- Department of Integrative Structural and Computational Biology, The Scripps Research Institute, La Jolla, CA, United States
Nicotinamide nucleotide transhydrogenase (TH) is an enzyme complex in animal mitochondria and bacteria that utilizes the electrochemical proton gradient across membranes to drive the production of NADPH. The enzyme plays an important role in maintaining the redox balance of cells with implications in aging and a number of human diseases. TH exists as a homodimer with each protomer containing a proton-translocating transmembrane domain and two soluble nucleotide binding domains that mediate hydride transfer between NAD(H) and NADP(H). The three-domain architecture of TH is conserved across species but polypeptide composition differs substantially. The complex domain coupling mechanism of TH is not fully understood despite extensive biochemical and structural characterizations. Herein the progress is reviewed, focusing mainly on structural findings from 3D crystallization of isolated soluble domains and more recently of the transmembrane domain and the holo-enzyme from Thermus thermophilus. A structural perspective and impeding challenges in further elucidating the mechanism of TH are discussed.
Introduction
Nicotinamide nucleotide transhydrogenase (TH) is a key enzyme residing in mitochondrial inner membrane and bacterial cytoplasmic membrane that helps to maintain cellular redox balance through the following reaction:
In this reaction, proton motive force across the membrane is utilized to drive hydride transfer from NADH to NADP+, resulting in the generation of NADPH under most physiological conditions (Jackson, 2003). TH is estimated to account for the generation of about 40% of NADPH in E. coli and an even higher percentage in the mitochondria of vertebrates (Sauer et al., 2004; Rydström, 2006a). Mitochondria utilize NADPH to maintain high levels of glutathione, a key component in cellular defense against the accumulation of reactive oxygen species which are implicated in many pathological conditions, such as cancer, diabetes, hypertension, heart disease, Alzheimer's, and Parkinson's diseases, as well as cell death and aging (Rydström, 2006b; Albracht et al., 2011; Gameiro et al., 2013; Ghosh et al., 2014; Lopert and Patel, 2014; Picard et al., 2015; Ho et al., 2017). Animal model and clinical investigations have indicated that some of these conditions can be attributed to the dysfunction or misexpression of TH (Heiker et al., 2013; Nickel et al., 2015; Roucher-Boulez et al., 2016; Leskov et al., 2017; Santos et al., 2017; Scott et al., 2017). Mutations in the enzyme have also been shown in patients with glucocorticoid deficiency syndrome (Meimaridou et al., 2012; Fujisawa et al., 2015; Weinberg-Shukron et al., 2015).
TH is a complex multi-domain protein arranged as a dimer, where each protomer has two soluble domains and a transmembrane domain (Figure 1) (Leung et al., 2015). Extensive biochemical and structural characterizations have essentially established the domain architecture, the catalytic reaction sites as well as the proton translocating pathway as will be discussed in this review. The soluble domains are located in the mitochondrial matrix or prokaryotic cytosol and include a 40 kDa NAD(H)-binding domain (domain I) and a 20 kDa NADP(H)-binding domain (domain III) which together catalyze the hydride transfer reaction. The 40 kDa transmembrane domain (domain II) forms the proton translocation channel. This three-domain architecture is universally conserved, suggesting a common mechanism for function. However, TH topologies and sequences vary substantially among species. For example, human TH is encoded by a single polypeptide chain, whereas TH from E. coli and T. thermophilus are encoded by two and three polypeptide chains, respectively. In E. coli TH, domain I and the first four transmembrane helices (TM 1–4) of domain II are encoded by one gene α, whereas in T. thermophilus, two separate genes (α1 and α2) encode domain I and the first three TM helices (TM 2–4) of domain II; the remaining TM helices (TM 6–14) of domain II together with domain III in both species are encoded by gene β. Of note, domain II differs in the number of TM helices in different species, for example, with 14 for human, 13 for E. coli and 12 for T. thermophilus. To date, most functional and structural characterizations have been performed on TH from lower organisms (Pedersen et al., 2008; Jackson, 2012), and it will be interesting to learn how function is conducted in TH from higher organisms with evolved sequences, and how mutations in human TH cause disease.
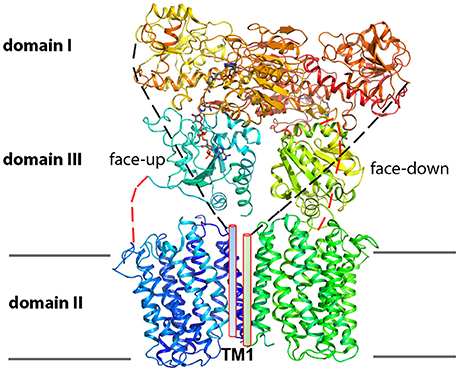
Figure 1. Architecture of holo-TH. TH varies in polypeptide compositions in different species but displays an overall conserved domain architecture. The cartoon is based on a holo-TH crystal structure from T. thermophilus (PDB: 4O9U), shown as a dimer with three domains. Domain I and domain III protrude from membrane-intercalated domain II (membrane boundaries in gray line), with domain III placed in the middle. A linker of 14–19 amino acids (red dashed lines), which is not revealed in 4O9U, connects domain II and III in all TH homologs. Domain I is expressed as a single polypeptide in T. thermophilus, but in mitochondrial TH it is fused with domain II through a linker (black dashed lines) and an additional TM1 (rod).
Mechanistic understanding of TH has been advanced significantly by the structure determinations of isolated soluble domains and more recently of the transmembrane domain and the holo-enzyme from T. thermophilus (Leung et al., 2015; Padayatti et al., 2017). The architecture of the three domains of TH is explicitly established with the NADP(H)-binding domain III sandwiched between domains I and II and showing significant conformational mobility. A novel mechanism of domain III swiveling has been proposed for its communication with each of the other two domains alternately within the biological dimer (Jackson et al., 2015). This manuscript will review progress in determining TH structure, new mechanistic insights that have resulted, and some questions that remain in order to fully elucidate the conformational dynamics and mechanism by which proton translocation is coupled to hydride transfer events that occur remotely.
Structures of Nucleotide Binding Domains I and III
Individual crystal structures of the two soluble domains of TH showed overall similarity across multiple species including human, bovine, E. coli, and R. rubrum (Prasad et al., 1999, 2002; White et al., 2000; Sundaresan et al., 2003; Johansson et al., 2005). Domain I is present as a dimer in solution and always crystallized as such, with the dimeric interface stabilized by a swapping beta-hairpin structure and two helices. Each monomer of domain I has two subdomains with the characteristic nucleotide binding Rossmann fold (Rossmann et al., 1974); yet only one of the subdomains actually binds NAD(H). Thus, in the domain I dimer that adopts a two-fold axis symmetry, the two NAD(H) binding sites are situated on opposite sides. In comparison, domain III is about half the size of domain I, also adopts the Rossmann fold for NADP(H) binding, but only exists as a monomer in solution. Of note, the orientation of NADP(H) in the binding pocket of domain III is flipped relative to that of NAD(H) in domain I.
Local conformational changes are observed in nucleotide-binding regions in domain I and domain III. Various modes of NAD(H) binding such as open, intermediate, and closed forms have been demonstrated, both in domain I structures and the apo structures (Prasad et al., 2002; Johansson et al., 2005). In E. coli TH holoenzyme, the dissociation constant (Kd) for NAD+ is 100–500 μM and that for NADH is 50 μM, comparable to values for the isolated domain I (Bizouarn et al., 2005). Domain III has hitherto only been crystallized in the presence of NADP(H), with loop D either in open or closed conformation (Prasad et al., 1999; White et al., 2000; Sundaresan et al., 2003). Notably, NADP(H) has extremely high binding affinity with the isolated domain III (apparent Kd value < nM) (Diggle et al., 1995; Fjellstrom et al., 1997; Peake et al., 1999), which is partly attributed to adjacent loop E folding over NADP(H) like a lid. Interestingly, for domain III in the intact E. coli TH, the binding affinity of NADP(H) is orders of magnitude weaker: the Kd values are 16 μM for NADP+ and 0.87 μM for NADPH (Bizouarn et al., 2005). It is not understood why the binding affinity of NADP(H) differs so widely between isolated domain III and the intact TH, but existing data suggest that the NADP(H)-binding site is affected by protein-protein interactions between domain III and other domains. For completion of the TH catalytic cycle, all nucleotide reactants must bind and products must dissociate from the binding sites. The holoenzyme likely uses protein conformational changes to alter nucleotide binding affinities and move the reaction (Jackson, 2012).
Structures of Domain I and Domain III in Complex
Co-crystallization of isolated domains I and III either from R. rubrum or T. thermophilus consistently yields a heterotrimeric complex at a 2:1 ratio (Figure 2A) (Cotton et al., 2001; Mather et al., 2004; Sundaresan et al., 2005; Bhakta et al., 2007; Leung et al., 2015). This stoichiometry agrees with findings in solution (Venning et al., 1998, 2001). The Kd of domain III binding to the domain I dimer is < 60 nM (Venning et al., 2001), while a second domain III binds with significantly lower affinity (Kd about 10−5 M) (Quirk et al., 1999). In the heterotrimeric structures, the two domain I components adopt the same two-fold axis as in the isolated domain I dimer structure, with similar protein fold and contact regions between the polypeptides; in addition a domain III is placed underneath one domain I subunit with the NADP(H) binding site face up. However, using the same two-fold axis to model a second “face-up” domain III into the void space under the other domain I subunit results in a steric clash between the two domain III subunits. This leaves a question of how the second domain III is made to fit in the dimeric structure of the holo-TH.
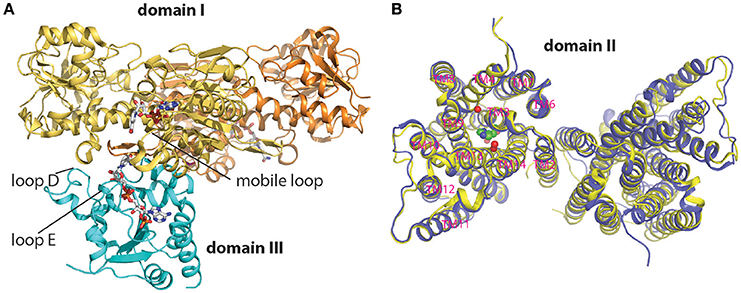
Figure 2. Reaction catalysis and proton-translocating sites in TH. (A) Hydride transfer between the proximate NAD(H) and NADP(H) binding sites of domains I and III. Shown is a nucleotide-bound (sticks), trimeric (domain I)2:(domain III) structure of TH from T. thermophilus (PDB: 4J16), with the NADP(H) binding site in domain III (cyan) facing up. Flexible loops involved in ligand binding are indicated. (B) Proton translocation in domain II. Domain II dimerization (from T. thermophilus) is mediated by TM2-TM2 interaction. Displacement of the second subunit is observed by the alignment of the two structures (PDB: 5UNI, blue; 4O93, yellow) solved under different pH conditions. Crystallographically solved water molecules (red balls) and the channel gating histidine residue (His42α2 on TM3, green sphere) are shown inside the proton channel within one subunit of 5UNI. Structures shown represent a view from the cytoplasmic side.
The heterotrimeric structures of domain I and domain III reveal proximity between the NAD(H) and NADP(H) binding sites (Figure 2A). In these structures, the single copy of domain III is inserted into the NAD(H)-binding cleft of one of the domain I subunits, and the flipped orientation of NADP(H) in domain III apparently facilitates a direct hydride transfer between the nicotinamide rings of NAD(H) and NADP(H) when the two ligands get close. Local conformational changes in the NAD(H) binding site as well as relative movement between the two subdomains within domain I appear to cause a “distal-to-proximal” motion of bound NAD(H) (Mather et al., 2004).
Structures of Transmembrane Domain II
Structural determination of the transmembrane domain of TH has recently been achieved by the use of lipidic cubic phase (LCP) crystallization (Leung et al., 2015; Padayatti et al., 2017). The LCP is a viscous bilayer matrix that is thought to better stabilize membrane proteins than detergents and more frequently results in a tighter and layered crystal packing (Caffrey and Cherezov, 2009). LCP crystallization succeeded for a truncated T. thermophilus TH domain II construct (containing two hydrophobic polypeptide chains: subunit α2 and a truncated version of subunit β from which the soluble domain III was removed from the C-terminus), using 1-(8Z-pentadecenoyl)-rac-glycerol (MAG 8.7) as the host lipid. Compared to the commonly used monoolein (MAG 9.9), MAG 8.7 has a shorter alkyl chain and tends to form a more fluidic cubic phase.
Domain II was crystallized at pH 8.5 and 6.5, respectively, and the solved crystal structures both appear as a dimer with TM2-TM2 association at the interface (Figure 2B). The two structures are quite similar overall, although alignment shows a slight displacement of the second domain II monomer, suggesting a degree of conformational flexibility to the association; yet at this stage no evidence is found for a substantial conformational change related to channel opening or closure. The crystal structures also reveal a putative channel surrounded by six TM helices (TM 3, 4, 9, 10, 13, and 14). It should be noted that these channel-forming helices and the TM2 at the dimeric interface are highly conserved among species, suggesting a common proton translocation pathway and possibly a similar mode of dimerization despite considerable homolog variation in the organization of this domain II (e.g., the presence of extra TM1 and TM5 in human TH).
The pH 6.5 crystal structure solved at a resolution of 2.1 Å revealed water molecules inside the TM helix bundles, which, in combination with molecular dynamics (MD) simulations, allows a clearer interpretation of the proton translocation channel and its key functional residues (Figure 2B) (Padayatti et al., 2017). A cluster of polar residues including a central histidine (His42α2) form an extended H-bonded network together with two bound water molecules in the cytoplasmic half of the channel, and this connection likely provides a proton conduit to the cytoplasmic side. A central role for His42α2 in proton translocation by TH is consistent with extensive biochemical characterizations; the central histidine is conserved in other TH species although its position may shift (Olausson et al., 1995; Rydström et al., 1998; Bragg and Hou, 2001; Yamaguchi et al., 2002). MD simulation showed that a water conduit is formed only when His42α2 is protonated. Gating of the isolated channel appears to be controlled by His42α2 protonation and also a distinct hydrophobic barrier region spanning about 7.5 Å below His42α2 in the middle of the channel. The presence of this long stretch of hydrophobic region for proton gating is remarkable for TH, in contrast to the water penetration through thin hydrophobic layers known for some other proton channels (Pielak and Chou, 2011; Takeshita et al., 2014; Thomaston et al., 2017). During MD simulations, a transient water wire is formed connecting His42α2 and Glu221β through the hydrophobic barrier, and the presence of the water wire coincides with a conformational change of Thr214β side chain located slightly below His42α2. Mutation of Thr214β to Ala causes >90% loss of channel-coupled enzymatic activity, biochemically supporting the proposed role of Thr214β in proton translocation (Padayatti et al., 2017).
The domain II structure further shows formation of a salt bridge between Asp202β on the cytosolic end of TM13 and Arg254β at the start of the linker region connecting domain II and domain III. This linkage is positioned on the membrane surface adjacent to the entrance of the proton channel. The Asp-Arg salt bridge at equivalent positions in E. coli TH (Asp213β and Arg265β) has been postulated and implicated as important for both proton translocation in domain II and NADP(H) binding in domain III (Althage et al., 2001; Bragg and Hou, 2001; Yamaguchi et al., 2002). Since in TH of various species the sequence of domain II always exists in conjunction with domain III (Figure 1), regulation of domain III movement may be directly linked to domain II and the proton channel activity, and vice versa.
Holo-TH Structures
A holo-TH structure from T. thermophilus has recently been solved in detergent micelles, albeit only at 6.93 Å resolution (Figure 1) (Leung et al., 2015). This low resolution structure seemingly reflects substantial conformational flexibility of the complex protein and the significant challenge in identifying suitable crystallization conditions. The soluble domain I dimer and membrane-intercalated domain II components in the holo-TH are roughly aligned with individually obtained domain structures. A remarkable realization from this structure is the asymmetric orientation of two domain III subunits, one being flipped ~180°C relative to the other. This distinct domain III arrangement in the dimeric TH brings one NADP(H) binding site close to the NAD(H) binding site of domain I, as shown in the heterotrimeric (domain I)2-domain III structures, while the other NADP(H) binding site faces down to approach the channel surface of domain II. The two orientations of domain III are supported by disulfide cross-linking of inserted cysteine pairs on separate domains (Leung et al., 2015). The flipped orientation of domain III also appears to coincide with that observed in the crystal packing of (domain I)2-domain III structures where a crystallographic symmetry mate of domain III is similarly facing down (Sundaresan et al., 2005; Bhakta et al., 2007). Consistent with crystal structures, cryogenic electron microscopy (EM) images (~18 Å) of the holo-TH confirm an asymmetric organization of TH dimers in non-crystalline conditions (Leung et al., 2015). Cryo-EM structures also suggest that one subunit of domain III is more disordered than the other as evidenced by much weaker electron density. While a further high-resolution structure of holo-TH is warranted, based on current structural findings, the two copies of domain III have been proposed to play distinct functional roles in coupling to proton translocation in domain II and in hydride transfer from/to domain I (Jackson et al., 2015).
Perspective and Concluding Remarks
The mechanistic understanding of mitochondria TH has benefited from extensive structural and biochemical characterizations of isolated domains and homolog proteins, particularly those from lower organisms. The use of stably expressed and purified TH from T. thermophilus has resulted in the recent structural determinations of the membrane-intercalated domain II and holo-TH. For the latter, higher-resolution structural determinations of the large multidomain membrane protein complex remain a significant challenge due to its significant conformational flexibility/heterogeneity. The solved holo-TH structure in detergents is of low resolution and lacks information for the linker (14–19 amino acids present in all TH) that connects domain II and domain III, and which is supposedly important to mediate the two domain interactions and the rotation of domain III as well. Modeling of human TH structure based on homologs is challenging given the significant sequence disparity, especially for domain II because of the presence of extra TM segments in the human enzyme (Metherell et al., 2016). The fusion of all three domains in a single polypeptide chain in human TH may impact its conformational landscape and energetics as well, although a common mechanism of the TH-catalyzed enzymatic reactions is expected for different species. At present, our understanding of the dynamic domain coupling and movements in TH is still very limited and mostly hypothetical. Structural insights into the mechanism of a dynamic TH will thus require the structural determinations of the protein complex in many conformational states. Recent technological breakthroughs in X-ray crystallography and cryo-EM have helped solve structures of many difficult-to-study systems. The use of novel lipid bilayer mimetics such as LCP (Caffrey, 2015) and nanodiscs (Denisov and Sligar, 2017) in the structural determinations of membrane proteins has gained popularity in addition to the traditional use of detergents. With the application and integration of these advanced techniques with new tools in TH studies, we anticipate that a comprehensive and detailed structural and mechanistic understanding can be achieved for this fundamentally important enzyme in many kingdoms of life.
Author Contributions
All authors listed have made a substantial, direct and intellectual contribution to the work, and approved it for publication.
Funding
This work was supported by the National Institutes of Health (5R01GM103838).
Conflict of Interest Statement
The authors declare that the research was conducted in the absence of any commercial or financial relationships that could be construed as a potential conflict of interest.
References
Albracht, S. P., Meijer, A. J., and Rydstrom, J. (2011). Mammalian NADH:ubiquinone oxidoreductase (Complex I) and nicotinamide nucleotide transhydrogenase (Nnt) together regulate the mitochondrial production of H(2)O(2)–implications for their role in disease, especially cancer. J. Bioenerg. Biomembr. 43, 541–564. doi: 10.1007/s10863-011-9381-4
Althage, M., Bizouarn, T., and Rydström, J. (2001). Identification of a region involved in the communication between the NADP(H) binding domain and the membrane domain in proton pumping E-coli transhydrogenase. Biochemistry 40, 9968–9976. doi: 10.1021/bi0103157
Bhakta, T., Whitehead, S. J., Snaith, J. S., Dafforn, T. R., Wilkie, J., Rajesh, S., et al. (2007). Structures of the dI2dIII1 complex of proton-translocating transhydrogenase with bound, inactive analogues of NADH and NADPH reveal active site geometries. Biochemistry 46, 3304–3318. doi: 10.1021/bi061843r
Bizouarn, T., van Boxel, G. I., Bhakta, T., and Jackson, J. B. (2005). Nucleotide binding affinities of the intact proton-translocating transhydrogenase from Escherichia coli. Biochim. Biophys. Acta 1708, 404–410. doi: 10.1016/j.bbabio.2005.04.004
Bragg, P. D., and Hou, C. (2001). Characterization of mutants of beta histidine91, beta aspartate213, and beta asparagine222, possible components of the energy transduction pathway of the proton-translocating pyridine nucleotide transhydrogenase of Escherichia coli. Arch. Biochem. Biophys. 388, 299–307. doi: 10.1006/abbi.2001.2298
Caffrey, M. (2015). A comprehensive review of the lipid cubic phase or in meso method for crystallizing membrane and soluble proteins and complexes. Acta Crystallogr. F Struct. Biol. Commun. 71(Pt 1), 3–18. doi: 10.1107/S2053230X14026843
Caffrey, M., and Cherezov, V. (2009). Crystallizing membrane proteins using lipidic mesophases. Nat. Protoc. 4, 706–731. doi: 10.1038/nprot.2009.31
Cotton, N. P., White, S. A., Peake, S. J., McSweeney, S., and Jackson, J. B. (2001). The crystal structure of an asymmetric complex of the two nucleotide binding components of proton-translocating transhydrogenase. Structure 9, 165–176. doi: 10.1016/S0969-2126(01)00571-8
Denisov, I. G., and Sligar, S. G. (2017). Nanodiscs in membrane biochemistry and biophysics. Chem. Rev. 117, 4669–4713. doi: 10.1021/acs.chemrev.6b00690
Diggle, C., Hutton, M., Jones, G. R., Thomas, C. M., and Jackson, J. B. (1995). Properties of the soluble polypeptide of the proton-translocating transhydrogenase from rhodospirillum-rubrum obtained by expression in Escherichia-Coli. Eur. J. Biochem. 228, 719–726. doi: 10.1111/j.1432-1033.1995.tb20315.x
Fjellström, O., Johansson, C., and Rydström, J. (1997). Structural and catalytic properties of the expressed and purified NAD(H)- and NADP(H)-binding domains of proton-pumping transhydrogenase from Escherichia coli. Biochemistry 36, 11331–11341. doi: 10.1021/bi970958f
Fujisawa, Y., Napoli, E., Wong, S., Song, G., Yamaguchi, R., Matsui, T., et al. (2015). Impact of a novel homozygous mutation in nicotinamide nucleotide transhydrogenase on mitochondrial DNA integrity in a case of familial glucocorticoid deficiency. BBA Clin. 3, 70–78. doi: 10.1016/j.bbacli.2014.12.003
Gameiro, P. A., Laviolette, L. A., Kelleher, J. K., Iliopoulos, O., and Stephanopoulos, G. (2013). Cofactor balance by nicotinamide nucleotide transhydrogenase (NNT) coordinates reductive carboxylation and glucose catabolism in the tricarboxylic acid (TCA) cycle. J. Biol. Chem. 288, 12967–12977. doi: 10.1074/jbc.M112.396796
Ghosh, D., Levault, K. R., and Brewer, G. J. (2014). Relative importance of redox buffers GSH and NAD(P)H in age-related neurodegeneration and Alzheimer disease-like mouse neurons. Aging Cell 13, 631–640. doi: 10.1111/acel.12216
Heiker, J. T., Kern, M., Kosacka, J., Flehmig, G., Stumvoll, M., Shang, E., et al. (2013). Nicotinamide nucleotide transhydrogenase mRNA expression is related to human obesity. Obesity 21, 529–534. doi: 10.1002/oby.20095
Ho, H. Y., Lin, Y. T., Lin, G., Wu, P. R., and Cheng, M. L. (2017). Nicotinamide nucleotide transhydrogenase (NNT) deficiency dysregulates mitochondrial retrograde signaling and impedes proliferation. Redox Biol. 12, 916–928. doi: 10.1016/j.redox.2017.04.035
Jackson, J. B. (2003). Proton translocation by transhydrogenase. FEBS Lett. 545:7. doi: 10.1016/S0014-5793(03)00224-2
Jackson, J. B. (2012). A review of the binding-change mechanism for proton-translocating transhydrogenase. Biochim. Biophys. Acta 1817, 1839–1846. doi: 10.1016/j.bbabio.2012.04.006
Jackson, J. B., Leung, J. H., Stout, C. D., Schurig-Briccio, L. A., and Gennis, R. B. (2015). Review and Hypothesis. New insights into the reaction mechanism of transhydrogenase: swivelling the dIII component may gate the proton channel. FEBS Lett. 589, 2027–2033. doi: 10.1016/j.febslet.2015.06.027
Johansson, T., Oswald, C., Pedersen, A., Tornroth, S., Okvist, M., Karlsson, B. G., et al. (2005). X-ray structure of domain I of the proton-pumping membrane protein transhydrogenase from Escherichia coli. J. Mol. Biol. 352, 299–312. doi: 10.1016/j.jmb.2005.07.022
Leskov, I., Neville, A., Shen, X., Pardue, S., Kevil, C. G., Granger, D. N., et al. (2017). Nicotinamide nucleotide transhydrogenase activity impacts mitochondrial redox balance and the development of hypertension in mice. J. Am. Soc. Hypertens. 11, 110–121. doi: 10.1016/j.jash.2016.12.002
Leung, J. H., Schurig-Briccio, L. A., Yamaguchi, M., Moeller, A., Speir, J. A., Gennis, R. B., et al. (2015). Division of labor in transhydrogenase by alternating proton translocation and hydride transfer. Science 347, 178–181. doi: 10.1126/science.1260451
Lopert, P., and Patel, M. (2014). Nicotinamide nucleotide transhydrogenase (Nnt) links the substrate requirement in brain mitochondria for hydrogen peroxide removal to the thioredoxin/peroxiredoxin (Trx/Prx) system. J. Biol. Chem. 289, 15611–15620. doi: 10.1074/jbc.M113.533653
Mather, O. C., Singh, A., van Boxel, G. I., White, S. A., and Jackson, J. B. (2004). Active-site conformational changes associated with hydride transfer in proton-translocating transhydrogenase. Biochemistry 43, 10952–10964. doi: 10.1021/bi0497594
Meimaridou, E., Kowalczyk, J., Guasti, L., Hughes, C. R., Wagner, F., Frommolt, P., et al. (2012). Mutations in NNT encoding nicotinamide nucleotide transhydrogenase cause familial glucocorticoid deficiency. Nat. Genet. 44, 740–742. doi: 10.1038/ng.2299
Metherell, L. A., Guerra-Assunção, J. A., Sternberg, M. J., and David, A. (2016). Three-dimensional model of human nicotinamide nucleotide transhydrogenase (NNT) and sequence-structure analysis of its disease-causing variations. Hum. Mutat. 37, 1074–1084. doi: 10.1002/humu.23046
Nickel, A. G., von Hardenberg, A., Hohl, M., Loffler, J. R., Kohlhaas, M., Becker, J., et al. (2015). Reversal of mitochondrial transhydrogenase causes oxidative stress in heart failure. Cell Metab. 22, 472–484. doi: 10.1016/j.cmet.2015.07.008
Olausson, T., Fjellström, O., Meuller, J., and Rydström, J. (1995). Molecular biology of nicotinamide nucleotide transhydrogenase–a unique proton pump. Biochim. Biophys. Acta 1231, 1–19. doi: 10.1016/0005-2728(95)00058-Q
Padayatti, P. S., Leung, J. H., Mahinthichaichan, P., Tajkhorshid, E., Ishchenko, A., Cherezov, V., et al. (2017). Critical role of water molecules in proton translocation by the membrane-bound transhydrogenase. Structure 25, 1111–1119.e3. doi: 10.1016/j.str.2017.05.022
Peake, S. J., Venning, J. D., and Jackson, J. B. (1999). A catalytically active complex formed from the recombinant dI protein of Rhodospirillum rubrum transhydrogenase, and the recombinant dIII protein of the human enzyme. Biochim. Biophys. Acta 1411, 159–169. doi: 10.1016/S0005-2728(99)00013-4
Pedersen, A., Karlsson, G. B., and Rydström, J. (2008). Proton-translocating transhydrogenase: an update of unsolved and controversial issues. J. Bioenerg. Biomembr. 40, 463–473. doi: 10.1007/s10863-008-9170-x
Picard, M., McManus, M. J., Gray, J. D., Nasca, C., Moffat, C., Kopinski, P. K., et al. (2015). Mitochondrial functions modulate neuroendocrine, metabolic, inflammatory, and transcriptional responses to acute psychological stress. Proc. Natl. Acad. Sci. U.S.A. 112, E6614–E6623. doi: 10.1073/pnas.1515733112
Pielak, R. M., and Chou, J. J. (2011). Influenza M2 proton channels. Biochim. Biophys. Acta 1808, 522–529. doi: 10.1016/j.bbamem.2010.04.015
Prasad, G. S., Sridhar, V., Yamaguchi, M., Hatefi, Y., and Stout, C. D. (1999). Crystal structure of transhydrogenase domain III at 1.2 A resolution. Nat. Struct. Biol. 6, 1126–1131. doi: 10.1038/70067
Prasad, G. S., Wahlberg, M., Sridhar, V., Sundaresan, V., Yamaguchi, M., Hatefi, Y., et al. (2002). Crystal structures of transhydrogenase domain I with and without bound NADH. Biochemistry 41, 12745–12754. doi: 10.1021/bi020251f
Quirk, P. G., Jeeves, M., Cotton, N. P., Smith, J. K., and Jackson, B. J. (1999). Structural changes in the recombinant, NADP(H)-binding component of proton translocating transhydrogenase revealed by NMR spectroscopy. FEBS Lett. 446, 127–132. doi: 10.1016/S0014-5793(99)00198-2
Rossmann, M. G., Moras, D., and Olsen, K. W. (1974). Chemical and biological evolution of nucleotide-binding protein. Nature 250, 194–199. doi: 10.1038/250194a0
Roucher-Boulez, F., Mallet-Motak, D., Samara-Boustani, D., Jilani, H., Ladjouze, A., Souchon, P. F., et al. (2016). NNT mutations: a cause of primary adrenal insufficiency, oxidative stress and extra-adrenal defects. Eur. J. Endocrinol. 175, 73–84. doi: 10.1530/EJE-16-0056
Rydström, J. (2006a). Mitochondrial NADPH, transhydrogenase and disease. Biochim. Biophys. Acta 1757, 721–726. doi: 10.1016/j.bbabio.2006.03.010
Rydström, J. (2006b). Mitochondrial transhydrogenase - a key enzyme in insulin secretion and, potentially, diabetes. Trends Biochem. Sci. 31, 355–358. doi: 10.1016/j.tibs.2006.05.003
Rydström, J., Hu, X., Fjellstrom, O., Meuller, J., Zhang, J., Johansson, C., et al. (1998). Domains, specific residues and conformational states involved in hydride ion transfer and proton pumping by nicotinamide nucleotide transhydrogenase from Escherichia coli. Biochim. Biophys. Acta 1365, 10–16. doi: 10.1016/S0005-2728(98)00038-3
Santos, L. R. B., Muller, C., de Souza, A. H., Takahashi, H. K., Spégel, P., Sweet, I. R., et al. (2017). NNT reverse mode of operation mediates glucose control of mitochondrial NADPH and glutathione redox state in mouse pancreatic beta-cells. Mol. Metab. 6, 535–547. doi: 10.1016/j.molmet.2017.04.004
Sauer, U., Canonaco, F., Heri, S., Perrenoud, A., and Fischer, E. (2004). The soluble and membrane-bound transhydrogenases UdhA and PntAB have divergent functions in NADPH metabolism of Escherichia coli. J. Biol. Chem. 279, 6613–6619. doi: 10.1074/jbc.M311657200
Scott, R., Van Vliet, G., and Deladoëy, J. (2017). Association of adrenal insufficiency with insulin-dependent diabetes mellitus in a patient with inactivating mutations in nicotinamide nucleotide transhydrogenase: a phenocopy of the animal model. Eur. J. Endocrinol. 176, C1–C2. doi: 10.1530/EJE-16-0970
Sundaresan, V., Chartron, J., Yamaguchi, M., and Stout, C. D. (2005). Conformational diversity in NAD(H) and interacting transhydrogenase nicotinamide nucleotide binding domains. J. Mol. Biol. 346, 617–629. doi: 10.1016/j.jmb.2004.11.070
Sundaresan, V., Yamaguchi, M., Chartron, J., and Stout, C. D. (2003). Conformational change in the NADP(H) binding domain of transhydrogenase defines four states. Biochemistry 42, 12143–12153. doi: 10.1021/bi035006q
Takeshita, K., Sakata, S., Yamashita, E., Fujiwara, Y., Kawanabe, A., Kurokawa, T., et al. (2014). X-ray crystal structure of voltage-gated proton channel. Nat. Struct. Mol. Biol. 21, 352–357. doi: 10.1038/nsmb.2783
Thomaston, J. L., Woldeyes, R. A., Nakane, T., Yamashita, A., Tanaka, T., Koiwai, K., et al. (2017). XFEL structures of the influenza M2 proton channel: room temperature water networks and insights into proton conduction. Proc. Natl. Acad. Sci. U.S.A. doi: 10.1073/pnas.1705624114. [Epub ahead of print].
Venning, J. D., Bizouarn, T., Cotton, N. P. J., Quirk, P. G., and Jackson, J. B. (1998). Stopped-flow kinetics of hydride transfer between nucleotides by recombinant domains of proton-translocating transhydrogenase. Eur. J. Biochem. 257, 202–209. doi: 10.1046/j.1432-1327.1998.2570202.x
Venning, J. D., Rodrigues, D. J., Weston, C. J., Cotton, N. P., Quirk, P. G., Errington, N., et al. (2001). The heterotrimer of the membrane-peripheral components of transhydrogenase and the alternating-site mechanism of proton translocation. J. Biol. Chem. 276, 30678–30685. doi: 10.1074/jbc.M104429200
Weinberg-Shukron, A., Abu-Libdeh, A., Zhadeh, F., Carmel, L., Kogot-Levin, A., Kamal, L., et al. (2015). Combined mineralocorticoid and glucocorticoid deficiency is caused by a novel founder nicotinamide nucleotide transhydrogenase mutation that alters mitochondrial morphology and increases oxidative stress. J. Med. Genet. 52, 636–641. doi: 10.1136/jmedgenet-2015-103078
White, S. A., Peake, S. J., McSweeney, S., Leonard, G., Cotton, N. P., and Jackson, J. B. (2000). The high-resolution structure of the NADP(H)-binding component (dIII) of proton-translocating transhydrogenase from human heart mitochondria. Structure 8, 1–12. doi: 10.1016/S0969-2126(00)00075-7
Keywords: hydride transfer, lipidic cubic phase, membrane protein, nucleotide binding, NADPH, proton channel, transhydrogenase, X-ray crystallography
Citation: Zhang Q, Padayatti PS and Leung JH (2017) Proton-Translocating Nicotinamide Nucleotide Transhydrogenase: A Structural Perspective. Front. Physiol. 8:1089. doi: 10.3389/fphys.2017.01089
Received: 07 July 2017; Accepted: 11 December 2017;
Published: 19 December 2017.
Edited by:
Mario Diaz, Universidad de La Laguna, SpainReviewed by:
Luis Gonzalo Cuello, Texas Tech University Health Sciences Center, United StatesIan Kerr, University of Nottingham, United Kingdom
Copyright © 2017 Zhang, Padayatti and Leung. This is an open-access article distributed under the terms of the Creative Commons Attribution License (CC BY). The use, distribution or reproduction in other forums is permitted, provided the original author(s) or licensor are credited and that the original publication in this journal is cited, in accordance with accepted academic practice. No use, distribution or reproduction is permitted which does not comply with these terms.
*Correspondence: Qinghai Zhang, cWluZ2hhaUBzY3JpcHBzLmVkdQ==