- 1Department of Biophysics, Faculty of Science, Centre of Region Haná for Biotechnological and Agricultural Research, Palacký University, Olomouc, Czech Republic
- 2INSERM UMR 850, School of Pharmacy, University Limoges, Limoges, France
- 3Department of Physical Chemistry, Faculty of Science, Regional Centre of Advanced Technologies and Materials, Palacký University, Olomouc, Czech Republic
- 4Laboratory of Biotransformation, Institute of Microbiology, Czech Academy of Sciences, Prague, Czech Republic
We examined the inhibitory effects of three flavonolignans and their dehydro- derivatives, taxifolin and quercetin on the activity of the Na+/K+-ATPase (NKA). The flavonolignans silychristin, dehydrosilychristin and dehydrosilydianin inhibited NKA with IC50 of 110 ± 40 μM, 38 ± 8 μM, and 36 ± 14 μM, respectively. Using the methods of molecular modeling, we identified several possible binding sites for these species on NKA and proposed the possible mechanisms of inhibition. The binding to the extracellular- or cytoplasmic C-terminal sites can block the transport of cations through the plasma membrane, while the binding on the interface of cytoplasmic domains can inhibit the enzyme allosterically. Fluorescence spectroscopy experiments confirmed the interaction of these three species with the large cytoplasmic segment connecting transmembrane helices 4 and 5 (C45). The flavonolignans are distinct from the cardiac glycosides that are currently used in NKA treatment. Because their binding sites are different, the mechanism of inhibition is different as well as the range of active concentrations, one can expect that these new NKA inhibitors would exhibit also a different biomedical actions than cardiac glycosides.
Introduction
Sodium pump (Na+/K+-ATPase, E.C. 3.6.3.9, NKA) is an enzyme of crucial importance for all animal cells. It is the major determinant of cytoplasmic Na+ and K+ concentrations and the resting plasma membrane potential. The steep Na+ gradient on plasma membrane is essential for variety of secondary active transporters, e.g., Na+/Ca2+- and Na+/H+- exchanger or Na+-dependent glucose transporter, and hence, NKA indirectly regulates also concentrations of other physiologically important solutes.
It is not surprising that an uncontrolled inhibition of NKA can result in severe diseases, e.g., renal failure, hypertension or diabetic neuropathies (Kaplan, 2002) or even death, and that the most specific NKA inhibitor cardiac glycoside ouabain was originally used as an arrow poison (Newman et al., 2008). Despite these risks, extracts containing cardiac glycosides were used to control heart tonics already in ancient medicine, and the extracts were prepared either from plants in Arabic medicine (Brewer, 2004) or secretions of frog Bufo bufo in Chinese medicine (Watabe et al., 1996). Compounds like digitalis or digoxin are still prescribed for control of congestive heart failure (Gheorghiade et al., 2004). However, the use of cardiac glycosides is limited by their very narrow useful concentration range (Newman et al., 2008), which stimulates further search for other NKA inhibiting compounds.
Silymarin is an extract from the seeds of milk thistle (Silybum marianum). It contains numerous polyphenolic compounds and it was shown to possess antioxidant (Vacek et al., 2013; Pyszková et al., 2015), hepatoprotective (Loguercio and Festi, 2011) or anticancer effects (Agarwal et al., 2006). In this study, we have tested effects on NKA activity for a flavonoid taxifolin (TAX) and three flavonolignans, namely silybin (SB), silychristin (SCH), and silydianin (SD), which are major silymarin compounds (Biedermann et al., 2014); their structures are shown in Figure 1. The corresponding 2,3-dehydro derivatives (DHSB, DHSCH, and DHSD, the 2,3-dehydrotaxifolin is termed quercetin, QUE) were also tested.
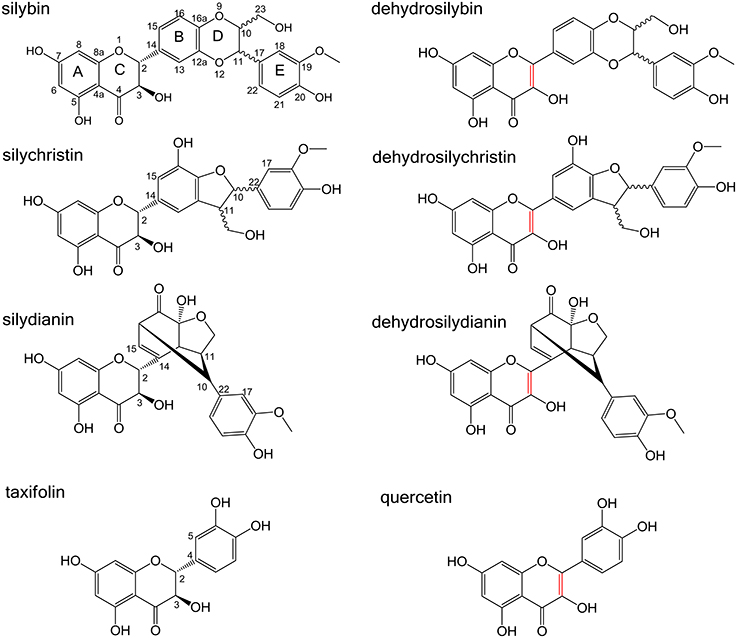
Figure 1. Structures of silybin (SB), silychristin (SCH), silydianin (SD) and their dehydro-derivatives (DHSB, DHSCH, DHSD), taxifolin (TAX), and quercetin (QUE). The 2,3-double bond in dehydroderivatives is highlighted in red.
The NKA catalytic cycle is usually described by the Albers-Post cycle (Jorgensen et al., 2003). It postulates that during the catalytic cycle, the enzyme adopts two major conformations, denoted as E1 and E2. In E1, the enzyme has high affinity to sodium and ATP and the binding sites are open to the cytoplasm, while in E2, the enzyme has high affinity to potassium, low affinity to ATP and the cation-binding sites are open to the extracellular space. High-resolution structures of NKA were obtained in several conformational states thanks to recent progresses in X-ray crystallography of membrane proteins (Morth et al., 2007; Ogawa et al., 2009; Nyblom et al., 2013). They revealed the binding sites for transported cations within the transmembrane domain, or binding site for some ligands, including ouabain. Notably, in the crystals assigned to the enzyme in E1 conformation, the cytoplasmic domains are assembled together (further referred to as a closed conformation), while in the E2 conformation, the cytoplasmic headpiece is widely opened (opened conformation).
These different structures provided a solid basis to figure out molecular processes responsible for the mechanisms of enzyme inhibition. Moreover, based on these high-resolution structures, molecular modeling allows further identification of the binding sites of flavonolignans that inhibit NKA, and strongly support rationalization of mechanisms of inhibition.
Materials and Methods
Chemicals
Unless stated otherwise, all used chemicals were from Sigma-Aldrich Chemie (Steinheim, Germany).
Species Tested for the Effect on NKA Activity
Silymarin was purchased from Liaoning Senrong Pharmaceutical Co., Ltd. (China), SB, SCH, and SD were isolated from the silymarin, and the dehydro- derivatives DHSB, DHSCH and DHSD were prepared as described previously (Džubák et al., 2006; Křenek et al., 2014; Pyszková et al., 2015). Silybin and silychristin were used as the natural diastereomeric mixture (ca. 1/1 and 95/5 respectively), silydianin is a single isomer. Dehydroderivatives were prepared from parent mixtures and are therefore enantiomeric mixtures (besides DHSD) (Pyszková et al., 2015). Taxifolin was purchased from Amagro (CZ). Quercetin was prepared by acidic hydrolysis of rutin with HCl/EtOH as described previously (Wang et al., 2011). Purity of all used compounds was over 95% (HPLC, PDA).
Isolation of Na+/K+-ATPase
The NKA was prepared from porcine kidney outer medulla using the method of Jorgensen and Klodos with some modifications (Jørgensen, 1988; Klodos et al., 2002; Kubala et al., 2014). An isolated enzyme was pipetted into small aliquots and stored at −20°C in ISE buffer (25 mM imidazole, 250 mM sucrose, 1 mM EDTA, pH 7.4) containing SDS detergent. The molar concentration of isolated NKA was estimated using the Bradford method with consideration of MW(α + β) = 165,000 Da. The protein purity >90% was estimated from SDS-PAGE (Figure 2).
Measurement of ATPase Activity
The measurements of NKA activity were performed using the Baginsky assay (Kubala et al., 2014). The assay was performed in microwell plates in 4 replicates for each point and automated in the pipetting station Freedom EVO (Tecan, Switzerland).
The reaction buffer was composed of 325 mM NaCl, 50 mM KCl, 7.5 mM MgCl2, and 75 mM imidazole, pH 7.2. In the experiments where the K+-dependence was estimated, the KCl concentration is ranging from 0 to 100 mM. The NKA (0.1 mg/mL) isolated from porcine kidney was mixed with reaction buffer without ATP. All inhibitors were solubilized in methanol immediately before the measurement and then premixed with the reaction buffer to the required concentration. Subsequently, 10 μL of inhibitor solution was added into 20 μL of enzyme solution and incubated for 2 min. In the control sample, only 10 μL of reaction buffer without inhibitor was added including corresponding amount of methanol. The reaction was started by the addition of ATP solution (20 μL, 7.5 mM in the stock, the final concentration in the reaction was 3 mM). The reaction proceeded for 6 min at room temperature and then was stopped by addition of 75 μL of staining solution, which was composed of 160 mM ascorbic acid, 3.7% (v/v) acetic acid, 3% (w/v) SDS, and 0.5% ammonium molybdate. The staining reaction was stopped after another 8 min by adding 125 μL of solution composed of 0.9% (w/v) bismuth citrate, 0.9% (w/v) sodium citrate and 3.7% HCl.
The Baginsky method detects a product of ATP hydrolysis, inorganic phosphate, which interacts with ammonium molybdate. The reaction results in a color change, which can be monitored as a change of absorbance at 710 nm, and was measured using microplate reader Synergy Mx (BioTek, USA). The calibration line was determined using KH2PO4 solutions, in 0–37.5 nM concentration range.
The specific NKA activity is standardly estimated using the treatment by ouabain, which serves as a specific inhibitor of NKA. The ATPase activity decreases to less than 10% in the presence of 10 mM ouabain. This residual activity in the presence of ouabain was subtracted from the total estimated ATPase activity in ouabain-untreated samples, and all data are presented as the ouabain-sensitive ATPase activity. The IC50 values were obtained from fitting the data to the logistic function.
Expression and Purification of the Isolated Large Cytoplasmic Segment C45
The large cytoplasmic segment connecting the transmembrane helices 4 and 5 (C45 loop, residues L354-I777 of the mouse brain sequence) with a (His)6-tag at the N-terminus was expressed in E. coli BL21 (Promega, USA) and purified using a Co2+-based affinity resin (Clontech, USA) as described previously (Grycova et al., 2009). Immediately after elution, the protein was dialyzed into 20 mM Tris, 140 mM NaCl, pH 7.4 buffer and stored at −20°C.
Protein were analyzed by Coomassie blue stained SDS PAGE and concentration was determined using the Bradford assay (Bradford, 1976) using BSA as a standard.
Absorption and Fluorescence Spectroscopy
Spectroscopic experiments were performed in 20 mM Tris, 140 mM NaCl, pH 7.4 buffer. SCH, DHSCH and DHSD as well as protein C45 were diluted to 5 μM concentration.
Absorption spectra were measured on spectrometer Specord 250 Plus (Analytic Jena, Germany) in the range 300–600 nm, with the bandpass 2 nm, a step 1 nm and a scan-speed 2 nm/s. The reference spectrum was acquired using the cuvette with a pure buffer.
Fluorescence emission spectra were measured using Fluorolog-3 (Horiba Scientific, USA). The spectra were scanned with a step of 1 nm, both excitation- and emission bandpass 5 nm and integration time 1 s. For SCH, the excitation was 325 nm and emission was recorded in the 340–600 nm interval, for DHSCH and DHSD, the excitation wavelength was 380 nm and spectra were scanned in the 400–600 nm interval. Signal from pure buffer was subtracted as a background.
Computation of Structures for Molecular Docking
Geometry optimizations of all structures (Figure 1) was performed using the density functional theory (DFT) formalism with the software package Gaussian09 (Frisch et al., 2009). The hybrid functional B3P86 has been used because it has repeatedly succeeded in describing most of polyphenol properties (Trouillas et al., 2006, 2008). Gibbs energies (G) were computed at B3P86/6-31+G(d,p) level at 298 K, 1 atm. After a vibrational frequency analysis, ground-state geometries were confirmed by the absence of any imaginary frequency. Quantum calculations were performed in the gas phase.
The SB (stereoisomer A) and DHSB (stereoisomer A) initial structures were taken from Trouillas et al. (2008) and further re-optimized. Structure of the most stable isomers of SCH (stereoisomer A), DHSCH (stereoisomer A), SD and DHSD were already presented in Pyszková et al. (2015).
Molecular Docking
The compounds SCH, DHSCH, and DHSD were docked to the opened and closed structures of NKA (PDB, ID, 3KDP, and 4HQJ) using Autodock Tools (Morris et al., 2009) and Autodock Vina (Trott and Olson, 2010) with the grid covering the whole protein. The values of parameters exhaustiveness was set to 100 and num_modes to 9999 in order to reveal all possible docking modes. In the default setting, the bonds creating different conformers were freely rotable, to find optimal geometry of molecules interacting with the pump.
Results
Conformational Analysis
Conformational analysis of both flavonoids TAX and its dehydro- analog QUE revealed two stable rotamers. In case of QUE, as reported previously (Trouillas et al., 2006), the planarity is observed, allowing π electron delocalization along the A, C and B rings, while for TAX, the planarity is lost due to the loss of the 2,3-double bond.
A conformation analysis of SB, DHSB, SCH, DHSCH, SD, and DHSD revealed 4 most stable representative conformers for all molecules within less than 1.6 kcal/mol difference in Gibbs energy (Figure 3). These conformers can be obtained by modification of the torsion angles Phi = C3–C2–C14–C15 and Psi = C11–C10–C22–C17 (Psi = C10–C11–C17–C22 for SB and DHSB) (Table 1), and the loss of the flavonoid moiety planarity is observed also for these molecules, when the 2,3-bond is hydrogenated (DHSB, DHSCH, and DHSD).
Effects on NKA Activity
Ouabain-sensitive ATPase activity was measured for increasing concentrations of all species (Figure 4). In the case of SB, DHSB, SD, and DHSD, the examined concentration range was limited due to lower solubility of these species. Substantial inhibition was observed only for SCH, DHSCH and DHSD with IC50 110 ± 40 μM, 38 ± 8 μM, and 36 ± 14 μM, respectively. In all these cases, we observed a Hill coefficient < 1, indicating the presence of multiple binding sites and negative cooperativity. The data are summarized in Table 2, and SCH, DHSCH, and DHSD were subject to further analyses.
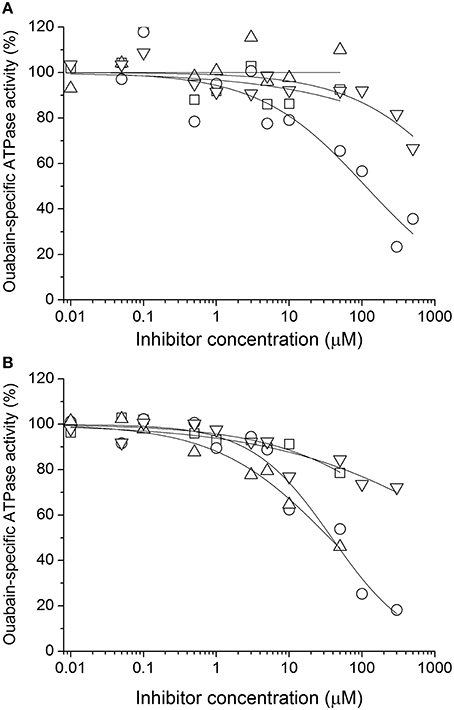
Figure 4. Inhibition of NKA by (A) SB (squares), SCH (circles), SD (up triangles), TAX (down triangles) and (B) by the corresponding dehydro- derivatives DHSB, DHSCH, DHSD and QUE. The reference value (100%) represents ouabain-specific activity of untreated NKA.
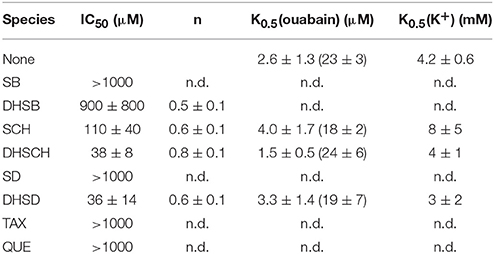
Table 2. Values of IC50 for inhibition of the NKA activity, n, Hill coefficient, K0.5(ouabain) indicates the value obtained in the presence of 5 mM KCl (in parentheses in 40 mM KCl) and 40 μM concentration of flavonolignan, K0.5(K+) denotes the values for K+-dependent activation of NKA obtained in the presence of 40 μM flavonolignan, n.d., not determined.
We have tested influence of these three species on the ouabain inhibition and K+-dependence of NKA activation. None of the species substantially influenced the IC50 for ouabain or the K+/ouabain antagonism (Figure 5). The NKA activity is K+-dependent and it increased with increasing concentration of potassium with K0.5(K+) = 4.2 ± 0.6 mM. In contrast to ouabain, which raised the K0.5(K+) to 14.8 ± 0.1 mM, none of the flavonolignans significantly altered the K+-dependence of NKA activity (Figure 6).
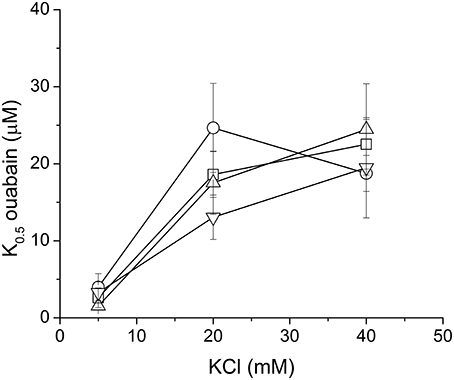
Figure 5. The K+/ouabain antagonism in the absence (squares) or in the presence of 40 μM SCH (circles), DHSCH (up triangles) or DHSD (down triangles).
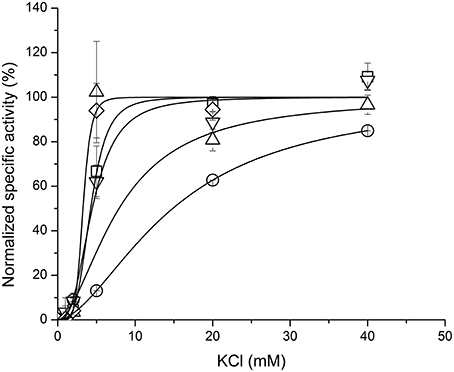
Figure 6. The K+-dependence of NKA activity in the absence of any ligand (squares) or in the presence of ouabain (circles), SCH (up triangles), DHSCH (down triangles) or DHSD (diamonds).
Molecular Docking
Molecular docking enables prediction of the binding sites for small ligands on large biomolecules. Binding to NKA was examined for both major conformations of the enzyme. All SCH, DHSCH, and DHSD bound with a similar affinities (−11 to −9 kcal/mol) to all sites in both opened and closed conformations. We identified five major binding sites, three of them were common to both the opened and closed conformations (Figure 7), one binding pose was exclusively observed only for the opened conformation, and another one in turn only for closed conformation. All conformers bound to at least one binding site, nevertheless, their relative preferences for individual binding sites differed (Table 3).
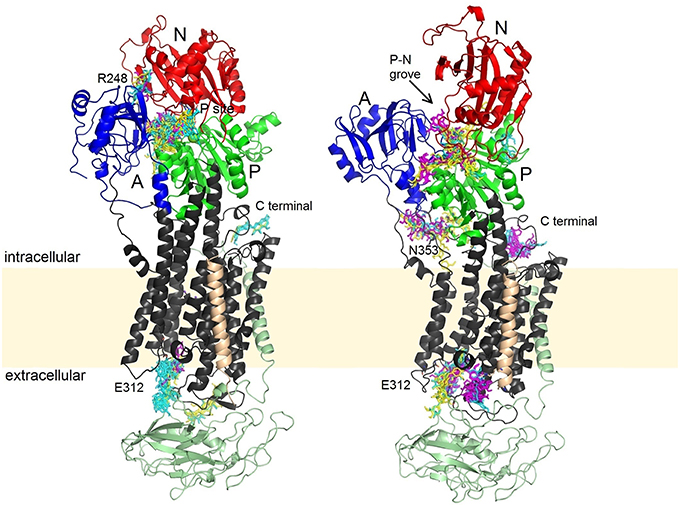
Figure 7. Binding sites of SCH (yellow), DHSCH (cyan) and DHSD (magenta) on the opened and closed structure. Beta subunit is in light green, the FXYD protein light orange, A, domain blue; N, domain red; P, domain green.
In the closed conformation, by far the most favored site was near the phosphorylation site. All the conformers can occupy this site, except for conformer 4 of DHSCH. All compounds bound also to another pose between the A and N domains on the other side near R248 (Figure 8). However, this site only exists in the closed conformation. It is much more selective and can be occupied only by conformer 3 of SCH, conformers 2 and 4 of DHSCH and conformer 1 of DHSD. Another possible binding location in the closed structure is on the extracellular side between E312 on TM4 and R886 (extracellular loop connecting TM7 and TM8), and it is the most favored site for conformer 4 of DHSCH. This site can serve as the entry for potassium ions during transport. Conformer 1 of SCH and conformers 1 and 4 of DHSCH are the only molecules that bind at the intracellular C-terminal site. Also this site was proposed to play a role in transport of cations through the plasma membrane (Toustrup-Jensen et al., 2009).
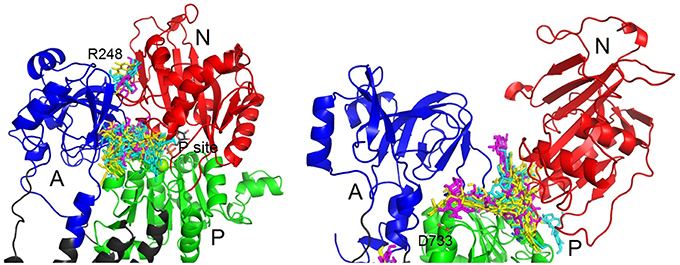
Figure 8. Zoom on the binding sites of SCH (yellow), DHSCH (cyan) and DHSD (magenta) on cytoplasmic loops in the opened and closed structure. A, domain is blue; N, domain red; P, domain green.
Also in the opened conformation, the binding to the groove between P and N cytoplasmic domains near the phosphorylation site was observed for all conformers, except for conformer 3 of DHSCH. It was the most preferred site for all SCH conformers and for conformers 1 of DHSCH and DHSD. The binding site specific only for the opened conformation is located under A domain, near the residues N353 and D740, where a regulatory potassium ion is bound (Schack et al., 2008). It is the most preferred site for conformers 2 and 4 of DHSCH and for conformer 2 of DHSD, but numerous other conformers can bind in this site as well. The site at the extracellular potassium entrance is the most preferred for the conformer 4 of DHSD. There is also a binding pose at the C-terminal of the protein, but it can be occupied only by DHSCH or DHSD, and for conformer 3 of both species it is the most preferred site.
Interaction with the Isolated Large Cytoplasmic Segment C45
Based on the prediction from molecular docking, we examined whether SCH, DHSCH, and DHSD could interact with the isolated large cytoplasmic segment C45 using absorption and fluorescence spectroscopy. Absorption spectra of SCH, DHSCH, and DHSD exhibited maxima at ca. 325, 385, and 375 nm, respectively, and the presence of C45 did not substantially alter the spectra (not shown), thus, providing no further clue about the interaction.
On the other hand, the interaction with C45 was clearly manifested in the fluorescence spectra for all three species. We observed no detectable fluorescence above background for the free SCH. The interaction with C45 protein turned out to be fluorogenic, and in the presence of C45, a bright SCH fluorescence appeared (quantum yield increased more than 1000 times compared to the free SCH) with a maximum at 394 nm (Figure 9). For the free DHSCH, we observed only very weak fluorescence with a maximum at 537 nm and an apparent shoulder at ca. 475 nm. In the presence of C45, the fluorescence intensity increased ca. 3-times, and two peaks at 466 and 527 nm could be resolved. Similarly for the free DHSD, there is only a weak fluorescence with a maximum at 472 nm. In the presence of C45, the three-fold intensity increase is accompanied by a shift of the maximum to 459 nm, and there is also an apparent shoulder at 510 nm.
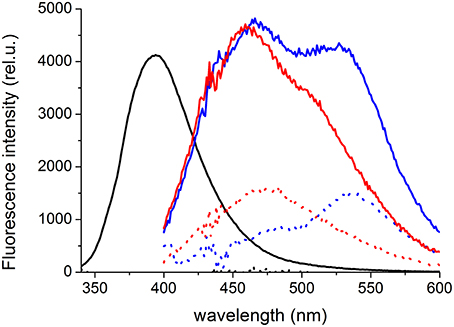
Figure 9. Fluorescence emission spectra of 5 μM SCH (black), DHSCH (blue) and DHSD (red) in the presence (solid line) or absence (dotted line) of 5 μM C45. The fluorescence intensity in the spectrum of SCH in the C45 presence was divided by a factor of 20 to fit the graph.
Discussion
Recent pharmacology greatly benefits from the species isolated from the plants used in traditional medicine. In addition, these species also serve as precursors or inspiration for synthesis of new active derivatives, which can turn out to be even more effective in biochemical interactions. This study was focused on interactions of a series of phenolic compounds from silymarin (being or not hydrogenated on 2,3-bond of the flavonoid moiety) with one of the most important enzymes in the animal metabolism, the NKA.
Bioavailability of most polyphenolic compounds naturally found in silymarin is limited by their lower solubility in water, only SCH and SD exhibit substantially higher solubility in aqueous environment. In our experiments, we observed that only SCH inhibited the NKA with IC50 of 110 ± 40 μM, and similar concentrations can hardly be reached within the living organism. On the other hand, two of the 2,3-dehydroderivatives, DHSCH and DHSD, inhibited NKA substantially more efficiently with IC50 of 38 ± 8 μM and 36 ± 14 μM, respectively. Interestingly, none of the flavonolignans exhibited influence on the known K+/ouabain antagonism (Müller-Ehmsen et al., 2001). Moreover, in contrast to ouabain, they did not alter the K+-dependence of NKA activity, suggesting that the flavonolignans have different mode of inhibition than ouabain.
Interaction of small molecules with large biomolecules is one of the key issues in structural biology, and recently, it has gained benefits from the development in computational techniques. The classical key-and-lock concept assumes that small molecules can specifically interact with enzymes when their geometries fit each other. The induced-fit concept that was proposed later, has not that strict requirements on the geometries of the interaction partners, and it assumes that after the first contact, the enzyme can rearrange its conformation to fit the shape of the small ligand (Koshland, 1995).
Our calculations revealed that all flavonolignans (including the dehydro- derivatives) can adopt several stable conformations. Their stabilizing energies calculated in the gas phase appeared very similar, suggesting that they are all present in solution in roughly equimolar mixtures. Although differences between flavonolignans conformers may play no important role in, e.g., their redox properties, the precise geometry is crucial in the interaction with enzymes, and some conformers can be tightly bound to the enzyme, while the others can be inactive.
However, the situation is probably more complex in our case. The fitting of the inhibition curves revealed the Hill coefficient different from 1 for all SCH, DHSCH, and DHSD, indicating the presence of multiple binding sites. Indeed, molecular docking revealed one extracellular binding site and four other sites in the cytoplasmic segment of the protein, indicating multiple possibilities of how the flavonolignans could inhibit the enzyme. The main enzyme function is translocation of cations through the plasma membrane. The cations are transiently bound to their binding sites formed by residues TM4, TM5, TM6, and TM8 in the transmembrane domain (Morth et al., 2007), while the extracellular- and cytoplasmic pathways to these binding sites alternatively open and close. Binding of flavonolignans to the extracellular binding site or to the C-terminal binding site can efficiently block these pathways, and thus, can stop the cation transport. The opening and closing of the extracellular- and cytoplasmic gates is accompanied by the change in the position of cytoplasmic domains, as a consequence of ATP-binding and hydrolysis (Kubala, 2006). Localization of the flavonolignan on the interfaces between N- and P- or N- and A-domains can hinder the interactions between cytoplasmic domains, and thus, inhibit the enzyme allosterically. All conformers of all SCH, DHSCH, and DHSD were able to bind to at least to one binding pose, but by far the most occupied site was the one at the interface of N- and P-domains near the phosphorylation site. It can be occupied by almost all conformers, it is present in both opened and closed enzyme conformations, and we experimentally verified binding of all SCH, DHSCH, and DHSD to the large cytoplasmic segment connecting TM4 and TM5 (C45) using fluorometry.
The C45 constitutes approx. 40% of the enzyme mass and forms the cytoplasmic domains N and P. It can be overexpressed without the rest of the enzyme in high quantities in E. coli (Grycova et al., 2009). So far, all the experiments indicate that it retains its structure, ability to bind ATP and TNP-ATP (Kubala et al., 2003a,b, 2004), and dynamic properties (Grycova et al., 2009; Kubala et al., 2009) as when it is a part of the entire enzyme. Its solubility greatly facilitates all subsequent experiments and this model system was successfully used for closer localization of binding sites of numerous other small molecules on the cytoplasmic part of NKA (Huličiak et al., 2012; Havlíková et al., 2013). Also in the case of SCH, DHSCH and DHSD, the spectroscopic experiments unambiguously confirmed that these molecules interact with C45. Moreover, in the case of DHSCH and DHSD, we could observe two peaks in the emission spectra of the protein-bound forms. It reveals that there are two binding modes of these species to the C45 and it is in line with predictions from molecular docking.
In conclusion, flavonolignans are proposed to be a novel class of NKA inhibitors, and particularly the 2,3-dehydroderivatives DHSCH and DHSD seem to be very promising agents. The flavonolignans are distinct from the cardiac glycosides that are currently used in NKA treatment. Because their binding sites are different, the mechanism of inhibition is different as well as the range of active concentrations, one can expect that these new NKA inhibitors would exhibit also a different biomedical actions than cardiac glycosides. Currently, the major problem seems to be the low specificity in interaction with biomolecules, which is probably partially related to significant number of stable flavonolignan conformers. Syntheses of more than 100 flavonolignan derivatives have been described (Biedermann et al., 2014), and further screening could identify a derivative that would be useful at lower concentrations and with higher specificity.
Author Contributions
MK designed the study, prepared the manuscript, performed the absorption- and fluorescence spectroscopy experiments. PČ performed the molecular docking computations, contributed to the interpretation of data. JG isolated NKA and performed the ATPase activity measurements. MB performed the conformational analysis and prepared the flavonolignan molecules for docking. TŠ expressed and purified the C45 protein for spectroscopic analyses. PT participated to the conformational analysis and manuscript preparation. DB isolated, prepared, purified and supplied the compounds studied.
Funding
This work was supported by the grant LO1024 from the National Program of Sustainability I, by scholarship for MB from the French Embassy in the Czech Republic, and by grants 15-03037S and P208/12/G016 from the Czech Science Foundation.
Conflict of Interest Statement
The authors declare that the research was conducted in the absence of any commercial or financial relationships that could be construed as a potential conflict of interest.
Acknowledgments
MB and PT wish to thank CALI (CAlcul en LImousin), where the calculations were performed. Prof. J. Ulrichová from the Dept. of Medical Chemistry and Biochemistry, Faculty of Medicine and Dentistry, Palacký University, is acknowledged for the logistic support and discusions.
References
Agarwal, R., Agarwal, C., Ichikawa, H., Singh, R. P., and Aggarwal, B. B. (2006). Anticancer potential of silymarin: from bench to bed side. Anticancer Res. 26, 4457–4498.
Biedermann, D., Vavříková, E., Cvak, L., and Křen, V. (2014). Chemistry of silybin. Nat. Prod. Rep. 31, 1138–1157. doi: 10.1039/C3NP70122K
Bradford, M. M. (1976). A rapid and sensitive method for the quantitation of microgram quantities of protein utilizing the principle of protein-dye binding. Anal. Biochem. 72, 248–254.
Brewer, H. (2004). Historical perspectives on health: early Arabic medicine. J. R. Soc. Promot. Health. 124, 184–187. doi: 10.1177/146642400412400412
Džubák, P., Hajdúch, M., Gažák, R., Svobodová, A., Psotová, J., Walterová, D., et al. (2006). New derivatives of silybin and 2,3-dehydrosilybin and their cytotoxic and P-glycoprotein modulatory activity. Bioorg. Med. Chem. 14, 3793–3810. doi: 10.1016/j.bmc.2006.01.035
Frisch, M. J., Trucks, G. W., Schlegel, H. B., Scuseria, G. E., Robb, M. A., Cheeseman, J. R., et al. (2009). Gaussian 09, version A02. Wallingford, CT: Gaussian. Inc.
Gheorghiade, M., Adams, K. F. Jr., and Colucci, W. S. (2004). Digoxin in the management of cardiovascular disorders. Circulation 109, 2959–2964. doi: 10.1161/01.CIR.0000132482.95686.87
Grycova, L., Sklenovsky, P., Lansky, Z., Janovska, M., Otyepka, M., Amler, E., et al. (2009). ATP and magnesium drive conformational changes of the Na+/K+-ATPase cytoplasmic headpiece. Biochim. Biophys. Acta 1788, 1081–1091. doi: 10.1016/j.bbamem.2009.02.004
Havlíková, M., Huličiak, M., Bazgier, V., Berka, K., and Kubala, M. (2013). Fluorone dyes have binding sites on both cytoplasmic and extracellular domains of Na,K-ATPase. Biochim. Biophys. Acta 1828, 568–576. doi: 10.1016/j.bbamem.2012.10.029
Huličiak, M., Vacek, J., Šebela, M., Orolinová, E., Znaleziona, J., Havlíková, M., et al. (2012). Covalent binding of cisplatin impairs the function of Na+/K+-ATPase by binding to its cytoplasmic part. Biochem. Pharmacol. 83, 1507–1513. doi: 10.1016/j.bcp.2012.02.015
Jørgensen, P. L. (1988). Biomembranes Part P: ATP-driven pumps and related transport: the Na,K-Pump. Methods Enzymol. 156, 29–43.
Jorgensen, P. L., Håkansson, K. O., and Karlish, S. J. D. (2003). Structure and mechanism of Na,K-ATPase: functional sites and their interactions. Annu. Rev. Physiol. 65, 817–849. doi: 10.1146/annurev.physiol.65.092101.142558
Kaplan, J. H. (2002). Biochemistry of Na,K-ATPase. Annu. Rev. Biochem. 71, 511–535. doi: 10.1146/annurev.biochem.71.102201.141218
Klodos, I., Esmann, M., and Post, R. L. (2002). Large-scale preparation of sodium-potassium ATPase from kidney outer medulla. Kidney Int. 62, 2097–2100. doi: 10.1046/j.1523-1755.2002.00654.x
Koshland, D. E. (1995). the key–lock theory and the induced fit theory. Angew. Chemie Int. Ed English. 33, 2375–2378. doi: 10.1002/anie.199423751
Křenek, K., Marhol, P., Peikerová, Z., Křen, V., and Biedermann, D. (2014). Preparatory separation of the silymarin flavonolignans by Sephadex LH-20 gel. Food Res. Int. 65, 115–120. doi: 10.1016/j.foodres.2014.02.001
Kubala, M. (2006). ATP-binding to P-type ATPases as revealed by biochemical, spectroscopic, and crystallographic experiments. Proteins 64, 1–12. doi: 10.1002/prot.20969
Kubala, M., Geleticova, J., Huliciak, M., Zatloukalova, M., Vacek, J., and Sebela, M. (2014). Na+/K+-ATPase inhibition by cisplatin and consequences for cisplatin nephrotoxicity. Biomed. Pap. 158, 194–200. doi: 10.5507/bp.2014.018
Kubala, M., Grycova, L., Lansky, Z., Sklenovsky, P., Janovska, M., Otyepka, M., et al. (2009). Changes in electrostatic surface potential of Na+/K+-ATPase cytoplasmic headpiece induced by cytoplasmic ligand(s) binding. Biophys. J. 97, 1756–1764. doi: 10.1016/j.bpj.2009.07.002
Kubala, M., Plášek, J., and Amler, E. (2003b). Limitations in linearized analyses of binding equilibria: binding of TNP-ATP to the H4-H5 loop of Na/K-ATPase. Eur. Biophys. J. 32, 363–369. doi: 10.1007/s00249-003-0278-y
Kubala, M., Plášek, J., and Amler, E. (2004). Fluorescence competition assay for the assessment of ATP binding to an isolated domain of Na+,K+-ATPase. Physiol. Res. 53, 109–113. doi: 10.1021/bi010270
Kubala, M., Teisinger, J., Ettrich, R., Hofbauerová, K., Kopecký, V., Baumruk, V., et al. (2003a). Eight amino acids form the ATP recognition site of Na+/K+-ATPase. Biochemistry 42, 6446–6452. doi: 10.1021/bi034162u
Loguercio, C., and Festi, D. (2011). Silybin and the liver: from basic research to clinical practice. World J. Gastroenterol. 17:2288. doi: 10.3748/wjg.v17.i18.2288
Morris, G. M., Huey, R., Lindstrom, W., Sanner, M. F., Belew, R. K., Goodsell, D. S., et al. (2009). AutoDock4 and AutoDockTools4: automated docking with selective receptor flexibility. J. Comput. Chem. 30, 2785–2791. doi: 10.1002/jcc.21256
Morth, J. P., Pedersen, B. P., Toustrup-Jensen, M. S., Sørensen, T. L.-M., Petersen, J., Andersen, J. P., et al. (2007). Crystal structure of the sodium–potassium pump. Nature 450, 1043–1049. doi: 10.1038/nature06419
Müller-Ehmsen, J., Juvvadi, P., Thompson, C. B., Tumyan, L., Croyle, M., Lingrel, J. B., et al. (2001). Ouabain and substrate affinities of human Na+ -K+ -ATPase α1β1, α2β1, and α3β1 when expressed separately in yeast cells. Am. J. Physiol. Cell Physiol. 281, 1355–1364.
Newman, R. A., Yang, P., Pawlus, A. D., and Block, K. I. (2008). Cardiac glycosides as novel cancer therapeutic agents. Mol. Interv. 8, 36–49. doi: 10.1124/mi.8.1.8
Nyblom, M., Poulsen, H., Gourdon, P., Andersson, M., Lindahl, E., and Fedosova, N. (2013). Crystal structure of Na+, K+-ATPase in the Na+-bound state. Science 342, 123–127. doi: 10.1126/science.1243352
Ogawa, H., Shinoda, T., Cornelius, F., and Toyoshima, C. (2009). Crystal structure of the sodium-potassium pump (Na+,K+-ATPase) with bound potassium and ouabain. Proc. Natl. Acad. Sci. U.S.A. 106, 13742–13747. doi: 10.1073/pnas.0907054106
Pyszková, M., Biler, M., Biedermann, D., Valentová, K., Kuzma, M., Vrba, J., et al. (2015). Flavonolignan 2,3-dehydroderivatives: preparation, antiradical and cytoprotective activity. Free Radic. Biol. Med. 90, 114–125. doi: 10.1016/j.freeradbiomed.2015.11.014
Schack, V. R., Morth, J. P., Toustrup-Jensen, M. S., Anthonisen, A. N., Nissen, P., Andersen, J. P., et al. (2008). Identification and function of a cytoplasmic K+ site of the Na+,K+-ATPase. J. Biol. Chem. 283, 27982–27990. doi: 10.1074/jbc.M803506200
Toustrup-Jensen, M. S., Holm, R., Einholm, A. P., Schack, V. R., Morth, J. P., Nissen, P., et al. (2009). The C terminus of Na+,K+-ATPase controls Na+ affinity on both sides of the membrane through Arg935. J. Biol. Chem. 284, 18715–18725. doi: 10.1074/jbc.M109.015099
Trott, O., and Olson, A. J. (2010). AutoDock Vina: improving the speed and accuracy of docking with a new scoring function, efficient optimization, and multithreading. J. Comput. Chem. 31, 455–461. doi: 10.1002/jcc.21334
Trouillas, P., Marsal, P., Siri, D., Lazzaroni, R., and Duroux, J.-L. (2006). A DFT study of the reactivity of OH groups in quercetin and taxifolin antioxidants: the specificity of the 3-OH site. Food Chem. 97, 679–688. doi: 10.1016/j.foodchem.2005.05.042
Trouillas, P., Marsal, P., Svobodová, A., Vostálová, J., Gazák, R., Hrbác, J., et al. (2008). Mechanism of the antioxidant action of silybin and 2,3-dehydrosilybin flavonolignans: a joint experimental and theoretical study. J. Phys. Chem. A. 112, 1054–1063. doi: 10.1021/jp075814h
Vacek, J., Zatloukalová, M., Desmier, T., Nezhodová, V., Hrbáč, J., Kubala, M., et al. (2013). Antioxidant, metal-binding and DNA-damaging properties of flavonolignans: a joint experimental and computational highlight based on 7-O-galloylsilybin. Chem. Biol. Interact. 205, 173–180. doi: 10.1016/j.cbi.2013.07.006
Wang, J., Zhao, L., Sun, G., Liang, Y., Wu, F., Chen, Z., et al. (2011). A comparison of acidic and enzymatic hydrolysis of rutin. J. Biotechnol. 10, 1460–1466. doi: 10.5897/AJB10.2077
Keywords: sodium pump, Na+/K+-ATPase, flavonolignans, inhibition, binding sites
Citation: Kubala M, Čechová P, Geletičová J, Biler M, Štenclová T, Trouillas P and Biedermann D (2016) Flavonolignans As a Novel Class of Sodium Pump Inhibitors. Front. Physiol. 7:115. doi: 10.3389/fphys.2016.00115
Received: 22 January 2016; Accepted: 14 March 2016;
Published: 30 March 2016.
Edited by:
Olga Vagin, University California, Los Angeles, USAReviewed by:
Pablo Martín-Vasallo, Universidad de La Laguna, SpainJoseph Isaac Shapiro, Marshall University, USA
Copyright © 2016 Kubala, Čechová, Geletičová, Biler, Štenclová, Trouillas and Biedermann. This is an open-access article distributed under the terms of the Creative Commons Attribution License (CC BY). The use, distribution or reproduction in other forums is permitted, provided the original author(s) or licensor are credited and that the original publication in this journal is cited, in accordance with accepted academic practice. No use, distribution or reproduction is permitted which does not comply with these terms.
*Correspondence: Martin Kubala, bWFydGluLmt1YmFsYUB1cG9sLmN6