- National Heart & Lung Institute, Imperial College London, London, UK
Contraction in the mammalian heart is controlled by the intracellular Ca2+ concentration as it is in all striated muscle, but the heart has an additional signaling system that comes into play to increase heart rate and cardiac output during exercise or stress. β-adrenergic stimulation of heart muscle cells leads to release of cyclic-AMP and the activation of protein kinase A which phosphorylates key proteins in the sarcolemma, sarcoplasmic reticulum and contractile apparatus. Troponin I (TnI) and Myosin Binding Protein C (MyBP-C) are the prime targets in the myofilaments. TnI phosphorylation lowers myofibrillar Ca2+-sensitivity and increases the speed of Ca2+-dissociation and relaxation (lusitropic effect). Recent studies have shown that this relationship between Ca2+-sensitivity and TnI phosphorylation may be unstable. In familial cardiomyopathies, both dilated and hypertrophic (DCM and HCM), a mutation in one of the proteins of the thin filament often results in the loss of the relationship (uncoupling) and blunting of the lusitropic response. For familial dilated cardiomyopathy in thin filament proteins it has been proposed that this uncoupling is causative of the phenotype. Uncoupling has also been found in human heart tissue from patients with hypertrophic obstructive cardiomyopathy as a secondary effect. Recently, it has been found that Ca2+-sensitizing drugs can promote uncoupling, whilst one Ca2+-desensitizing drug Epigallocatechin 3-Gallate (EGCG) can reverse uncoupling. We will discuss recent findings about the role of uncoupling in the development of cardiomyopathies and the molecular mechanism of the process.
Introduction
The heart has a unique system for rapidly and precisely adjusting cardiac output to meet the demands put upon it. The rhythmic contraction and relaxation of heart muscle is due to the rise and fall of sarcoplasmic calcium ion (Ca2+) concentration under neural control. Contraction is initiated by Ca2+ release from the sarcoplasmic reticulum via the Ryanodine receptor and is terminated by Ca2+-uptake by the ATP-powered sarcoplasmic Ca2+ pump (SERCA). Ca2+ binds to troponin C (TnC), the Ca2+ receptor of the contractile apparatus to switch on contractile interactions between actin and the myosin motor protein in the thick filaments (Gordon et al., 2000; Macleod et al., 2010).
Independently of this Ca2+-switch, the speed and force of contraction is modulated in a graded way by changing the inotropic state of muscle. The inotropic state is largely controlled by the sympathetic system that releases β-adrenergic agonists at nerve endings and into the circulation from the adrenal glands. These bind to and activate β1 receptors on the cardiomyocyte surface and initiate a cascade leading to increased intracellular cyclic AMP concentrations which in turn activate the cyclic AMP dependent protein kinase (PKA) (Macleod et al., 2010). PKA phosphorylates several proteins in the sarcolemma, sarcoplasmic reticulum and the contractile apparatus, thus regulating their activity. The combined result of the action of PKA is a co-ordinated increase in cardiac output due to increased heart rate (chronotropy) increased force of contraction (inotropy) and increased rate of relaxation (lusitropy) (Layland et al., 2005).
PKA phosphorylates Myosin Binding Protein-C (MyBP-C) and troponin I (TnI) within the cardiac myofibril. TnI is the inhibitory component of the trimeric troponin molecule that makes up the Ca2+-switch of the contractile apparatus. TnI binds to TnC when Ca2+ is bound to TnC, whilst in the absence of Ca2+, the C-terminus of TnI is released and is able to interact with actin and tropomyosin to inhibit the thin filament's interaction with the motor protein, myosin. Thus, the interactions of TnC with TnI and Ca2+ are crucial for the Ca2+ control of muscle. Early studies showed that β-adrenergic stimulation of contraction was associated with enhanced phosphorylation of TnI (England, 1976; Solaro et al., 1976), that TnI was bis-phosphorylated at serines 22 and 23 in the cardiac-specific N-terminal extension by PKA (Mittmann et al., 1990; Al-Hillawi et al., 1995; Ayaz-Guner et al., 2009) and that the primary effect of phosphorylation of TnI in vitro was reduced Ca2+-sensitivity and faster dissociation of Ca2+ from TnC (Solaro et al., 1976; Robertson et al., 1982; Zhang et al., 1995; Dong et al., 2007). This can cause an increase in the rate of relaxation (lusitropic response) which is essential when heart rate is increased (Kentish et al., 2001; Layland et al., 2005). Transgenic mouse studies have demonstrated the physiological importance of TnI phosphorylation since mice with unphosphorylatable TnI have a blunted response to β-adrenergic stimulation and this leads to an enhanced susceptibility to the development of heart failure under stress (Fentzke et al., 1999; Pi et al., 2002; Yasuda et al., 2007).
Over the last 10 years it has become evident that the modulation of myofilament Ca2+-sensitivity by TnI phosphorylation is quite a labile system and that mutations associated with cardiomyopathies in particular, can lead to disruption of the system. This was first noted with mutations in TnI that caused hypertrophic cardiomyopathy (HCM) (Deng et al., 2001, 2003) but its physiological significance was uncovered by studies on dilated cardiomyopathy (DCM) (Dyer et al., 2007, 2009; Memo et al., 2013). DCM is a major cause of heart failure in humans and a substantial proportion of cases of DCM are inherited. Mutations in the thin filament proteins [actin, tropomyosin, troponin T (TnT), TnI, and TnC] that are associated with familial DCM have been studied particularly closely (reviewed in Chang and Potter, 2005; Morimoto, 2008; Marston, 2011). By studying isolated thin filaments with the quantitative in vitro motility assay (IVMA) it was found that in all of these DCM-causing mutations the myofilament Ca2+-sensitivity is independent of the level of TnI phosphorylation. Therefore, by analogy with the S22/23A transgenic mice, it was proposed that this uncoupling was necessary and sufficient to cause the DCM phenotype (Memo et al., 2013).
In this review we show that “uncoupling” of TnI phosphorylation from changes in Ca2+-sensitivity is a widespread phenomenon with significant implications for the understanding of heart disease and its treatment.
Methodology
Phosphorylation Measurement
As there is a link between troponin (Tn) phosphorylation and Ca2+-sensitivity in cardiac muscle, measurement of troponin I (TnI) phosphorylation levels in situ is very important. Quantitative methods such as Top-down mass spectrometry and phosphate affinity SDS-PAGE has clearly established that serines 22 and 23 are the main amino acids phosphorylated in native heart tissue in rats, mice or humans (Zabrouskov et al., 2008; Ayaz-Guner et al., 2009; Marston and Walker, 2009; Messer et al., 2009; Sancho Solis et al., 2009; Wang et al., 2012) (these are often numbered 23 and 24 according to the coding sequence, however the N terminal methionine is missing in all mature TnI in heart tissue). The first quantitative studies used non-equilibrium pH gradient electrophoresis in 1 or 2D (Ardelt et al., 1998; Kobayashi et al., 2005). Measurement of phosphorylation became much easier with the introduction of specific methods to detect phosphoproteins using the phosphoprotein gel stain, Pro-Q Diamond (Steinberg et al., 2003) or antibodies to phosphorylated Tn (Al-Hillawi et al., 1998; Haworth et al., 2004). This methodology has been widely adopted, but has its limitations, since to be quantitative it requires the use of an external standard, which may introduce systematic errors (Figure 1A) (Messer et al., 2007; Zaremba et al., 2007).
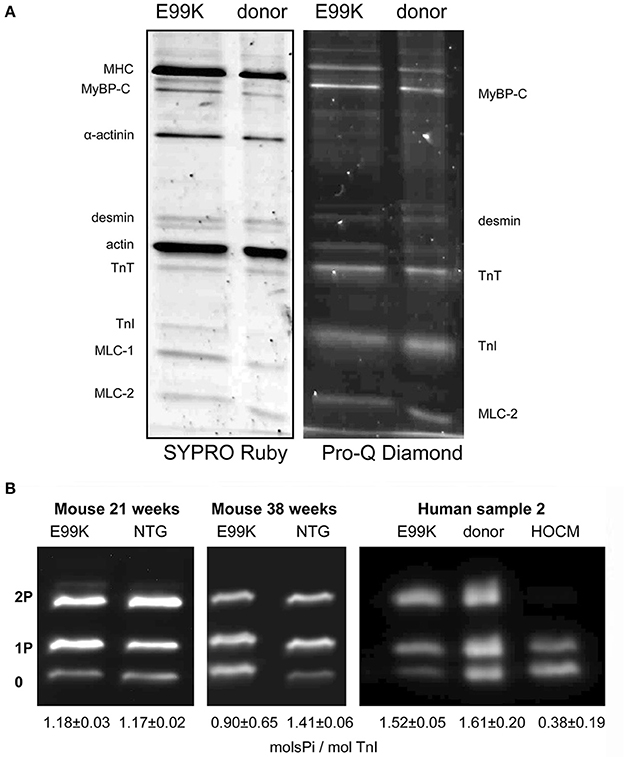
Figure 1. SDS-PAGE analysis of myofibrillar proteins and phosphoproteins. (A) Myofibril fraction of heart muscle separated by SDS-PAGE and stained successively with Pro-Q Diamond phosphoprotein stain and SYPRO Ruby total protein stain. Left, NTG and ACTC E99K mouse heart myofibrils (38 weeks male). Right, donor and ACTC E99K heart sample 2 myofibrils (Song et al., 2011). The TnI band is strongly stained together with MyBP-C, TnT and MLC-2. (B) Phosphate affinity SDS-PAGE analysis of TnI phospho-species. Left, comparison of TnI phosphorylation in 21-week-old female ACTC E99K and NTG mouse myofibrils. Right, comparison of TnI phosphorylation in myofibrils from ACTC E99K sample 2, donor heart and a typical interventricular septum sample from a patient with hypertrophic obstructive cardiomyopathy (HOCM) (Song et al., 2011). All samples show a high level of phosphorylation, except for the failing heart sample.
Thus, to overcome this, we developed the use of phosphate affinity SDS-PAGE which was first developed by the Kinoshita group (Kinoshita et al., 2006). Phos-Tags are Mn2+-dependent specific chelators of phosphoproteins, when added to standard SDS-PAGE, phosphoproteins are retarded in proportion to the number of phosphates per mole of protein (Messer et al., 2009). Thus, unphosphorylated, monophosphorylated and bisphosphorylated species of phosphoproteins can be separated (Figure 1B). We have used Phos-Tags in conjunction with a specific cardiac TnI antibody to accurately measure phosphorylation levels in myofibrillar extracts from human heart tissue. The advantage of phosphate affinity SDS-PAGE is that it permits rapid identification and direct quantification of the mono and bis-phosphorylated TnI.
All the methods for measuring Ser22/23 phosphorylation in intact tissue give similar results: flash-frozen mouse or rat heart yield a phosphorylation level of 1–1.5 mol Pi/ mol TnI with up to 40% of TnI being the bis-phosphorylated species, whilst human donor heart samples have 1.5–2 mols Pi/mol TnI with up to 60% of bis-phosphorylated species. These types of samples have been widely used in the study of the role of TnI phosphorylation in modulating muscle regulation, however there is still controversy as to whether these samples are actually representative of the “normal” heart (Jweied et al., 2007; Marston and Detombe, 2008).
In contrast, pathological samples from hearts transplanted for idiopathic dilated cardiomyopathy or septal myectomies from patients with hypertrophic obstructive cardiomyopathy (HOCM) generally have a low level of phosphorylation (0.1–0.4 mols Pi/mol TnI) (Van Der Velden et al., 2003; Messer et al., 2007, 2009; Zaremba et al., 2007; Ayaz-Guner et al., 2009; Hamdani et al., 2009; Jacques et al., 2009; Bayliss et al., 2012b).
Manipulation of TnI Phosphorylation Levels
To investigate the relationship between TnI phosphorylation and myofilament Ca2+-sensitivity, the Ca2+-sensitivity needs to be compared with phosphorylated and unphosphorylated Tn, thus the phosphorylation levels need to be manipulated. Initial in vitro work used Tn reconstituted from recombinant subunits expressed in E.coli; TnI could then be readily phosphorylated with PKA catalytic subunit. For transgenic mouse studies, unphosphorylatable TnI could be overexpressed (either slow skeletal TnI in place of cardiac or mutant TnI with Ser 22/23 mutated to alanine Fentzke et al., 1999; Pi et al., 2002; Yasuda et al., 2007). Phosphorylated TnI could be simulated with Ser 22/23 mutated to aspartic acid (Dohet et al., 1995; Mamidi et al., 2012). The first studies of native human heart Tn in IVMA or skinned myocyte contractility compared donor and failing human heart muscle samples, since they had high and low levels of phosphorylation respectively (Van Der Velden et al., 2003; Messer et al., 2007). However, it was not certain whether differences in Ca2+-sensitivity observed (Figure 2A) were due to the different phosphorylation levels or other disease-related factors.
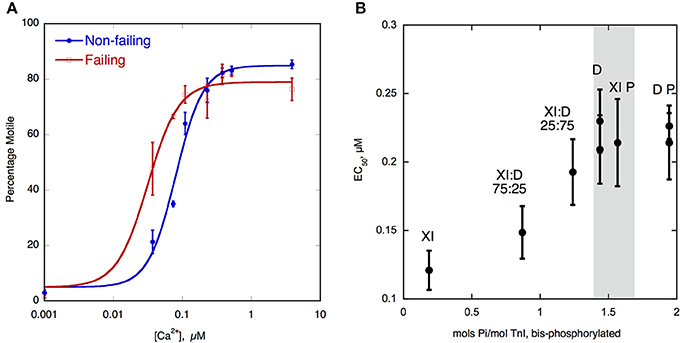
Figure 2. (A) Ca2+-regulation of thin filament motility by non-failing (donor) and failing heart troponin. Thin filament motility was measured by motility assay over a range of [Ca2+] in paired cells. The percentage of filaments motile is plotted as a function of [Ca2+] for a representative experiment. In blue lines and points, non-failing thin filaments, red lines and open points, failing thin filaments. TnI phosphorylation levels are shown in Figure 1B. The points ± s.e.m. are the mean of four determinations of percentage motile measured in one motility cell. The curves are fits of the data to the Hill equation (Messer et al., 2014). (B) Relationship between EC50 for Ca2+-activation of thin filaments and TnI bisphosphorylation. EC50 for thin filaments containing human heart Tn was plotted against the level of TnI bis-phosphorylation. EC50 was measured by IVMA and bis-phosphorylation of TnI (approximates to phosphorylation at Ser22 and 23) was measured by phosphate affinity SDS-PAGE. D, donor heart Tn, XI donor Tn with TnI exchanged, XI:D mixed donor and cTnI-exchanged donor, XI P PKA-treated cTnI-exchanged donor, D P PKA treated donor heart Tn. The gray band corresponds to the phosphorylation level range of human donor and wild-type mouse Tn, see Figure 1B (Memo et al., 2013).
Ideally, one should be able to study the same sample at different phosphorylation levels. This can be done by dephosphorylation or phosphorylation. The phosphorylation level of Tn isolated from heart tissue may be reduced by treatment with a phosphatase (shrimp alkaline phosphatase has proved to be the most reliable enzyme) or increased by PKA treatment (Bayliss et al., 2012b). PKA treatment has been used successfully for many years to increase the level of phosphorylation of isolated myocytes or skinned muscle strips (Hamdani et al., 2009; Kooij et al., 2010) but dephosphorylation is not usually successful, either there is inadequate reduction in phosphorylation level or the enzyme preparations cause degradation of the muscle. To dephosphorylate heart muscle in laboratory animals a different method may be used. For instance, mice can be treated with Propranolol (8 mg/kg) to block β1-adrenoreceptors and deactivate PKA to reduce phosphorylation levels of PKA substrates including Tn and MyBP-C (Bailin, 1979; Wang et al., 2011; Vikhorev et al., 2013).
Normal Relationship between TnI Phosphorylation and Ca2+-regulation
It was established, soon after the discovery of troponin I (TnI) phosphorylation, that phosphorylation of troponin (Tn) modulates Ca2+-regulation by Tn by reducing the Ca2+-sensitivity and increasing the force or crossbridge turnover rate at maximally activating Ca2+ concentrations (Ray and England, 1976; Bailin, 1979; Mope et al., 1980). The magnitude of the Ca2+-sensitivity shift has been consistently been measured in the 2–3-fold range. It has been demonstrated that the reduced Ca2+-sensitivity is due to an increase in the rate of Ca2+-dissociation from Tn in the thin filaments (Robertson et al., 1982; Zhang et al., 1995; Dong et al., 2007), thereby providing a mechanism for the lusitropic (faster relaxation) response to β-adrenergic stimulation. Two recent studies have directly shown the relationship between phosphorylation and Ca2+-sensitivity. A study by Messer et al. used the in vitro motility assay (IVMA) with isolated human cardiac Tn in reconstituted thin filaments. By manipulating the level of Tn phosphorylation and then measuring the level using phosphate affinity SDS-PAGE (Phos-Tags) (Messer et al., 2009), a consistent relationship between phosphorylation level and Ca2+-sensitivity was found (Memo et al., 2013) (Figure 2B). A similar study by Kooij et al. measured the force in individual cardiomyocytes and found a similar relationship between TnI phosphorylation at Ser22/23 and Ca2+-sensitivity (Kooij et al., 2010). A reduced level of cTnI phosphorylation has been observed in end-stage failing hearts and this correlates with the increased Ca2+-sensitivity seen when failing hearts were compared to donor hearts (Figure 2A) (Wolff et al., 1995; Bodor et al., 1997; Van Der Velden et al., 2003; Messer et al., 2007).
The Discovery of Uncoupling in Familial Cardiomyopathies
Initial investigations into the functional consequences of cardiomyopathy mutations did not consider the role of TnI phosphorylation, but when this was investigated, uncoupling was immediately apparent. Uncoupling was first reported in a series of studies from Kornelia Jaquet's laboratory. Deng et al. studied the cTnI HCM mutation R145G and compared phosphorylated and unphosphorylated recombinant R145G mutant Tn in reconstituted thin filaments regulating actomyosin ATPase. The authors found that the shift in pCa50 due to bisphosphorylation, observed with wild-type Tn, was not statistically significant (Deng et al., 2001). Later, two other HCM-causing mutations in cTnI, G203S, and K206Q, were also shown to uncouple, although the effect with G203S was only partial (Deng et al., 2003). A study on the cTnI HCM mutation R21C found that the Ca2+-sensitivity decrease due to PKA phosphorylation was smaller when compared to wild-type (Gomes et al., 2005). A similar study on the cTnC HCM mutation L29Q also found that the Ca2+-sensitivity (measured by both actomyosin ATPase activity and IVMA) was not affected by PKA phosphorylation of cTnI (Schmidtmann et al., 2005). In fact, this study was the first to suggest that the mutation hindered the transduction of the phosphorylation signal from TnI to TnC. Dong et al. have investigated the effects of cTnI phosphorylation on the kinetics of Ca2+ regulation of Tn both in wild-type and mutant Tn (Dong et al., 2007). The authors not only looked at the HCM-causing L29Q mutation in TnC but also the DCM-causing mutation TnC G159D and found that both mutations inhibited the ability of PKA phosphorylation of cTnI to reduce Ca2+-sensitivity and speed up Ca2+ dissociation (Dong et al., 2008).
The DCM-causing TnC G159D mutation is one of the best characterized clinically (Mogensen et al., 2004; Kaski et al., 2007) and the uncoupling phenomenon was also investigated in two further studies. Biesiadecki et al. reported that the cTnC G159D mutation, exchanged into skinned mouse cardiac fibers, had no direct effect on the myofilament response to Ca2+ but it blunted the phosphorylation-dependent change in Ca2+ sensitive tension development without altering cross-bridge cycling rate (Biesiadecki et al., 2007). Dyer et al., investigated Tn containing the TnC G159D mutation in mutant Tn isolated from an explanted heart muscle sample in comparison with donor heart Tn using IVMA and similarly found that, unlike donor heart, Ca2+-sensitivity and maximum sliding speed of thin filaments containing G159D Tn were not sensitive to changes in TnI phosphorylation levels (Dyer et al., 2007, 2009).
These seminal studies on uncoupling investigated mutations in the regions of TnI and TnC that could be directly involved in the phosphorylation-dependent interaction that modulates Ca2+-sensitivity. However, subsequent studies showed that mutations in any protein of the thin filament can induce uncoupling, including actin (ACTC E361G and E99K mutations) tropomyosin (E40K, E54K, and D230N mutations) and troponin T (TnT) (4 mutations recorded to date) in addition to 5 mutations in cTnC and 5 mutations in TnI. The currently known mutations causing uncoupling are summarized in Table 1. Thus, uncoupling may be induced by indirect allosteric effects of mutations anywhere within the thin filament and uncoupling seems to be correlated with mutations identified as causing cardiomyopathies.
Uncoupling as a Primary Cause of Familial Dilated Cardiomyopathy
The recent study by Memo et al. (2013) investigated the uncoupling phenomenon in thin filaments containing a wide range of mutations associated with familial DCM, using the IVMA to measure myofilament Ca2+-sensitivity. It was found that when TnI was fully phosphorylated, the mutations had different effects on Ca2+-sensitivity of thin filaments compared to non-failing; some increased Ca2+-sensitivity (cTnT R141W and ΔK210, cTnI3 K36Q and α-Tropomyosin E40K), some decreased it (α-Tropomyosin D230N, cTnC G159D) whereas for α-actin E361G and α-Tropomyosin E54K there was no change in Ca2+-sensitivity. This confirmed that the simple hypothesis that Ca2+-sensitivity is always reduced by DCM-causing mutations, that we and others had proposed, is no longer tenable (Chang and Potter, 2005; Mirza et al., 2005; Morimoto, 2008). In contrast, when the Ca2+-sensitivity of thin filaments containing phosphorylated and unphosphorylated TnI were compared, it was found that incorporation of any of these mutations caused uncoupling (Memo et al., 2013) (Figure 3).
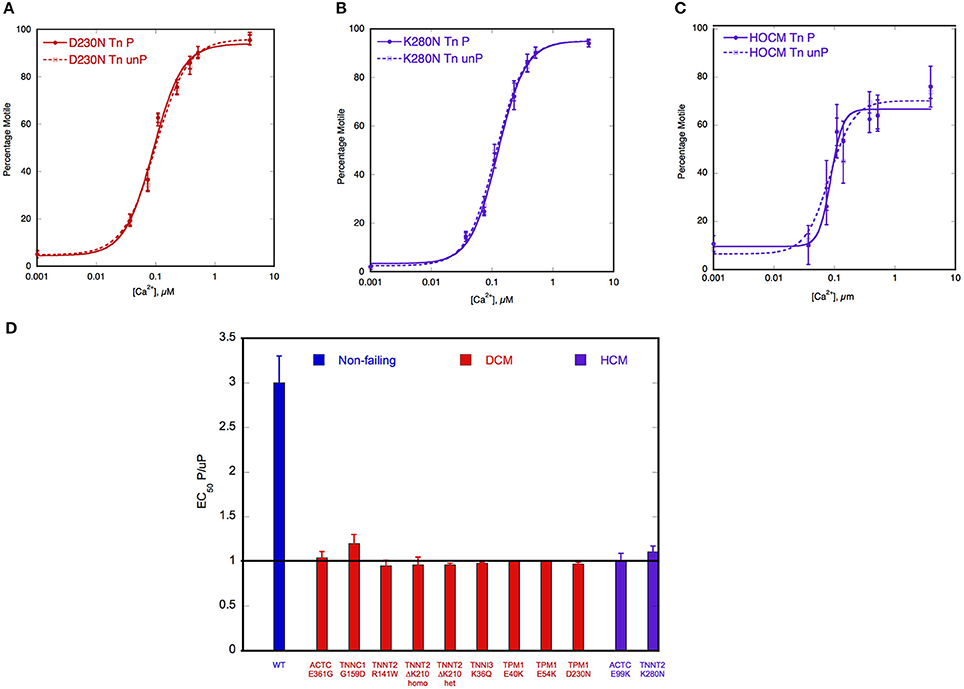
Figure 3. Demonstration of uncoupling due to DCM and HCM mutations and secondary to HOCM. Thin filament motility was measured by motility assay over a range of [Ca2+] in paired cells. The percentage of filaments motile is plotted as a function of [Ca2+] for a representative experiment. Solid lines and points, phosphorylated thin filaments, dotted line and open points, unphosphorylated thin filaments (obtained by phosphatase treatment). The points ± s.e.m. are the mean of four determinations of percentage motile measured in one motility cell. The curves are fits of the data to the Hill equation. (A) Natively phosphorylated and unphosphorylated human donor heart Tn with DCM related α- tropomyosin D230N (100%) and rabbit skeletal muscle α-actin (Memo et al., 2013). (B) Natively phosphorylated and unphosphorylated human donor heart Tn with exchanged recombinant HCM related TnT K280N, human heart tropomyosin and rabbit skeletal muscle α-actin (Messer et al., 2014). (C) Thin filaments containing Tn from a HOCM heart (0.1 mol Pi/mol TnI) or protein kinase A-treated HOCM heart Tn (1.6 mol Pi/mol TnI) (Bayliss et al., 2012b). (D) EC50 of phosphorylated Tn relative to EC50 of unphosphorylated Tn is plotted for wild-type thin filaments and thin filaments containing DCM and HCM-causing mutations. Error bars show s.e.m. of up to 7 replicate comparative measurements (Memo et al., 2013; Messer et al., 2014).
Another study found uncoupling in rare TnC variants identified in DCM: cTnC Y5H, M103I, and I148V either decreased or abolished the effects of PKA phosphorylation on Ca2+-sensitivity (Pinto et al., 2011). Since all the known DCM-causing mutations in thin filament proteins have now been shown to cause uncoupling, whilst having a very variable effect on absolute Ca2+-sensitivity and no DCM mutation has been demonstrated to have normal coupling, there is a strong case for uncoupling to be causative of DCM due to mutations of thin filament components. Thus, a blunting of the heart's response to β-adrenergic stimulation seems to be necessary and sufficient to generate the DCM phenotype. Mechanisms and physiological consequences of uncoupling are discussed in detail later in this review.
Uncoupling is a Common Feature of Cardiomyopathies
Uncoupling is widespread, not only is it observed with mutations that cause familial DCM, it is also observed with mutations that cause hypertrophic cardiomyopathy (HCM). The HCM phenotype has been generally thought to be due to mutations increasing myofilament Ca2+-sensitivity, but it is possible that uncoupling is also characteristic of HCM (Marston, 2011). In addition to the early reports of HCM-causing TnI mutations, described above, uncoupling has also been demonstrated in cTnT R92W (Guinto et al., 2009), cTnT K280N (Messer et al., 2012), α-Tropomyosin E180G (Alves et al., 2014), cardiac actin E99K (Song et al., 2011) and cTnI R21C (Wang et al., 2011) (see Table 1). The situation is less clear-cut in studies using human heart samples; Sequeira et al. (2013) reported that some HCM mutations were uncoupled, but others showed a partial decrease in Ca2+-sensitivity when phosphorylated indicating that uncoupling is not necessarily an all-or-nothing effect in human heart but may be graded. Relevant to these observations is the report that in human heart samples the effect of phosphorylation on EC50 was dependent upon background phosphorylation levels of other myofilament proteins (Kooij et al., 2010).
Uncoupling has been demonstrated to occur as a secondary effect unrelated to any mutation. In the obstructive variant of HCM (HOCM) the hypertrophied interventricular septum causes LVOTO (left ventricular outflow tract obstruction) and pressure overload. Several abnormalities in the contractile proteins in septal tissue from HOCM patients have been observed including; low phosphorylation levels of TnI and MyBP-C (Messer et al., 2009; Copeland et al., 2010b), differences in actin isoform expression (Copeland et al., 2010a) and loss of function in myosin (Jacques et al., 2008). Most of these abnormalities are shared with end-stage failing heart, but the Ca2+-sensitivity of Tn from HOCM samples, studied by IVMA, was not as expected from its low TnI phosphorylation level and this was found to be due to uncoupling of the relationship between Ca2+-sensitivity and TnI phosphorylation (Gallon et al., 2007; Jacques et al., 2008; Bayliss et al., 2012b). Uncoupling was demonstrated directly by comparing HOCM Tn with PKA-treated HOCM Tn to bring phosphorylation up to the same level as donor heart Tn. There was no change in Ca2+-sensitivity (Figures 3C,D). This uncoupling was independent of the mutation causing HCM and was even observed when no mutation was identified. Exchange experiments were carried out to identify which component of the Tn complex was responsible for the uncoupling and the abnormality was shown to be in TnT, although no covalent modifications were found (Bayliss et al., 2012b). This uncoupling in HOCM may be related to the severe pressure overload that patients having myectomy operations exhibit and therefore it is possible that the uncoupling is caused by the pressure overload itself. It would, for instance, be interesting to look at aortic stenosis samples where the patients have pressure overload but not HCM (Marston et al., 2012).
The occurrence of uncoupling in other types of cardiomyopathy has not been tested; it is clear that in most cases of idiopathic DCM, Ca2+-sensitivity is fully coupled to the level of TnI phosphorylation (Messer et al., 2007). On the other hand, it is possible that mutations in sarcomeric proteins that are not part of the contractile apparatus, such as titin or Z-line proteins, also undergo uncoupling, since these can show a blunted response to β-adrenergic stimulation in vitro that is characteristic of uncoupling. Recent studies have shown a blunted β-adrenergic response in MLP W4R and TCAP KO mice (Knoell et al., 2010, 2011) and uncoupling could be inferred from experiments on a mouse model with a titin mutation (Gramlich et al., 2009).
Uncoupling can be Induced by Small Molecules and Phosphorylation
The key to the modulation of Ca2+-sensitivity by cTnI phosphorylation is the interaction of the N-terminal peptide 1–29 of cTnI with TnC, therefore it may be possible to induce uncoupling with small molecules that bind to TnC and change the Ca2+-sensitivity (Ca2+ sensitizers or desensitizers). Of particular interest are the Ca2+-sensitizing drugs EMD57033 and Bepridil (Li et al., 2008). When tested in the IVMA, with thin filaments containing native human Tn, both these drugs increased Ca2+-sensitivity by 2–3-fold and at the same time uncoupled Ca2+-sensitivity from TnI phosphorylation (see Figure 4A). The effect of these drugs is therefore quite analogous to the effect of many HCM-causing mutations (Table 1, Figures 3, 4).
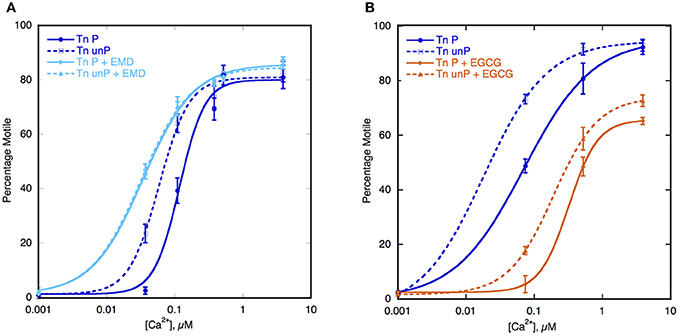
Figure 4. Effect of Ca2+-sensitizers and desensitizers on coupling in thin filaments. Thin filament motility was measured by motility assay over a range of [Ca2+] in paired cells. The percentage of filaments motile is plotted as a function of [Ca2+] for representative experiments. Solid lines and points, phosphorylated thin filaments, dotted line and open points, unphosphorylated thin filaments (obtained by phosphatase treatment). The points ± s.e.m. are the mean of four determinations of percentage motile measured in one motility cell. The curves are fits of the data to the Hill equation. (A) The effect of the Ca2+-sensitizer EMD57033 (EMD). In dark blue, thin filaments in the absence of EMD and in light blue are thin filaments in the presence of 30 μM EMD (Messer et al., 2014). (B) The effect of the Ca2+-desensitizer EGCG. In dark blue, thin filaments in the absence of EGCG and in orange, thin filaments in the presence of 100 μM EGCG (Messer et al., 2014).
In contrast EGCG [(−)-Epigallocatechin 3-Gallate], is a Ca2+-desensitizer that binds at a site formed by the TnI-TnC complex and it has been found to enhance the binding of the N terminal helix1 of TnI to TnC (Robertson et al., 2009; Tadano et al., 2010; Botten et al., 2013). When tested in the IVMA, with thin filaments containing native human Tn, EGCG decreased Ca2+-sensitivity both in wild-type and in DCM-mutant thin filaments and in both phosphorylated and unphosphorylated filaments, thus preserving coupling (Figure 4B). Most strikingly it is also capable of restoring coupling to thin filaments containing mutations that induce uncoupling (Messer et al., 2014).
Another perturbation that can induce uncoupling is phosphorylation of troponin subunits. A study by Nixon et al. found that phosphorylation of cTnI at Ser 150 by AMP-activated protein kinase (AMPK) increased Ca2+-sensitivity of isolated cardiac myofibrils. It also blunted the PKA-dependent calcium desensitization induced by phosphorylation at Ser 22/23 and uncoupled the effects of phosphorylation from β-adrenergic stimulation (Nixon et al., 2012).
Molecular Mechanism of Uncoupling
The phosphorylation dependence of cardiac Tn Ca2+ regulation is due to the interaction of a 30 amino acid N-terminal extension of TnI, containing the PKA-specific phosphorylation sites at Ser 22 and 23, with cTnC (Solaro et al., 2008).
The N-terminal segment of cTnI interacts with the regulatory Ca2+-binding loop in the N-terminal lobe of TnC in the unphosphorylated state. This affects the cTnC interaction with both the regulatory Ca2+ and the TnI switch peptide (144–160) (Li et al., 2008). When TnI is unphosphorylated there is a weak ionic bond between the N terminal and the regulatory Ca2+-binding EF hand of TnC (Howarth et al., 2007). When Ser 22 and 23 are phosphorylated the binding is further weakened (Keane et al., 1990; Ferrieres et al., 2000; Ward et al., 2004a,b; Baryshnikova et al., 2008). Therefore, the unphosphorylated state of TnI is a special state, which is destabilized by phosphorylation, resulting in a lower Ca2+-sensitivity and higher rate of Ca2+ dissociation. Since the initial interaction is quite weak, the loss of the interaction produces only a 2–3-fold change in Ca2+-sensitivity and the rate of Ca2+ dissociation. This appears to be sufficient to generate the lusitropic effect since heart rate also increases by a maximum of 2–3-fold.
We propose that the unphosphorylated state can also be disrupted by mutations or other alterations in any component of the thin filament resulting in the same destabilized state for both phosphorylated and unphosphorylated Tn; in this way uncoupling could be considered as a default state in cardiomyopathies (Liu et al., 2012; Memo et al., 2013).
Recent studies have begun to determine the structure of TnI in complex with TnC in the phosphorylated and unphosphorylated states that forms the basis of the coupling mechanism. X-ray crystallography has defined the core structure of Tn but mobile segments, including the N-terminus of TnI, were not present in the crystal structure (Takeda et al., 2003). NMR studies have defined the structure of the missing peptides based on their binary complexes. A best guess structure of the N-terminal peptide conformation in the phosphorylated and unphosphorylated states was proposed by building these structures onto the Tn core structure (Howarth et al., 2007). Molecular dynamics simulations of the entire Tn molecule have further refined these structures.
The molecular dynamics simulations indicate a possible structure of TnI N-terminus interacting with TnC (Gould et al., 2014) (Figure 5). The most striking feature is that in the presence of Ca2+, the unphosphorylated N terminus of TnI settles in a position looping over the N-terminus of TnC within 50 ns of the start of simulation. The peptide is mostly very mobile and unstructured except for 20RRSS24 that was consistently close to TnC for up to 1 μs of simulation. These four amino acids also exhibited a lower root mean square fluctuation (RMSF) than surrounding residues. When the Ser 22 and 23 were phosphorylated in silico, the two serines become more mobile relative to arginines 20 and 21 suggesting a weakening of their interaction with TnC. In addition, Ca2+ becomes more exposed to solvent and the interaction of the “switch peptide” with TnC is altered. Thus, coupling can be accounted for by the formation of a weak ionic complex between TnC and TnI Ser 22 and 23 that is destabilized by phosphorylation.
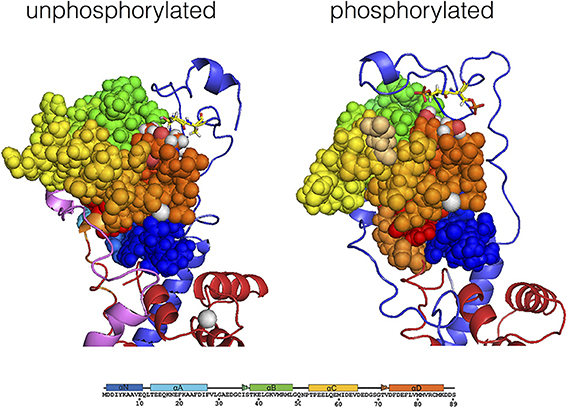
Figure 5. Modulation of the interaction of TnI Ser 22 and 23 with the N terminal lobe of TnC by phosphorylation, determined by molecular dynamics simulations. Average structure after 700 ns simulation for unphosphorylated Tn, left. The Tn was phosphorylated in silico after 550 ns of simulation and the average structure after a further 200 ns is shown, right. The N-terminal lobe of cTnC is shown as spheres with color coding along the peptide chain as indicated by the sequence below; Ser 69 and Thr 71 are colored according to their atoms (oxygen red, hydrogen white). The backbone of cTnI N-terminus is shown in blue; Ser 22 and 23 are shown in yellow in stick representation. Molecular dynamics suggests that with unphosphorylated Tn there is a close interaction of Ser 22 and 23 with cTnC Ser 69 and Thr 71 which is much less prominent when phosphorylated (Gould et al., 2014).
It is interesting to note that when the DCM-causing mutation K36Q in cTnI was introduced in the presence of Ca2+, the simulation showed that Ser 22 and 23 no longer interacted closely with cTnC, in accord with our hypothesis that the Ca2+-cTnC-cTnI N terminus interaction is unique and is destabilized directly by phosphorylation and also allosterically by mutations and other perturbations. Molecular dynamics simulations also show that phosphorylation is associated with long-range conformational changes in Tn and associated proteins that provides a mechanism for mutations in TnT, tropomyosin and actin to induce uncoupling (Manning et al., 2011). It should be noted that this mechanism for uncoupling is the opposite to one proposed by Biesiadecki et al. (2007) where the DCM mutation TnC G159D was proposed to stabilize the interaction of Ser 22 and 23 with cTnC when phosphorylated.
Physiological Relevance of Uncoupling
How is uncoupling of the relationship between TnI phosphorylation and myofilament Ca2+-sensitivity related to the DCM phenotype associated with such mutations? We think it is likely that uncoupling would compromise the heart's response to β1-adrenergic stimulation leading to a reduced cardiac reserve.
The effects of cardiomyopathy-causing mutations on the heart's response to β-adrenergic agonists have not been routinely measured. However, the studies of Song et al. on the ACTC mutations E361G and E99K investigated this question and clearly showed that the response to dobutamine stimulation was blunted (Song et al., 2010, 2011; Marston et al., 2013) (Figure 6). The effect of adrenergic agonists was tested in several other models of HCM and DCM (see Table 2) and they all showed blunting of the response in at least one parameter. It is particularly interesting to note the blunting effect of the muscle LIM protein (MLP) W4R mutation associated with DCM (Knoell et al., 2010), since this protein is a component of the Z-line and is not known to have any function in regulating the contractile apparatus: in this case the putative uncoupling might be a secondary effect similar to that seen in myectomy samples. Nguyen et al. found that young, pre-hypertrophic TNNI3 G203S HCM transgenic mice lacked the normal physiological response to chronic intense swimming exercise, compatible with a blunted response to adrenergic stimulation independent of disease phenotype (Nguyen et al., 2007).
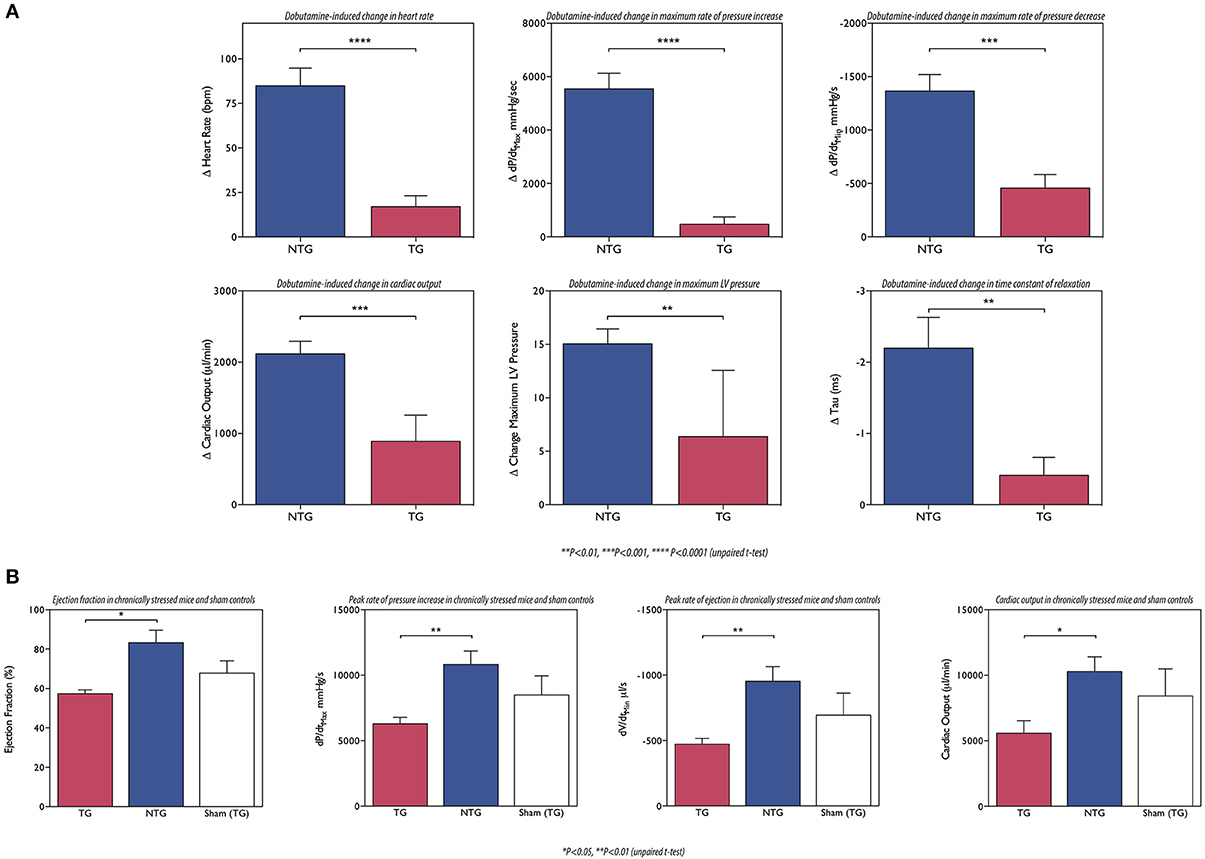
Figure 6. (A) The ACTC E361G mutation blunts the lusitropic, inotropic and chronotropic response to dobutamine in vivo: mice were examined using a pressure volume catheter. The dobutamine-induced acceleration of relaxation (peak rate of relaxation and time constant of relaxation) was significantly lower in ACTC E361G mice indicating a blunted lusitropic response. The inotropic response to dobutamine was also blunted in ACTC E361G mice as indicated by a blunted increase in maximum pressure and the peak rate of pressure increase. Furthermore, dobutamine-induced increase in heart rate (chronotropic effect) was also blunted. Taken together with the attenuated increase in cardiac output these data suggest a significantly diminished cardiac reserve in ACTC E361G mice in vivo (Wilkinson, 2014). (B) The ACTC E361G mutation predisposes TG mice to systolic heart failure under chronic stress: chronic stress was achieved using subcutaneously implanted Alzet osmotic mini pumps to deliver a 4-week infusion of angiotensin II (2 mg/kgBW/day, in saline). Sham controls received mini pumps carrying saline only. At the end of the 4-week infusion period the mice were examined using a pressure volume catheter. The chronic stress treatment evoked symptoms of systolic heart failure in ACTC E361G mice, characterized by decreased ejection fraction, cardiac output and maximum rates of contraction and ejection compared to NTG mice (Wilkinson, 2014).
This loss of cardiac reserve is likely to predispose the heart to failure when under stress. It is notable that most mouse models of DCM-causing mutations show little or no phenotype at rest (ACTC E361G, TNNT2 ΔK210, MYBPC3 knock-in (KI) (see Table 2) and TTN KI Gramlich et al., 2009), especially when heterozygous like the patients with these mutations. This is compatible with the primary defect being in the response to β-adrenergic stimulation that is absent at rest. Several experiments have addressed this question by exposing transgenic mice with HCM or DCM-causing mutations to chronic stress by pressure overload (TAC) or by chronic stimulation with isoprenaline or angiotensin II. In general, they demonstrate that the mutant-containing mice show earlier and more severe symptoms of dilation and heart failure than wild-type. For instance: Wilkinson applied chronic stress by angiotensin II infusion (2 mg/KgBV/da by osmotic minipumps). After 4 weeks ACTC E361G DCM mice had significantly lower dP/dtmax, cardiac output and ejection fraction, compared to NTG (Wilkinson, 2014) (Figure 6). Similarly Gramlich et al. studied a titin mutation that causes DCM (TTN 2-bp insertion mutation (c.43628insAT)) (Gramlich et al., 2009). The authors induced cardiac hypertrophy by a 2-week infusion with angiotensin II. Both wild-type and KI mice developed cardiac hypertrophy after 1 week. After 2 weeks, hypertrophy in wild-type animals was further increased, whereas their heterozygous littermates showed left ventricular dilatation with impaired systolic function and increased myocardial fibrosis.
Whilst it is recognized that the uncoupling phenomenon provides a satisfactory molecular mechanism for thin-filament based mutations that cause DCM, the role of uncoupling in HCM is not as clear. Since Ca2+-sensitivity has been observed to be increased 2–3-fold in virtually every HCM mutation investigated (Marston, 2011), it is likely that this is the primary trigger for the HCM phenotype and that it dominates over the uncoupling phenomenon. It is possible that increased Ca2+-sensitivity and uncoupling are linked properties of thin filaments since the Ca2+-sensitizers EMD57033 and bepridil are also uncouplers and the coupling constant is generally greatest when Ca2+-sensitivity is lowest. It will be very interesting to investigate whether any HCM mutations (or Ca2+-sensitizers) can be found that increase Ca2+-sensitivity but do not uncouple.
Clinical Relevance of Uncoupling
Uncoupling inevitably leads to blunting of the response to β-adrenergic agonists but the lack of response to dobutamine is of course not only due to uncoupling. Heart failure is associated also with desensitization of β-receptors, such that the activation of PKA is attenuated, or the activity of phosphatase increased whilst coupling is intact (Houser and Margulies, 2003; Champion, 2005; El-Armouche et al., 2007; Messer et al., 2007).
Patient studies from the 80 and 90 s using echocardiography showed that IDCM and HCM patients could be classified into dobutamine responders and non-responders and that the non-responders have a poor prognosis whilst the responders can respond to treatment (Borow et al., 1988; Dubois-Randé et al., 1992; Naqvi et al., 1999) These studies predate the discovery of mutations in contractile proteins that cause familial DCM as well as the discovery of uncoupling, but given our current understanding of FDCM we would predict that the dobutamine non-responders correspond to those patients with FDCM mutations causing uncoupling and hence presumably the dobutamine response would be of clinical interest as a potential diagnostic to distinguish familial DCM from acquired IDCM.
This dichotomy would suggest that different treatments would be optimum for the two cases. Drugs are available that impact on β-receptors but so far no drugs act positively on the TnI-phosphorylation-Ca2+-sensitivity coupling mechanism. Our recent finding that EGCG is capable of recoupling in vitro, although it has different effects in vivo (Feng et al., 2012), suggests that specific modulation of the coupling process may be a viable target for future therapy.
Conflict of Interest Statement
The authors declare that the research was conducted in the absence of any commercial or financial relationships that could be construed as a potential conflict of interest.
Acknowledgment
This work was supported by grants from The British Heart Foundation (RG/11/20/29266 and FS/12/29568).
References
Al-Hillawi, E., Bhandari, D. G., Trayer, H. R., and Trayer, I. P. (1995). The effects of phosphorylation of cardiac troponin-I on its interactions with actin and troponin-C. Eur. J. Biochem. 228, 962–970. doi: 10.1111/j.1432-1033.1995.tb20347.x
Al-Hillawi, E., Chilton, D., Trayer, I. P., and Cummins, P. (1998). Phosphorylation-specific antibodies for human cardiac troponin-I. Eur. J. Biochem. 256, 535–540. doi: 10.1046/j.1432-1327.1998.2560535.x
Alves, M. L., Dias, F. A. L., Gaffin, R. D., Simon, J. N., Montminy, E. M., and Wolska, B. M. (2014). Desensitization of myofilaments to Ca2+ as a therapeutic target for hypertrophic cardiomyopathy with mutations in thin filament proteins. Circ. Cardiovasc. Gen. 7, 132–143. doi: 10.1161/CIRCGENETICS.113.000324
Ardelt, P., Dorka, P., Jaquet, K., Heilmeyer, L. M. G., Kortke, H., Korfer, R., et al. (1998). Microanalysis and distribution of cardiac troponin I phosphospecies in heart areas. Biol. Chem. 379, 341–347. doi: 10.1515/bchm.1998.379.3.341
Ayaz-Guner, S., Zhang, J., Li, L., Walker, J. W., and Ge, Y. (2009). In vivo phosphorylation site mapping in mouse cardiac troponin i by high resolution top-down electron capture dissociation mass spectrometry: Ser22/23 are the only sites basally phosphorylated. Biochemistry 48, 8161–8170. doi: 10.1021/bi900739f
Baryshnikova, O. K., Li, M. X., and Sykes, B. D. (2008). Modulation of cardiac troponin C function by the cardiac-specific N-terminus of troponin I: influence of PKA phosphorylation and involvement in cardiomyopathies. J. Mol. Biol. 375, 735–751. doi: 10.1016/j.jmb.2007.10.062
Bayliss, C., Messer, A., Leung, M.-C., Ward, D., Van Der Velden, J., Poggesi, C., et al. (2012a). *Functional investigation of troponin with the homozygous HCM mutation, TNNT2 K280M, obtained from an explanted heart. Cardiovasc. Res. 93, S107. doi: 10.1093/cvr/cvr336
Bayliss, C. R., Jacques, A. M., Leung, M.-C., Ward, D. G., Redwood, C. S., Gallon, C. E., et al. (2012b). Myofibrillar Ca2+-sensitivity is uncoupled from troponin i phosphorylation in hypertrophic obstructive cardiomyopathy due to abnormal troponin T. Cardiovasc. Res. 97, 500–508. doi: 10.1093/cvr/cvs322
Biesiadecki, B. J., Kobayashi, T., Walker, J. S., John Solaro, R., and De Tombe, P. P. (2007). The troponin C G159D mutation blunts myofilament desensitization induced by troponin I Ser23/24 phosphorylation. Circ. Res. 100, 1486–1493. doi: 10.1161/01.RES.0000267744.92677.7f
Bodor, G. S., Oakeley, A. E., Allen, P. D., Crimmins, D. L., Ladenson, J. H., and Anderson, P. A. W. (1997). Troponin I phosphorylation in the normal and failing adult human heart. Circulation 96, 1495–1500. doi: 10.1161/01.CIR.96.5.1495
Borow, K. M., Lang, R. M., Neumann, A., Carroll, J. D., and Rajfer, S. I. (1988). Physiologic mechanisms governing hemodynamic responses to positive inotropic therapy in patients with dilated cardiomyopathy. Circulation 77, 625–637. doi: 10.1161/01.CIR.77.3.625
Botten, D., Fugallo, G., Fraternali, F., and Molteni, C. (2013). A computational exploration of the interactions of the green tea polyphenol (-)-epigallocatechin 3-gallate with cardiac muscle troponin C. PLoS ONE 8:e70556. doi: 10.1371/journal.pone.0070556
Carballo, S., Robinson, P., Otway, R., Fatkin, D., Jongbloed, J. D., De Jonge, N., et al. (2009). Identification and functional characterization of cardiac troponin I as a novel disease gene in autosomal dominant dilated cardiomyopathy. Circ. Res. 105, 375–382. doi: 10.1161/CIRCRESAHA.109.196055
Carrier, L., Knoll, R., Vignier, N., Keller, D. I., Bausero, P., Prudhon, B., et al. (2004). Asymmetric septal hypertrophy in heterozygous cMyBP-C null mice. Cardiovasc. Res. 63, 293–304. doi: 10.1016/j.cardiores.2004.04.009
Champion, H. C. (2005). Targeting protein phosphatase 1 in heart failure. Circ. Res. 96, 708–710. doi: 10.1161/01.RES.0000164359.95588.25
Chang, A. N., and Potter, J. D. (2005). Sarcomeric protein mutations in dilated cardiomyopathy. Heart Fail. Rev. 10, 225–235. doi: 10.1007/s10741-005-5252-6
Copeland, O., Nowak, K., Laing, N., Ravenscroft, G., Messer, A. E., Bayliss, C. R., et al. (2010a). Investigation of changes in skeletal muscle alpha-actin expression in normal and pathological human and mouse hearts. J. Mus. Res. Cell. Motil. 31, 207–214. doi: 10.1007/s10974-010-9224-7
Copeland, O., Sadayappan, S., Messer, A. E., Stienen, G. J., Velden, J., and Marston, S. B. (2010b). Analysis of cardiac myosin binding protein-C phosphorylation in human heart muscle. J. Mol. Cell. Cardiol. 49, 1003–1011. doi: 10.1016/j.yjmcc.2010.09.007
Deng, Y., Schmidtmann, A., Kruse, S., Filatov, V., Heilmeyer, L. M. Jr., Jaquet, K., et al. (2003). Phosphorylation of human cardiac troponin I G203S and K206Q linked to familial hypertrophic cardiomyopathy affects actomyosin interaction in different ways. J. Mol. Cell. Cardiol. 35, 1365–1374. doi: 10.1016/j.yjmcc.2003.08.003
Deng, Y., Schmidtmann, A., Redlich, A., Westerdorf, B., Jaquet, K., and Thieleczek, R. (2001). Effects of phosphorylation and mutation R145G on human cardiac troponin I function. Biochemistry 40, 14593–14602. doi: 10.1021/bi0115232
Dohet, C., Al-Hillawi, E., Trayer, I. P., and Ruegg, J. C. (1995). Reconstitution of skinned cardiac fibres with human recombinant cardiac troponin-I mutants and troponin-C. FEBS Lett. 377, 131–134. doi: 10.1016/0014-5793(95)01319-9
Dong, W. J., Jayasundar, J. J., An, J., Xing, J., and Cheung, H. C. (2007). Effects of PKA phosphorylation of cardiac troponin I and strong crossbridge on conformational transitions of the N-domain of cardiac troponin C in regulated thin filaments. Biochemistry 46, 9752–9761. doi: 10.1021/bi700574n
Dong, W., Xing, J., Ouyang, Y., An, J., and Cheung, H. C. (2008). Structural kinetics of Cardiac troponin C mutants linked to familial hypertrophic and dilated cardiomyopathy in troponin complexes. J. Biol. Chem. 283, 3424–3432. doi: 10.1074/jbc.M703822200
Du, C. K., Morimoto, S., Nishii, K., Minakami, R., Ohta, M., Tadano, N., et al. (2007). Knock-in mouse model of dilated cardiomyopathy caused by troponin mutation. Circ. Res. 101, 185–194. doi: 10.1161/CIRCRESAHA.106.146670
Dubois-Randé, J. L., Merlet, P., Roudot, F., Benvenuti, C., Adnot, S., Hittinger, L., et al. (1992). Beta-adrenergic contractile reserve as a predictor of clinical outcome in patients with idiopathic dilated cardiomyopathy. Am. Heart J. 124, 679–685. doi: 10.1016/0002-8703(92)90278-4
Dyer, E., Jacques, A., Hoskins, A., Ward, D., Gallon, C., Messer, A., et al. (2009). Functional analysis of a unique troponin C mutation, Gly159Asp that causes familial dilated cardiomyopathy, studied in explanted heart muscle. Circ. Heart Fail. 2, 456–464. doi: 10.1161/CIRCHEARTFAILURE.108.818237
Dyer, E., Wells, D., Redwood, C., and Marston, S. B. (2007). *In vitro motility studies of HCM and DCM mutations in cardiac muscle actin. Biophys. J. 92, 481A.
El-Armouche, A., Pohlmann, L., Schlossarek, S., Starbatty, J., Yeh, Y. H., Nattel, S., et al. (2007). Decreased phosphorylation levels of cardiac myosin-binding protein-C in human and experimental heart failure. J. Mol. Cell. Cardiol. 43, 223–229. doi: 10.1016/j.yjmcc.2007.05.003
England, P. J. (1976). Studies on the phosphorylation of the inhibitory subunit of troponin during modification of contraction in perfused rat heart. Biochem. J. 160, 295–304.
Feng, W., Hwang, H. S., Kryshtal, D. O., Yang, T., Padilla, I. T., Tiwary, A. K., et al. (2012). Coordinated regulation of murine cardiomyocyte contractility by nanomolar (-)-epigallocatechin-3-gallate, the major green tea catechin. Mol. Pharmacol. 82, 993–1000. doi: 10.1124/mol.112.079707
Fentzke, R. C., Buck, S. H., Patel, J. R., Lin, H., Wolska, B. M., Stojanovic, M. O., et al. (1999). Impaired cardiomyocyte relaxation and diastolic function in transgenic mice expressing slow skeletal troponin I in the heart. J. Physiol. 517, 143–157. doi: 10.1111/j.1469-7793.1999.0143z.x
Ferrieres, G., Pugniere, M., Mani, J. C., Villard, S., Laprade, M., Doutre, P., et al. (2000). Systematic mapping of regions of human cardiac troponin I involved in binding to cardiac troponin C: N- and C-terminal low affinity contributing regions. FEBS Lett. 479, 99–105. doi: 10.1016/S0014-5793(00)01881-0
Gallon, C., Jacques, A., Messer, A., Tsang, V., McKenna, W., and Marston, S. (2007). *Altered functions and post-translational modification of contractile proteins in myectomy samples from HOCM patients. J. Mol. Cell. Cardiol. 42, S167. doi: 10.1016/j.yjmcc.2007.03.437
Gomes, A. V., Harada, K., and Potter, J. D. (2005). A mutation in the N-terminus of troponin I that is associated with hypertrophic cardiomyopathy affects the Ca(2+)-sensitivity, phosphorylation kinetics and proteolytic susceptibility of troponin. J. Mol. Cell. Cardiol. 39, 754–765. doi: 10.1016/j.yjmcc.2005.05.013
Gordon, A. M., Homsher, E., and Regnier, M. (2000). Regulation of contraction in striated muscle. Physiol. Rev. 80, 853–924.
Gould, I., Messer, A. E., Papadaki, M., and Marston, S. B. (2014). *Modulation of the interaction between troponin I N-terminal peptide and troponin C by phosphorylation studied by molecular dynamics. Biophys. J. 106, 349a.
Gramlich, M., Michely, B., Krohne, C., Heuser, A., Erdmann, B., Klaassen, S., et al. (2009). Stress-induced dilated cardiomyopathy in a knock-in mouse model mimicking human titin-based disease. J. Mol. Cell. Cardiol. 47, 352–358. doi: 10.1016/j.yjmcc.2009.04.014
Guinto, P. J., Haim, T. E., Dowell-Martino, C. C., Sibinga, N., and Tardiff, J. C. (2009). Temporal and mutation-specific alterations in Ca2+ homeostasis differentially determine the progression of cTnT-related cardiomyopathies in murine models. Am. J. Physiol. Heart Circ. Physiol. 297, H614–H626. doi: 10.1152/ajpheart.01143.2008
Hamdani, N., De Waard, M., Messer, A. E., Boontje, N. M., Kooij, V., Van Dijk, S., et al. (2009). Myofilament dysfunction in cardiac disease from mice to men. J. Musc. Res. Cell. Motil 29, 189–201. doi: 10.1007/s10974-008-9160-y
Haworth, R. S., Cuello, F., Herron, T. J., Franzen, G., Kentish, J. C., Gautel, M., et al. (2004). Protein kinase D is a novel mediator of cardiac troponin I phosphorylation and regulates myofilament function. Circ. Res. 95, 1091–1099. doi: 10.1161/01.RES.0000149299.34793.3c
Houser, S. R., and Margulies, K. B. (2003). Is depressed myocyte contractility centrally involved in heart failure? Circ. Res. 92, 350–358. doi: 10.1161/01.RES.0000060027.40275.A6
Howarth, J. W., Meller, J., Solaro, R. J., Trewhella, J., and Rosevear, P. R. (2007). Phosphorylation-dependent conformational transition of the cardiac specific N-extension of troponin I in cardiac troponin. J. Mol. Biol. 373, 706–722. doi: 10.1016/j.jmb.2007.08.035
Inoue, T., Kobirumaki-Shimozawa, F., Kagemoto, T., Fujii, T., Terui, T., Kusakari, Y., et al. (2013). Depressed Frank-Starling mechanism in the left ventricular muscle of the knock-in mouse model of dilated cardiomyopathy with troponin T deletion mutation ΔK210. J. Mol. Cell. Cardiol. 63, 69–78. doi: 10.1016/j.yjmcc.2013.07.001
Jacques, A., Briceno, N., Messer, A., Gallon, C., Jalizadeh, S., Garcia, E., et al. (2008). The molecular phenotype of human cardiac myosin associated with hypertrophic obstructive cardiomyopathy. Cardiovasc. Res. 79, 481–491. doi: 10.1093/cvr/cvn094
Jacques, A., Hoskins, A., Kentish, J., and Marston, S. B. (2009). From genotype to phenotype: a longitudinal study of a patient with hypertrophic cardiomyopathy due to a mutation in the MYBPC3 gene. J. Musc. Res. Cell. Motil. 29, 239–246. doi: 10.1007/s10974-009-9174-0
Javadpour, M. M., Tardiff, J. C., Pinz, I., and Ingwall, J., S. (2003). Decreased energetics in murine hearts bearing the R92Q mutation in cardiac troponin T. J. Clin. Invest. 112, 768–775. doi: 10.1172/JCI15967
Jweied, E., Detombe, P., and Buttrick, P. M. (2007). The use of human cardiac tissue in biophysical research: the risks of translation. J. Mol. Cell. Cardiol. 42, 722–726. doi: 10.1016/j.yjmcc.2007.02.002
Kaski, J. P., Burch, M., and Elliott, P. M. (2007). Mutations in the cardiac Troponin C gene are a cause of idiopathic dilated cardiomyopathy in childhood. Cardiol. Young 17, 675–677. doi: 10.1017/S1047951107001291
Keane, A. M., Trayer, I. P., Levine, B. A., Zeugner, C., and Ruegg, C. J. (1990). Peptide mimetics of an actin-binding site on myosin span two functional domains on actin. Nature 344, 265–268. doi: 10.1038/344265a0
Kentish, J. C., McCloskey, D. T., Layland, J., Palmer, S., Leiden, J. M., Martin, A. F., et al. (2001). Phosphorylation of troponin I by protein kinase A accelerates relaxation and crossbridge cycle kinetics in mouse ventricular muscle. Circ. Res. 88, 1059–1065. doi: 10.1161/hh1001.091640
Kinoshita, E., Kinoshita-Kikuta, E., Takiyama, K., and Koike, T. (2006). Phosphate-binding tag, a new tool to visualize phosphorylated proteins. Mol. Cell. Proteomics 5, 749–757. doi: 10.1074/mcp.T500024-MCP200
Knoell, R., Kostin, S., Klede, S., Savvatis, K., Klinge, L., Stehle, I., et al. (2010). A common MLP (muscle LIM protein) variant is associated with cardiomyopathy. Circ. Res. 106, 695–704. doi: 10.1161/CIRCRESAHA.109.206243
Knoell, R., Linke, W. A., Zou, P., Miocic, S., Kostin, S., Buyandelger, B., et al. (2011). Telethonin deficiency is associated with maladaptation to biomechanical stress in the mammalian heart. Circ. Res. 109, 758–769. doi: 10.1161/CIRCRESAHA.111.245787
Knollmann, B. C., Blatt, S. A., Horton, K., De Freitas, F., Miller, T., Bell, M., et al. (2001). Inotropic stimulation induces cardiac dysfunction in transgenic mice expressing a troponin T (I79N) mutation linked to familial hypertrophic cardiomyopathy. J. Biol. Chem. 276, 10039–10048. doi: 10.1074/jbc.M006745200
Kobayashi, T., Yang, X., Walker, L. A., Van Breemen, R. B., and Solaro, R. J. (2005). A non-equilibrium isoelectric focusing method to determine states of phosphorylation of cardiac troponin I: identification of Ser-23 and Ser-24 as significant sites of phosphorylation by protein kinase C. J. Mol. Cell. Cardiol. 38, 213–218. doi: 10.1016/j.yjmcc.2004.10.014
Kooij, V., Saes, M., Jaquet, K., Zaremba, R., Foster, D. B., Murphy, A., et al. (2010). Effect of troponin I Ser23/24 phosphorylation on Ca2+-sensitivity in human myocardium depends on the phosphorylation background. J. Mol. Cell. Cardiol. 48, 954–963. doi: 10.1016/j.yjmcc.2010.01.002
Layland, J., Solaro, R. J., and Shah, A. M. (2005). Regulation of cardiac contractile function by troponin I phosphorylation. Cardiovasc. Res. 66, 12–21. doi: 10.1016/j.cardiores.2004.12.022
Li, A. Y., Stevens, C. M., Liang, B., Rayani, K., Little, S., Davis, J., et al. (2013). Familial hypertrophic cardiomyopathy related cardiac troponin C L29Q mutation alters length-dependent activation and functional effects of phosphomimetic troponin I*. PLoS ONE 8:e79363. doi: 10.1371/journal.pone.0079363
Li, M. X., Robertson, I. M., and Sykes, B. D. (2008). Interaction of cardiac troponin with cardiotonic drugs: a structural perspective. Biochem. Biophys. Res. Commun. 369, 88–99. doi: 10.1016/j.bbrc.2007.12.108
Liu, B., Tikunova, S. B., Kline, K. P., Siddiqui, J. K., and Davis, J. P. (2012). Disease-related cardiac troponins alter thin filament Ca2+ association and dissociation rates. PLoS ONE 7:e38259. doi: 10.1371/journal.pone.0038259
Macleod, K. T., Marston, S. B., Poole-Wilson, P. A., Severs, N. J., and Sugden, P. H. (2010). “Cardiac myocytes and the cardiac action potential,” in Oxford Textbook of Medicine, 5th Edn. eds D. A. Warrel, T. M. Cox, and J. D. Firth (Oxford, UK: Oxford University Press), 2603–2617.
Mamidi, R., Gollapudi, S. K., Mallampalli, S. L., and Chandra, M. (2012). Alanine or aspartic acid substitutions at serine23/24 of cardiac troponin I decrease thin filament activation, with no effect on crossbridge detachment kinetics. Arch. Biochem. Biophys. 525, 1–8. doi: 10.1016/j.abb.2012.05.024
Manning, E. P., Tardiff, J. C., and Schwartz, S. D. (2011). A model of calcium activation of the cardiac thin filament. Biochemistry 50, 7405–7413. doi: 10.1021/bi200506k
Marston, S. B. (2011). How do mutations in contractile proteins cause the primary familial cardiomyopathies? J. Cardiovasc. Transl. Res. 4, 245–255. doi: 10.1007/s12265-011-9266-2
Marston, S. B., Copeland, O. A. N., and Messer, A. (2012). *Pressure overload is associated with low levels of troponin I and myosin binding protein C phosphorylation in the hearts of patients with aortic stenosis. Circulation 126, A14155.
Marston, S. B., Song, W., Wilkinson, R., and Vikhorev, P. G. (2013). DCM-causing mutation ACTC E361G blunts responses to adrener- gic agonists, reduces cardiac reserve and predispose to heart failure under chronic stress in a transgenic mouse model. J. Mol. Cell. Cardiol. 65, s108. doi: 10.1016/j.vjmcc.2013.10.011
Marston, S. B., and Walker, J. W. (2009). Back to the future: new techniques show that forgotten phosphorylation sites are present in contractile proteins of the heart whilst intensively studied sites appear to be absent. J. Mus. Res. Cell. Motil. 30, 93–95. doi: 10.1007/s10974-009-9184-y
Marston, S., and Detombe, P. (2008). Point/Counterpoint. Troponin phosphorylation and myofilament Ca2+-sensitivity in heart failure: increased or decreased? J. Mol. Cell. Cardiol. 45, 603–607. doi: 10.1016/j.yjmcc.2008.07.004
Memo, M., Leung, M.-C., Ward, D. G., Dos Remedios, C., Morimoto, S., Zhang, L., et al. (2013). Mutations in thin filament proteins that cause familial dilated cardiomyopathy uncouple troponin I phosphorylation from changes in myofibrillar Ca2+-sensitivity. Cardiovasc. Res. 99, 65–73. doi: 10.1093/cvr/cvt071
Messer, A. E., Jacques, A. M., and Marston, S. B. (2007). Troponin phosphorylation and regulatory function in human heart muscle: dephosphorylation of Ser23/24 on troponin I could account for the contractile defect in end-stage heart failure. J. Mol. Cell. Cardiol. 42, 247–259. doi: 10.1016/j.yjmcc.2006.08.017
Messer, A. E., Memo, M., Bayliss, C. R., Leung, M.-C., and Marston, S. B. (2012). *Does uncoupling of troponin I phosphorylation from changes in myofibrillar Ca2+-sensitivity play a role in the pathogenesis of cardiomyopathy? Biophys. J. 102, 556a. doi: 10.1016/j.bpj.2011.11.3033
Messer, A. E., Papadaki, M., and Marston, S. B. (2014). *Effects of EMD57033 and EGCG on Modulation of Ca2+-Sensitivity by Pka Phosphorylation. Biophys. J. 106, 726a–727a. doi: 10.1016/j.bpj.2013.11.4010
Messer, A., Gallon, C., McKenna, W., Elliott, P., Dos Remedios, C., and Marston, S. (2009). The use of phosphate-affinity SDS-PAGE to measure the troponin I phosphorylation site distribution in human heart muscle. Proteomics Clin. Appl. 3, 1371–1382. doi: 10.1002/prca.200900071
Mirza, M., Marston, S., Willott, R., Ashley, C., Mogensen, J., McKenna, W., et al. (2005). Dilated cardiomyopathy mutations in three thin filament regulatory proteins result in a common functional phenotype. J. Biol. Chem. 280, 28498–28506. doi: 10.1074/jbc.M412281200
Mittmann, K., Jaquet, K., and Heilmeyer, L. M. Jr. (1990). A common motif of two adjacent phosphoserines in bovine, rabbit and human cardiac troponin I. FEBS Lett. 273, 41–45. doi: 10.1016/0014-5793(90)81046-Q
Mogensen, J., Murphy, R. T., Shaw, T., Bahl, A., Redwood, C., Watkins, H., et al. (2004). Severe disease expression of cardiac troponin C and T mutations in patients with idiopathic dilated cardiomyopathy. J. Am. Coll. Cardiol. 44, 2033–2040. doi: 10.1016/j.jacc.2004.08.027
Moore, R. K., Grinspan, L. T., Jimenez, J., Guinto, P. J., Ertz-Berger, B., and Tardiff, J. C. (2013). HCM-linked Δ160E cardiac troponin T mutation causes unique progressive structural and molecular ventricular remodeling in transgenic mice. J. Mol. Cell. Cardiol. 58, 188–198. doi: 10.1016/j.yjmcc.2013.02.004
Mope, L., McClellan, G. B., and Winegrad, S. (1980). Calcium sensitivity of the contractile system and phosphorylation of troponin in hyperpermeable cardiac cells. J. Gen. Physiol. 75, 271–282. doi: 10.1085/jgp.75.3.271
Morimoto, S. (2008). Sarcomeric proteins and inherited cardiomyopathies. Cardiovasc. Res. 77, 659–666. doi: 10.1093/cvr/cvm084
Naqvi, T. Z., Goel, R. K., Forrester, J. S., and Siegel, R. J. (1999). Myocardial contractile reserve on dobutamine echocardiography predicts late spontaneous improvement in cardiac function in patients with recent onset idiopathic dilated cardiomyopathy. J. Am. Coll. Cardiol. 34, 1537–1544. doi: 10.1016/S0735-1097(99)00371-X
Nguyen, L., Chung, J., Lam, L., Tsoutsman, T., and Semsarian, C. (2007). Abnormal cardiac response to exercise in a murine model of familial hypertrophic cardiomyopathy. Int. J. Cardiol. 119, 245–248. doi: 10.1016/j.ijcard.2006.09.001
Nixon, B. R., Thawornkaiwong, A., Jin, J., Brundage, E. A., Little, S. C., Davis, J. P., et al. (2012). AMP-activated protein kinase phosphorylates cardiac troponin I at Ser-150 to increase myofilament calcium sensitivity and blunt PKA-dependent function. J. Biol. Chem. 287, 19136–19147. doi: 10.1074/jbc.M111.323048
Pi, Y.-Q., Kemnitz, K. R., Zhang, D., Kranias, E. G., and Walker, J. W. (2002). Phosphorylation of troponin I controls cardiac twitch dynamics. Evidence from phosphorylation site mutants expressed on a troponin I-null background in mice. Circ. Res. 90, 649–656. doi: 10.1161/01.RES.0000014080.82861.5F
Pinto, J. R., Siegfried, J. D., Parvatiyar, M. S., Li, D., Norton, N., Jones, M. A., et al. (2011). Functional characterization of TNNC1 rare variants identified in dilated cardiomyopathy. J. Biol. Chem. 286, 34404–34412. doi: 10.1074/jbc.M111.267211
Rajan, S., Ahmed, R. P., Jagatheesan, G., Petrashevskaya, N., Boivin, G. P., Urboniene, D., et al. (2007). Dilated cardiomyopathy mutant tropomyosin mice develop cardiac dysfunction with significantly decreased fractional shortening and myofilament calcium sensitivity. Circ. Res. 101, 205–214. doi: 10.1161/CIRCRESAHA.107.148379
Ray, K. P., and England, P. J. (1976). Phosphorylation of the inhibitory subunit of troponin and its effect on the calcium dependence of cardiac myofibril adenosine triphosphatase. FEBS Lett. 70, 11–16. doi: 10.1016/0014-5793(76)80716-8
Robertson, I. M., Li, M. X., and Sykes, B. D. (2009). Solution structure of human cardiac troponin C in complex with the green tea polyphenol, (-)-epigallocatechin 3-gallate. J. Biol. Chem. 284, 23012–23023. doi: 10.1074/jbc.M109.021352
Robertson, S. P., Johnson, J. D., Holroyde, M. J., Kranias, E. G., Potter, J. D., and Solaro, R. J. (1982). The effect of troponin I phosphorylation on the Ca2+-binding properties of the Ca2+-regulatory site of bovine cardiac troponin. J. Biol. Chem. 257, 260–263.
Sancho Solis, R., Ge, Y., and Walker, J. W. (2009). Single amino acid sequence polymorphisms in rat cardiac troponin revealed by top-down tandem mass spectrometry. J. Mus. Res. Cell. Motil. 29, 203–212. doi: 10.1007/s10974-009-9168-y
Schmidtmann, A., Lindow, C., Villard, S., Heuser, A., Mügge, A., Gessner, R., et al. (2005). Cardiac troponin C-L29Q, related to hypertrophic cardiomyopathy, hinders the transduction of the protein kinase A dependent phosphorylation signal from cardiac troponin I to C. FEBS J. 272, 6087–6097. doi: 10.1111/j.1742-4658.2005.05001.x
Sequeira, V., Wijnker, P. J., Nijenkamp, L. L., Kuster, D. W., Najafi, A., Witjas-Paalberends, E. R., et al. (2013). Perturbed length-dependent activation in human hypertrophic cardiomyopathy with missense sarcomeric gene mutations. Circ. Res. 112, 1491–1505. doi: 10.1161/CIRCRESAHA.111.300436
Solaro, R. J., Moir, A. G. J., and Perry, S. V. (1976). Phosphorylation of troponin I and the inotropic effect of adrenaline in the perfused rabbit heart. Nature 262, 615–616. doi: 10.1038/262615a0
Solaro, R. J., Rosevear, P., and Kobayashi, T. (2008). The unique functions of cardiac troponin I in the control of cardiac muscle contraction and relaxation. Biochem. Biophys. Res. Commun. 369, 82–87. doi: 10.1016/j.bbrc.2007.12.114
Song, W., Dyer, E., Stuckey, D., Copeland, O., Leung, M., Bayliss, C., et al. (2011). Molecular mechanism of the Glu99lys mutation in cardiac actin (ACTC gene) that causes apical hypertrophy in man and mouse. J. Biol. Chem. 286, 27582–27593. doi: 10.1074/jbc.M111.252320
Song, W., Dyer, E., Stuckey, D., Leung, M.-C., Memo, M., Mansfield, C., et al. (2010). Investigation of a transgenic mouse model of familial dilated cardiomyopathy. J. Mol. Cell. Cardiol. 49, 380–389. doi: 10.1016/j.yjmcc.2010.05.009
Steinberg, T. H., Agnew, B. J., Gee, K. R., Leung, W. Y., Goodman, T., Schulenberg, B., et al. (2003). Global quantitative phosphoprotein analysis using multiplexed proteomics technology. Proteomics 3, 1128–1144. doi: 10.1002/pmic.200300434
Stöhr, A., Friedrich, F. W., Flenner, F., Geertz, B., Eder, A., Schaaf, S., et al. (2013). Contractile abnormalities and altered drug response in engineered heart tissue from Mybpc3-targeted knock-in mice. J. Mol. Cell. Cardiol. 63, 189–198. doi: 10.1016/j.yjmcc.2013.07.011
Sun, N., Yazawa, M., Liu, J., Han, L., Sanchez-Freire, V., Abilez, O. J., et al. (2012). Patient-specific induced pluripotent stem cells as a model for familial dilated cardiomyopathy. Sci. Transl. Med. 4:130ra147. doi: 10.1126/scitranslmed.3003552
Tadano, N., Du, C., Yumoto, F., Morimoto, S., Ohta, M., Xie, M., et al. (2010). Biological actions of green tea catechins on cardiac troponin C. Br. J. Pharmacol. 161, 1034–1043. doi: 10.1111/j.1476-5381.2010.00942.x
Takeda, N., Yamashita, A., Maeda, K., and Maeda, Y. (2003). Structure of the core domain of human cardiac troponin in the Ca2+-saturated form. Nature 424, 35–41. doi: 10.1038/nature01780
Van Der Velden, J., Papp, Z., Zaremba, R., Boontje, N. M., De Jong, J. W., Owen, V. J., et al. (2003). Increased Ca2+-sensitivity of the contractile apparatus in end-stage human heart failure results from altered phosphorylation of contractile proteins. Cardiovasc. Res. 57, 37–47. doi: 10.1016/S0008-6363(02)00606-5
Vikhorev, S. W., Wilkinson, R., Copeland, O. A. N., Messer, A. E., Ferenczi, M. A., and Marston, S. B. (2013). Modulation of cardiac myofibril Ca2+-sensitivity by phosphorylation, sarcomere length and a DCM-causing mutation. Biophys. J. Rev. 104, 312a. doi: 10.1016/j.bpj.2012.11.1730
Wang, Y., Pinto, J. R., Solis, R. S., Dweck, D., Liang, J., Diaz-Perez, Z., et al. (2012). Generation and functional characterization of knock-in mice harboring the cardiac troponin I-R21C mutation associated with hypertrophic cardiomyopathy. J. Biol. Chem. 287, 2156–2167. doi: 10.1074/jbc.M111.294306
Wang, Y., Pinto, J., Sancho Solis, R., Dweck, D., Liang, J., Diaz-Perez, Z., et al. (2011). The generation and functional characterization of knock in mice harboring the cardiac Troponin I-R21C mutation associated with hypertrophic cardiomyopathy. J. Biol. Chem. 287, 2156–2167. doi: 10.1074/jbc.M111.294306
Ward, D. G., Brewer, S. M., Calvert, M. J., Gallon, C. E., Gao, Y., and Trayer, I. P. (2004a). Characterization of the interaction between the N-terminal extension of human cardiac troponin I and troponin C. Biochemistry 43, 4020–4027. doi: 10.1021/bi036128l
Ward, D. G., Brewer, S. M., Gallon, C. E., Gao, Y., Levine, B. A., and Trayer, I. P. (2004b). NMR and mutagenesis studies on the phosphorylation region of human cardiac troponin I. Biochemistry 43, 5772–5781. doi: 10.1021/bi036310m
Wilkinson, R. (2014). An Investigation Into the Disease Causing Mechanism in Familial Dilated Cardiomyopathy. Ph.D., Imperial College, London.
Wolff, M. R., Whitesell, L. F., and Moss, R. L. (1995). Calcium sensitivity of isometric tension is increased in canine experimental heart failure. Circ. Res. 76, 781–789. doi: 10.1161/01.RES.76.5.781
Yasuda, S., Coutu, P., Sadayappan, S., Robbins, J., and Metzger, J. M. (2007). Cardiac transgenic and gene transfer strategies converge to support an important role for troponin I in regulating relaxation in cardiac myocytes. Circ. Res. 101, 377–386. doi: 10.1161/CIRCRESAHA.106.145557
Zabrouskov, V., Ge, Y., Schwartz, J., and Walker, J. W. (2008). Unraveling molecular complexity of phosphorylated human cardiac troponin I by top down electron capture dissociation/electron transfer dissociation mass spectrometry. Mol. Cell. Proteomics 7, 1838–1849. doi: 10.1074/mcp.M700524-MCP200
Zaremba, R., Merkus, D., Hamdani, N., Lamers, J., Paulus, W., Dos Remedios, C., et al. (2007). Quantitative analysis of myofilament protein phosphorylation in small cardiac biopsies. Proteomics Clin. Appl. 1, 1285–1290. doi: 10.1002/prca.200600891
Keywords: troponin I, phosphorylation, cardiomyopathies, Ca sensitivity, heart muscle, myofilament
Citation: Messer AE and Marston SB (2014) Investigating the role of uncoupling of troponin I phosphorylation from changes in myofibrillar Ca2+-sensitivity in the pathogenesis of cardiomyopathy. Front. Physiol. 5:315. doi: 10.3389/fphys.2014.00315
Received: 20 June 2014; Accepted: 02 August 2014;
Published online: 25 August 2014.
Edited by:
Julien Ochala, KIng's College London, UKReviewed by:
Jose Renato Pinto, Florida State University, USARanganath Mamidi, Case Western Reserve University, USA
Copyright © 2014 Messer and Marston. This is an open-access article distributed under the terms of the Creative Commons Attribution License (CC BY). The use, distribution or reproduction in other forums is permitted, provided the original author(s) or licensor are credited and that the original publication in this journal is cited, in accordance with accepted academic practice. No use, distribution or reproduction is permitted which does not comply with these terms.
*Correspondence: Andrew E. Messer, Imperial Centre for Translational and Experimental Medicine, Hammersmith Campus, Du Cane Road, London, UK e-mail:YS5tZXNzZXJAaW1wZXJpYWwuYWMudWs=