002, 2004). Thus, TnI enhanced the Ca2+ binding sensitivity of
∼31- to 1338-fold. Likewise, the
–TnI complex also displayed a monophasic decrease in fluorescence upon Ca2+ binding with a Kd(Ca) of 80 ± 5 nM (Figure 2C). Thus, TnI enhanced the Ca2+ binding sensitivity of
∼700-fold. It is not known if these fluorescent changes sense only one of the N-terminal Ca2+ binding sites or if both sites now bind Ca2+ with a similar affinity in the presence of TnI. The Hill coefficients for the
–TnI and
–TnI complexes were 1.20 ± 0.06 and 0.76 ± 0.06, respectively, which might suggest these fluorescent changes were monitoring a single Ca2+ binding site. However, interpretation of Hill coefficients based on fluorescence changes as a measure of number of Ca2+ binding sites or cooperativity must be taken with caution (Valencia et al., 2003). In any regard, the fluorescence of
and
were sensitive to Ca2+ binding in the presence and absence of TnI and appeared to report the Ca2+ binding properties of the N-terminal regulatory domain of TnC. Even though the fluorescent probes are located on the N-terminal domain of TnC, it may be that these apparent N-terminal Ca2+ affinities are affected by Ca2+ binding or Mg2+ exchange with the C-terminal EF-hands of TnC.
Comparison of the Calcium Dependence of Skeletal Muscle Force Generation by Native and TnC Reconstituted Fibers
Before the kinetics of Ca2+ dissociation from was addressed, we verified that this TnC construct was biologically active. TnCT54C,C101S and
were individually reconstituted into single, rabbit skinned psoas fibers to test the biological function, force versus pCa relationship (Figure 3). The endogenous TnC (TnCEndog) fibers exhibited a Ca2+ dependent increase in force development with a [Ca2+]50 of 1.1 ± 0.1 μM and a Hill coefficient of 3.5 ± 0.8 (Figure 3). After TnCEndog extraction, the average force generated by the single skinned muscle fibers was 3 ± 2% of the maximal force (data not shown). Subsequent reconstitution of the muscle fibers with TnCT54C,C101S or
recovered 90 ± 7 or 87 ± 6% of the maximal force at pCa 4, respectively. Figure 3 demonstrates that the Ca2+ dependence of force development controlled by TnCT54C,C101S or
was similar to that controlled by TnCEndog. Furthermore, the inset of Figure 3 demonstrates that
or
incorporated into isolated myofibrils and that their fluorescence originated from the location of the thin filaments. Thus, both TnCT54C,C101S and
possessed nearly identical biological activity to that observed for TnCEndog judged by the Ca2+ sensitivity of force development and extent of force recovery.
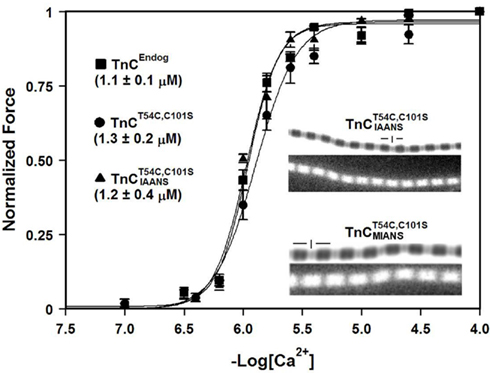
Figure 3. Comparison of the calcium dependence of skeletal muscle force generation by native and TnC reconstituted fibers. The Ca2+ dependence of isometric force generation in native (■ TnCEndog) single rabbit skinned psoas fibers is compared to that of fibers reconstituted with TnCT54C,C101S (●) or (▲) as a function of pCa. The experimental conditions are described in “Experimental Procedures.” Each data point represents the mean ± SE from at least four separate fibers individually normalized and fit with a logistic sigmoid function mathematically equivalent to the Hill equation. The inset shows the phase contrast (top of paired panels) and IAANS or MIANS fluorescence (bottom of paired panels) images obtained from representative rigor psoas myofibrils reconstituted with
or
. The vertical lines designate the location of the Z-lines, and the horizontal lines designate the location of the A bands.
Comparison of Calcium Dissociation Kinetics from Isolated
, TnCT54C,C101S, and 
Figure 4A shows that as Ca2+ dissociated from isolated at 15°C, its fluorescence decreased in a biphasic manner with rates of 934 ± 61 and 0.348 ± 0.002/s, with the slow rate comprising only ∼5 ± 1% of the total signal. Figure 4B shows that this biphasic fluorescence decrease corresponded to that of the fast rate being associated with Ca2+ dissociation from at least one of the N-terminal sites (>1000/s) and the slow rate being associated with Ca2+ dissociation from the C-terminal sites (0.344 ± 0.003/s) as measured from unlabeled TnCT54C,C101S using Quin-2 (data summarized in Table 1). A similar conclusion was reached upon comparison of the Quin-2 Ca2+ dissociation rates from TnCT54C,C101S and the fluorescent kinetic changes of
at 4°C (Figures 4A,B and Table 1). The low Ca2+ affinity associated decrease in
fluorescence observed in Figure 2B was confirmed in the stopped-flow apparatus by modulating the Ca2+ saturation state of the labeled protein (data not shown). However, as EGTA removed Ca2+ from
at both 4 and 15°C the kinetic increase in fluorescence was too fast to observe (>1000/s). Furthermore, the relatively small amplitude C-terminal associated fluorescent kinetic decrease of
was not distinguished in the Ca2+ titration shown in Figure 2B, likely due to this signal change being overwhelmed by the large N-terminal associated increase in fluorescence.
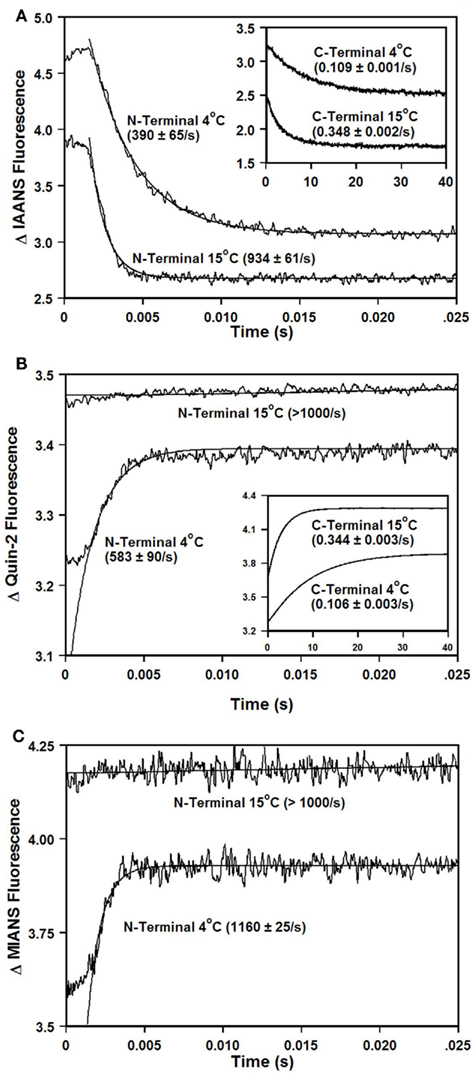
Figure 4. Comparison of calcium dissociation kinetics from isolated , TnCT54C,C101S, and
. (A) Shows the time course of decrease in IAANS fluorescence as Ca2+ was removed by EGTA from the N-terminal regulatory and C-terminal structural [(A) inset] Ca2+-binding sites of
.
(0.6 μM) in 10 mM MOPS, 90 mM KCl, 1 mM Mg2+, 1 mM DTT at pH 7.0 plus 200 μM Ca2+ was rapidly mixed with an equal volume of the same buffer plus 10 mM EGTA at 4 and 15°C. IAANS emission fluorescence was monitored through a 420- to 470-nm band-pass filter with excitation at 330 nm. (B) Shows the time course of increase in Quin-2 fluorescence as Ca2+ was removed from the N-terminal regulatory and C-terminal structural [(B) inset] Ca2+-binding sites of TnCT54C,C101S by Quin-2. TnCT54C,C101S (6 μM) in 10 mM MOPS, 90 mM KCl, 1 mM Mg2+, 1 mM DTT at pH 7.0 plus 60 μM Ca2+ was rapidly mixed with an equal volume of the same buffer plus 150 μM Quin-2 at 4 and 15°C. Control experiments where Ca2+ (60 μM) was rapidly mixed with an equal volume of Quin-2 (150 μM) were flat lines. Quin-2 emission fluorescence was monitored through a 510-nm broad band-pass interference filter with excitation at 330 nm. (C) Shows the time course of increase in MIANS fluorescence as Ca2+ was removed by EGTA from the N-terminal regulatory Ca2+-binding sites of
.
(0.6 μM) in 10 mM MOPS, 90 mM KCl, 1 mM Mg2+, 1 mM DTT at pH 7.0 plus 200 μM Ca2+ was rapidly mixed with an equal volume of the same buffer plus 10 mM EGTA at 4 and 15°C. MIANS emission fluorescence was monitored through a 420- to 470-nm band-pass filter with excitation at 325 nm. The traces in (A) through (C) have been staggered for visual clarity.
Figure 4C shows that when TnCT54C,C101S was labeled with the environmentally sensitive fluorescent probe MIANS, only the rapid N-terminal Ca2+ dissociation rate was observed at 4°C (see also Table 1). Thus, the rates of Ca2+ dissociation from the N- and C-terminal domains of TnCT54C,C101S were not drastically altered by fluorescent labeling with IAANS or MIANS.
Comparison of Calcium Dissociation Kinetics from
,
, and TnCT54C,C101S in the Presence of TnI
Addition of TnI to slowed the rate of Ca2+ dissociation from the N-terminal domain at least ∼106-fold, whereas the rate of Ca2+ dissociation from the C-terminal domain was slowed by TnI only ∼5.8-fold at 15°C (Figure 5A and Table 2). The slowing of the C-terminal rate by TnI was consistent with that observed with TnCIAEDANS (see Table 2). Figure 5B demonstrates that as Ca2+ was removed from the
–TnI complex the fluorescence increased at a rate similar to the N-terminal rate observed with the
–TnI complex at both 4 and 15°C (Figure 5A; see also Table 2). These N-terminal and C-terminal rates were similar to the actual rate of Ca2+ dissociation from the TnCT54C,C101S–TnI complex measured at 4 and 15°C using Quin-2 (Figure 5C and Table 2). Thus, in the presence and absence of TnI,
fluorescence followed Ca2+ dissociation from both the N- and C-terminal sites of TnC, whereas
fluorescence was only sensitive to N-terminal Ca2+ dissociation. Since both of these fluorescent TnC constructs report Ca2+ dissociation events from TnC ± TnI with relatively high fidelity, it lends credence to the suggestion that these probes will do the same when incorporated into myofibrils.
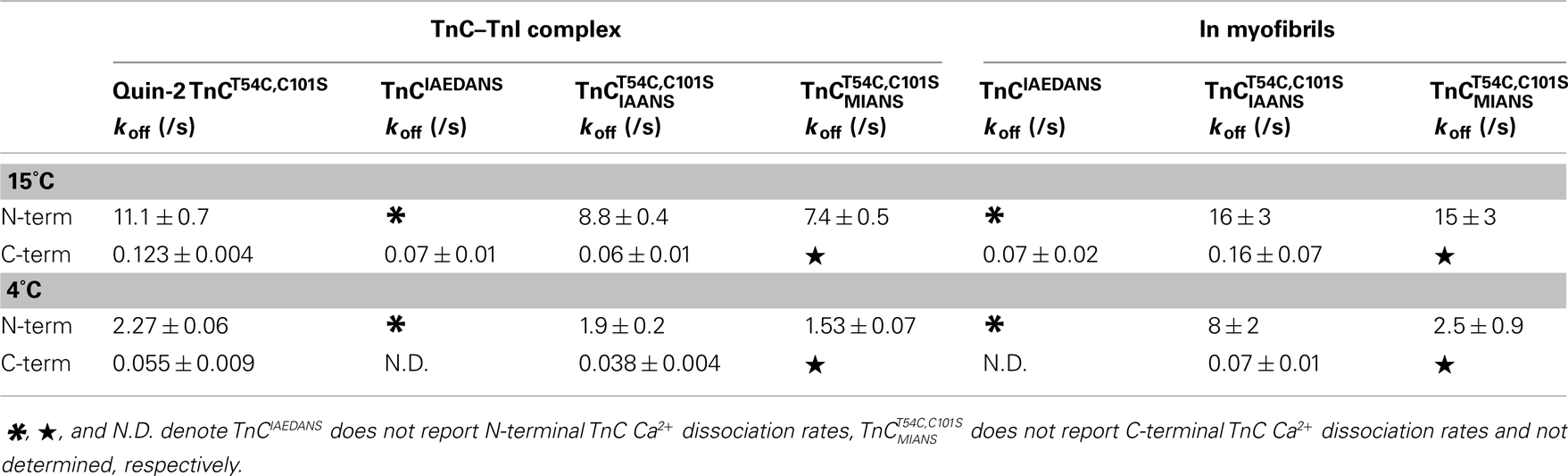
Table 2. Comparison of calcium dissociation kinetics from the different TnC constructs in the presence of TnI and myofibrils.
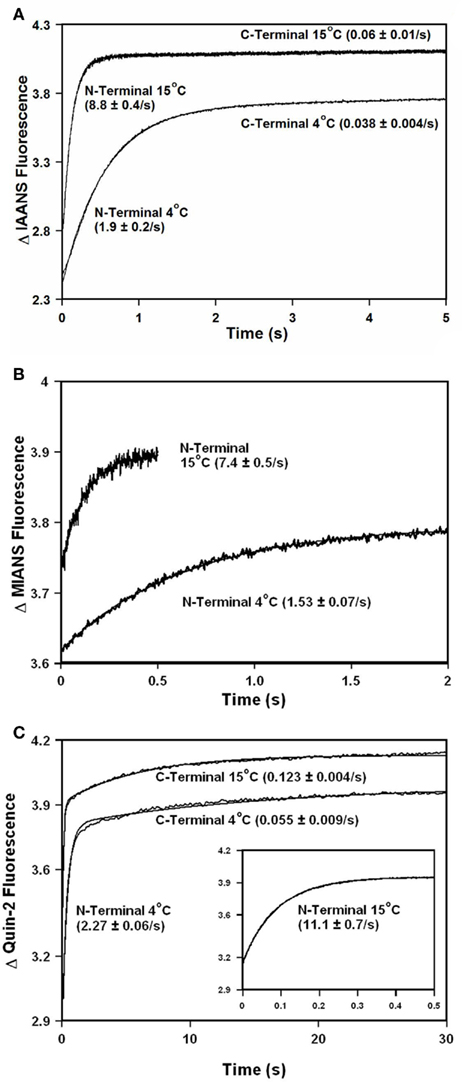
Figure 5. Comparison of calcium dissociation kinetics from ,
, and TnCT54C,C101S in the presence of TnI. (A) Shows the time course of increase in IAANS fluorescence as Ca2+ was removed by EGTA from the N-terminal regulatory and C-terminal structural Ca2+-binding sites of the
–TnI complex.
(0.6 μM) plus TnI (1.2 μM) in 10 mM MOPS, 90 mM KCl, 1 mM Mg2+, 1 mM DTT at pH 7.0 plus 200 μM Ca2+ was rapidly mixed with an equal volume of the same buffer plus 10 mM EGTA at 4 and 15°C. IAANS emission fluorescence was monitored as described in the legend of Figure 4A. (B) Shows the time course of increase in MIANS fluorescence as Ca2+ was removed by EGTA from the N-terminal regulatory Ca2+-binding sites of the
–TnI complex.
(0.6 μM) plus TnI (1.2 μM) in 10 mM MOPS, 90 mM KCl, 1 mM Mg2+, 1 mM DTT at pH 7.0 plus 200 μM Ca2+ was rapidly mixed with an equal volume of the same buffer plus 10 mM EGTA at 4 and 15°C. MIANS emission fluorescence was monitored as described in the legend of Figure 4C. (C) Shows the time course of increase in Quin-2 fluorescence as Ca2+ was removed from the N-terminal regulatory [(C) inset shows expanded 15°C trace] and C-terminal structural Ca2+-binding sites of the TnCT54C,C101S–TnI complex by Quin-2. TnCT54C,C101S (6 μM) plus TnI (9 μM) in 10 mM MOPS, 90 mM KCl, 1 mM Mg2+, 1 mM DTT at pH 7.0 plus ∼30 μM Ca2+ was rapidly mixed with an equal volume of the same buffer plus 150 μM Quin-2 at 4 and 15°C. Control experiments where Ca2+ (30 μM) was rapidly mixed with an equal volume of Quin-2 (150 μM) were flat lines. Quin-2 emission fluorescence was monitored as described in the legend of Figure 4B. The traces in (A) through (C) have been staggered for visual clarity.
Comparison of Calcium Dissociation Kinetics from
and
Reconstituted Myofibrils
Figure 6A shows that as Ca2+ was dissociated from reconstituted myofibrils at 15°C the fluorescence decreased in a biphasic manner with rates of 16 ± 3 and 0.16 ± 0.07/s with the slow rate comprising 45 ± 19% of the total signal. The amplitude of the fast phase began at the level of the Ca2+ saturated myofibrils reconstituted with
, which was a flat line indicating that no change in fluorescence was observed until Ca2+ was removed from the myofibrils and that a faster third phase was not present (Figure 6A). However, rigorous verification of the latter point can not be made since there was an ∼50 ms scatter artifact preceding the data collection making measurements of any rapid kinetic change impossible. Furthermore, slight changes in ionic strength and pH caused by the EGTA buffer may have also influenced the fluorescence signal. The initial scatter artifact was likely due to the myofibrils being a suspension of protein filaments, rather than a simple solution. Decreasing the temperature of the system to 4°C slowed both fast and slow rates approximately twofold (Figure 6A). The biphasic nature of the fluorescence decrease at both temperatures was consistent with that measured from isolated
and the
–TnI complex (Table 2). Both kinetic phases from the myofibrils were approximately twofold faster than those observed in the
–TnI complex (Table 2).
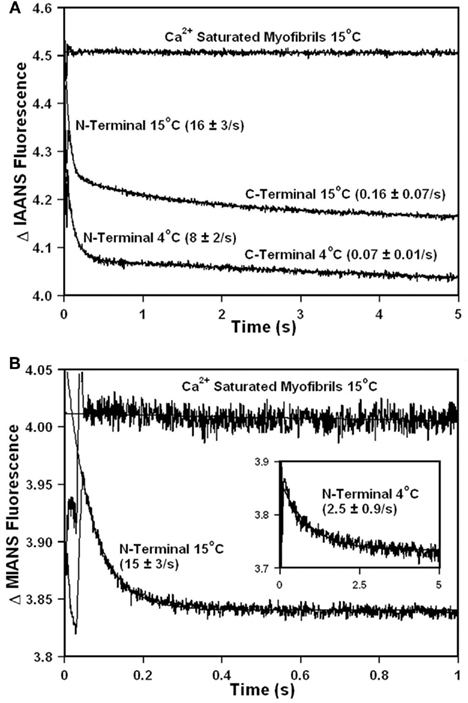
Figure 6. Comparison of calcium dissociation kinetics from and
reconstituted myofibrils. (A) Shows the time course of decrease in IAANS fluorescence as Ca2+ was removed by EGTA from the N-terminal regulatory and C-terminal structural Ca2+-binding sites of
reconstituted rigor rabbit psoas myofibrils. ∼1 mg/ml
reconstituted myofibrils in 10 mM MOPS, 90 mM KCl, 1 mM Mg2+, 1 mM DTT, 0.02% Tween-20 at pH 7.0 plus 200 μM Ca2+ was rapidly mixed with an equal volume of the same buffer plus 10 mM EGTA at 4 and 15°C. The flat line represents the 15°C fluorescence level of Ca2+ saturated myofibrils in which EGTA in the second syringe was replaced with 200 μM Ca2+. IAANS emission fluorescence was monitored as described in the legend of Figure 4A. (B) Shows the time course of decrease in MIANS fluorescence as Ca2+ was removed by EGTA from the N-terminal regulatory Ca2+-binding sites of
reconstituted rigor rabbit psoas myofibrils. ∼1 mg/ml
reconstituted myofibrils in 10 mM MOPS, 90 mM KCl, 1 mM Mg2+, 1 mM DTT, 0.02% Tween-20 at pH 7.0 plus 200 μM Ca2+ was rapidly mixed with an equal volume of the same buffer plus 10 mM EGTA at 4 [(B) inset] and 15°C. MIANS emission fluorescence was monitored as described in the legend of Figure 4C. The 4°C trace in (A) has been staggered for visual clarity.
Upon Ca2+ removal from reconstituted myofibrils at 15°C, the fluorescence decreased in a monophasic manner at a rate of 15 ± 3/s, which was similar to the fast phase observed with
reconstituted myofibrils (Figure 6B; Table 2). The amplitude of the mono-exponential fluorescence decay began at approximately the fluorescence level of the Ca2+ saturated myofibrils, again indicating that no faster second rate was present. Furthermore, decreasing the temperature of the system to 4°C slowed the monophasic rate of
fluorescence approximately sixfold, consistent with the same temperature decrease slowing the rate of the
–TnI complex ∼4.8-fold (Inset of Figure 6B and Table 2). The monophasic nature of the fluorescence decrease at both temperatures was consistent with that measured from isolated
and the
–TnI complex (Table 2). At 15°C, the myofibril kinetic rate was approximately twofold faster than that measured from the
–TnI complex, whereas at 4°C the two rates were nearly identical (Table 2). Thus, in the reconstituted myofibrils,
fluorescence followed Ca2+ dissociation from both the N- and C-terminal sites of TnC, whereas
and TnCIAEDANS fluorescence was only sensitive to N- and C-terminal Ca2+ dissociation, respectively.
It was not possible to determine the actual Ca2+ dissociation rates from TnC in the myofibrils using Quin-2. Quin-2 reported multiple rates from the myofibrils ranging from 400 to 0.04/s (data not shown). Accurate estimates of the different rates measured by Quin-2 were not feasible due to the complexity of the fluorescent signal. Not surprisingly, the data suggests there are additional Ca2+ binding proteins in the myofibril besides TnC from which Quin-2 non-discriminately removed Ca2+. Consistent with this interpretation, electron probe X-ray microanalysis of Ca2+ binding to skeletal fibers at high [Ca2+]free ((pCa 5.5) showed Ca2+ binding to the thin filaments (TnC), thick filaments (possibly myosin light chains), and M-lines (unknown origin; Cantino et al., 1993, 1998).
All the myofibril experiments in this study were performed under rigor conditions. The addition of ATP (1 mM) to the myofibrils in the presence of Ca2+ caused the myofibrils to irreversibly hypercontract (under a light microscope the myofibrils shortened so extensively that they were almost not visible – data not shown). The strong cross-bridge inhibitors, BDM (up to 20 mM) and BTS (up to 200 μM), were able to inhibit the hypercontraction of the myofibrils in the presence of Ca2+ and ATP, but the myofibrils still significantly shortened and aggregated (data not shown). Since these myofibrils were not under any passive force, it might be that dissociation of rigor cross-bridges or cycling of only a few active cross-bridges cause shortening of the myofibrils. Alternatively, these compounds may block the cross-bridge cycle at a step that occurs only after the power stroke (Shaw et al., 2003). In any regard, it is unknown why the myofibrils shorten and aggregate in the presence of the strong cross-bridge inhibitors.
Discussion
Since it was not possible to distinguish the true rate of Ca2+ dissociation from TnC in the myofibrils using Quin-2 (due to the presence of numerous other Ca2+ binding proteins contaminating the signal), a fluorescent TnC had to be utilized. There are always concerns that intrinsic or extrinsic labeling of a protein will substantially modify the parameter one wants to measure. This was definitely the case for measuring the Ca2+ sensitivity and rate of Ca2+ dissociation from isolated TnCF29W, which increased the Kd(Ca) approximately sixfold and slowed the rate of Ca2+ dissociation from the N-terminal regulatory domain of TnC > 3-fold compared to wild type TnC (Davis et al., 2002; Valencia et al., 2003). However, the rate of Ca2+ dissociation from the N-terminal regulatory domain of the TnCF29W–TnI complex was nearly identical to that of the wild type complex (Davis et al., 2002). Thus, the F29W mutation affected the rate of Ca2+ dissociation from TnC in isolation, but not when complexed with TnI or its peptide, TnI96–148 (Davis et al., 2002, 2004).
The evidence that the extrinsically labeled IAEDANS, IAANS, and MIANS TnC moieties accurately report Ca2+ dissociation from the structural and regulatory domains of TnC in isolation and in the TnC–TnI complex is as follows: (1) the structural changes reported by the fluorescent TnCs occurred at the N- and C-terminal Ca2+ dissociation rates in isolation and in the TnC–TnI complex as verified by Quin-2 from the unlabeled TnC constructs; (2) the different fluorescent TnCs reported similar kinetic changes from the N- and C-terminal domains of TnC in isolation and in the TnC–TnI complex; (3) the Ca2+ dependent fluorescence changes in isolated and
were consistent with sequential Ca2+ binding as observed with wild type TnC (Li et al., 1995; Pearlstone et al., 2000; Valencia et al., 2003); and (4) the Ca2+ sensitivities of the
–TnI and
–TnI complexes were within the range reported for the TnCF29W–TnI96–148 complex (Davis et al., 2002, 2004), TnCF29W–TnI complex, and TnC–TnI complexes (containing different fluorescent TnIs; Oliveira and Reinach, 2003).
The circumstantial evidence that the extrinsically labeled fluorescent TnC moieties accurately report Ca2+ dissociation from the structural and regulatory domains of TnC in the myofibrils is as follows: (1) the different fluorescent probes accurately reported the Ca2+ binding and dissociation rates in isolation and the TnC–TnI complex (see above); (2) the different fluorescent TnCs reported similar kinetic changes from the N- and C-terminal domains of TnC in the myofibrils; (3) decreasing the experimental temperature had quantitatively similar effects on the kinetics measured from the TnC–TnI complexes and from the myofibrils; and (4) TnCT54C,C101S and recovered near maximal forces with similar [Ca2+]50 and Hill coefficients as observed with the endogenous fibers.
The Ca2+ dissociation rate from TnCIAEDANS supported the hypothesis that the rate of Ca2+ dissociation from the C-terminal structural domain of TnC in the myofibrils, and presumably muscle, is too slow to control relaxation. However, TnCIAEDANS fluorescence did not report the N-terminal regulatory domain Ca2+ kinetics. This fluorescent TnC did allow us to have confidence that was at least measuring the C-terminal structural domain Ca2+ dissociation events with relatively high fidelity in the myofibrils.
The similarity of the N-terminal Ca2+ dissociation rates measured with and
in the myofibrils is important considering that MIANS only reported the N-terminal structural changes. It may be that MIANS is a slightly less environmentally sensitive probe compared to IAANS, so that even though the same structural changes occur in both systems, only IAANS was able to measure the C-terminal associated events. In all,
appeared to have the highest fidelity of N-terminal Ca2+ dissociation kinetics across the systems studied as compared to the wild type (Davis et al., 2002) and unlabeled systems. Furthermore,
reported N-terminal Ca2+ dissociation rates in the myofibrils at 4 and 15°C that were close to those observed from the
–TnI complex. These results support the hypothesis that the TnC–TnI complex is the simplest biochemical model system for Ca2+ dissociation from TnC in skeletal muscle (Davis et al., 2002, 2004).
The reason for the approximately twofold faster rates of Ca2+ dissociation from the regulatory N-domain of and
at 15°C in the reconstituted myofibrils as compared to their respective TnC–TnI complexes is not known. It may be that addition of troponin T (TnT) to the TnC–TnI complex may slightly increase the rate of Ca2+ dissociation from the regulatory N-domain of TnC as was observed with the human slow skeletal Tn system (Gomes et al., 2004). Alternatively, other thin filament proteins such as actin and tropomyosin may also modulate the rate of Ca2+ dissociation from Tn in the myofibril. Consistent with this hypothesis, a thin filament system reconstituted with fluorescently labeled IANBD skeletal Tn (primarily labeled on TnI) exhibited an ∼10-fold faster rate of structural change as Ca2+ dissociated from the regulatory domain of TnC as compared to the isolated fluorescent Tn (Rosenfeld and Taylor, 1985). Interestingly, the same paper showed that addition of myosin subfragment 1 to the thin filament system in the absence of ATP decreased the rate of structural change as Ca2+ dissociated from the N-terminal regulatory domain of TnC ∼10-fold, back to that of the labeled Tn complex. The rate of structural change as Ca2+ dissociated from the N-terminal regulatory domain of TnC on the thin filaments in the presence of myosin subfragment 1 (∼1.6 and 15–20/s at 4 and 20°C, respectively; Rosenfeld and Taylor, 1985) is consistent with the Ca2+ dissociation rates we observed in the rigor myofibrils at 4 and 15°C. It would appear that the structural changes in the Tn complex are controlled by Ca2+ dissociation as was observed in FRET studies of regulated thin filaments (Shitaka et al., 2004).
However, according to the thin filament results discussed above, one would have expected biphasic N-terminal kinetics from the fluorescent TnC constructs in the myofibrils since some of the reconstituted Tn complexes are in the A-band whereas other Tn complexes are in the I band (locations of thick and thin overlap and non-overlap, respectively). Based on thin (∼1.1 μm) and thick (∼0.8 μm) filament lengths in the half sarcomere of rabbit psoas muscle (Morimoto and Harrington, 1973; Fowler, 1996, and references within), at a sarcomere length of ∼2.5 μm, ∼60% of the thin filaments would be overlapped by the thick filaments. Thus, nearly 40% of the thin filament would not have a rigor cross-bridge bound near a Tn complex. In this region of non-overlap Ca2+ dissociation from the N-terminal regulatory sites of TnC should have been very fast according to the thin filament study (Rosenfeld and Taylor, 1985). We can not necessarily rule out this possibility since an ∼10-fold faster rate could not be observed in the myofibril suspensions due to the initial large scatter and/or mixing time artifact. However, based on the amplitude of the N-terminal rate at both 4 and 15°C in the myofibrils beginning at the Ca2+ saturated state, we do not believe this is the case. However, slight changes in ionic strength and pH caused by the EGTA buffer may have influenced the intensity of the fluorescence signal. Furthermore, this speculation does not take into account possible cooperative effects of rigor cross-bridge binding affecting neighboring Tn, which could potentially slow the rate of Ca2+ dissociation from additional Tn complexes in the non-overlap region. The existence and cause of this cooperative effect is controversial. Reported cooperative effects of cross-bridge binding on skeletal Tn have ranged from non-existent to only a few cross-bridges influencing the entire thin filament (Fuchs, 1985; Guth and Potter, 1987; Gordon et al., 2000).
Since the myofibrils significantly shortened in the presence of Ca2+ and ATP ± strong cross-bridge inhibitors, we were not able to delineate the effect of cross-bridge binding on the rate of Ca2+ dissociation from the regulatory domain of TnC. In the future it may be possible to accomplish these experiments with ATPγS or by minimally cross-linking the myofibrils (Glyn and Sleep, 1985; Kraft et al., 1999). Stretching the myofibrils beyond overlap ± myosin subfragment 1 may also be an approach to test the effect of cross-bride binding on Tn Ca2+ dissociation kinetics. Alternatively, it might be possible to simultaneously measure contraction/relaxation and TnC fluorescence changes from myofibrils mounted in force measuring devices, where sarcomere length can be better maintained (Tesi et al., 2002; Poggesi et al., 2005).
Although different buffer conditions were utilized for the fiber, titration, and stopped-flow studies, we do not believe this to have compromised the qualitative interpretation of our data. Consistent with our findings, Johnson et al., 1997 and references within) demonstrated that the rate of relaxation from intact frog skeletal muscle fibers (∼27/s) was nearly identical to that of the rate of Ca2+ dissociation from a fluorescently labeled skeletal TnIAANS complex (labeled on TnI) in solution at 20°C. This paper also demonstrated that as the rate of the Ca2+ transient decay was increased (with only marginal decreases in peak amplitude), the relaxation kinetics plateaued at a rate (∼9/s) again nearly identical to that of the Ca2+ dissociation rate from the N-terminal regulatory domain of TnIAANS at 10°C. Mathematical modeling of Ca2+ spark dynamics, also measured in frog skeletal muscle at 18°C, suggested that Ca2+ dissociates at ∼13/s from the N-terminal regulatory domain of TnC (Baylor et al., 2002). Furthermore, we have shown that relaxation of rabbit psoas fibers was slowed approximately twofold at 15°C by reconstitution of the fibers with a TnC mutant that had a quantitatively slowed rate of Ca2+ dissociation from the N-terminal regulatory domain of the TnC–TnI complex (Luo et al., 2002; Davis et al., 2004). All of these studies demonstrate that the rate of Ca2+ dissociation from the N-terminal regulatory domain of TnC in muscle is similar to that measured in the myofibrils. Thus, the rate of Ca2+ dissociation from TnC may influence the kinetics of skeletal muscle relaxation.
There are a diverse group of skeletal myopathies that typically manifest as muscle weakness (Ochala, 2008). Mutations in several thin filament proteins including, actin, tropomyosin, TnI, and TnT have been linked to these skeletal muscle diseases. Many of the protein modifications alter the Ca2+ sensitivity of force development (Michele and Metzger, 2000; Robinson et al., 2007). Although not known, these modifications may alter the rate of Ca2+ dissociation from TnC. This effect may be more significant for slow muscle where force development is more dependent on motor-unit recruitment and much less so on increasing its low frequency of motor-unit firing (Fruend, 1983; Navarrete and Vrbová, 1983). Thus, if the rate of Ca2+ dissociation is increased in these slow fibers, the twitch may relax too quickly, leading to a decrease in force production at any given frequency of muscle stimulation. In fact, a skeletal myopathy associated tropomyosin mutation (M9R) expressed in cardiac myocytes, accelerated relaxation (Michele et al., 2002). These findings are similar to the effects of other thin filament, cardiac disease related mutations that increase or decrease the rate of cardiac muscle relaxation (Du et al., 2007, 2008). Thus, the rate of Ca2+ dissociation from TnC may have profound effects on both skeletal and cardiac muscle physiology and pathophysiology.
Conflict of Interest Statement
The authors declare that the research was conducted in the absence of any commercial or financial relationships that could be construed as a potential conflict of interest.
Acknowledgments
We thank Dr. Lawrence Smillie for the generous gifts of the chicken fast-twitch skeletal muscle TnC plasmid and TnI protein and Zhenyun Yang for help in preparing the myofibril samples. This research was funded, in part, by NIH grant HL091986 to Dr. Jonathan P. Davis, NIH grant HL087462 to Dr. Svetlana B. Tikunova, NIH grant TL1RR025753 from the National Center For Research Resources to Sean C. Little, and by separate awards from the American Heart Association, to Dr. Jonathan P. Davis, Catalina Alionte, and Darl R. Swartz.
References
Baylor, S. M., Hollingworth, S., and Chandler, W. K. (2002). Comparison of simulated and measured calcium sparks in intact skeletal muscle fibers of the frog. J. Gen. Physiol. 120, 349–368.
Cantino, M. E., Allen, T. S., and Gordon, A. M. (1993). Subsarcomeric distribution of calcium in demembranated fibers of rabbit psoas muscle. Biophys. J. 64, 211–222.
Cantino, M. E., Eichen, J. G., and Daniels, S. B. (1998). Distributions of calcium in A and I bands of skinned vertebrate muscle fibers stretched to beyond filament overlap. Biophys. J. 75, 948–956.
Davis, J. P., Rall, J. A., Alionte, C., and Tikunova, S. B. (2004). Mutations of hydrophobic residues in the N-terminal domain of troponin C affect calcium binding and exchange with the troponin C-troponin I96-148 complex and muscle force production. J. Biol. Chem. 279, 17348–17360.
Davis, J. P., Rall, J. A., Reiser, P. J., Smillie, L. B., and Tikunova, S. B. (2002). Engineering competitive magnesium binding into the first EF-hand of skeletal troponin C. J. Biol. Chem. 277, 49716–49726.
Du, C. K., Morimoto, S., Nishi, K., Minakami, R., Ohta, M., Tadano, N., Lu, Q. W., Wang, Y. Y., Zhan, D. Y., Mochizuki, M., Kita, S., Miwa, Y., Takahashi-Yanaga, F., Iwamoto, T., Ohtsuki, I., and Sasaquri, T. (2007). Knock-in mouse model of dilated cardiomyopathy caused by troponin mutation. Circ. Res. 101, 185–194.
Du, J., Liu, J., Feng, H. Z., Hossain, M. M., Gobara, N., Zhang, C., Li, Y., Jean-Charles, P. Y., Jin, J. P., and Huang, X. P. (2008). Impaired relaxation is the main manifestation in transgenic mice expressing a restrictive cardiomyopathy mutation, R193H, in cardiac TnI. Am. J. Physiol. Heart Circ. Physiol. 294, H2604–H2613.
Fowler, V. M. (1996). Regulation of actin filament length in erythrocytes and striated muscle. Curr. Opin. Cell Biol. 8, 86–96.
Fruend, H. J. (1983). Motor unit and muscle activity in voluntary motor control. Physiol. Rev. 63, 387–431.
Fuchs, F. (1985). The binding of calcium to detergent-extracted rabbit psoas muscle fibres during relaxation and force generation. J. Muscle Res. Cell Motil. 6, 477–486.
Glyn, H., and Sleep, J. (1985). Dependence of adenosine triphosphatase activity of rabbit psoas muscle fibres and myofibrils on substrate concentration. J. Physiol. (Lond.) 365, 259–276.
Golosinska, K., Pearlstone, J. R., Borgford, T., Oikawa, K., Kay, C. M., Carpenter, M. R., and Smillie, L. B. (1991). Determination of and corrections to sequences of turkey and chicken troponins-C. Effects of Thr-130 to Ile mutation on Ca2+ affinity. J. Biol. Chem. 266, 15797–15809.
Gomes, A. V., Venkatraman, G., Davis, J. P., Tikunova, S. B., Engel, P., Solaro, R. J., and Potter, J. D. (2004). Cardiac troponin T isoforms affect the Ca(2+) sensitivity of force development in the presence of slow skeletal troponin I: insights into the role of troponin T isoforms in the fetal heart. J. Biol. Chem. 279, 49579–49587.
Gordon, A. M., Homsher, E., and Regnier, M. (2000). Regulation of contraction in striated muscle. Physiol. Rev. 80, 853–924.
Greaser, M. L., Moss, R. L., and Reiser, P. J. (1988). Variations in contractile properties of rabbit single muscle fibres in relation to troponin T isoforms and myosin light chains. J. Physiol. (Lond.) 406, 85–98.
Guth, K., and Potter, J. D. (1987). Effect of rigor and cycling cross-bridges on the structure of troponin C and on the Ca2+ affinity of the Ca2+-specific regulatory sites in skinned rabbit psoas fibers. J. Biol. Chem. 262, 13627–13635.
Houdusse, A., Love, M. L., Dominguez, R., Grabarek, Z., and Cohen, C. (1997). Structures of four Ca2+-bound troponin C at 2.0 A resolution: further insights into the Ca2+-switch in the calmodulin superfamily. Structure 5, 1695–1711.
Johnson, J. D., Jiang, Y., and Flynn, M. (1997). Modulation of Ca2+ transients and tension by intracellular EGTA in intact frog muscle fibers. Am. J. Physiol. 272, C1437–C1444.
Johnson, J. D., Nakkula, R. J., Vasulka, C., and Smillie, L. B. (1994). Modulation of Ca2+ exchange with the Ca(2+)-specific regulatory sites of troponin C. J. Biol. Chem. 269, 8919–8923.
Johnson, J. D., Robinson, D. E., Robertson, S. P., Schwartz, A., and Potter, J. D. (1981). “Ca2+ exchange with troponin and the regulation of muscle contraction,” in The Regulation of Muscle Contraction Excitation-Contraction Coupling, eds A. D. Grinnell, and M. A. B. Brazier (New York, NY: Academic Press), 241–259.
Kraft, T., Xu, S., Brenner, B., and Yu, L. C. (1999). The effect of thin filament activation on the attachment of weak binding cross-bridges: a two-dimensional x-ray diffraction study on single muscle fibers. Biophys. J. 76, 1494–1513.
Kreutziger, K. L., Piroddi, N., Scellini, B., Tesi, C., Poggesi, C., and Regnier, M. (2008). Thin filament Ca2+ binding properties and regulatory unit interactions alter kinetics of tension development and relaxation in rabbit skeletal muscle. J. Physiol. (Lond.) 586, 3683–3700.
Lee, R. S., Tikunova, S. B., Kline, K. P., Zot, H. G., Hasbun, J. E., Minh, N. V., Swartz, D. R., Rall, J. A., and Davis, J. P. (2010). Effect of Ca2+ binding properties on the rate of skeletal muscle force redevelopment. Am. J. Physiol. Cell Physiol. 299, C1091–C1099.
Li, M. X., Chandra, M., Pearlstone, J. R., Racher, K. I., Trigo-Gonzalez, G., Borgford, T., Kay, C. M., and Smillie, L. B. (1994). Properties of isolated recombinant N and C domains of chicken troponin C. Biochemistry 33, 917–925.
Li, M. X., Gagne, S. M., Tsuda, S., Kay, C. M., Smillie, L. B., and Sykes, B. D. (1995). Calcium binding to the regulatory N-domain of skeletal muscle troponin C occurs in a stepwise manner. Biochemistry 34, 8330–8340.
Luo, Y., Davis, J. P., Smillie, L. B., and Rall, J. A. (2002). Determinants of relaxation rate in rabbit skinned skeletal muscle fibres. J. Physiol. (Lond.) 545, 887–901.
Martyn, D. A., Freitag, C. J., Chase, P. B., and Gordon, A. M. (1999). Ca2+ and cross-bridge-induced changes in troponin C in skinned skeletal muscle fibers: effects of force inhibition. Biophys. J. 76, 1480–1493.
Metzger, J. M., Greaser, M. L., and Moss, R. L. (1989). Variations in cross-bridge attachment rate and tension with phosphorylation of myosin in mammalian skinned skeletal muscle fibers. Implications for twitch potentiation in intact muscle. J. Gen. Physiol. 93, 855–883.
Michele, D. E., Coutu, P., and Metzger, J. M. (2002). Divergent abnormal muscle relaxation by hypertrophic cardiomyopathy and nemaline myopathy mutant tropomyosins. Physiol. Genomics 9, 103–111.
Michele, D. E., and Metzger, J. M. (2000). Physiological consequences of tropomyosin mutations associated with cardiac and skeletal myopathies. J. Mol. Med. 78, 543–553.
Morano, I., and Ruegg, J. C. (1991). What does TnCDANZ fluorescence reveal about the thin filament state? Pflugers Arch. 418, 333–337.
Morimoto, K., and Harrington, W. F. (1973). Isolation and composition of thick filaments from rabbit skeletal muscle. J. Mol. Biol. 77, 165–175.
Navarrete, R., and Vrbová, G. (1983). Changes of activity patterns in slow and fast muscles during postnatal development. Dev. Brain Res. 8, 11–19.
Norman, C., Rall, J. A., Tikunova, S. B., and Davis, J. P. (2007). Modulation of the rate of cardiac muscle contraction by troponin C constructs with various calcium binding affinities. Am. J. Physiol. Heart Circ. Physiol. 293, H2580–H2587.
Ochala, J. (2008). Thin filament proteins mutations associated with skeletal myopathies: defective regulation of muscle contraction. J. Mol. Med. 86, 1197–1204.
Oliveira, D. C., and Reinach, F. C. (2003). The calcium-induced switch in the troponin complex probed by fluorescent mutants of troponin I. Eur. J. Biochem. 270, 2937–2944.
Pearlstone, J. R., Chandra, M., Sorenson, M. M., and Smillie, L. B. (2000). Biological function and site II Ca2+-induced opening of the regulatory domain of skeletal troponin C are impaired by invariant site I or II Glu mutations. J. Biol. Chem. 275, 35106–35115.
Poggesi, C., Tesi, C., and Stehle, R. (2005). Sarcomeric determinants of striated muscle relaxation kinetics. Pflugers Arch. 449, 505–517.
Ramos, C. H. (1999). Mapping subdomains in the C-terminal region of troponin I involved in its binding to troponin C and to thin filament. J. Biol. Chem. 274, 18189–18195.
Robertson, S. P., and Potter, J. D. (1984). The regulation of free calcium ion concentration by metal chelators. Meth. Pharmacol. 5, 63–75.
Robinson, P., Lipscomb, S., Preston, L. C., Altin, E., Watkins, H., Ashley, C. C., and Redwood, C. S. (2007). Mutations in fast skeletal troponin I, troponin T, and beta-tropomyosin that cause distal arthrogryposis all increase contractile function. FASEB J. 21, 896–905.
Rosenfeld, S. S., and Taylor, E. W. (1985). Kinetic studies of calcium binding to regulatory complexes from skeletal muscle. J. Biol. Chem. 260, 252–261.
Satyshur, K. A., Pyzalska, D., Greaser, M., Rao, S. T., and Sundaralingam, M. (1994). Structure of chicken skeletal muscle troponin C at 1.78 A resolution. Acta Crystallogr. D Biol. Crystallogr. 50, 40–49.
Sayle, R. A., and Milner-White, E. J. (1995). RasMol: biomolecular graphics for all. Trends Biochem. Sci. 20, 374–376.
Shaw, M. A., Ostap, E. M., and Goldman, Y. E. (2003). Mechanism of inhibition of skeletal muscle actomyosin by N-benzyl-p-toluenesulfonamide. Biochemistry 42, 6128–6135.
Shitaka, Y., Kimura, C., Iio, T., and Miki, M. (2004). Kinetics of the structural transition of muscle thin filaments observed by fluorescence resonance energy transfer. Biochemistry 43, 10739–10747.
Shitaka, Y., Kimura, C., and Miki, M. (2005). The rates of switching movement of troponin T between three states of skeletal muscle thin filaments determined by fluorescence resonance energy transfer. J. Biol. Chem. 280, 2613–2619.
Stephenson, D. G., and Williams, D. A. (1981). Calcium-activated force responses in fast- and slow-twitch skinned muscle fibers of the rat at different temperatures. J. Physiol. (Lond.) 317, 281–302.
Swartz, D. R., Moss, R. L., and Greaser, M. L. (1997). Characteristics of troponin C binding to the myofibrillar thin filament: extraction of troponin C is not random along the length of the thin filament. Biophys. J. 73, 293–305.
Tesi, C., Piroddi, N., Colomo, F., and Poggesi, C. (2002). Relaxation kinetics following sudden Ca(2+) reduction in single myofibrils from skeletal muscle. Biophys. J. 83, 2142–2151.
Tikunova, S. B., Rall, J. A., and Davis, J. P. (2002). Effect of hydrophobic residue substitutions with glutamine on Ca(2+) binding and exchange with the N-domain of troponin C. Biochemistry 41, 6697–6705.
Valencia, F. F., Paulucci, A. A., Quaggio, R. B., Da Silva, A. C., Farah, C. S., and Reinach, F. C. (2003). Parallel measurement of Ca2+ binding and fluorescence emission upon Ca2+ titration of recombinant skeletal muscle troponin C. Measurement of sequential calcium binding to the regulatory sites. J. Biol. Chem. 278, 11007–11014.
Vinogradova, M. V., Stone, D. B., Malanina, G. G., Karatzaferi, C., Cooke, R., Mendelson, R. A., and Fletterick, R. J. (2005). Ca2+-regulated structural changes in troponin. Proc. Natl. Acad. Sci. U.S.A. 102, 5038–5043.
Wahr, P. A., Johnson, J. D., and Rall, J. A. (1998). Determinants of relaxation rate in skinned frog skeletal muscle fibers. Am. J. Physiol. 274, C1608–C1615.
Keywords: troponin, calcium, myofibril, skeletal, muscle, relaxation, dissociation
Citation: Little SC, Tikunova SB, Norman C, Swartz DR and Davis JP (2011) Measurement of calcium dissociation rates from troponin C in rigor skeletal myofibrils. Front. Physio. 2:70. doi: 10.3389/fphys.2011.00070
Received: 31 August 2011; Accepted: 19 September 2011;
Published online: 11 October 2011.
Edited by:
Julio L. Vergara, University of California Los Angeles, USAReviewed by:
Julien Ochala, Uppsala University, SwedenD. George Stephenson, La Trobe University, Australia
Copyright: © 2011 Little, Tikunova, Norman, Swartz and Davis. This is an open-access article subject to a non-exclusive license between the authors and Frontiers Media SA, which permits use, distribution and reproduction in other forums, provided the original authors and source are credited and other Frontiers conditions are complied with.
*Correspondence: Jonathan P. Davis, Department of Physiology and Cell Biology, The Ohio State University, 209 Hamilton Hall, 1645 Neil Avenue, Columbus, OH 43210, USA. e-mail:ZGF2aXMuODEyQG9zdS5lZHU=