- The Second Affiliated Hospital and Yuying Children's Hospital of Wenzhou Medical University, Wenzhou, China
Adult Leydig cells (ALCs) are the steroidogenic cells in the testes that produce testosterone. ALCs develop postnatally from a pool of stem cells, referred to as stem Leydig cells (SLCs). SLCs are spindle-shaped cells that lack steroidogenic cell markers, including luteinizing hormone (LH) receptor and 3β-hydroxysteroid dehydrogenase. The commitment of SLCs into the progenitor Leydig cells (PLCs), the first stage in the lineage, requires growth factors, including Dessert Hedgehog (DHH) and platelet-derived growth factor-AA. PLCs are still spindle-shaped, but become steroidogenic and produce mainly androsterone. The next transition in the lineage is from PLC to the immature Leydig cell (ILC). This transition requires LH, DHH, and androgen. ILCs are ovoid cells that are competent for producing a different form of androgen, androstanediol. The final stage in the developmental lineage is ALC. The transition to ALC involves the reduced expression of 5α-reductase 1, a step that is necessary to make the cells to produce testosterone as the final product. The transitions along the Leydig cell lineage are associated with the progressive down-regulation of the proliferative activity, and the up-regulation of steroidogenic capacity, with each step requiring unique regulatory signaling.
Introduction
Adult Leydig cells (ALCs) are located in the interstitial compartment of the testis. The cells synthesize testosterone that is essential for the physiological functions of the male reproductive system. The pubertal development of ALCs is required for the initiation and maintenance of spermatogenesis as well as for the promotion of male secondary sexual characteristics. ALC originates from an undifferentiated stem cell, called stem Leydig cell (SLC). In rodents, two independent Leydig cell (LC) populations develop sequentially in the testis, one during the fetal period and the other during the puberty (Chen et al., 2017).
In mice and rats, the first generation, fetal Leydig cells (FLCs), develop between the testis cords on gestational days 11–12 (Figure 1A; Huhtaniemi and Pelliniemi, 1992; Yao et al., 2002). FLCs are differentiated from the WT1+ somatic progenitor pools in the gonadal primordium, which may also serve as SLCs for the adult Leydig cells (Liu et al., 2016). Once developed, FLCs become the terminally differentiated cells. They have the capacity to produce androstenedione and insulin-like factor 3 (INSL3) just prior to birth (Habert and Brignaschi, 1991; Zimmermann et al., 1999; Adham et al., 2000; O'Shaughnessy et al., 2000; Shima et al., 2013). Because the Sertoli cells contain 17β-hydroxysteroid dehydrogenase 3, the androstenedione produced by FLCs is further converted into testosterone (Shima et al., 2013). The androgen produced by FLCs is crucial for the development of male reproductive tract and the descent of the testis (Huhtaniemi and Pelliniemi, 1992). INSL3 is a critical factor for initiating the testis descent process (Adham et al., 2000).
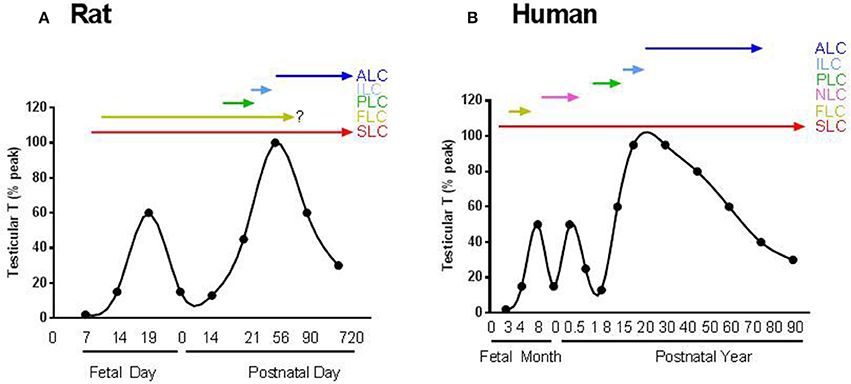
Figure 1. The scheme for the androgen production and possible involvement of Leydig cells in the life span. (A) Rat; (B) Human. SLC, FLC, PLC, ILC, ALC, and NLC represent stem, fetal, progenitor, immature, adult and neonatal Leydig cells, respectively. There are two androgen peaks for rats and three androgen peaks for human.
Rodent FLCs develop independently from luteinizing hormone (LH) since the cells are well developed even in the absence of LH or their receptors (Lei et al., 2001; Zhang et al., 2001; Ma et al., 2004). Rodent FLCs remain in the testicular interstitium after birth but may involute gradually, with only a few persisting in the adult testis (Kerr and Knell, 1988). There is still controversy about whether FLCs ultimately die, dedifferentiate or survive in adult testis (Huhtaniemi and Pelliniemi, 1992; Wen et al., 2016). A recent study showed that FLCs indeed persisted in adult mouse testis (Shima et al., 2015). Even if they were survived through the adulthood, the contribution to androgen production by these FLC could be minimal because the relative number is low compared to adult population (Kerr and Knell, 1988).
In rodents, the first stage in ALC lineage that have committed from SLC is the progenitor Leydig cell (PLC) (Shan and Hardy, 1992). PLCs are first evident in rat testis on neonatal day 11 by their expressions of steroidogenic enzymes, including cytochrome P450 cholesterol side chain cleavage enzyme (CYP11A1), 17α-hydroxylase/20-lyase (CYP17A1), 3β-hydroxysteroid dehydrogenase (HSD3B) and LH receptor (LHCGR) (Figure 1A; Ariyaratne et al., 2000). By postnatal day 21, PLC reached the maximal level in the testis. By postnatal day 35, PLCs are completely converted into ILCs, and by day 56, most of ILCs become ALCs (Chen et al., 2009).
In the primate and human testes, there is another generation of LCs (Figure 1B), which develop and involute for a very short period of time during the neonatal stage (Nistal et al., 1986; Prince, 1990, 1992, 2001). The origin of this generation of neonatal LCs in human and other primates is still unclear. Unlike rodents, androgen production by FLCs in primates and human are highly relied on the placenta-secreted chorionic gonadotropin (Nistal et al., 1986). When chorionic gonadotropin level decreases in the second trimester during human pregnancy, FLC number reduces sharply (Codesal et al., 1990). In human, due to the transient activation of the hypothalamic-pituitary-testicular axis during the first 6 months after birth, the second generation of LCs develops which increases androgen production significantly and thereafter the cells either involute or dedifferentiate because the hypothalamic-pituitary-testicular axis becomes inactive again (Prince, 1990). This quiescence in LC activity and androgen production lasts for years until the beginning of the development of the third generation of LC, ALC. Although the exact physiological functions of this brief testosterone surge by neonatal LCs in humans are not clear, it is hypothesized that it might be necessary for imprinting various cell types in androgen dependent organs, including the brain, to insure their proper responses to androgen by the adult (Svechnikov et al., 2010). For example, it was found recently that in adult female, there is a central pattern generator (CPG) in brain that specifically controls parturition and the milk-ejection reflex. This CPG is active in both male and female neonates, but is inactivated in males after the first week of life by the neonatal testosterone pulse. This suggests that the neonatal LCs are important for gender-specific perinatal programming (Israel et al., 2014).
The fetal and adult populations of LCs in rodents have distinct morphological and functional differences: For example, FLCs have abundant lipid droplets and are encased by several layers of collagen-enriched membrane in clusters (Huhtaniemi and Pelliniemi, 1992) and they develop independent of LH (Zhang et al., 2001), while ALCs have few lipid droplets and do not form clusters and they becomes desensitized when they are exposed to high concentration of LH due to the presence of an inhibitory guanine nucleotide-binding protein, which does not exist in FLCs (Warren et al., 1984; Eskola et al., 1994). However, a recent lineage tracing study in mouse shows that both FLCs and ALCs develop from a common Wilms tumor protein 1 (Wt1) positive precursor cells (Liu et al., 2016). In this review, we will mainly focus on the development of ALC population.
Adult Leydig Cell Ontogeny
Our understanding of the development of ALC was mostly coming from studies of rodents (rat and mice). Therefore, in this review, we will focus on the results from these two species, with occasional mentions of other species if necessary. In rats and mice, the ALC lineage can be divided into four distinct stages: stem, progenitor, immature, and adult (Figure 1 and Table 1).
Stem Leydig Cells (SLCs)
Until recently, a fundamental unanswered question about ALC development is whether these cells ultimately come from a single source of undifferentiated self-renewing SLCs. SLC should be defined to have the following three characteristics: (1) the capacity to self-renew for an extend period of time; (2) the potential to commit a lineage and expand the progenitor pool and differentiate to give rise the large number of progenies; and (3) the ability to move back to niche when transplanted back to the home organ (homing). SLCs are expected to be present in the testis in small numbers at adulthood and to be maintained by self-renewing. Furthermore, one progeny after an SLC division would be expected to undergo commitment into the LC lineage. The committed SLC, or PLC, amplifies its number through rapid cell divisions so as to create a pool of cells that are necessary to establish a right size of ALC population. It has been noted for some time that the postnatal rat testis contains spindle-shaped cells with few mitochondria or smooth endoplasmic reticulum that surround the seminiferous tubules and/or testicular blood vessels (Hardy et al., 1989). Cell dynamic analysis of the various populations in the interstitial compartment during the pubertal period suggested strongly that this spindle-shaped mesenchymal-like population may be the precursor of ALC (Hardy et al., 1989).
We isolated these cells by Percoll density-gradient centrifugation followed by immune-selections (Ge et al., 2006). Based on the 3 characteristics listed above, it was confirmed that these cells might be indeed the long-searched SLC. First, they express some stem cell markers, including Nestin and platelet-derived growth factor α receptor (PDGFRA) but do not express the LC lineage biomarkers, such as LHCGR, CYP11A1, HSD3B1 or CYP17A1 (Table 1; Ge et al., 2006). The cells can also proliferate, differentiate into the cells that can produce testosterone, and are capable of cloning (homing) in the testicular niche if they are transplanted back to the testis (Ge et al., 2006).
Various efforts were tried to find a specific protein marker to identify these cells from the rest of testicular cells (Chen et al., 2017). PDGFAA can specifically bind to PDGFRA to exert its signaling (Mariani et al., 2002). In the testis of mice that lack of PDGFAA no ALCs are developed, suggesting that PDGFRA signaling is critical for SLC function (Gnessi et al., 2000). The SLCs also express some levels of platelet-derived growth factor β receptor (PDGFRB), which is responsive to platelet-derived growth factor-BB, and the expression of this receptor disappears when SLCs enter the LC lineage (Stanley et al., 2012; Odeh et al., 2014).
In addition to PDGFRA, Nestin is another protein that may be served as the marker. nestin-positive SLCs contribute to the LC regeneration in the LC-depleted testis (Davidoff et al., 2004) that were induced by ethane dimethane sulfonate, a drug that specifically kills LCs (Teerds et al., 1988, 1989). In mouse testis, the nestin-positive SLCs can also proliferate, differentiate into the LC lineage in vitro and clone in the interstitial niche if they are transplanted back to the testis (Table 1; Jiang et al., 2014). Interestingly, nestin-positive SLCs also express CD51, a biomarker for the mesenchymal stem cells (Rux et al., 2016). Like nestin-positive cells, CD51-positive cells are also able to self-renew and differentiate into the multiple mesenchymal cell lineages and ALCs in vitro, as well as to clone back in the testicular niche (Table 1; Zang et al., 2017). This suggests that CD51-positive SLCs are multipotent. In addition to CD51, another biomarker for the mesenchymal stem cells (Paulini et al., 2016), CD90, may also be used to isolate the peritubular-associated SLC from the adult rat testis (Li et al., 2016). Interestingly, the CD90-positive cells can proliferate and differentiate into LC lineage in vitro in the absence of LH. The fact that these cells can be induced to differentiate into Leydig cells with Desert hedgehog (DHH), in the absence of other factors, including LH, suggests strongly that DHH may be the important SLC commitment factor that is necessary for the differentiation of SLC into Leydig lineage (Li et al., 2016).
Another biomarker of SLCs could be chicken ovalbumin upstream promoter transcription factor II (NR2F2 or COUP-TFII). Using lineage tracing analysis, it is found that NR2F2-positive cells can differentiate into ALCs (Table 1; Kilcoyne et al., 2014). Conditional knockout of NR2F2 during the pre-pubertal period prevented the formation of ALC population (Qin et al., 2008), suggesting that NR2F2-positive cells are critical seed cells for LC development. SLCs, judged by the expression of NR2F2, are present in the interstitium during the whole lifespan (Figure 1) and these cells are abundant during the neonatal and pre-pubertal periods (Kilcoyne et al., 2014).
Progenitor Leydig Cells (PLCs)
In rat testis, PLC, the earliest identifiable cell stage in the differentiated LC lineage, first appears on postnatal day 11 (Ariyaratne et al., 2000). PLC is a small spindle-shaped cell that is morphologically similar to the undifferentiated SLC from which it is derived but contains LC markers, such as the steroidogenic enzymes CYP11A1, HSD3B1, and CYP17A1 (Shan et al., 1993). On postnatal day 12, PLCs also begin to express a truncated LHCGR (Figure 1A; Ge and Hardy, 2007).
PLCs may be called as amplifying cells because they have a high proliferative capacity and they express very higher levels of cyclin A2, a somatic cell cycle protein (Ge and Hardy, 1997). Additional cell cycle regulatory proteins, including cyclin-dependent kinase 2, cyclin-dependent kinase 25, cyclin B, cyclin C, cyclin D, and cyclin E are also abundant in PLCs (Ge et al., 2005; Stanley et al., 2011). PLCs retain the stem cell markers, PDGFRA, leukemia inhibitory factor receptor, and c-Kit (Ge et al., 2005; Stanley et al., 2011). Although CYP11A1, HSD3B, and CYP17A1 all appear in PLCs of wild-type mice, PLCs in the LHCGR knockout mouse is only positive for HSD3B but negative for both CYP11A1 and CYP17A1 (Zhang et al., 2004), suggesting that HSD3B may appear earlier than other steroidogenic proteins and therefore can be used as a better biomarker for the cells during the transition from SLCs into PLCs.
PLCs do not express 17β-hydroxysteroid dehydrogenase 3 (HSD17B3), the critical enzyme to catalyze the formation of testosterone in the last step of steroidogenic pathway (Ge and Hardy, 1998). However, PLCs express high levels of androgen-metabolizing enzymes, 5α-reductase 1 (SRD5A1) and 3α-hydroxysteroid dehydrogenase (AKR1C9) (Ge and Hardy, 1998; Viger et al., 2005). Although PLCs have some potential to make androgens, they cannot make testosterone because of lacking HSD17B3 (Ge and Hardy, 1998). Thus, the androstenedione, formed after the sequential catalysis by three enzymes (CYP11A1, HSD3B, and CYP17A1) is metabolized into androstanedione by SRD5A1 and further into androsterone by AKR1C9, which is secreted as the end product of the cells (Figure 2; Ge and Hardy, 1998).
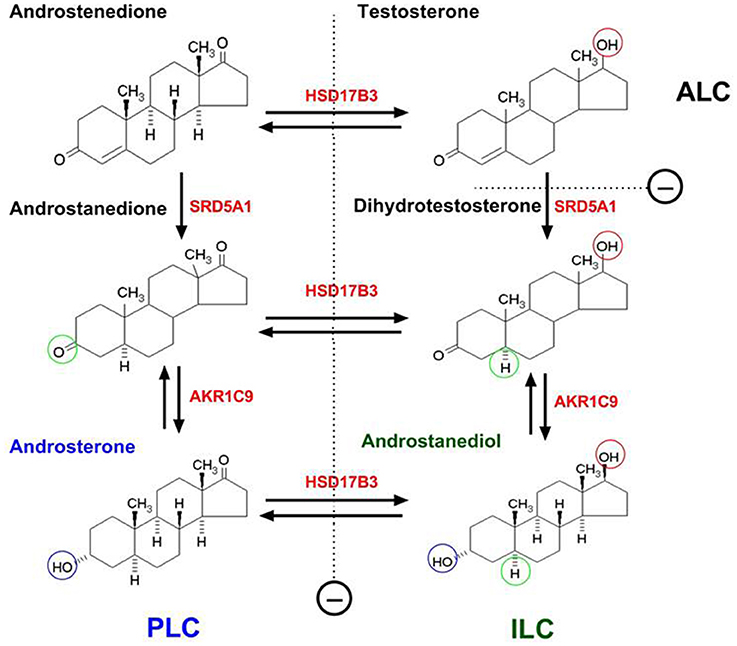
Figure 2. The difference of progenitor, immature and adult Leydig cells in the products of androgen in rats due to their differential expressions of steroidogenic enzymes. PLC, ILC, and ALC represent progenitor, immature, and adult Leydig cells, respectively. PLC lacks of 17β-hydroxysteroid dehydrogenase 3 (HSD17B3) but contains higher levels of 5α-reductase 1 (SRD5A1) and 3α-hydroxysteroid dehydrogenase (AKR1C9), thus producing primarily androsterone. ILC begins to express HSD17B3 and also contains SRD5A1 and AKR1C9, thus producing predominantly androstanediol. ALC secretes mainly testosterone due to the silence of SRD5A1. SRD5A1 is a unidirectional enzyme. Other steroidogenic enzymes are bidirectional.
As they develop, PLCs enlarge the size and become ovoid-shaped (Benton et al., 1995). Their mitotic capacities are reduced when they acquire some of the differentiated functions of mature cells in the LC lineage (Ge and Hardy, 1997, 1998). Mouse PLCs are identified in the testis on postnatal day 10 (Baker et al., 1996; Hu et al., 2010b). Mouse PLCs also have high proliferative capacities to expand the cell pool in order to establish a right size of ALC eventually (Baker et al., 1996; Hu et al., 2010b).
Immature Leydig Cells (ILCs)
The second transition in the lineage is from PLCs into ILCs. ILC is most commonly seen in rat testis from days 28 to 35 postpartum (Figure 1; Hardy et al., 1989). The cell is ovoid-shaped and appears to have more advanced smooth endoplasmic reticulum than PLC (Shan et al., 1993). In the rat, ILCs contain numerous lipid droplets (Shan and Hardy, 1992). The activities of CYP11A1, HSD3B1, and CYP17A1 increase in ILCs as they matures from PLCs (Ge and Hardy, 1998). ILCs begin to express HSD17B3 and therefore they can make testosterone from androstenedione (Ge and Hardy, 1998). However, ILCs still have higher levels of SRD5A1 (Viger and Robaire, 1995) and AKR1C9 (Shan et al., 1993), thus the formed testosterone by HSD17B3 is quickly metabolized into dihydrotestosterone by SRD5A1 and further into androstanediol by AKR1C9. The latter is secreted into the circulation as the final product of the cells (Figure 2; Ge and Hardy, 1998).
At this stage, a retinol dehydrogenase 2, which has 3α-oxidative activity that can convert androstanediol back to dihydrotestosterone (Hardy et al., 2000), also appears in ILCs. This enzyme is absent in PLCs (Ge et al., 1999). Interestingly, ILCs also begin to express 11β-hydroxysteroid dehydrogenase 1 (HSD11B1) on postnatal day 28 (Phillips et al., 1989). HSD11B1 is an oxidoreductase that controls local levels of glucocorticoid (Agarwal et al., 1989). ILCs in mouse testis seems also to express SRD5A1 and AKR1C9 and produces androstanediol during puberty (Wang and Hardy, 2004). Although it is clear that different stages of developing LCs produce different forms of androgens, it is still unclear whether there is any physiological significance behind it.
Around postnatal day 35, the number of ILCs is about 50% of ALCs in the adult testis (Hardy et al., 1989). This suggests that ILCs, on average, divide at least once in order to establish a right size of ALC population in adulthood. Indeed, even with reduced levels of cell cycle regulating proteins, including cyclin A2, cyclin B, cyclin C, and cyclin E (Ge et al., 2005; Stanley et al., 2011), ILCs still have maintained some proliferative capacity (Ge and Hardy, 1997).
Adult Leydig Cells (ALCs)
ALCS in rat testis is formed on postnatal days 49–56 (Figure 1; Hardy et al., 1989). ALCs are irregularly round and are larger than ILCs (Shan et al., 1993). ALCs have well developed smooth endoplasmic reticulum and more mitochondria than ILCs and have increased expressions of CYP11A1, HSD3B, CYP17A1, and HSD17B3. At this stage, the SRD5A1 expression is silenced (Viger and Robaire, 1995), which leads to testosterone as the major product of the cells (Figure 2; Ge and Hardy, 1998). Testosterone is synthesized from cholesterol in ALCs in a multistep process catalyzed by four enzymes sequentially: CYP11A1, HSD3B, CYP17A1, and HSD17B3 (Payne and O'Shaughnessy, 1996). The transportation of cholesterol from the cytosol into the inner membrane needs steroidogenic acute regulatory protein (StAR) (Clark et al., 1995). The expression of this protein is activated by LH via cAMP signaling pathway (Stocco, 2001). In addition to steroidogenic enzymes that are directly responsible for testosterone production, HSD11B1 is also highly expressed by ALC (Ge et al., 1997b). This enzyme may behave primarily as a protecting protein to prevent the cells from the negative effects of excessive glucocorticoid exposure. HSD11B1 is a bi-directional enzyme that can convert glucocorticoid between the active/inactive states. The directions are regulated by another enzyme, hexose-6-phosphate dehydrogenase. Since this regulatory enzyme is very low in ALCs (Ge et al., 1997a; Li et al., 2015) HSD11B1 mainly functions as an oxidase to metabolize glucocorticoid into an inactive form. Also, in rat ALCs, a CYP2A1 begins to express and it can catalyze testosterone into 7α-hydroxytestosterone, which also involves in the regulation of HSD11B1 activities (Hu et al., 2010a). In the rat testis, ALCs rarely, if ever, divide; the ALC number is maintained relative stable at about 25 million cells per testis (Hardy et al., 1989).
Regulation of Leydig Cell Development
The proliferation and differentiation of cells in the LC lineage are regulated by multiple growth factors and hormones (Figure 3 and Table 2). These factors act via various signaling pathway, positively or negatively controlling the progression of each of developmental LC stages.
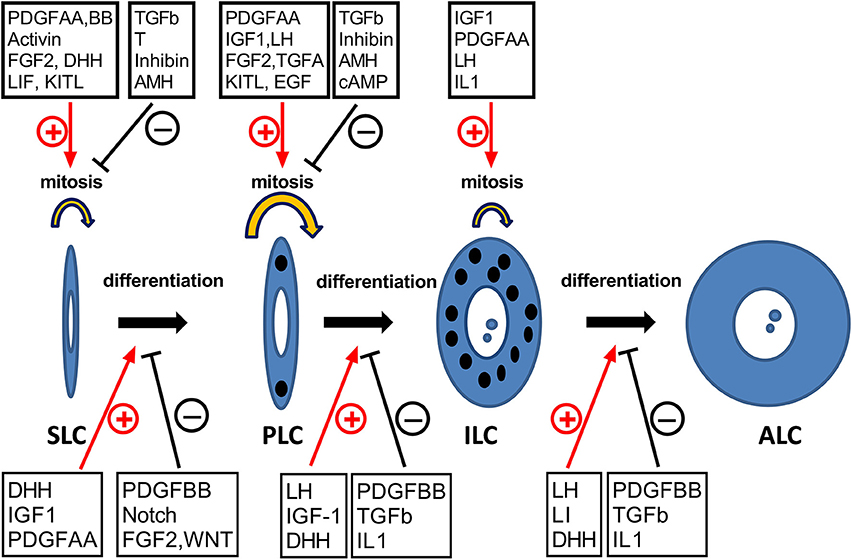
Figure 3. The hormones and growth factors that may potentially regulate the development of Leydig cells. SLC, PLC, ILC, and ALC represent stem, progenitor, immature, and adult Leydig cells, respectively. ⊕, stimulation; θ, inhibition.
Transcription Factors
Several transcription factors are critical for LC development. One of these factors is steroidogenic factor 1 (SF1, also known as NR5A1 or AD4BP). NR5A1 is a common transcription factor for the somatic stem cells in the gonads (Sadovsky et al., 1995), the adrenals (Gut et al., 2005), and the pituitary (Zhao et al., 2001). Inactivation of NR5A1 causes gonadal agenesis and loss of all somatic cell lineages in the gonad, including LCs (Sadovsky et al., 1995; Zhao et al., 2001). The importance of NR5A1 in LC development is also evident by the observations that forced expression of this gene, by itself, can convert stem cells of other organs and fibroblasts into LC lineage by promoting the expression of LHCGR and other steroidogenic enzymes (Cyp11a1, Hsd3b, Cyp17a1, and Hsd17b3; Yang et al., 2017).
GATA4 is a transcription factor with a double zinc finger binding domain that recognizes the GATA motif in the promoters of the target genes (Tevosian, 2014). GATA4 is expressed in SLCs and is a key positive transcriptional regulator of genes involved in steroid biosynthesis, including transcriptional factor NR5A1 (Hu et al., 2013). GATA4 is required for the formation of genital ridge in mice (Hu et al., 2013). One of the functions of GATA4 could be to induce NR5A1 expression.
Different from NR5A1 and GATA4, NR2F2 seems to play a very narrow and specific role in LC development. Without it, the maturation of PLC to ALC is inhibited. Knockout of NR2F2 on postnatal day 14 does not influence the formation of PLCs but significantly blocks the transition of PLCs into ILCs as judged by failed changes in cell morphology and by remarkable reductions in the expressions of steroidogenic enzymes such as Cyp11a1, Hsd3b, and Cyp17a1 (Qin et al., 2008).
NR4A1 (also known as Nur77, NGFI-B, or TR3) is another major nuclear receptor transcription factors involved in the regulation of LC development and steroidogenesis (Zhang and Mellon, 1997). NR4A1-binding regions are identified within the promoters of several LC steroidogenic-related genes, including rat Cyp17a1 (Zhang and Mellon, 1997), mouse Star (Martin et al., 2008), and human HSD3B (Martin and Tremblay, 2005). Interestingly, NR4A1 is regulated by LH, which may mediate the roles of LH in the development of LCs (Song et al., 2001).
Leukemia Inhibitor Factor (LIF)
In addition to transcriptional factors, multiple external factors are identified that may regulate SLC commitment and progression. One such factor is LIF, a member of the interleukin-6 cytokine family (You et al., 2017), which includes interleukin 11, interleukin 27, and ciliary neurotrophic factor. These factors bind to homo- or heteromeric receptors, of which they share a common gp130 subunit (Cheng et al., 1994; Moreno Frias et al., 2004). LIF receptor contains two subunits, gp130 and gp190 (Cheng et al., 1994; Moreno Frias et al., 2004). When LIF binds to gp190, the bound receptor causes the heterodimerization of gp130 and gp190 subunits, which subsequently induces a rapid activation of janus kinases, resulting in the phosphorylation of tyrosine residues of gp190 and gp130 (Golshan et al., 2014). These phosphorylated tyrosine residues activate signal transducer and activator of their target transcription families (Heinrich et al., 1998; Moser et al., 2001; Xi et al., 2012).
The expression level of LIF in rat fetal testis is low which is significantly increased during puberty and reaches the highest level around postnatal day 45 (Piquet-Pellorce et al., 2000). LIF is required for the self-renewal of embryonic stem cells (Wang et al., 2017), indicating that it may also be critical for the self-renewal of SLCs. In rat testis, LIF is first detected during gestation period. It is expressed predominantly by the peritubular cells surrounding the seminiferous tubules (Piquet-Pellorce et al., 2000), indicating that these LIF expressing cells may form a niche for SLCs since SLCs were also reported to reside on the surface of seminiferous tubules (Zhang et al., 2013; Odeh et al., 2014; Li et al., 2016). Indeed, LIF is capable of stimulating the proliferation of SLCs in vitro (Ge et al., 2006).
Desert Hedgehog (DHH)
The Hedgehog signaling pathway is involved in a number of developmental processes during embryogenesis (see review, Varjosalo and Taipale, 2008). Hedgehog factors have three members: DHH, Indian Hedgehog, and Sonic Hedgehog. These Hedgehogs induce a common signal transduction pathway via binding to a receptor complex consisting of patched receptors (Ptch1 and Ptch2) and Smoothened on the cell membrane (Yao et al., 2002; Park et al., 2007). This signaling pathway involves three transcription factors Gli1, Gli2, and Gli3 (Ingham and Mcmahon, 2001). Gli1 acts as a transcriptional activator while Gli2 and Gli3 are needed to be processed by proteasomes, thus repressing target gene transcription (Varjosalo and Taipale, 2008). Patched inhibits Smoothened activity by preventing its accumulation within cilia (Rohatgi et al., 2007). Binding of Hedgehog to Patch releases its inhibitory effect on Smoothened, allowing Smoothened to induce the downstream signaling related with Gli proteins (see review Varjosalo and Taipale, 2008).
In the testis, DHH is secreted by Sertoli cells after the activation of Sex-determining Region of Y-chromosome (SRY) and the expression persists into the adult (Bitgood et al., 1996). Ptch1 is present in the LC lineage (Clark et al., 2000). DHH-Ptch1 signaling seems to be critical for LC development. Rat testis with a null mutation of DHH gene (Dhh) lacks ALCs (Kawai et al., 2010). DHH may have similar functions for human SLC development because a patient with a homozygous mutation in DHH gene shows partial gonadal dysgenesis with the loss of ALCs (Werner et al., 2015). The development of ALCs is also defective in Dhh knockout male mice, and the mice are sterile (Clark et al., 2000). Loss of Dhh leads to a reduced number of FLCs, indicating that both FLCs and ALCs require DHH regulation (Yao et al., 2002). In Dhh knockout mice, the migration of fetal LC precursors from the mesonephros and their proliferation and survival are not affected, suggesting that DHH mainly affects the differentiation of fetal LC precursors to LC lineage (Yao et al., 2002).
Using rat seminiferous tubule culture system, we demonstrate that DHH is required for both proliferation and differentiation of SLCs into ALC lineage (Li et al., 2016). DHH alone is sufficient to induce the differentiation of CD90-positive SLCs isolated from the seminiferous tubules into LC lineage (Li et al., 2016). Although the mechanism by which DHH induces LC differentiation is still unknown, NR5A1 seems to be involved since DHH is able to stimulate the expressions of this transcriptional factor in Leydig cells (Yao et al., 2002; Barsoum et al., 2013). Ectopic activation of the Hedgehog signaling in the ovary also stimulated NR5A1 expression (Barsoum et al., 2013), which induced the formation of LC-like cells in the ovary, where LCs normally are absent. It is possible that DHH not only affects Leydig cell development, but also may play a role in the maintenance of steroidogenic function of ALC. Conditional knockout of Wt1 gene in Sertoli cells in 2 month-old mice resulted in loss of ALC functions, as shown by a severe reduction in testosterone production and significant downregulations of steroidogenic enzymes and LHCGR, all possibly because of loss of Dhh expression, since the defects in steroidogenesis can be partially rescued by a hedgehog pathway agonist (Chen et al., 2014).
Platelet-Derived Growth Factors
Platelet-derived growth factors (PDGF) are a family of proteins that have been shown to exert mitogenic effects on undifferentiated mesenchyme and progenitor cell populations (Brennan et al., 2003). PDGF consist of several subunits as the homo- or heteromeric complex. For example, PDGF-A and PDGF-B subunits can form AA, AB, and BB dimers, which can bind to their respective receptors.
PDGF receptor α (PDGFRA) is expressed in the LC lineage cells, including the peritubularly located SLCs (Gnessi et al., 1995). The major ligand of PDGFRA is PDGF-AA (Mariani et al., 2002). It has been shown that ALCs do not differentiate in the Pdgfa knockout mice, suggesting that PDGF-AA signaling is critical for the SLC function (Gnessi et al., 2000). Interestingly, SLCs also express some levels of platelet-derived growth factor receptor β (PDGFRB) (Gnessi et al., 1995), whose nature ligand is PDGF-BB (Stanley et al., 2012). The expression of PDGFRB is silenced when SLCs enter the LC lineage in rats (Stanley et al., 2011; Odeh et al., 2014).
Two PDGF receptors-mediated signaling has similar and dissimilar actions in SLCs (Odeh et al., 2014; Li et al., 2016). Both significantly stimulate the proliferation of SLCs when they bind to their respective receptors (Li et al., 2016). PDGFRA signaling stimulates the differentiation of SLCs into the LC lineage when it binds to PDGF-AA (Odeh et al., 2014), while PDGFRB signaling inhibits the differentiation of SLCs into the LC lineage when it binds to PDGF-BB (Odeh et al., 2014). After the treatment of PDGF-BB, peritubular myoid cells are differentiated, indicating that SLCs are multipotent, being capable of committing into either the LC lineage or the myoid cell lineage, depending on the stimulating PDGF ligands (Odeh et al., 2014; unpublished observation).
The expression of PDGFRA is regulated by the transcription factors SP1 and SP3 (Bergeron et al., 2011). The downstream signaling of PDGFRA in regulating the commitment of SLCs into the LC lineage is still unclear. Two PDGF target genes (Sgpl1 and Plekha1) have a significant role in the development of ALCs, since knockouts of either Sgpl1 or Plekha1 genes caused a phenotype similar to that of Pdgfa mutation, with a dramatic loss of ALC population (Schmahl et al., 2008). Sphingosine-1-phosphate lyase, encoded by Sgpl1, is an enzyme that irreversibly cleaves sphingosine-1-phosphate (Kihara et al., 2003), suggesting that sphingosine-1-phosphate may mediate the downstream signaling of PDGFRA in the LC development. Plekha1 encodes a pleckstrin homology domain-containing adapter protein, which is localized in the plasma membrane, where it specifically binds to phosphatidylinositol 3,4-bisphosphate and thus possibly involved in the formation of signaling complexes in the plasma membrane (Dixon et al., 2011). PDGFRA is also important for FLC development because Pdgfrα−/− male mice have reduced FLC numbers (Brennan et al., 2003).
Kit Ligand (KITL)
KIT ligand (also called stem cell factor) is a growth factor that can bind its receptor, KIT (c-kit) to activate its tyrosine kinase activity. Autophosphorylation of the receptor initiates signaling transduction cascades, including activation of PI3-kinase (PI3K) signaling by binding to a p85 subunit of PI3-kinase. In the testis, the KIT ligand is secreted by Sertoli cells. There are two forms of the ligand, KITL1 and KITL2, which are produced via alternatively spliced Kitl mRNAs. KITL1 is processed to produce soluble KIT ligand, and KITL2 is a membrane-bound factor (Minegishi et al., 2003). KIT is expressed by germ cells and SLCs (Rothschild et al., 2003). KIT ligand plays a critical role in the development of primordial germ cells. In mice with null mutation of either KIT or KIT ligand, primordial germ cells cannot proliferate and migrate, which leads to only a few primordial germ cells in the gonad (Augustowska et al., 2003a; Min et al., 2004). In mice with reduced KIT and KIT ligand, the postnatal spermatogenesis is blocked (Augustowska et al., 2003b). A mutation in KIT that lost its binding ability to PI3-kinase due to the substitution of tyrosine by phenylalanine, also caused severe deficiencies in spermatogenesis (Rothschild et al., 2003). These results indicate that KIT system is important to spermatogenesis.
In the newborn rat and mice testis, KIT is also expressed in the LC lineage, includingSLCs by postnatal day 5 (Manova et al., 1990; Sandlow et al., 1996). The role of KIT ligand in LC development is supported by both in vivo and in vitro studies. In KIT mutant mouse, LC has a reduced capacity of steroidogenesis (Rothschild et al., 2003). Using an in vitro culture system of seminiferous tubules from LC-depleted testis, it is found that KIT ligand can affect both proliferation and differentiation of the peritubularly located SLC. Interestingly, the two effects happened at different concentrations, with proliferation taking place at high concentrations (10 and 100 ng/ml) while differentiation taking place at low concentration (1 ng/ml) (Liu et al., 2017).
Insulin-Like Growth Factor 1 (IGF1)
Insulin-like growth factor 1 (IGF1), a factor with a similar structure to insulin, plays a very diversified effects cross our body. IGF1 is primarily secreted by the liver and is also produced locally by the target tissues. In the testis, IGF1 is secreted by peritubular cells, spermatocytes, and LCs (Handelsman et al., 1985; Lin et al., 1986a,b; Dombrowicz et al., 1992; Ha et al., 2016). The secretion of IGF1 in liver is regulated by growth factor. IGF1 initiates the intracellular signaling by binding its specific receptor and activating its tyrosine kinase activity (Yakar et al., 2002; Ha et al., 2016). IGF1 receptor is present in the LC lineage (Vannelli et al., 1988).
FLC seems not requiring IGF1 for its development since Igf1 gene knockout does not affect FLC population in mice (Baker et al., 1996). However, the deletion of Igf1 gene results in a significant reduction in testosterone production of the adult testis due to a reduced LC numbers (Baker et al., 1996). IGF1 is capable of stimulating the proliferation of PLCs and ILCs, and knockout Igf1 completely abolishes their proliferations in vivo (Hu et al., 2010b). The replacement of IGF-1 to these null mice restored the LC population by increasing the proliferations of PLCs and ILCs (Hu et al., 2010b). Interestingly, IGF1 may not affect the proliferation of SLCs (Hu et al., 2010b).
In addition to cell proliferation, IGF1 also stimulates LC differentiation. Deletion of Igf1 significantly delayed the maturation of ILCs to ALCs since the cells in the mutant adult testis still had characteristics of ILCs, with significantly decreased expression of CYP11A1, HSD3B, CYP17A1, and HSD17B3 and increased expression of SRD5A1 (Wang et al., 2003). LH can stimulate IGF1 secretion and upregulate IGF1 receptor expression in LCs (Lin et al., 1988; Cailleau et al., 1990; Nagpal et al., 1991) so it is possible that IGF1 may partially mediate the effect of LH in Leydig cell development (Khan et al., 1992b; Ge and Hardy, 1997). Replacement of IGF1 can also increase testosterone production in growth hormone deficient Snell dwarf mice, which have low circulating IGF1 levels (Dombrowicz et al., 1992).
Transforming Growth Factor β1 (TGFb)
Transforming growth factor β1 (TGFb1) is a member of the TGFb superfamily. It exerts its signaling via binding to TGFb type II receptor and TGFb type I receptor (also termed activin receptor-like kinase-5, ALK5), both of which are serine/threonine kinase receptors (Goumans et al., 2002). Binding of TGFb to type II receptor induces the formation of heteromeric complexes with ALK5, within which type II receptor phosphorylates ALK5, turning on receptor kinase activity. Activation of ALK5 induces Smad2 and/or Smad3 phosphorylation at C-terminal serines, and the activated Smad2 and/or Smad3 forms a heterotrimeric complex with Smad4, which translocates to the nucleus for transcription regulation (Goumans et al., 2002).
Among the superfamily, TGFb is the most prominent type in the testis (Skinner and Moses, 1989). On postnatal day 21, about 50% of the PLCs express TGFb while ILCs from postnatal day 35 have undetectable level of expression (Teerds and Dorrington, 1993). TGFb inhibits both the proliferation and differentiation of rat SLCs on the surface of seminiferous tubules (Li et al., 2016). However, its effects on PLC proliferation seems to depend on the presence of other factors. For example, TGFb causes a small but significant increase in PLC proliferation when used by itself but inhibits the proliferations induced by transforming growth factor α or IGF1 (Khan et al., 1992b) when used with these factors together. TGFb also potently inhibits the steroidogenesis in PLCs (Khan et al., 1999). It represses LH-stimulated testosterone production in LCs through decreasing LHCGR and steroidogenesis-related genes such as Star and Cyp17a1 (Le Roy et al., 1999). One of the possible mechanisms by which TGFb affect Leydig cells is through modifying nuclear factor NR4A1 activity. It was found that TGFb/ALK5/SMAD3 signaling can repressed the ability of NR4A1 transcription factor to bind the promoters of the target genes (Park et al., 2014).
Anti-Müllerian Hormone (AMH)
Anti-Müllerian hormone (AMH, also called Müllerian inhibiting substance) is another member of the TGFb superfamily. AMH is a hormone that inhibits the development of the Müllerian ducts in the male embryo to insure the development of the male reproductive system. Its productions are high in the fetal and neonatal testes (mainly by immature Sertoli cells) and the production declines by the puberty (Vigier et al., 1989; Racine et al., 1998). The AMH receptor type II (AMHR2) is a serine/threonine kinase receptor that is also expressed in the LC lineage (Racine et al., 1998). Overexpression of human AMH in mice reduced LC numbers in the testis (Lyet et al., 1995). In contrast, AMH and AMHR2 deficiency in mice increased LC numbers (Lyet et al., 1995; Racine et al., 1998) and AMH/AMHR2 double knockout in mice have caused LC hyperplasia (Mishina et al., 1996). Treatments of AMH in vitro and in vivo significantly inhibited LC steroidogenesis by downregulating Lhcgr and Cyp17a1 expressions (Sriraman et al., 2001). AMH also inhibits the regeneration of LCs in ethane dimethane sulfonate-treated rats by inhibiting PLC proliferation and promoting LC apoptosis (Salva et al., 2004). Based on the studies of a transgenic mice model, it was found that AMH inhibited PLC proliferation by activating ALK3 (Wu et al., 2012).
Activin and Inhibin
Activin is also a member of the TGFb superfamily (Sharpe, 2006). Activin is a dimeric ligand, containing either homodimer or heterodimer of the 3 inhibin b subunits βA (encoded by Inhba), βB (encoded by Inhbb), or βC (encoded by Inhbc). Activin A (two βA subunits) acts via a heteromeric complex of receptor serine kinases, which include at least two type I (I and IB) and two type II (II and IIB) receptors (Sharpe, 2006). These receptors are transmembrane proteins, which contain an extracellular domain with the cysteine-rich region for the ligand-binding, a transmembrane domain, and a cytoplasmic domain with serine/threonine specificity for signaling. Type I receptors, including activin A receptor type I, are essential for signaling. Type II receptors are required for binding to activin A and for the expression of type I receptors. Type I and II receptors form a stable complex upon activin A binding, leading to phosphorylation of type I receptors by type II receptors (Sharpe, 2006). Activin A has been shown to be an important growth factor in the testis to regulate its functions (Hutson and Hasthorpe, 2005; Paul et al., 2005; Rey et al., 2005; Mendis-Handagama et al., 2006). As the product of FLCs, activin A regulates Sertoli cell mitosis and the testis cord expansion (Paul et al., 2005). Rat neonatal testis contains high number of SLCs (Chen et al., 2009) and a higher level of activin A is observed during this period (Kai et al., 2005). With the ALC development, activin A protein levels decrease while two activin signaling inhibitors, follistatin and BMP, and activin membrane-bound inhibitor increases (Borch et al., 2005). Follistatin increases in the mouse testis after postnatal day 4 (Trbovich et al., 2004). Activin A increases rat SLC and PLC proliferations. However, activin A may inhibit SLC differentiation to maintain their stemness (Li et al., 2017). Activin also inhibits ALC steroidogenesis (Lin et al., 1989).
Inhibin is another member of TGFb superfamily. The first component of the dimer ligand is a β subunit identical to the β subunit in activin while the second component is a more distantly-related α subunit (Burger and Igarashi, 1988). Inhibin is secreted by the Sertoli cells (Skinner et al., 1989). Interestingly, androgens can stimulate inhibin productions, which implies thatLCs can affect the development of themselves via inhibin (Meachem et al., 2001) since it was showed that inhibin can inhibit SLC proliferation (Li et al., 2017). Much less is known about the mechanism by which inhibin works. It may act via competing with activin for binding to activin receptors and/or binding to inhibin-specific receptors (Robertson et al., 1992).
Fibroblast Growth Factor 2
Fibroblast growth factor 2 (FGF2) belongs to a heparin-binding growth factor family. It can affect multiple cell functions, including proliferation, migration, survival, and differentiation. The factor can affect diverse cell types and tissues (Eswarakumar et al., 2005). FGF2 is also identified in testis (Ueno et al., 1987). It is expressed in the LC lineage, and its level decreases with the progression of PLCs into ALCs (Ge et al., 2005). The involvement of FGF2 in the development and function of LCs could be anticipated, because of the mesenchymal origin of this lineage. FGF2 dramatically stimulates SLC proliferation while it inhibits its differentiation (Li et al., 2016). FGF2 stimulates the proliferation of SLCs in ethane dimethane sulfonate-treated LC regeneration model (Liu et al., 2014). FGF2 can downregulate NR5A1 expression which could be one of the machanisms that FGF2 inhibits the PLC differentiation. Although FGF2 can stimulate ILC steroidogenesis in the absence of LH, FGF2 has a biphasic effect on LH binding to its receptors (LHCGR) in ILCs, with low concentrations (0.1–1.0 ng/ml) inhibitory and high concentrations (10–100 ng/ml) stimulatory (Murono et al., 1993). The late may be mediated by FGF2 binding to a different receptor, heparan sulfate proteoglycans (Murono et al., 1993).
Luteinizing Hormone (LH)
Cells in Leydig cell lineage are the only cells that respond to LH in the testis. LH binds to the LHCGR in LCs, resulting in both acute- and trophic-effects. Acute-effects involve the mobilization and delivery of cholesterol to mitochondran to start the steroidogenesis. The trophic effects involve, increases in gene transcriptions and steroidogenic enzyme activities. Both effects are required for the maintenance of an optimal steroidogenesis in the cells (Dombrowicz et al., 1996). Without LH stimulation, LC steroidogenic enzyme activities are reduced. Although LH stimulation is required for LC development, it seems unlikely that the horomone is required for the commitment of SLC into the LC lineage because PLCs can be formed in Lhcgr knockout mouse testis (Zhang et al., 2004). LH seems to not affect FLC differentiation because FLCs are well developed in the Lhcgr- and Lhb- knockout mice (Ma et al., 2004; Zhang et al., 2004). However, studies of LHb and GnRH knockout (GnRHhpg) mice, which are deficient in circulating LH, make it apparent that LH plays a critical role in the development of ALCs. Lhb-null mice have decreased testes size, with very few ALC in the interstitial compartment, and with reduced serum testosterone levels (Ma et al., 2004). In the GnRH knockout mice, LC number is about 10% of control (Baker and O'Shaughnessy, 2001). Moreover, LH/hCG administration to GnRH knockout mice in vivo increases LC proliferation (Dombrowicz et al., 1992). In addition to the ligand, knockout of Lhcgr also significantly reduced ALC numbers (Lei et al., 2001; Zhang et al., 2001). Overall, these results suggest that LH signaling is critical to both ALC differentiation and their precursor proliferation. However, the specific mechanism that is responsible for its stimulation on Leydig cell proliferation is not well studied, though activation of ERK1/2 cascade may be involved (Ge and Hardy, 1997; Shiraishi and Ascoli, 2007).
Androgen
Androgen receptor (NR3C4) is a nuclear receptor and is expressed in SLCs and the LC lineage and other testicular cells such as Sertoli cells and peritubular myoid cells (Bremner et al., 1994; Shan et al., 1995). The levels of NR3C4 are high in SLCs, PLCs and ILCs compared to ALCs (Shan and Hardy, 1992). The presence of NR3C4 in the LC lineage suggests that androgen produced by FLCs, adrenal glands or the LCs themselves might regulate the development and function of LCs. In NR3C4 mutated mice in which there is an androgen insensitivity, reduced differentiation of LCs and decreased LC numbers have been noted (O'Shaughnessy and Murphy, 1993; Murphy et al., 1994). Consistent with this, when PLCs are cultured in vitro, adding androgen greatly increased the efficiency of the cells to differentiate into androgen-producing cells (Hardy et al., 1990). All these evidences strongly suggest that androgen is essential for the development and maturation of ALC.
In mouse testis with global knockout of NR3C4, FLCs are normal while ALC number are reduced to 60% of control level by the adult, with very low expression of HSD17B3, suggesting that the differentiation of SLCs into ALCs is disrupted (O'Shaughnessy et al., 2002). Because NR3C4 is expressed by many testicular cell types, the result of global knockout, by itself, cannot tell which cell or cells are responsible for the ALC developmental defects. Recent studies, including cell-specific knockouts, demonstrated that NR3C4 in Sertoli cells (De Gendt et al., 2005; Hazra et al., 2013; Rebourcet et al., 2014a,b) and peritubular myoid cells (Welsh et al., 2009, 2012) are critical for ALC development. Interestingly, knockout of NR3C4 in Sertoli cells only reduced LC number but not the steroidogenic capacity (Chang et al., 2004). Sertoli cell specific NR3C4 deletion caused a significant downregulation of PDGF-AA, which, by reducing SLC/PLC proliferations, could be one of the mechanisms for the reduced ALC numbers (Chang et al., 2004).
To understand whether NR3C4 signaling in LCs also affect their own development, LC-specific knockout was attempted by using Amhr2-Cre (Xu et al., 2007). Though Amhr2-Cre was unable to produce a complete knockout in LCs and plus the gene is also disrupted in some Sertoli cells, the experiment indeed showed that the differentiation of LC is delayed (Xu et al., 2007). Fatty acid binding protein 4 (Fabp4)-Cre was also used to produce LC-specific knockout, which though only yielded disruptions in about 75% LCs, they were LC-specific. The results indicated that NR3C4 in LCs is indeed important for ALC maturation (O'Hara et al., 2014). mFLE-Cre was used to produce NR3C4 knockout in FLCs which, as expected, did not affect FLCs. Also, testosterone levels and fertility were normal in the adult mice, suggesting that NR3C4 signaling in FLCs is dispensable for male reproductive functions in the late life (Shima et al., 2015). However, in another study that NR3C4 is disrupted in the fetal testis or androgen level is reduced by the period resulted in a significantly reduction in SLC number (by 40%), and led to a significantly lower ALC numbers by the adult, suggesting that androgen proflies in fetal period is still important to the development of ALC in the late life (Kilcoyne et al., 2014).
Notch
Notch signaling plays a critical role in determining cell fate and maintaining stem cells numbers (Lai, 2004). Four Notch receptors (Notch1–Notch4), the transmembrane receptors, can bind to multiple Notch ligands, including delta-like 1, delta-like 3, delta-like 4, jagged 1, and jagged 2. Binding the ligands activates γ-secretase-dependent proteolysis within the transmembrane domain to release the Notch intracellular domain (De Strooper et al., 1999; Huppert et al., 2000). The domain is then translocated to the nucleus where it binds DNA in a complex with an obligate co-factor, RBPJK (Jarriault et al., 1995), activating the transcription of its downstream target effectors, the HES and HEY proteins. The HEY and HES proteins are two families of basic helix-loop-helix Orange transcriptional repressors (Nakagawa et al., 2000).
Notch signaling pathway may play an important role in LC development. Notch signaling proteins are expressed in the fetal testis, ILCs and ALCs (Tang et al., 2008). Activation of Notch signaling in gonadal SLCs dramatically reduces FLC numbers (Tang et al., 2008). Conditional deletion of jagged 1 (a Notch ligand) in the interstitial cells leads to a significant increase in ALC numbers (Defalco et al., 2013). Activation of Notch signaling represses the GATA4-related promoter activity which may inhibit the expression of steroidogenesis-related genes (Star and Hsd3b) (George et al., 2015). Blocking Notch signaling by a γ-secretase inhibitor DAPT, can significantly promote the differentiation of SLCs into ALCs in a rat seminiferous tubule culture system (Li et al., 2016).
Other Factors
In addition to the factors discussed above, there are other factors that may also have potential effects on the LC development, although the in vivo evidences or specific mechanistic studies for these factors are lacking.
In addition to androgen, estrogen may also affect LC development. Two types of estrogen receptors α and β have been identified. Estrogen receptor α and β are detected in mouse and rat LCs (Zhou et al., 2002; Akingbemi et al., 2003). Estrogen receptors α is also found in the LC lineage (Zhou et al., 2002; Akingbemi et al., 2003). In the estrogen receptor knockout mice, LC steroidogenic capacity is increased relative to wild-type controls (Akingbemi et al., 2003). However, since estrogens are capable of affecting pituitary LH secretions by a negative feedback mechanism, these in vivo results cannot distinguish a direct effect of estrogen from an indirect effect from the pituitary LH (Akingbemi et al., 2003), though there is in vitro evidence to suggest the former. For example, estrogen seems able to bind G protein-coupled receptor 30 and inhibit LC steroidogenesis (Vaucher et al., 2014).
Epidermal growth factor (EGF) family contains seven ligands: EGF, transforming growth factor-α (TGFA), heparin-binding EGF-like growth factor, betacellulin, amphiregulin, epiregulin, and epigen (Singh et al., 2016). They bind to a family of receptors including the EGF receptor (EGFR), HER2, HER3, and HER4 (Singh et al., 2016). EGF is able to stimulate rat ILC proliferation via the phosphorylation of ERK1/2 and Akt (Tai et al., 2009). EGF is also capable of stimulating steroidogenesis of ALCs (Verhoeven and Cailleau, 1986). Another way that EGF signaling can affect Leydig cells is that the receptor can be trans-activated by LH/cAMP/PKA signaling which can further induce the subsequent activation of mitogen-activated protein kinase (MAPK), ultimately leading to StAR phosphorylation and mitochondrial translocation (Evaul and Hammes, 2008).
TGFA is another member of the EGF family. TGFA is present in the rat LC lineage, from as early as the progenitor stage (Teerds et al., 1990). TGFA stimulates PLC proliferation like EGF (Khan et al., 1992b) and increases the androgen production, as well as the expression levels of Cyp17a1, Star, and Scarb1, suggesting that it can function as a positive regulator in ILC development (Millena et al., 2004).
Wnt4 is a member of the Wnt family. Wnt4 binds to Frizzled cell-surface receptors to activate signaling cascades to eventually affect gene expressions. Overexpression of Wnt4 disrupts the formation of FLCs and thus the androgen production, possibly by affecting the migration of SLCs into the testis cord (Jeays-Ward et al., 2003; Jordan et al., 2003). The activation of Wnt signaling leads to the inhibition of GSK3β (Valvezan and Klein, 2012). Indeed, in vitro experiment indicated that the metal lithium, which inhibits GSK3β, robustly stimulated SLC and PLC differentiation (Li et al., 2016).
Interleukins (including interleukin-1, 2, and 6) are produced by testicular macrophages. They may play a role in LC development since deletion of testicular macrophages dramatically delayed the regeneration of LCs in the ethane dimethane sulfonate-treated model (Gaytan et al., 1994). In vitro, interleukin-1β (IL1) stimulates the mitosis of PLCs (Khan et al., 1992a). Interleukins are certainly a group of molecules that have potentials to influence LC development and functions, though there is still debate about their specific effects on steroidogenesis (Calkins et al., 1988; Guo et al., 1990; Hales, 1992).
Role of Sertoli Cells
Sertoli cells play a very critical role in the development of LCs. When ILCs were co-cultured with Sertoli cells, they produced much higher amount of androgens and developed more abundant smooth endoplasmic reticulum (Tabone et al., 1984; Perrard-Sapori et al., 1987). Deletion of Sertoli cells during the neonatal and adult period caused significant reductions in PLC and ALC numbers (Rebourcet et al., 2014b). However, deletion of Sertoli cells during the fetal period seems not affecting FLCs (Rebourcet et al., 2014b). As shown above, specific knockout of NR3C4 in Sertoli cells significantly affected ALC development, indicating that NR3C4 may play an important role in regulating Sertoli cell paracine factors that may be critical for ALC development and functions.
Summary
The postnatal development of ALCs begins with a pool of SLCs. Several pieces of evidence suggest that SLCs are multipotent and are able to differentiate into different types of cells, in addition to cells in Leydig lineage. It has been shown that SLCs are able to self-renew indefinitely, differentiate and thus commit to the LC lineage, and replenish their niche in the testes. After SLC commitment into Leydig lineage, there are two distinct intermediate stages before the cells matured to ALC. The first stage is represented by spindle-shaped PLC that expresses the steroidogenic enzymes CYP11A1, HSD3B, and CYP17A1 and then followed by the second stage, the ovoid-shaped ILCs that expresses HSD17B3 and HSD11B1. The transitions from SLC to PLC and ILC and finally to ALC are regulated by multiple hormones (LH and androgen) and growth factors (leukemia inhibitory factor, Desert Hedgehog, Platelet-derived growth factors, Kit ligand, insulin-like growth factor 1, fibroblast growth factor 2, notch ligands, and transforming growth factors, etc.). The understanding of the development of SLCs into the LC lineage will surely help us to better understand the development of male reproductive system and the mechanisms behind some of the testicular dysgenesis syndrome and hypogonadism, as well as how environmental factors may affect the male reproductive system. There are still plenty unknowns about SLCs and their regulation. The studies of SLCs themselves could benefit the regenerative medicine that the hypogonadism and infertility could be managed with stem cell solutions someday.
Author Contributions
LY and RG wrote the review. XL prepared tables. LL prepared figures. HC reviewed the manuscript.
Conflict of Interest Statement
The authors declare that the research was conducted in the absence of any commercial or financial relationships that could be construed as a potential conflict of interest.
Acknowledgments
The research is supported by NSFC grants (31171425, 81300471, 81471411, 81601264, and 81601266) and Zhejiang Provincial NSF funding (LY15H310008).
References
Adham, I. M., Emmen, J. M., and Engel, W. (2000). The role of the testicular factor INSL3 in establishing the gonadal position. Mol. Cell. Endocrinol. 160, 11–16. doi: 10.1016/S0303-7207(99)00188-4
Agarwal, A. K., Monder, C., Eckstein, B., and White, P. C. (1989). Cloning and expression of rat cDNA encoding corticosteroid 11β-dehydrogenase. J. Biol. Chem. 264, 18939–18943.
Akingbemi, B. T., Ge, R., Rosenfeld, C. S., Newton, L. G., Hardy, D. O., Catterall, J. F., et al. (2003). Estrogen receptor-alpha gene deficiency enhances androgen biosynthesis in the mouse Leydig cell. Endocrinology 144, 84–93. doi: 10.1210/en.2002-220292
Ariyaratne, H. B., Mendis-Handagama, S. M., Hales, D. B., and Mason, I. J. (2000). Studies of the onset of Leydig cell differentiation in the prepubertal rat testis. Biol. Reprod. 63, 165–171. doi: 10.1095/biolreprod63.1.165
Augustowska, K., Gregoraszczuk, E. E., Grochowalski, A., Milewicz, T., Mika, M., Krzysiek, J., et al. (2003a). Comparison of accumulation and altered steroid secretion by placental tissue treated with TCDD and natural mixture of PCDDs-PCDFs. Reproduction 126, 681–687. doi: 10.1530/rep.0.1260681
Augustowska, K., Gregoraszczuk, E. L., Milewicz, T., Krzysiek, J., Grochowalski, A., and Chrzaszcz, R. (2003b). Effects of dioxin (2,3,7,8-TCDD) and PCDDs/PCDFs congeners mixture on steroidogenesis in human placenta tissue culture. Endocr. Regul. 37, 11–19.
Baker, J., Hardy, M. P., Zhou, J., Bondy, C., Lupu, F., Bellve, A. R., et al. (1996). Effects of an Igf1 gene null mutation on mouse reproduction. Mol. Endocrinol. 10, 903–918.
Baker, P. J., and O'Shaughnessy, P. J. (2001). Role of gonadotropin in regulating numbers of Leydig and Sertoli cells during fetal and postnatal development in mice. Reproduction 122, 227–234. doi: 10.1530/rep.0.1220227
Barsoum, I. B., Kaur, J., Ge, R. S., Cooke, P. S., and Yao, H. H. (2013). Dynamic changes in fetal Leydig cell populations influence adult Leydig cell populations in mice. FASEB J. 27, 2657–2666. doi: 10.1096/fj.12-225060
Benton, L., Shan, L. X., and Hardy, M. P. (1995). Differentiation of adult Leydig cells. J. Steroid. Biochem. Mol. Biol. 53, 61–68. doi: 10.1016/0960-0760(95)00022-R
Bergeron, F., Bagu, E. T., and Tremblay, J. J. (2011). Transcription of platelet-derived growth factor receptor alpha in Leydig cells involves specificity protein 1 and 3. J. Mol. Endocrinol. 46, 125–138. doi: 10.1530/JME-10-0145
Bitgood, M. J., Shen, L., and Mcmahon, A. P. (1996). Sertoli cell signaling by Desert hedgehog regulates the male germline. Curr. Biol. 6, 298–304. doi: 10.1016/S0960-9822(02)00480-3
Borch, J., Dalgaard, M., and Ladefoged, O. (2005). Early testicular effects in rats perinatally exposed to DEHP in combination with DEHA–apoptosis assessment and immunohistochemical studies. Reprod. Toxicol. 19, 517–525. doi: 10.1016/j.reprotox.2004.11.004
Bremner, W. J., Millar, M. R., Sharpe, R. M., and Saunders, P. T. (1994). Immunohistochemical localization of androgen receptors in the rat testis: evidence for stage-dependent expression and regulation by androgens. Endocrinology 135, 1227–1234. doi: 10.1210/endo.135.3.8070367
Brennan, J., Tilmann, C., and Capel, B. (2003). Pdgfr-alpha mediates testis cord organization and fetal Leydig cell development in the XY gonad. Genes Dev. 17, 800–810. doi: 10.1101/gad.1052503
Burger, H. G., and Igarashi, M. (1988). Inhibin: definition and nomenclature, including related substances. J. Clin. Endocrinol. Metab. 66, 885–886. doi: 10.1677/joe.0.1170159
Cailleau, J., Vermeire, S., and Verhoeven, G. (1990). Independent control of the production of insulin-like growth factor I and its binding protein by cultured testicular cells. Mol. Cell. Endocrinol. 69, 79–89. doi: 10.1016/0303-7207(90)90091-L
Calkins, J. H., Sigel, M. M., Nankin, H. R., and Lin, T. (1988). Interleukin-1 inhibits Leydig cell steroidogenesis in primary culture. Endocrinology 123, 1605–1610. doi: 10.1210/endo-123-3-1605
Chang, C., Chen, Y. T., Yeh, S. D., Xu, Q., Wang, R. S., Guillou, F., et al. (2004). Infertility with defective spermatogenesis and hypotestosteronemia in male mice lacking the androgen receptor in Sertoli cells. Proc. Natl. Acad. Sci. U.S.A. 101, 6876–6881. doi: 10.1073/pnas.0307306101
Chen, H., Ge, R. S., and Zirkin, B. R. (2009). Leydig cells: From stem cells to aging. Mol. Cell. Endocrinol. 306, 9–16. doi: 10.1016/j.mce.2009.01.023
Chen, H., Wang, Y., Ge, R., and Zirkin, B. R. (2017). Leydig cell stem cells: identification, proliferation and differentiation. Mol. Cell. Endocrinol. 445, 65–73. doi: 10.1016/j.mce.2016.10.010
Chen, M., Wang, X., Wang, Y., Zhang, L., Xu, B., Lv, L., et al. (2014). Wt1 is involved in leydig cell steroid hormone biosynthesis by regulating paracrine factor expression in mice. Biol. Reprod. 90:71. doi: 10.1095/biolreprod.113.114702
Cheng, L., Gearing, D. P., White, L. S., Compton, D. L., Schooley, K., and Donovan, P. J. (1994). Role of leukemia inhibitory factor and its receptor in mouse primordial germ cell growth. Development 120, 3145–3153.
Clark, A. M., Garland, K. K., and Russell, L. D. (2000). Desert hedgehog (Dhh) gene is required in the mouse testis for formation of adult-type Leydig cells and normal development of peritubular cells and seminiferous tubules. Biol. Reprod. 63, 1825–1838. doi: 10.1095/biolreprod63.6.1825
Clark, B. J., Soo, S. C., Caron, K. M., Ikeda, Y., Parker, K. L., and Stocco, D. M. (1995). Hormonal and developmental regulation of the steroidogenic acute regulatory protein. Mol. Endocrinol. 9, 1346–1355.
Codesal, J., Regadera, J., Nistal, M., Regadera-Sejas, J., and Paniagua, R. (1990). Involution of human fetal Leydig cells. An immunohistochemical, ultrastructural and quantitative study. J. Anat. 172, 103–114.
Davidoff, M. S., Middendorff, R., Enikolopov, G., Riethmacher, D., Holstein, A. F., and Muller, D. (2004). Progenitor cells of the testosterone-producing Leydig cells revealed. J. Cell Biol. 167, 935–944. doi: 10.1083/jcb.200409107
Defalco, T., Saraswathula, A., Briot, A., Iruela-Arispe, M. L., and Capel, B. (2013). Testosterone levels influence mouse fetal Leydig cell progenitors through notch signaling. Biol. Reprod. 88:91. doi: 10.1095/biolreprod.112.106138
De Gendt, K., Atanassova, N., Tan, K. A., De Franca, L. R., Parreira, G. G., Mckinnell, C., et al. (2005). Development and function of the adult generation of Leydig cells in mice with Sertoli cell-selective or total ablation of the androgen receptor. Endocrinology 146, 4117–4126. doi: 10.1210/en.2005-0300
De Strooper, B., Annaert, W., Cupers, P., Saftig, P., Craessaerts, K., Mumm, J. S., et al. (1999). A presenilin-1-dependent gamma-secretase-like protease mediates release of Notch intracellular domain. Nature 398, 518–522. doi: 10.1038/19083
Dixon, M. J., Gray, A., Boisvert, F. M., Agacan, M., Morrice, N. A., Gourlay, R., et al. (2011). A screen for novel phosphoinositide 3-kinase effector proteins. Mol. Cell. Proteomics 10:M110 003178. doi: 10.1074/mcp.M110.003178
Dombrowicz, D., Hooghe-Peters, E. L., Gothot, A., Sente, B., Vanhaelst, L., Closset, J., et al. (1992). Cellular localization of IGF-I and IGF-II mRNAs in immature hypophysectomized rat testis and epididymis after in vivo hormonal treatment. Arch. Int. Physiol. Biochim. Biophys. 100, 303–308. doi: 10.3109/13813459209000717
Dombrowicz, D., Sente, B., Reiter, E., Closset, J., and Hennen, G. (1996). Pituitary control of proliferation and differentiation of Leydig cells and their putative precursors in immature hypophysectomized rat testis. J. Androl. 17, 639–650.
Eskola, V., Rannikko, A., Huhtaniemi, I., and Warren, D. W. (1994). Ontogeny of the inhibitory guanine nucleotide-binding regulatory protein in the rat testis: mRNA expression and modulation of LH and FSH action. Mol. Cell. Endocrinol. 102, 63–68. doi: 10.1016/0303-7207(94)90098-1
Eswarakumar, V. P., Lax, I., and Schlessinger, J. (2005). Cellular signaling by fibroblast growth factor receptors. Cytokine Growth Factor Rev. 16, 139–149. doi: 10.1016/j.cytogfr.2005.01.001
Evaul, K., and Hammes, S. R. (2008). Cross-talk between G protein-coupled and epidermal growth factor receptors regulates gonadotropin-mediated steroidogenesis in Leydig cells. J. Biol. Chem. 283, 27525–27533. doi: 10.1074/jbc.M803867200
Gaytan, F., Bellido, C., Morales, C., Reymundo, C., Aguilar, E., and Van Rooijen, N. (1994). Selective depletion of testicular macrophages and prevention of Leydig cell repopulation after treatment with ethylene dimethane sulfonate in rats. J. Reprod. Fertil. 101, 175–182. doi: 10.1530/jrf.0.1010175
Ge, R. S., Dong, Q., Sottas, C. M., Chen, H., Zirkin, B. R., and Hardy, M. P. (2005). Gene expression in rat leydig cells during development from the progenitor to adult stage: a cluster analysis. Biol. Reprod. 72, 1405–1415. doi: 10.1095/biolreprod.104.037499
Ge, R. S., Dong, Q., Sottas, C. M., Papadopoulos, V., Zirkin, B. R., and Hardy, M. P. (2006). In search of rat stem Leydig cells: identification, isolation, and lineage-specific development. Proc. Natl. Acad. Sci. U.S.A. 103, 2719–2724. doi: 10.1073/pnas.0507692103
Ge, R. S., Gao, H. B., Nacharaju, V. L., Gunsalus, G. L., and Hardy, M. P. (1997a). Identification of a kinetically distinct activity of 11beta-hydroxysteroid dehydrogenase in rat Leydig cells. Endocrinology 138, 2435–2442. doi: 10.1210/endo.138.6.5165
Ge, R. S., Hardy, D. O., Catterall, J. F., and Hardy, M. P. (1997b). Developmental changes in glucocorticoid receptor and 11beta-hydroxysteroid dehydrogenase oxidative and reductive activities in rat Leydig cells. Endocrinology 138, 5089–5095. doi: 10.1210/endo.138.12.5614
Ge, R. S., Hardy, D. O., Catterall, J. F., and Hardy, M. P. (1999). Opposing changes in 3alpha-hydroxysteroid dehydrogenase oxidative and reductive activities in rat leydig cells during pubertal development. Biol. Reprod. 60, 855–860. doi: 10.1095/biolreprod60.4.855
Ge, R. S., and Hardy, M. P. (1997). Decreased cyclin A2 and increased cyclin G1 levels coincide with loss of proliferative capacity in rat Leydig cells during pubertal development. Endocrinology 138, 3719–3726. doi: 10.1210/endo.138.9.5387
Ge, R. S., and Hardy, M. P. (1998). Variation in the end products of androgen biosynthesis and metabolism during postnatal differentiation of rat Leydig cells. Endocrinology 139, 3787–3795. doi: 10.1210/endo.139.9.6183
Ge, R. S., and Hardy, M. P. (2007). “Regulation of Leydig cells during pubertal development,” in The Leydig Cell in Health and Disease, eds A. H. Payne and M. P. Hardy (Totowa, NJ: Humana Press), 55–70.
George, R. M., Hahn, K. L., Rawls, A., Viger, R. S., and Wilson-Rawls, J. (2015). Notch signaling represses GATA4-induced expression of genes involved in steroid biosynthesis. Reproduction 150, 383–394. doi: 10.1530/REP-15-0226
Gnessi, L., Basciani, S., Mariani, S., Arizzi, M., Spera, G., Wang, C., et al. (2000). Leydig cell loss and spermatogenic arrest in platelet-derived growth factor (PDGF)-A-deficient mice. J. Cell Biol. 149, 1019–1026. doi: 10.1083/jcb.149.5.1019
Gnessi, L., Emidi, A., Jannini, E. A., Carosa, E., Maroder, M., Arizzi, M., et al. (1995). Testicular development involves the spatiotemporal control of PDGFs and PDGF receptors gene expression and action. J. Cell Biol. 131, 1105–1121. doi: 10.1083/jcb.131.4.1105
Golshan, M., Hatef, A., Zare, A., Socha, M., Milla, S., Gosiewski, G., et al. (2014). Alternations in neuroendocrine and endocrine regulation of reproduction in male goldfish (Carassius auratus) following an acute and chronic exposure to vinclozolin, in vivo. Aquat. Toxicol. 155, 73–83. doi: 10.1016/j.aquatox.2014.06.004
Goumans, M. J., Valdimarsdottir, G., Itoh, S., Rosendahl, A., Sideras, P., and Ten Dijke, P. (2002). Balancing the activation state of the endothelium via two distinct TGF-beta type I receptors. EMBO J. 21, 1743–1753. doi: 10.1093/emboj/21.7.1743
Guo, H., Calkins, J. H., Sigel, M. M., and Lin, T. (1990). Interleukin-2 is a potent inhibitor of Leydig cell steroidogenesis. Endocrinology 127, 1234–1239. doi: 10.1210/endo-127-3-1234
Gut, P., Huber, K., Lohr, J., Bruhl, B., Oberle, S., Treier, M., et al. (2005). Lack of an adrenal cortex in Sf1 mutant mice is compatible with the generation and differentiation of chromaffin cells. Development 132, 4611–4619. doi: 10.1242/dev.02052
Ha, W. T., Jeong, H. Y., Lee, S. Y., and Song, H. (2016). Effects of the insulin-like growth factor pathway on the regulation of mammary gland development. Dev. Reprod. 20, 179–185. doi: 10.12717/DR.2016.20.3.179
Habert, R., and Brignaschi, P. (1991). Developmental changes in in vitro testosterone production by dispersed Leydig cells during fetal life in rats. Arch. Androl. 27, 65–71. doi: 10.3109/01485019108987654
Hales, D. B. (1992). Interleukin-1 inhibits Leydig cell steroidogenesis primarily by decreasing 17 alpha-hydroxylase/C17-20 lyase cytochrome P450 expression. Endocrinology 131, 2165–2172. doi: 10.1210/endo.131.5.1425417
Handelsman, D. J., Spaliviero, J. A., Scott, C. D., and Baxter, R. C. (1985). Identification of insulin-like growth factor-I and its receptors in the rat testis. Acta Endocrinol. 109, 543–549. doi: 10.1530/acta.0.1090543
Hardy, D. O., Ge, R. S., Catterall, J. F., Hou, Y. T., Penning, T. M., and Hardy, M. P. (2000). Identification of the oxidative 3alpha-hydroxysteroid dehydrogenase activity of rat Leydig cells as type II retinol dehydrogenase. Endocrinology 141, 1608–1617. doi: 10.1210/endo.141.5.7445
Hardy, M. P., Kelce, W. R., Klinefelter, G. R., and Ewing, L. L. (1990). Differentiation of Leydig cell precursors in vitro: a role for androgen. Endocrinology 127, 488–490. doi: 10.1210/endo-127-1-488
Hardy, M. P., Zirkin, B. R., and Ewing, L. L. (1989). Kinetic studies on the development of the adult population of Leydig cells in testes of the pubertal rat. Endocrinology 124, 762–770. doi: 10.1210/endo-124-2-762
Hazra, R., Jimenez, M., Desai, R., Handelsman, D. J., and Allan, C. M. (2013). Sertoli cell androgen receptor expression regulates temporal fetal and adult Leydig cell differentiation, function, and population size. Endocrinology 154, 3410–3422. doi: 10.1210/en.2012-2273
Heinrich, P. C., Behrmann, I., Muller-Newen, G., Schaper, F., and Graeve, L. (1998). Interleukin-6-type cytokine signalling through the gp130/Jak/STAT pathway. Biochem. J. 334 (Pt 2), 297–314. doi: 10.1042/bj3340297
Hu, G. X., Lian, Q. Q., Chen, B. B., Prasad, P. V., Kumar, N., Zheng, Z. Q., et al. (2010a). 7alpha-hydroxytestosterone affects 11beta-hydroxysteroid dehydrogenase 1 direction in rat Leydig cells. Endocrinology 151, 748–754. doi: 10.1210/en.2009-0917
Hu, G. X., Lin, H., Chen, G. R., Chen, B. B., Lian, Q. Q., Hardy, D. O., et al. (2010b). Deletion of the Igf1 gene: suppressive effects on adult Leydig cell development. J. Androl. 31, 379–387. doi: 10.2164/jandrol.109.008680
Hu, Y. C., Okumura, L. M., and Page, D. C. (2013). Gata4 is required for formation of the genital ridge in mice. PLoS Genet. 9:e1003629. doi: 10.1371/journal.pgen.1003629
Huhtaniemi, I., and Pelliniemi, L. J. (1992). Fetal Leydig cells: cellular origin, morphology, life span, and special functional features. Proc. Soc. Exp. Biol. Med. 201, 125–140. doi: 10.3181/00379727-201-43493
Huppert, S. S., Le, A., Schroeter, E. H., Mumm, J. S., Saxena, M. T., Milner, L. A., et al. (2000). Embryonic lethality in mice homozygous for a processing-deficient allele of Notch1. Nature 405, 966–970. doi: 10.1038/35016111
Hutson, J. M., and Hasthorpe, S. (2005). Abnormalities of testicular descent. Cell Tiss. Res. 322, 155–158. doi: 10.1007/s00441-005-1126-4
Ingham, P. W., and Mcmahon, A. P. (2001). Hedgehog signaling in animal development: paradigms and principles. Genes Dev. 15, 3059–3087. doi: 10.1101/gad.938601
Israel, J. M., Cabelguen, J. M., Le Masson, G., Oliet, S. H., and Ciofi, P. (2014). Neonatal testosterone suppresses a neuroendocrine pulse generator required for reproduction. Nat. Commun. 5, 3285–3295. doi: 10.1038/ncomms4285
Jarriault, S., Brou, C., Logeat, F., Schroeter, E. H., Kopan, R., and Israel, A. (1995). Signallingdownstream of activated mammalian Notch. Nature 377, 355–358.
Jeays-Ward, K., Hoyle, C., Brennan, J., Dandonneau, M., Alldus, G., Capel, B., et al. (2003). Endothelial and steroidogenic cell migration are regulated by WNT4 in the developing mammalian gonad. Development 130, 3663–3670. doi: 10.1242/dev.00591
Jiang, M. H., Cai, B., Tuo, Y., Wang, J., Zang, Z. J., Tu, X., et al. (2014). Characterization of Nestin-positive stem Leydig cells as a potential source for the treatment of testicular Leydig cell dysfunction. Cell Res. 24, 1466–1485. doi: 10.1038/cr.2014.149
Jordan, B. K., Shen, J. H., Olaso, R., Ingraham, H. A., and Vilain, E. (2003). Wnt4 overexpression disrupts normal testicular vasculature and inhibits testosterone synthesis by repressing steroidogenic factor 1/beta-catenin synergy. Proc. Natl. Acad. Sci. U.S.A. 100, 10866–10871. doi: 10.1073/pnas.1834480100
Kai, H., Shono, T., Tajiri, T., and Suita, S. (2005). Long-term effects of intrauterine exposure to mono-n-butyl phthalate on the reproductive function of postnatal rats. J. Pediatr. Surg. 40, 429–433. doi: 10.1016/j.jpedsurg.2004.10.009
Kawai, Y., Noguchi, J., Akiyama, K., Takeno, Y., Fujiwara, Y., Kajita, S., et al. (2010). A missense mutation of the Dhh gene is associated with male pseudohermaphroditic rats showing impaired Leydig cell development. Reproduction 141, 217–225. doi: 10.1530/REP-10-0006
Kerr, J. B., and Knell, C. M. (1988). The fate of fetal Leydig cells during the development of the fetal and postnatal rat testis. Development 103, 535–544.
Khan, S. A., Khan, S. J., and Dorrington, J. H. (1992a). Interleukin-1 stimulates deoxyribonucleic acid synthesis in immature rat Leydig cells in vitro. Endocrinology 131, 1853–1857. doi: 10.1210/endo.131.4.1396331
Khan, S. A., Mirsafian, M., Howdeshell, K., and Dorrington, J. H. (1999). Transforming growth factor-beta inhibits DNA synthesis in immature rat Leydig cells in vitro. Mol. Cell. Endocrinol. 148, 21–28. doi: 10.1016/S0303-7207(98)00244-5
Khan, S., Teerds, K., and Dorrington, J. (1992b). Growth factor requirements for DNA synthesis by Leydig cells from the immature rat. Biol. Reprod. 46, 335–341. doi: 10.1095/biolreprod46.3.335
Kihara, A., Ikeda, M., Kariya, Y., Lee, E. Y., Lee, Y. M., and Igarashi, Y. (2003). Sphingosine-1-phosphate lyase is involved in the differentiation of F9 embryonal carcinoma cells to primitive endoderm. J. Biol. Chem. 278, 14578–14585. doi: 10.1074/jbc.M211416200
Kilcoyne, K. R., Smith, L. B., Atanassova, N., Macpherson, S., Mckinnell, C., Van Den Driesche, S., et al. (2014). Fetal programming of adult Leydig cell function by androgenic effects on stem/progenitor cells. Proc. Natl. Acad. Sci. U.S.A. 111, E1924–1932. doi: 10.1073/pnas.1320735111
Lai, E. C. (2004). Notch signaling: control of cell communication and cell fate. Development 131, 965–973. doi: 10.1242/dev.01074
Lei, Z. M., Mishra, S., Zou, W., Xu, B., Foltz, M., Li, X., et al. (2001). Targeted disruption of luteinizing hormone/human chorionic gonadotropin receptor gene. Mol. Endocrinol. 15, 184–200. doi: 10.1210/mend.15.1.0586
Le Roy, C., Lejeune, H., Chuzel, F., Saez, J. M., and Langlois, D. (1999). Autocrine regulation of Leydig cell differentiated functions by insulin-like growth factor I and transforming growth factor beta. J. Steroid Biochem. Mol. Biol. 69, 379–384. doi: 10.1016/S0960-0760(99)00075-8
Li, L., Wang, Y., Li, X., Liu, S., Wang, G., Lin, H., et al. (2017). Regulation of development of rat stem and progenitor Leydig cells by activin. Andrology 5, 125–132. doi: 10.1111/andr.12253
Li, X., Hu, G., Wang, Y. Y., Hu, Y. Y., Zhou, H., Latif, S. A., et al. (2015). Metabolic coupling determines the activity: comparison of 11beta-hydroxysteroid dehydrogenase 1 and its coupling between liver parenchymal cells and testicular leydig cells. PLoS ONE 10:e0141767. doi: 10.1371/journal.pone.0141767
Li, X., Wang, Z., Jiang, Z., Guo, J., Zhang, Y., Li, C., et al. (2016). Regulation of seminiferous tubule-associated stem Leydig cells in adult rat testes. Proc. Natl. Acad. Sci. U.S.A. 113, 2666–2671. doi: 10.1073/pnas.1519395113
Lin, T., Blaisdell, J., and Haskell, J. F. (1987). Transforming growth factor-beta inhibits Leydig cell steroidogenesis in primary culture. Biochem. Biophys. Res. Commun. 146, 387–394. doi: 10.1016/0006-291X(87)90541-9
Lin, T., Blaisdell, J., and Haskell, J. F. (1988). Hormonal regulation of type I insulin-like growth factor receptors of Leydig cells in hypophysectomized rats. Endocrinology 123, 134–139. doi: 10.1210/endo-123-1-134
Lin, T., Calkins, J. K., Morris, P. L., Vale, W., and Bardin, C. W. (1989). Regulation of Leydig cell function in primary culture by inhibin and activin. Endocrinology 125, 2134–2140. doi: 10.1210/endo-125-4-2134
Lin, T., Haskell, J., Vinson, N., and Terracio, L. (1986a). Characterization of insulin and insulin-like growth factor I receptors of purified Leydig cells and their role in steroidogenesis in primary culture: a comparative study. Endocrinology 119, 1641–1647. doi: 10.1210/endo-119-4-1641
Lin, T., Haskell, J., Vinson, N., and Terracio, L. (1986b). Direct stimulatory effects of insulin-like growth factor-I on Leydig cell steroidogenesis in primary culture. Biochem. Biophys. Res. Commun. 137, 950–956. doi: 10.1016/0006-291X(86)90317-7
Liu, C., Rodriguez, K., and Yao, H. H. (2016). Mapping lineage progression of somatic progenitor cells in the mouse fetal testis. Development 143, 3700–3710. doi: 10.1242/dev.135756
Liu, H., Yang, Y., Zhang, L., Liang, R., Ge, R. S., Zhang, Y., et al. (2014). Basic fibroblast growth factor promotes stem Leydig cell development and inhibits LH-stimulated androgen production by regulating microRNA expression. J. Steroid. Biochem. Mol. Biol. 144 (Pt B), 483–491. doi: 10.1016/j.jsbmb.2014.09.016
Liu, S., Chen, X., Wang, Y., Li, L., Wang, G., Li, X., et al. (2017). A role of KIT receptor signaling for proliferation and differentiation of rat stem Leydig cells in vitro. Mol. Cell. Endocrinol. 444,1–8. doi: 10.1016/j.mce.2017.01.023
Lyet, L., Louis, F., Forest, M. G., Josso, N., Behringer, R. R., and Vigier, B. (1995). Ontogeny of reproductive abnormalities induced by deregulation of anti-mullerian hormone expression in transgenic mice. Biol. Reprod. 52, 444–454. doi: 10.1095/biolreprod52.2.444
Ma, X., Dong, Y., Matzuk, M. M., and Kumar, T. R. (2004). Targeted disruption of luteinizing hormone beta-subunit leads to hypogonadism, defects in gonadal steroidogenesis, and infertility. Proc. Natl. Acad. Sci. U.S.A. 101, 17294–17299. doi: 10.1073/pnas.0404743101
Manova, K., Nocka, K., Besmer, P., and Bachvarova, R. F. (1990). Gonadal expression of c-kit encoded at the W locus of the mouse. Development 110, 1057–1069.
Mariani, S., Basciani, S., Arizzi, M., Spera, G., and Gnessi, L. (2002). PDGF and the testis. Trends Endocrinol. Metab. 13, 11–17. doi: 10.1016/S1043-2760(01)00518-5
Martin, L. J., Boucher, N., Brousseau, C., and Tremblay, J. J. (2008). The orphan nuclear receptor NUR77 regulates hormone-induced StAR transcription in Leydig cells through cooperation with Ca2+/calmodulin-dependent protein kinase I. Mol. Endocrinol. 22, 2021–2037. doi: 10.1210/me.2007-0370
Martin, L. J., and Tremblay, J. J. (2005). The human 3beta-hydroxysteroid dehydrogenase/Delta5-Delta4 isomerase type 2 promoter is a novel target for the immediate early orphan nuclear receptor Nur77 in steroidogenic cells. Endocrinology 146, 861–869. doi: 10.1210/en.2004-0859
Meachem, S. J., Nieschlag, E., and Simoni, M. (2001). Inhibin B in male reproduction: pathophysiology and clinical relevance. Eur. J. Endocrinol. 145, 561–571. doi: 10.1530/eje.0.1450561
Mendis-Handagama, S. M., Di Clementi, N., Ariyaratne, H. B., and Mrkonjich, L. (2006). Detection of anti-Mullerian hormone receptor II protein in the postnatal rat testis from birth to sexual maturity. Histol. Histopathol. 21, 125–130.
Millena, A. C., Reddy, S. C., Bowling, G. H., and Khan, S. A. (2004). Autocrine regulation of steroidogenic function of Leydig cells by transforming growth factor-alpha. Mol. Cell. Endocrinol. 224, 29–39. doi: 10.1016/j.mce.2004.07.004
Min, L., Takemori, H., Nonaka, Y., Katoh, Y., Doi, J., Horike, N., et al. (2004). Characterization of the adrenal-specific antigen IZA (inner zone antigen) and its role in the steroidogenesis. Mol. Cell. Endocrinol. 215, 143–148. doi: 10.1016/j.mce.2003.11.025
Minegishi, T., Hirakawa, T., Abe, K., Kishi, H., and Miyamoto, K. (2003). Effect of IGF-1 and 2,3,7,8-tetrachlorodibenzo-p-dioxin (TCDD) on the expression of LH receptors during cell differentiation in cultured granulosa cells. Mol. Cell. Endocrinol. 202, 123–131. doi: 10.1016/S0303-7207(03)00073-X
Mishina, Y., Rey, R., Finegold, M. J., Matzuk, M. M., Josso, N., Cate, R. L., et al. (1996). Genetic analysis of the Mullerian-inhibiting substance signal transduction pathway in mammalian sexual differentiation. Genes Dev. 10, 2577–2587. doi: 10.1101/gad.10.20.2577
Moreno Frias, M., Jimenez Torres, M., Garrido Frenich, A., Martinez Vidal, J. L., Olea-Serrano, F., and Olea, N. (2004). Determination of organochlorine compounds in human biological samples by GC-MS/MS. Biomed. Chromatogr. 18, 102–111. doi: 10.1002/bmc.300
Moser, V. C., Barone, S. Jr., Smialowicz, R. J., Harris, M. W., Davis, B. J., Overstreet, D., et al. (2001). The effects of perinatal tebuconazole exposure on adult neurological, immunological, and reproductive function in rats. Toxicol. Sci. 62, 339–352. doi: 10.1093/toxsci/62.2.339
Murono, E. P., Washburn, A. L., Goforth, D. P., and Wu, N. (1993). Basic fibroblast growth factor-induced increase in 125I-human chorionic gonadotropin binding to luteinizing hormone receptors in cultured immature Leydig cells is mediated by binding to heparan sulfate proteoglycans. Mol. Cell. Endocrinol. 97, 109–114. doi: 10.1016/0303-7207(93)90216-7
Murphy, L., Jeffcoate, I. A., and O'Shaughnessy, P. J. (1994). Abnormal Leydig cell development at puberty in the androgen-resistant Tfm mouse. Endocrinology 135, 1372–1377. doi: 10.1210/endo.135.4.7925099
Nagpal, M. L., Wang, D., Calkins, J. H., Chang, W. W., and Lin, T. (1991). Human chorionic gonadotropin up-regulates insulin-like growth factor-I receptor gene expression of Leydig cells. Endocrinology 129, 2820–2826. doi: 10.1210/endo-129-6-2820
Nakagawa, O., Mcfadden, D. G., Nakagawa, M., Yanagisawa, H., Hu, T., Srivastava, D., et al. (2000). Members of the HRT family of basic helix-loop-helix proteins act as transcriptional repressors downstream of Notch signaling. Proc. Natl. Acad. Sci. U.S.A. 97, 13655–13660. doi: 10.1073/pnas.250485597
Nistal, M., Paniagua, R., Regadera, J., Santamaria, L., and Amat, P. (1986). A quantitative morphological study of human Leydig cells from birth to adulthood. Cell Tissue Res. 246, 229–236. doi: 10.1007/BF00215884
Odeh, H. M., Kleinguetl, C., Ge, R., Zirkin, B. R., and Chen, H. (2014). Regulation of the proliferation and differentiation of leydig stem cells in the adult testis. Biol. Reprod. 90:123. doi: 10.1095/biolreprod.114.117473
O'Hara, L., Mcinnes, K., Simitsidellis, I., Morgan, S., Atanassova, N., Slowikowska-Hilczer, J., et al. (2014). Autocrine androgen action is essential for Leydig cell maturation and function, and protects against late-onset Leydig cell apoptosis in both mice and men. FASEB J. 29, 894–910. doi: 10.1096/fj.14-255729
O'Shaughnessy, P. J., Baker, P. J., Heikkila, M., Vainio, S., and Mcmahon, A. P. (2000). Localization of 17beta-hydroxysteroid dehydrogenase/17-ketosteroid reductase isoform expression in the developing mouse testis–androstenedione is the major androgen secreted by fetal/neonatal leydig cells. Endocrinology 141, 2631–2637. doi: 10.1210/endo.141.7.7545
O'Shaughnessy, P. J., Johnston, H., Willerton, L., and Baker, P. J. (2002). Failure of normal adult Leydig cell development in androgen-receptor-deficient mice. J. Cell Sci. 115, 3491–3496.
O'Shaughnessy, P. J., and Murphy, L. (1993). Cytochrome P-450 17 α-hydroxylase protein and mRNA in the testis of the testicular feminized (Tfm) mouse. J. Mol. Endocrinol. 11, 77–82. doi: 10.1677/jme.0.0110077
Park, E., Song, C. H., Park, J. I., Ahn, R. S., Choi, H. S., Ko, C., et al. (2014). Transforming growth factor-beta1 signaling represses testicular steroidogenesis through cross-talk with orphan nuclear receptor Nur77. PLoS ONE 9:e104812. doi: 10.1371/journal.pone.0104812
Park, S. Y., Tong, M., and Jameson, J. L. (2007). Distinct roles for steroidogenic factor 1 and desert hedgehog pathways in fetal and adult Leydig cell development. Endocrinology 148, 3704–3710. doi: 10.1210/en.2006-1731
Paul, C., Rhind, S. M., Kyle, C. E., Scott, H., Mckinnell, C., and Sharpe, R. M. (2005). Cellular and hormonal disruption of fetal testis development in sheep reared on pasture treated with sewage sludge. Environ. Health Perspect. 113, 1580–1587. doi: 10.1289/ehp.8028
Paulini, J., Higuti, E., Bastos, R. M., Gomes, S. A., and Rangel, E. B. (2016). Mesenchymal stem cells as therapeutic candidates for halting the progression of diabetic nephropathy. Stem Cells Int. 2016:9521629. doi: 10.1155/2016/9521629
Payne, A. H., and O'Shaughnessy, P. J. (1996). “Structure, function and regulation of steroidogenic enzymes in the Leydig cell,” in The Leydig cell, eds M. P. H. A.H. Payne, and L. D. Russell (Vienna, IL: Cache River Press), 259–286.
Perrard-Sapori, M. H., Chatelain, P. C., Rogemond, N., and Saez, J. M. (1987). Modulation of Leydig cell functions by culture with Sertoli cells or with Sertoli cell-conditioned medium: effect of insulin, somatomedin-C and FSH. Mol. Cell. Endocrinol. 50, 193–201. doi: 10.1016/0303-7207(87)90017-7
Phillips, D. M., Lakshmi, V., and Monder, C. (1989). Corticosteroid 11b-dehydrogenase in rat testis. Endocrinology 125, 209–216. doi: 10.1210/endo-125-1-209
Piquet-Pellorce, C., Dorval-Coiffec, I., Pham, M. D., and Jegou, B. (2000). Leukemia inhibitory factor expression and regulation within the testis. Endocrinology 141, 1136–1141. doi: 10.1210/endo.141.3.7399
Prince, F. P. (1990). Ultrastructural evidence of mature Leydig cells and Leydig cell regression in the neonatal human testis. Anat. Record 228, 405–417. doi: 10.1002/ar.1092280406
Prince, F. P. (1992). Ultrastructural evidence of indirect and direct autonomic innervation of human Leydig cells: comparison of neonatal, childhood and pubertal ages. Cell Tissue Res. 269, 383–390. doi: 10.1007/BF00353893
Prince, F. P. (2001). The triphasic nature of Leydig cell development in humans, and comments on nomenclature. J. Endocrinol. 168, 213–216. doi: 10.1677/joe.0.1680213
Qin, J., Tsai, M. J., and Tsai, S. Y. (2008). Essential roles of COUP-TFII in Leydig cell differentiation and male fertility. PLoS ONE 3:e3285. doi: 10.1371/journal.pone.0003285
Racine, C., Rey, R., Forest, M. G., Louis, F., Ferre, A., Huhtaniemi, I., et al. (1998). Receptors for anti-mullerian hormone on Leydig cells are responsible for its effects on steroidogenesis and cell differentiation. Proc. Natl. Acad. Sci. U.S.A. 95, 594–599. doi: 10.1073/pnas.95.2.594
Rebourcet, D., O'Shaughnessy, P. J., Monteiro, A., Milne, L., Cruickshanks, L., Jeffrey, N., et al. (2014a). Sertoli cells maintain Leydig cell number and peritubular myoid cell activity in the adult mouse testis. PLoS ONE 9:e105687. doi: 10.1371/journal.pone.0105687
Rebourcet, D., O'Shaughnessy, P. J., Pitetti, J. L., Monteiro, A., O'hara, L., Milne, L., et al. (2014b). Sertoli cells control peritubular myoid cell fate and support adult Leydig cell development in the prepubertal testis. Development 141, 2139–2149. doi: 10.1242/dev.107029
Rey, R. A., Codner, E., Iniguez, G., Bedecarras, P., Trigo, R., Okuma, C., et al. (2005). Low risk of impaired testicular Sertoli and Leydig cell functions in boys with isolated hypospadias. J. Clin. Endocrinol. Metab. 90, 6035–6040. doi: 10.1210/jc.2005-1306
Robertson, D. M., Risbridger, G. P., and De Kretser, D. M. (1992). The physiology of testicular inhibin and related proteins. 105. Baill. Clin. Endocrinol. Metab. 6, 355–372. doi: 10.1016/S0950-351X(05)80154-5
Rohatgi, R., Milenkovic, L., and Scott, M. P. (2007). Patched1 regulates hedgehog signaling at the primary cilium. Science 317, 372–376. doi: 10.1126/science.1139740
Rothschild, G., Sottas, C. M., Kissel, H., Agosti, V., Manova, K., Hardy, M. P., et al. (2003). A role for kit receptor signaling in Leydig cell steroidogenesis. Biol. Reprod. 69, 925–932. doi: 10.1095/biolreprod.102.014548
Rux, D. R., Song, J. Y., Swinehart, I. T., Pineault, K. M., Schlientz, A. J., Trulik, K. G., et al. (2016). Regionally restricted hox function in adult bone marrow multipotent mesenchymal stem/stromal cells. Dev. Cell 39, 653–666. doi: 10.1016/j.devcel.2016.11.008
Sadovsky, Y., Crawford, P. A., Woodson, K. G., Polish, J. A., Clements, M. A., Tourtellotte, L. M., et al. (1995). Mice deficient in the orphan receptor steroidogenic factor 1 lack adrenal glands and gonads but express P450 side-chain-cleavage enzyme in the placenta and have normal embryonic serum levels of corticosteroids. Proc. Natl. Acad. Sci. U.S.A. 92, 10939–10943. doi: 10.1073/pnas.92.24.10939
Salva, A., Hardy, M. P., Wu, X. F., Sottas, C. M., Maclaughlin, D. T., Donahoe, P. K., et al. (2004). Mullerian-inhibiting substance inhibits rat Leydig cell regeneration after ethylene dimethanesulphonate ablation. Biol. Reprod. 70, 600–607. doi: 10.1095/biolreprod.103.021550
Sandlow, J. I., Feng, H. L., Cohen, M. B., and Sandra, A. (1996). Expression of c-KIT and its ligand, stem cell factor, in normal and subfertile human testicular tissue. J. Androl. 17, 403–408.
Schmahl, J., Rizzolo, K., and Soriano, P. (2008). The PDGF signaling pathway controls multiple steroid-producing lineages. Genes Dev. 22, 3255–3267. doi: 10.1101/gad.1723908
Shan, L. X., and Hardy, M. P. (1992). Developmental changes in levels of luteinizing hormone receptor and androgen receptor in rat Leydig cells. Endocrinology 131, 1107–1114. doi: 10.1210/endo.131.3.1505454
Shan, L. X., Phillips, D. M., Bardin, C. W., and Hardy, M. P. (1993). Differential regulation of steroidogenic enzymes during differentiation optimizes testosterone production by adult rat Leydig cells. Endocrinology 133, 2277–2283. doi: 10.1210/endo.133.5.8404681
Shan, L. X., Zhu, L. J., Bardin, C. W., and Hardy, M. P. (1995). Quantitative analysis of androgen receptor messenger ribonucleic acid in developing Leydig cells and Sertoli cells by in situ hybridization. Endocrinology 136, 3856–3862. doi: 10.1210/endo.136.9.7649092
Sharpe, R. M. (2006). Pathways of endocrine disruption during male sexual differentiation and masculinization. Best Pract. Res. Clin. Endocrinol. Metab. 20, 91–110. doi: 10.1016/j.beem.2005.09.005
Shima, Y., Matsuzaki, S., Miyabayashi, K., Otake, H., Baba, T., Kato, S., et al. (2015). Fetal leydig cells persist as an androgen-independent subpopulation in the postnatal testis. Mol. Endocrinol. 29, 1581–1593. doi: 10.1210/me.2015-1200
Shima, Y., Miyabayashi, K., Haraguchi, S., Arakawa, T., Otake, H., Baba, T., et al. (2013). Contribution of Leydig and Sertoli cells to testosterone production in mouse fetal testes. Mol. Endocrinol. 27, 63–73. doi: 10.1210/me.2012-1256
Shiraishi, K., and Ascoli, M. (2007). Lutropin/choriogonadotropin stimulate the proliferation of primary cultures of rat Leydig cells through a pathway that involves activation of the extracellularly regulated kinase 1/2 cascade. Endocrinology 148, 3214–3225. doi: 10.1210/en.2007-0160
Singh, B., Carpenter, G., and Coffey, R. J. (2016). EGF receptor ligands: recent advances. F1000Res 5:F100. doi: 10.12688/f1000research.9025.1
Skinner, M. K., Mclachlan, R. I., and Bremner, W. J. (1989). Stimulation of Sertoli cell inhibin secretion by the testicular paracrine factor PModS. Mol. Cell. Endocrinol. 66, 239–249. doi: 10.1016/0303-7207(89)90036-1
Skinner, M. K., and Moses, H. L. (1989). Transforming growth factor beta gene expression and action in the seminiferous tubule: peritubular cell-Sertoli cell interactions. Mol. Endocrinol. 3, 625–634. doi: 10.1210/mend-3-4-625
Song, K. H., Park, J. I., Lee, M. O., Soh, J., Lee, K., and Choi, H. S. (2001). LH induces orphan nuclear receptor Nur77 gene expression in testicular Leydig cells. Endocrinology 142, 5116–5123. doi: 10.1210/endo.142.12.8525
Sriraman, V., Niu, E., Matias, J. R., Donahoe, P. K., Maclaughlin, D. T., Hardy, M. P., et al. (2001). Mullerian inhibiting substance inhibits testosterone synthesis in adult rats. J. Androl. 22, 750–758.
Stanley, E., Lin, C. Y., Jin, S., Liu, J., Sottas, C. M., Ge, R., et al. (2012). Identification, proliferation, and differentiation of adult Leydig stem cells. Endocrinology 153, 5002–5010. doi: 10.1210/en.2012-1417
Stanley, E. L., Johnston, D. S., Fan, J., Papadopoulos, V., Chen, H., Ge, R. S., et al. (2011). Stem Leydig cell differentiation: gene expression during development of the adult rat population of Leydig cells. Biol. Reprod. 85, 1161–1166. doi: 10.1095/biolreprod.111.091850
Stocco, D. M. (2001). Tracking the role of a StAR in the sky of the new millenium. Mol. Endocrinol. 15, 1245–1254. doi: 10.1210/mend.15.8.0697
Svechnikov, K., Izzo, G., Landreh, L., Weisser, J., and Soder, O. (2010). Endocrine disruptors and Leydig cell function. J. Biomed. Biotechnol. 2010:684504. doi: 10.1155/2010/684504
Tabone, E., Benahmed, M., Reventos, J., and Saez, J. M. (1984). Interactions between immature porcine Leydig and Sertoli cells in vitro. An ultrastructural and biochemical study. Cell Tissue Res. 237, 357–362. doi: 10.1007/BF00217157
Tai, P., Shiraishi, K., and Ascoli, M. (2009). Activation of the lutropin/choriogonadotropin receptor inhibits apoptosis of immature Leydig cells in primary culture. Endocrinology 150, 3766–3773. doi: 10.1210/en.2009-0207
Tang, H., Brennan, J., Karl, J., Hamada, Y., Raetzman, L., and Capel, B. (2008). Notch signaling maintains Leydig progenitor cells in the mouse testis. Development 135, 3745–3753. doi: 10.1242/dev.024786
Teerds, K. J., De Rooij, D. G., Rommerts, F. F., Van Den Hurk, R., and Wensing, C. J. (1989). Stimulation of the proliferation and differentiation of Leydig cell precursors after the destruction of existing Leydig cells with ethane dimethyl sulphonate (EDS) can take place in the absence of LH. J. Androl. 10, 472–477. doi: 10.1002/j.1939-4640.1989.tb00143.x
Teerds, K. J., De Rooij, D. G., Rommerts, F. F., and Wensing, C. J. (1988). The regulation of the proliferation and differentiation of rat Leydig cell precursor cells after EDS administration or daily HCG treatment. J. Androl. 9, 343–351. doi: 10.1002/j.1939-4640.1988.tb01061.x
Teerds, K. J., and Dorrington, J. H. (1993). Localization of transforming growth factor beta 1 and beta 2 during testicular development in the rat. Biol. Reprod. 48, 40–45.
Teerds, K. J., Rommerts, F. F., and Dorrington, J. H. (1990). Immunohistochemical detection of transforming growth factor-alpha in Leydig cells during the development of the rat testis. Mol. Cell Endocrinol. 69, R1–R6. doi: 10.1095/biolreprod48.1.40
Tevosian, S. G. (2014). Transgenic mouse models in the study of reproduction: insights into GATA protein function. Reproduction 148, R1–R14. doi: 10.1530/REP-14-0086
Trbovich, A. M., Martinelle, N., O'Neill, F. H., Pearson, E. J., Donahoe, P. K., Sluss, P. M., et al. (2004). Steroidogenic activities in MA-10 Leydig cells are differentially altered by cAMP and Mullerian inhibiting substance. J. Steroid Biochem. Mol. Biol. 92, 199–208. doi: 10.1016/j.jsbmb.2004.07.002
Ueno, N., Baird, A., Esch, F., Ling, N., and Guillemin, R. (1987). Isolation and partial characterization of basic fibroblast growth factor from bovine testis. Mol. Cell. Endocrinol. 49, 189–194. doi: 10.1016/0303-7207(87)90212-7
Valvezan, A. J., and Klein, P. S. (2012). GSK-3 and Wnt Signaling in Neurogenesis and Bipolar Disorder. Front. Mol. Neurosci. 5:1. doi: 10.3389/fnmol.2012.00001
Vannelli, B. G., Barni, T., Orlando, C., Natali, A., Serio, M., and Balboni, G. C. (1988). Insulin-like growth factor-I (IGF-I) and IGF-I receptor in human testis: an immunohistochemical study. Fertil. Steril. 49, 666–669. doi: 10.1016/S0015-0282(16)59837-9
Varjosalo, M., and Taipale, J. (2008). Hedgehog: functions and mechanisms. Genes Dev. 22, 2454–2472. doi: 10.1101/gad.1693608
Vaucher, L., Funaro, M. G., Mehta, A., Mielnik, A., Bolyakov, A., Prossnitz, E. R., et al. (2014). Activation of GPER-1 estradiol receptor downregulates production of testosterone in isolated rat Leydig cells and adult human testis. PLoS ONE 9:e92425. doi: 10.1371/journal.pone.0092425
Verhoeven, G., and Cailleau, J. (1986). Stimulatory effects of epidermal growth factor on steroidogenesis in Leydig cells. Mol. Cell. Endocrinol. 47, 99–106. doi: 10.1016/0303-7207(86)90020-1
Viger, R. S., and Robaire, B. (1995). Steady state steroid 5 a-reductase messenger ribonucleic acid levels and immunocytochemical localization of the type 1 protein in the rat testis during postnatal development. Endocrinology 136, 5409–5415. doi: 10.1210/endo.136.12.7588289
Viger, R. S., Silversides, D. W., and Tremblay, J. J. (2005). New insights into the regulation of mammalian sex determination and male sex differentiation. Vitamins Hormones 70, 387–413. doi: 10.1016/S0083-6729(05)70013-3
Vigier, B., Forest, M. G., Eychenne, B., Bezard, J., Garrigou, O., Robel, P., et al. (1989). Anti-Mullerian hormone produces endocrine sex reversal of fetal ovaries. Proc. Natl. Acad. Sci. U.S.A. 86, 3684–3688. doi: 10.1073/pnas.86.10.3684
Wang, G., and Hardy, M. P. (2004). Development of leydig cells in the insulin-like growth factor-I (igf-I) knockout mouse: effects of igf-I replacement and gonadotropic stimulation. Biol. Reprod. 70, 632–639. doi: 10.1095/biolreprod.103.022590
Wang, G. M., O'Shaughnessy, P. J., Chubb, C., Robaire, B., and Hardy, M. P. (2003). Effects of insulin-like growth factor I on steroidogenic enzyme expression levels in mouse leydig cells. Endocrinology 144, 5058–5064. doi: 10.1210/en.2003-0563
Wang, X. J., Qiao, Y., Xiao, M. M., Wang, L., Chen, J., Lv, W., et al. (2017). Opposing roles of acetylation and phosphorylation in LIFR-dependent self-renewal growth signaling in mouse embryonic stem cells. Cell Rep. 18, 933–946. doi: 10.1016/j.celrep.2016.12.081
Wang, Y., Yuan, K., Li, X., Su, Z., Guan, H., Su, Y., et al. (2016). Leukemia inhibitory factor stimulates steroidogenesis of rat immature Leydig cells via increasing the expression of steroidogenic acute regulatory protein. Growth Factors 34, 1–11. doi: 10.1016/j.jcrysgro.2015.09.031
Warren, D. W., Huhtaniemi, I. T., Tapanainen, J., Dufau, M. L., and Catt, K. J. (1984). Ontogeny of gonadotropin receptors in the fetal and neonatal rat testis. Endocrinology 114, 470–476. doi: 10.1210/endo-114-2-470
Welsh, M., Moffat, L., Belling, K., De Franca, L. R., Segatelli, T. M., Saunders, P. T., et al. (2012). Androgen receptor signalling in peritubular myoid cells is essential for normal differentiation and function of adult Leydig cells. Int. J. Androl. 35, 25–40. doi: 10.1111/j.1365-2605.2011.01150.x
Welsh, M., Saunders, P. T., Atanassova, N., Sharpe, R. M., and Smith, L. B. (2009). Androgen action via testicular peritubular myoid cells is essential for male fertility. FASEB J. 23, 4218–4230. doi: 10.1096/fj.09-138347
Wen, Q., Cheng, C. Y., and Liu, Y. X. (2016). Development, function and fate of fetal Leydig cells. Semin. Cell Dev. Biol. 59, 89–98. doi: 10.1016/j.semcdb.2016.03.003
Werner, R., Merz, H., Birnbaum, W., Marshall, L., Schroder, T., Reiz, B., et al. (2015). 46,XY gonadal dysgenesis due to a homozygous mutation in desert hedgehog (DHH) identified by exome sequencing. J. Clin. Endocrinol. Metab. 100, E1022–1029. doi: 10.1210/jc.2015-1314
Wu, X., Zhang, N., and Lee, M. M. (2012). Mullerian inhibiting substance recruits ALK3 to regulate Leydig cell differentiation. Endocrinology 153, 4929–4937. doi: 10.1210/en.2012-1168
Xi, J., Yang, Z., Zeng, C., Hu, X., and Wang, J. (2012). Suppressive effect of triadimefon, a triazole fungicide, on spatial learning and reference memory in rats. Behav. Pharmacol. 23, 727–734. doi: 10.1097/FBP.0b013e32835a7c63
Xu, Q., Lin, H. Y., Yeh, S. D., Yu, I. C., Wang, R. S., Chen, Y. T., et al. (2007). Infertility with defective spermatogenesis and steroidogenesis in male mice lacking androgen receptor in Leydig cells. Endocrine 32, 96–106. doi: 10.1007/s12020-007-9015-0
Yakar, S., Rosen, C. J., Beamer, W. G., Ackert-Bicknell, C. L., Wu, Y., Liu, J. L., et al. (2002). Circulating levels of IGF-1 directly regulate bone growth and density. J. Clin. Invest. 110, 771–781. doi: 10.1172/JCI0215463
Yang, Y., Li, Z., Wu, X., Chen, H., Xu, W., Xiang, Q., et al. (2017). Direct Reprogramming of Mouse Fibroblasts toward Leydig-like Cells by Defined Factors. Stem Cell Reports 8, 39–53. doi: 10.1016/j.stemcr.2016.11.010
Yao, H. H., Whoriskey, W., and Capel, B. (2002). Desert Hedgehog/Patched 1 signaling specifies fetal Leydig cell fate in testis organogenesis. Genes Develop. 16, 1433–1440. doi: 10.1101/gad.981202
You, X., Li, Y., Wang, X., Xu, J., Zheng, X., and Sui, C. (2017). Residue analysis and risk assessment of tebuconazole in jujube (Ziziphus jujuba Mill). Biomed. Chromatogr. 31:e3917. doi: 10.1002/bmc.3917
Zang, Z. J., Wang, J., Chen, Z., Zhang, Y., Gao, Y., Su, Z., et al. (2017). Transplantation of CD51+ stem Leydig cells: A new strategy for the treatment of testosterone deficiency. Stem Cells 35, 1222–1232. doi: 10.1002/stem.2569
Zhang, F. P., Pakarainen, T., Zhu, F., Poutanen, M., and Huhtaniemi, I. (2004). Molecular characterization of postnatal development of testicular steroidogenesis in luteinizing hormone receptor knockout mice. Endocrinology 145, 1453–1463. doi: 10.1210/en.2003-1049
Zhang, F. P., Poutanen, M., Wilbertz, J., and Huhtaniemi, I. (2001). Normal prenatal but arrested postnatal sexual development of luteinizing hormone receptor knockout (LuRKO)mice. Mol. Endocrinol. 15, 172–183. doi: 10.1210/mend.15.1.0582
Zhang, L., Wang, H., Yang, Y., Liu, H., Zhang, Q., Xiang, Q., et al. (2013). NGF induces adult stem Leydig cells to proliferate and differentiate during Leydig cell regeneration. Biochem. Biophys. Res. Commun. 436, 300–305. doi: 10.1016/j.bbrc.2013.05.098
Zhang, P., and Mellon, S. H. (1997). Multiple orphan nuclear receptors converge to regulate rat P450c17 gene transcription: novel mechanisms for orphan nuclear receptor action. Mol. Endocrinol. 11, 891–904. doi: 10.1210/mend.11.7.9940
Zhao, L., Bakke, M., Krimkevich, Y., Cushman, L. J., Parlow, A. F., Camper, S. A., et al. (2001). Steroidogenic factor 1 (SF1) is essential for pituitary gonadotrope function. Development 128, 147–154.
Zhou, Q., Nie, R., Prins, G. S., Saunders, P. T., Katzenellenbogen, B. S., and Hess, R. A. (2002). Localization of androgen and estrogen receptors in adult male mouse reproductive tract. J. Androl. 23, 870–881.
Keywords: Leydig cells, testosterone, development, steroidogenic factor 1, Desert Hedgehog
Citation: Ye L, Li X, Li L, Chen H and Ge R-S (2017) Insights into the Development of the Adult Leydig Cell Lineage from Stem Leydig Cells. Front. Physiol. 8:430. doi: 10.3389/fphys.2017.00430
Received: 02 February 2017; Accepted: 06 June 2017;
Published: 28 June 2017.
Edited by:
Ravinder Anand-Ivell, University of Nottingham, United KingdomReviewed by:
Ilpo Huhtaniemi, Imperial College London, United KingdomT. Rajendra Kumar, University of Colorado Anschutz Medical Campus, United States
Peter O'Shaughnessy, University of Glasgow, United Kingdom
Luc J. Martin, Université de Moncton, Canada
Copyright © 2017 Ye, Li, Li, Chen and Ge. This is an open-access article distributed under the terms of the Creative Commons Attribution License (CC BY). The use, distribution or reproduction in other forums is permitted, provided the original author(s) or licensor are credited and that the original publication in this journal is cited, in accordance with accepted academic practice. No use, distribution or reproduction is permitted which does not comply with these terms.
*Correspondence: Ren-Shan Ge, cmVuc2hhbl9nZUB3bXUuZWR1LmNu