- 1Institute of Biosciences and Bioresources, National Research Council (CNR), Naples, Italy
- 2Department of Biomedical Sciences, University of North Dakota, Grand Forks, ND, United States
- 3Brain Institute, Florida Atlantic University, Jupiter, FL, United States
- 4Institute of Genetics and Biophysics, National Research Council (CNR), Naples, Italy
- 5Harriet L. Wilkes Honors College, Florida Atlantic University, Jupiter, FL, United States
The dopamine transporter (DAT) is a cell membrane protein whose main function is to reuptake the dopamine (DA) released in the synaptic cleft back into the dopaminergic neurons. Previous studies suggested that the activity of DAT is regulated by allosteric proteins such as Syntaxin-1A and is altered by drugs of abuse such as amphetamine (Amph). Because Caenorhabditis elegans expresses both DAT (DAT-1) and Syntaxin-1A (UNC-64), we used this model system to investigate the functional and behavioral effects caused by lack of expression of unc-64 in cultured dopaminergic neurons and in living animals. Using an inheritable RNA silencing technique, we were able to knockdown unc-64 specifically in the dopaminergic neurons. This cell-specific knockdown approach avoids the pleiotropic phenotypes caused by knockout mutations of unc-64 and ensures the transmission of dopaminergic specific unc-64 silencing to the progeny. We found that, similarly to dat-1 knockouts and dat-1 silenced lines, animals with reduced unc-64 expression in the dopaminergic neurons did not respond to Amph treatment when tested for locomotor behaviors. Our in vitro data demonstrated that in neuronal cultures derived from animals silenced for unc-64, the DA uptake was reduced by 30% when compared to controls, and this reduction was similar to that measured in neurons isolated from animals silenced for dat-1 (40%). Moreover, reduced expression of unc-64 in the dopaminergic neurons significantly reduced the DA release elicited by Amph. Because in C. elegans DAT-1 is the only protein capable to reuptake DA, these data show that reduced expression of unc-64 in the dopaminergic neurons decreases the capability of DAT in re-accumulating synaptic DA. Moreover, these results demonstrate that decreased expression of unc-64 in the dopaminergic neurons abrogates the locomotor behavior induced by Amph. Taken together these data suggest that Syntaxin-1A plays an important role in both functional and behavioral effects caused by Amph.
Introduction
The DAT is a plasma membrane protein which reuptakes the DA released in the synaptic cleft back into the neurons. By so doing, DAT plays a central role in controlling the extracellular content of DA and regulating the amplitude of the dopaminergic signaling. DAT has been involved in the etiology and treatment of various neurologic disorders including schizophrenia, attention deficit hyperactivity disorder (ADHD) and Parkinson’s disease (Miller et al., 1999; Wang et al., 2013; Markota et al., 2014). Interestingly, DAT is also one of the major targets for psychostimulants such as cocaine and Amph; and while cocaine is a DAT blocker, Amph is also a DAT substrate, thus preventing DA uptake, and a releaser causing DA release by inducing reverse transport of DA from inside to outside the neurons through DAT (Khoshbouei et al., 2003). The resulting increase of extracellular DA is believed to be the first step that ultimately generates the behavioral outcomes produced by Amph.
Previous data showed that the regulatory effects of Amph on DAT require the participation of ancillary proteins such as Syntaxin-1A (Binda et al., 2008). Syntaxin-1A is a member of the SNARE (soluble N-ethylmaleimide-sensitive factor attachment protein receptor) proteins complex involved in the process of membrane vesicle fusion which leads to exocytosis and thus neurotransmitter release (Salaün et al., 2004). The neuronal specific isoform can bind to and regulate different plasma membrane proteins including ion channels (Naren et al., 1998; Arien et al., 2003; Condliffe et al., 2004; Tsuk et al., 2004) and neurotransmitter transporters (Deken et al., 2000; Geerlings et al., 2001; Haase et al., 2001; Horton and Quick, 2001; Quick, 2003, 2006; Sung et al., 2003; Wang et al., 2003; Fan et al., 2006). Studies focused on the DAT/ Syntaxin-1A interaction have showed that Syntaxin-1A directly binds at the N-terminal domain of DAT (Lee et al., 2004), reduces the capability of DAT to reuptake DA (Cervinski et al., 2010) and increases the ability of Amph to cause DA efflux (Binda et al., 2008). However, few studies have been performed to assess whether the interaction and/or lack of interaction between Syntaxin-1A and DAT may cause behavioral outcomes in living animals (Carvelli et al., 2008; Cartier et al., 2015).
Previously, we showed that, like in mammals, the C. elegans Syntaxin-1A homolog UNC-64 interacts with the C. elegans DAT (DAT-1) and regulates the electrogenic properties of DAT-1 (Carvelli et al., 2008). Here we investigated the effects caused by reduced expression of the unc-64 gene in the dopaminergic neurons on DAT-1 function and DA-mediated behaviors. Because the unc-64 knockout animals die shortly after embryogenesis (van Swinderen et al., 2001), we created transgenic animals expressing heritable and cell-specific knockdown of the unc-64 gene in the dopaminergic neurons (pdat-1::unc-64 sas). We found that the pdat-1::unc-64 sas animals show a slight but statistically significant reduction in body bends when tested in plates without bacteria but they swim normally. However, when treated with Amph, the percentage of animals exhibiting SWIP was significantly reduced with respect to wild type animals. These behavioral results were supported by in vitro data showing that cultured neurons isolated from pdat-1::unc-64 sas animals exhibit reduced DA uptake and reduced DA release induced by Amph. Taken together these data suggest that Syntaxin-1A is required to mediate the physiological and behavioral effects caused by Amph in C. elegans.
Materials and Methods
Worms Husbandry and Strains
All C. elegans strains were cultivated under standard conditions, in non-crowded conditions, at 20°C on NGM plates seeded with the OP50 or NA22 Escherichia coli strains (Brenner, 1974), except for worms that were grown on E. coli strain HB101. The N2 wild type (Bristol variety), RM2702 dat-1(ok157) III, CB1112 cat-2(e1112) II, OH7547 otIs199 [pcat-2::GFP; prgef-1::dsRed; rol-6 (su1006)], and CB246 unc-64(e246) III strains were provided by the Caenorhabditis Genetics Center (CGC, University of Minnesota, United States). BY200 vtIs1 [pdat-1::GFP; rol-6(su1006)] V strain was obtained from Dr. Randy Blakely at the Brain Institute, Florida Atlantic University. To knockdown GFP encoding gene (gfp) in the dopaminergic neurons under the promoter of cat-2 (pcat-2), the following transgene was used: gbEx525 [GBF312 pcat-2::gfp RNAi sas; podr-1::RFP]; whereas, under the promoter of dat-1 (pdat-1) we used: gbEx572 [GBF326 pdat-1::gfp RNAi sas; pJM371 pelt-2::NLS::RFP]. To knockdown the dat-1 gene under pdat-1, the following transgenes were used: gbEx584 [GBF334 pdat-1::dat-1 RNAi sas; podr-1::RFP; EM282 pcat-2::GFP] and gbEx624 [GBF334 pdat-1::dat-1 RNAi sas; podr-1::RFP; GBF325 pdat-1::GFP]. To knockdown the cat-2 gene: gbEx574 [GBF327 pdat-1::cat-2 RNAi sas; podr-1::RFP]. To knockdown the kal-1 gene: gbEx599 [GBF339 pdat-1::kal-1 RNAi sas; podr-1::RFP; GBF325 pdat-1::GFP]. To knockdown the unc-64 gene: gbEx585 [GBF335 pdat-1::unc-64 RNAi sas; podr-1::RFP; EM282 pcat-2::GFP] and gbEx613 [GBF335 pdat-1::unc-64 RNAi sas; podr-1::RFP; GBF325 pdat-1::GFP].
Construction of Transgenes for Neuron-Specific Knockdown
The construction of transgenes for neuron-specific knockdown was made by PCR fusion as previously described (Esposito et al., 2007). Genomic sequences, corresponding to the target gene and to promoters were amplified separately from C. elegans genomic DNA, unless otherwise noted. The promoter regions of cat-2 or dat-1 genes were chosen based on the cis-regulatory modules (CRM) necessary to drive expression specifically in dopaminergic neurons (Flames and Hobert, 2009; Illiano et al., 2017). The promoter specific expression in dopaminergic neurons was experimentally controlled by fusing them to gfp and by confirming that both were expressed in all dopaminergic neurons and only in them (data not shown). For the cat-2 promoter a 600 bp fragment, upstream of the ATG, was amplified using the following primers: Pf cat-2: ataataaaactgcgtggcgtg; Pr cat-2: ctcttccaatttttcaagggg. The nested primer used for the second step of fusion was: Pf∗2 cat-2: cgtgttgttaagaacgtgcttgatcg. For the dat-1 promoter a 795 bp fragment, upstream of the ATG, was amplified using the following primers: Pf dat-1: aaagtctttctgcccacacaa; Pr dat-1: agtaaaccgtagcgggatcag. The nested primer used for the second step was: Pf∗ dat-1: cgacctcatacactttctctcg. To amplify the C. elegans target genes to be silenced, we amplified the same exon rich regions that have been used for RNAi by feeding experiments (Kamath et al., 2003). For gfp expression and knockdown the fragment to be fused were amplified from A. Fire (Stanford University, United States) plasmids pPD95.75 and L4417, respectively. Primers used to fuse the gfp fragment to dat-1 promoter for expression were: Tf pdat-1::gfp sas: ctgatcccgctacggtttactTCACTATAGGGAGACCGGCA; Tr pdat-1::gfp sas: ctgatcccgctacggtttactTCACTATAGGGCGAATTGGG. Primers used to fuse the gfp fragment to cat-2 promoter for expression were: Tf pcat-2::gfp sas: ccccttgaaaaattggaagagTCACTATAGGGAGACCGGCA; Tr pcat-2::gfp sas: ccccttgaaaaattggaagagTCACTATAGGGCGAATTGGG. Primers used to amplify the gfp region (890 bp) for knockdown were: Tfa gfp sas: gttgtaaaacgacggccagt; Tfs gfp sas: GGCCGATTCATTAATGCAG; Tf∗ gfp sas: tcactataGGGAGACCGGCA; Tr∗ gfp sas: tcactatagggcgaattggg. Primers used to amplify the cat-2 region (1092 bp) for knockdown were: Tfa cat-2 sas: caagctcttgtgatccgtga; Tfs cat-2 sas: acaatctgctgaacgccttt Tf∗ cat-2 sas: GAAATTCTCGATTTTCGCCA; Tr∗ cat-1 sas: CTTCTTTGCACAACCCGAAT. Primers used to fuse the cat-2 fragment to dat-1 promoter for knockdown were: Tf pdat-1::cat-2 sas: ctgatcccgctacggtttactGAAATTCTCGATTTTCGCCA; Tr pdat-1::cat-2 sas: ctgatcccgctacggtttactCTTCTTTGCACAACCCGAAT. Primers used to amplify the dat-1 region (1190 bp) for knockdown were: Tfs dat-1 sas: TTCGAACCTGATCTCAACCC; Tfa dat-1 sas: TGCAGTTGGTGCCTACAGG; Tf∗ dat-1 sas: AAGCAAATGCACCGAACTCT; Tr∗dat-1 sas: AGCTCCAGCAAAACTTCCAA. Primers used to fuse the dat-1 fragment to dat-1 promoter for knockdown were: Tf pdat-1::dat-1 sas: ctgatcccgctacggtttactAAGCAAATGCACCGAACTCT; Tr pdat-1::dat-1 sas: ctgatcccgctacggtttactAGCTCCAGCAAAACTTCCAA. Primers used to amplify the unc-64 region (1999 bp) for knockdown were: Tfa1 unc-64 sas: cttttcgtgtcgagacctgtc; Tfs unc-64 sas: AATGCCAGGAATATACTGAATGAG; Tr∗ unc-64 (1) sas: CTCAATTCGATCAACCATCTCTC; Tf∗ unc-64 (1) sas: AGAGATTCGTGGAAGTGTGGATA. Primers used to fuse unc-64 fragments to dat-1 promoter for knockdown were: Tf pdat-1::unc-64 sas: ctgatcccgctacggtttactAGAGATTCGTGGAAGTGTGGATA; Tr pdat-1::unc-64 sas: ctgatcccgctacggtttactCTCAATTCGATCAACCATCTCTC. All the Tf and Tr primers had at their 5′-end 20/21 additional nucleotides complementary to 3′ end of the promoter used to drive the knockdown. A mixture of sense and anti-sense PCR fusion product at the concentration of 50 ng/μL was microinjected together with co-transformation markers into the gonad of animals using standard microinjection technique (Mello et al., 1991). The following co-transformation markers were injected at the concentration of 30 ng/μL: podr-1::RFP, expressed in the AWB and AWC neurons, a kind gift from C. Bargmann, Rockefeller University, United States; pJM371 pelt-2::NLS::RFP, expressed in intestinal cell nuclei, a kind gift from J. D. McGhee, University of Calgary, United States; EM#282 pcat-2::GFP expressed in dopaminergic neurons, a kind gift from S. Emmons, Albert Einstein College of Medicine, New York, United States. To test the silencing of gfp under the control of dat-1 or cat-2 promoters, the two PCR constructs were injected in two different integrated transgenic strains, in which the gfp is expressed in dopaminergic neurons with a “complementary” approach, i.e., pdat-1::gfp (RNAi sas) silencing construct was injected in otIs199 [pcat-2::GFP] transgenic strain and vice versa, pcat-2::gfp (RNAi sas) silencing construct was injected in BY200 (vtIs1 [pdat-1::GFP; rol-6(su1006)]) transgenic strain to avoid any disturbance of the same promoter on gene knockdown and on gene expression. To follow dopaminergic neurons in cultures some of the transgenic lines (i.e., gbEx624, gbEx613, and gbEx599) were obtained adding to the injection mix also GBF325 pdat-1::GFP fusion construct at 1 ng/μL. At least two lines for each genotype were analyzed in all cases and data pooled together.
Microscopy and Imaging
Animals were mounted and anesthetized with 0.01% tetramisole hydrochloride (Sigma-Aldrich, St. Louis, MO, United States) on 4% agar pads. The analysis of GFP expression and gfp knockdown in dopaminergic neurons was performed using Zeiss Axioskop microscopes (Carl Zeiss, Oberkochen, Germany).
Basal Slowing Response Assay
Basal slowing response assay was performed as previously described (Chase et al., 2004). Young adults, 18–22 h post-L4 stage at 20°C, were assayed. The locomotion rate of young adult animals was quantified by counting the number of body bends completed in five consecutive 20-s intervals in the presence or in absence of HB101 bacteria. Data were collected for six animals per condition, for a total of 30 measurements per condition. The percent of slowing was calculated by dividing the difference between locomotion rates on and off food by the locomotion rate off food.
Swimming-Induced Paralysis Assays
Animals were grown in agar plates seeded with NA22 bacteria and SWIP assays were performed as previously described (Carvelli et al., 2010). Briefly, in each SWIP trial, 8–16 age-synchronized larva-4 animals were placed in 40 μl of vehicle (200 mM sucrose) with or without 0.5 mM Amph (NIDA, Research Triangle Institute) in a single well of a Pyrex spot plate (Thermo Fisher Scientific, Waltham, MA, United States). Paralyzed animals were counted after 10 min using an inverted microscope (Carl Zeiss, Inc., Thornwood, NY, United States). The number of paralyzed animals was reported as a percentage of the total number of animals observed in each test ± standard error.
Caenorhabditis elegans Primary Cultures, [3H]DA Uptake and Release Experiments
We prepared embryonal cultures from animals grown on NA22 bacteria, as previously described (Carvelli et al., 2004). Briefly, 2-day-old embryonic cells (106 cells/well) were pre-loaded with 5 nM [3H]DA (NEN) for 30 min at room temperature. Cells were washed five times with bath solution containing 145 mM NaCl or NMDG+, 5 mM KCl, 1 mM CaCl2, 5 mM MgCl2, 10 mM HEPES, and 20 mM D-glucose (pH 7.2 and 350 osmolarity) and 100 μM Amph or bath solution were then applied for 1 min to induce DA release. Samples were collected and counted for radioactivity. For uptake experiments 2 × 106 cells were plated per well. Two days after seeded, cells were washed with bath solution and incubated with 50 nM [3H]DA for 5 min at room temperature. Uptake was terminated by washing the cells three times with ice-cold bath solution. Cells were quickly lysed using 1% SDS and samples were collected in vials to count radioactivity.
Statistical Analysis
GraphPad Prism software (GraphPad Software, Inc., San Diego, CA, United States) software was used for statistical analyses. The statistical significance was determined using one-way ANOVA with Bonferroni post-test, Kruskal–Wallis and Student’s t-tests. The SWIP data passed the Shapiro–Wilk normality test (α = 0.05). Data are reported as averages of multiple observations ± Standard error.
Results
Cell-Specific RNAi Efficiently Silences Genes in Dopaminergic Neurons
unc-64 null mutants exhibit larval lethality, locomotion abnormalities, pharyngeal pumping and defecation defects (van Swinderen et al., 2001). Knockdown of unc-64 using systemic RNAi causes growth and locomotion defects (Shephard et al., 2011). These defects do not allow using these deficient unc-64 animals to perform behavioral assays and to explore the role of unc-64 specifically in the dopaminergic neurons. To dissect the role played by unc-64 specifically in dopaminergic neurons, in otherwise wild type animals, we created transgenic animals in which a dopaminergic specific promoter drives the expression of part of the gene, in the sense and antisense directions (RNAi sas) (Esposito et al., 2007; Gallotta et al., 2016). We initially tested the specificity and the efficiency of two dopaminergic-specific promoters, pcat-2 and pdat-1 (Flames and Hobert, 2009). The cat-2 gene encodes the enzyme homolog to tyrosine hydroxylase, the rate-limiting enzyme required to produce DA. The dat-1 gene encodes the DA transporter homolog to DAT, required to regulate synaptic DA signaling by controlling extracellular DA levels (Jayanthi et al., 1998). In C. elegans, these genes have been described to be specifically expressed only in the dopaminergic neurons (Flames and Hobert, 2009; Illiano et al., 2017). After confirming the dopaminergic-specific expression of pcat-2 and pdat-1 using a reporter gene (data not shown), we tested their efficiency in silencing the GFP in transgenic lines where GFP is constitutively expressed in all dopaminergic neurons (Figure 1A). The pdat-1 promoter was largely more efficient in silencing the gfp (strain pdat-1::gfp sas), with only 15% of all dopaminergic neurons detectable by fluorescence microscopy (p < 0.001 vs control), while when using pcat-2 (strain pcat-2::gfp sas), 90% GFP-positive neurons were still detectable, a percentage very similar to controls (98%). These results showed that the transgene driven neuron-specific silencing technique (RNAi sas) can be successfully applied to the dopaminergic neurons, that pdat-1 is the appropriate promoter for this approach and we therefore used only this promoter in all the experiments. We then tested the efficiency of the dat-1 promoter in silencing a gene known to play a function in the dopaminergic neurons. Thus, we evaluated the ability of pdat-1 to knockdown cat-2 gene by performing BSR experiments. This behavioral assay tests the ability of wild type animals to slow down their rate of locomotion when they encounter a bacterial lawn. This behavior is mediated by DA; in fact, cat-2 null mutants do not exhibit BSR (Sawin et al., 2000). We used the BSR assay to evaluate the efficiency of the cat-2 gene knockdown in dopaminergic neurons using the RNAi sas technique (Figure 1B). We observed that, similar to cat-2(e1112) null mutants, the transgenic animals silenced for cat-2 (pdat-1::cat-2 sas) exhibited a defective BSR with respect to control animals (pdat-1::gfp sas). By representing BSR as the ratio between the locomotion rate off food and on food (% of Slowing in Figure 1B) we found that, similar to the cat-2(e1112) null mutants, the pdat-1::cat-2 sas animals exhibited a reduction in BSR, 6 and 15%, respectively. On the other hand, both the pdat-1::gfp sas animals (controls) and the wild type animals exhibited normal BSR values (45%). These results demonstrate that the RNAi sas technique is efficient, gene specific, and allows altering a DA-mediated behavior.
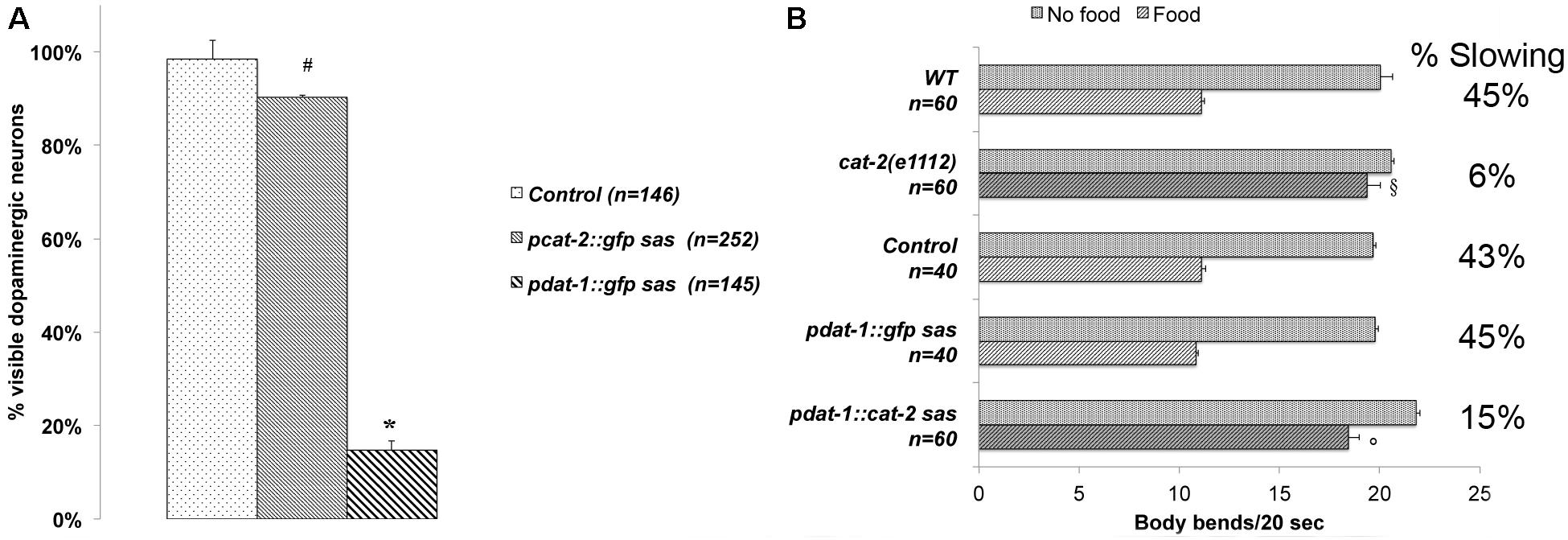
FIGURE 1. (A) dat-1 promoter is more efficient in silencing GFP expression in the dopaminergic neurons than the cat-2 promoter. Using a cell-specific RNAi technique (Esposito et al., 2007), the two promoters pcat-2 and pdat-1 were employed to silence the gfp gene specifically expressed in the dopaminergic neurons. Animals carry an integrated transgene for the expression of GFP only in dopaminergic neurons. The percentage of visible dopaminergic neurons expressing GFP over the number of neurons expected (eight per animal) is reported. pdat-1 promoter was significantly more efficient (∗p < 0.001, control vs pdat-1::gfp sas) in silencing the GFP expression than pcat-2 (#p > 0.01, control vs pcat-2::gfp sas). n is the number of animals observed. Statistical analysis was performed using one-way ANOVA with Bonferroni post-test. (B) cat-2 silencing through the dat-1 promoter is sufficient to mimic a cat-2 dependent behavior. Similar to cat-2(e1112) null mutants (Sawin et al., 2000), cat-2 silenced animals (pdat-1::cat-2 sas) do not slowdown in presence of food. On the other hand, wild type, controls (non-transgenic siblings) and GFP silenced (pdat-1::gfp sas) animals reduce their locomotion rate when encounter bacteria. The locomotion rate for 20 s off and on food is reported as percent of slowing (% Slowing) calculated by dividing the difference between locomotion rates on and off food by the locomotion rate off food. n represents the number of animals tested. Kruskal–Wallis non-parametric with Dunn’s multiple comparison post-test shows significant difference between wild type animals on food vs cat-2(e1112) on food (§ p < 0.001) and pdat-1::gfp sas on food vs pdat-1::cat-2 sas on food (°p < 0.001).
Dopamine Neuron-Specific Silencing of unc-64 Causes DA-Dependent Behaviors
The results shown in Figure 1B encouraged us to apply the RNAi sas technique to specifically knockdown unc-64 in the dopaminergic neurons. Therefore using the dat-1 promoter, we created the pdat-1::unc-64 sas mutants. Contrary to what observed in unc-64 null or hypomorphic mutants or after systemic RNAi (van Swinderen et al., 2001; Shephard et al., 2011), the pdat-1::unc-64 sas animals were viable and did not present obvious developmental defects. To assess whether unc-64 was knocked down in the dopaminergic neurons, we tested these mutants for BSR. As mentioned above, the BSR phenotype depends on DA and specifically extracellular DA released by the dopaminergic neurons. Since, UNC-64 is an essential factor for vesicular fusion and neurotransmitter release, we hypothesized that the lack of function of UNC-64 would prevent DA release and consequently would cause defective BSR. Our results show that like the null mutants cat-2(e1112), which cannot synthesize DA, the pdat-1::unc-64 sas lines, which cannot release DA via vesicle fusion, failed to show BSR, 6.4 and 3%, respectively (Figure 2A). For these experiments we created, as negative control, transgenic animals that were knocked down for the kal-1 gene using pdat-1 (pdat-1::kal-1 sas). We reasoned that kal-1 silencing represents the proper control because, while gfp is exogenously injected in worms to create transgenic animals, kal-1 is natively expressed in most C. elegans neurons (Rugarli et al., 2002). As expected, both the wild type and pdat-1::kal-1 sas animals exhibited BSR when encountering food (58.3 and 48.4%, respectively; Figure 2A). In absence of food, we observed a slight but statistically significant 23% reduction (#p < 0.001) of body bends in the pdat-1::unc-64 sas animals with respect to controls (pdat-1::kal-1 sas). These data suggest that the pdat-1::unc-64 sas animals have a modest reduction in locomotion. To better understand the extent of this reduction, we tested the hypomorphic unc-64(e246) mutant. Although non-lethal, the unc-64(e246) mutation produces a point mutation in the unc-64 gene (Ogawa et al., 1998) which causes severe locomotion defects. In fact, in our BSR experiments the unc-64(e246) animals besides lacking the BSR phenotype, also showed a strong reduction of body bends (4 ± 0.4) in absence of food with respect to wild type animals (13.6 ± 0.5; ˆp < 0.0001). Since the number of body bends in absence of food of the pdat-1::unc-64 sas animals was significantly higher (10 ± 0.4) then those observed in unc-64(e246) mutants, this result might suggest that in the pdat-1::unc-64 sas mutants the unc-64 silencing occurs in a restricted number of cells, most likely the dopaminergic neurons, rather than malfunctioning in every cells, as in unc-64(e246) animals. On the other hand, the number of body bends of the pdat-1::unc-64 sas tested on food was significantly higher than those measured in the pdat-1::kal-1 sas animals (&p < 0.001, Kruskal–Wallis test). This result shows that pdat-1::unc-64 sas mutants are impaired in the BSR behavior compared to the control animals (pdat-1::kal-1 sas and wild type).
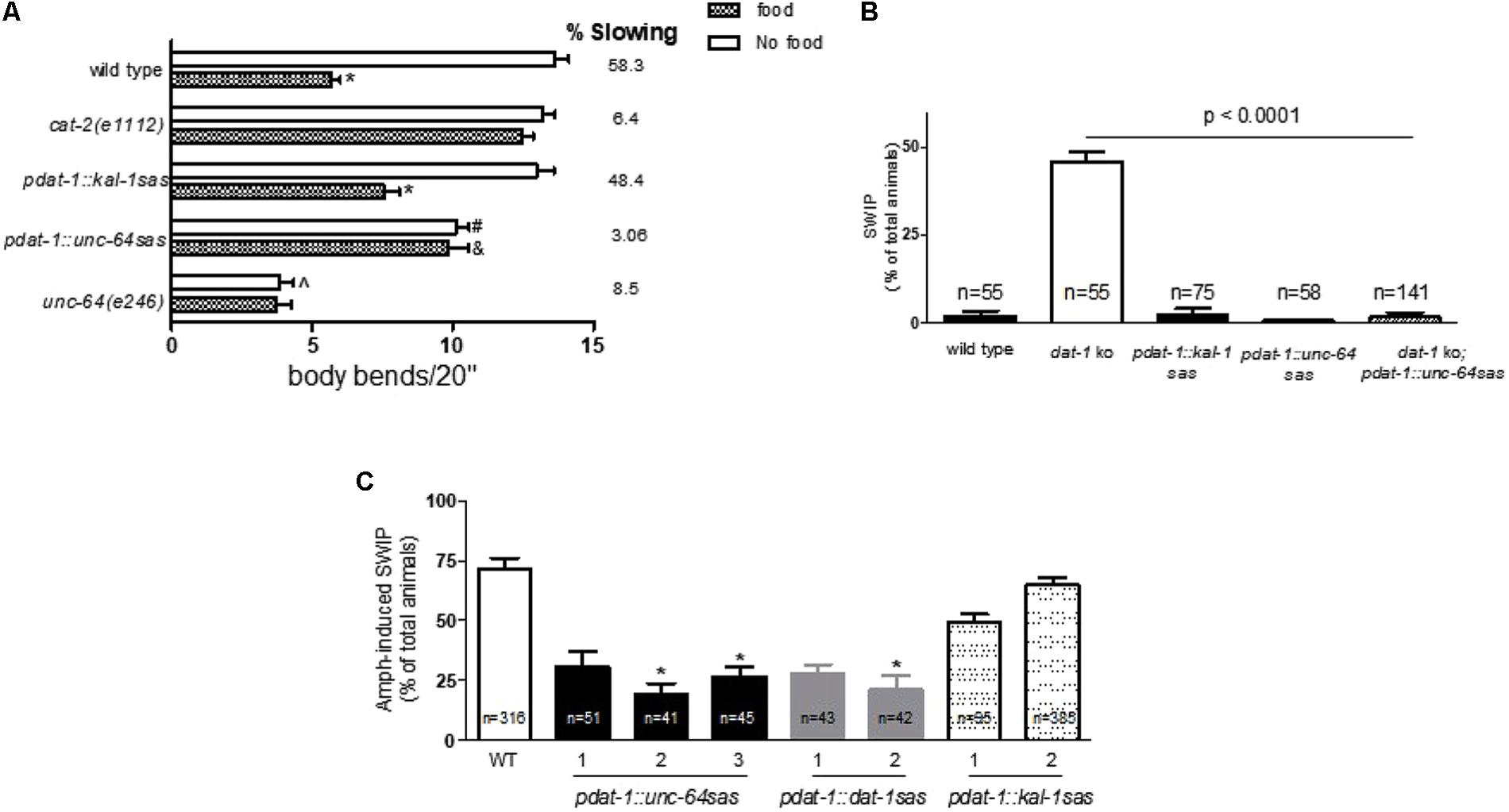
FIGURE 2. Dopamine neuron-selective unc-64 knockdown alters DA-dependent behaviors. (A) Similarly to cat-2(e1112) knockouts (6.4%), the pdat-1::unc-64 sas lines exhibit highly reduced BSR (3%) with respect to wild type and pdat-1::kal-1 sas animals, 58 and 48%, respectively (∗p < 0.0001). In presence of food, the pdat-1::unc-64 sas mutants show a significant 33% increase (&p < 0.001) in body bends with respect to the control (pdat-1::kal-1 sas). The pdat-1::unc-64 sas mutants exhibit a slight but significant 21% reduction in body bends in absence of food with respect to pdat-1::kal-1 sas animals (#p < 0.001). Under the same conditions, the unc-64(e246) mutants exhibit a 71% reduction in body bends with respect to wild type animals (ˆp < 0.0001). Animals were assayed as in Figure 1B. At least 18 animals were tested per each sample. (B) unc-64 silencing in the dopaminergic neurons recovers the dat-1 knockout phenotype. dat-1 ko animals exhibit SWIP when forced to swim for 10 min (McDonald et al., 2007). This phenotype was recovered in the dat-1 ko;pdat-1::unc-64 sas double mutants (Kruskal–Wallis non-parametric test). Wild type, pdat-1;kal-1 sas (controls) and pdat-1::unc-64 animals do not exhibit SWIP. (C) Similarly to dat-1 null mutants (Carvelli et al., 2010) and the two dat-1 silenced strains (pdat-1::dat-1 sas), animals that are silenced for unc-64 specifically in the dopaminergic neurons using the dat-1 promoter (pdat-1::unc-64 sas) exhibit reduced Amph-induced swimming-induced paralysis (SWIP). Two lines of kal-1 silenced animals (pdat-1::kal-1 sas), used as negative control, exhibit AMPH-induced SWIP values that are comparable to those observed in wild type animals. The three black bars for pdat-1::unc-64 sas, two gray bars for pdat-1::dat-1 sas and two dotted bars for pdat-1::kal-1 sas represent independent lines. In (A–C), statistical analysis was performed using one-way ANOVA followed by both Bonferroni and Kruskal–Wallis non-parametric post-tests. n represents the number of animals tested per each strain.
To further investigate the lack of function of UNC-64 in the dopaminergic neurons in the pdat-1::unc-64 sas animals, we utilized another DA-dependent behavior: SWIP. Previously, we showed that when immersed in liquid solutions, wild type worms swim vigorously for hours; however, dat-1 null mutants exhibit SWIP, i.e., animals sink to the bottom of the well and do not move (McDonald et al., 2007). Since we showed that SWIP is in part caused by an increase of extracellular DA due to lack of function of DAT-1 (McDonald et al., 2007; Carvelli et al., 2008, 2010), we hypothesized that SWIP observed in dat-1 null mutants can be recovered by knocking down unc-64 in the dopaminergic neurons. In this case, even if DAT-1 cannot reuptake DA (dat-1 ko), the animals would not show SWIP because DA cannot be released (pdat-1::unc-64 sas). Thus, using the pdat-1, we knocked down unc-64 in the dat-1 null mutants and create the dat-1 ko;pdat-1::unc-64 sas double mutants. We found that the double mutants did not exhibited SWIP (p < 0.001 vs dat-1 ko, Figure 2B), confirming therefore that the RNAi sas technique we used, causes genetic ablation of unc-64 in the dopaminergic neurons and, as a consequence, it prevents DA release. No SWIP was observed in control animals (wild type and pdat-1::kal-1 sas) or pdat-1::unc-64 sas mutants (Figure 2B). Taken together, these results suggest that the pdat-1::unc-64 sas mutants have reduced ability to release DA most likely because of the reduced expression of unc-64 in the dopaminergic neurons.
Dopamine Neuron-Specific Silencing of unc-64 Reduces Amph-Induced Behaviors
Previously, we demonstrated that Amph, a drug that increases extracellular DA (Di Chiara and Imperato, 1988), causes SWIP in C. elegans (Carvelli et al., 2010). Here we investigated whether reduced expression of unc-64 in the dopaminergic neurons (pdat-1::unc-64 sas) would alter Amph-induced SWIP. We found that three independent transgenic lines of pdat-1::unc-64 sas animals treated with Amph exhibited significantly reduced SWIP with respect to wild type animals (black bars in Figure 2C; ∗p < 0.0001). We also measured SWIP in transgenic animals that were silenced for the dat-1 (pdat-1::dat-1 sas) or kal-1 (pdat-1::kal-1 sas) genes, used as positive and negative controls, respectively. Interestingly, the reduction observed in the pdat-1::unc-64 sas animals was comparable to that observed in the two independent transgenic lines of pdat-1::dat-1 sas animals (gray bars in Figure 2C; ∗p < 0.0001). And, as expected, the two independent transgenic lines of pdat-1::kal-1 sas animals did not show significant change in Amph-induced SWIP with respect to wild type animals (dotted bars in Figure 2C). These results suggest that unc-64 might play a role in the mechanism of action of Amph that ultimately generates SWIP.
Dopaminergic-Specific Silencing of unc-64 Reduces Amph-Induced DA Release and DA Uptake
The strong reduction of Amph-induced SWIP measured in the pdat-1::unc-64 sas animals suggested that UNC-64, like its mammalian homolog Syntaxin-1A, is a protein required by Amph to induce DA efflux (Binda et al., 2008; Daberkow et al., 2013). To test this hypothesis we performed in vitro experiments using C. elegans primary cultures (Christensen et al., 2002; Carvelli et al., 2004). After preloading the embryonic cells with [3H]DA, cells were treated with Amph to induce release of [3H]DA. We found that cells derived from pdat-1::unc-64 sas animals had significant reduced [3H]DA release with respect to the control pdat-1::kal-1 sas cells (Figure 3A; ∗p < 0.05, t-test).
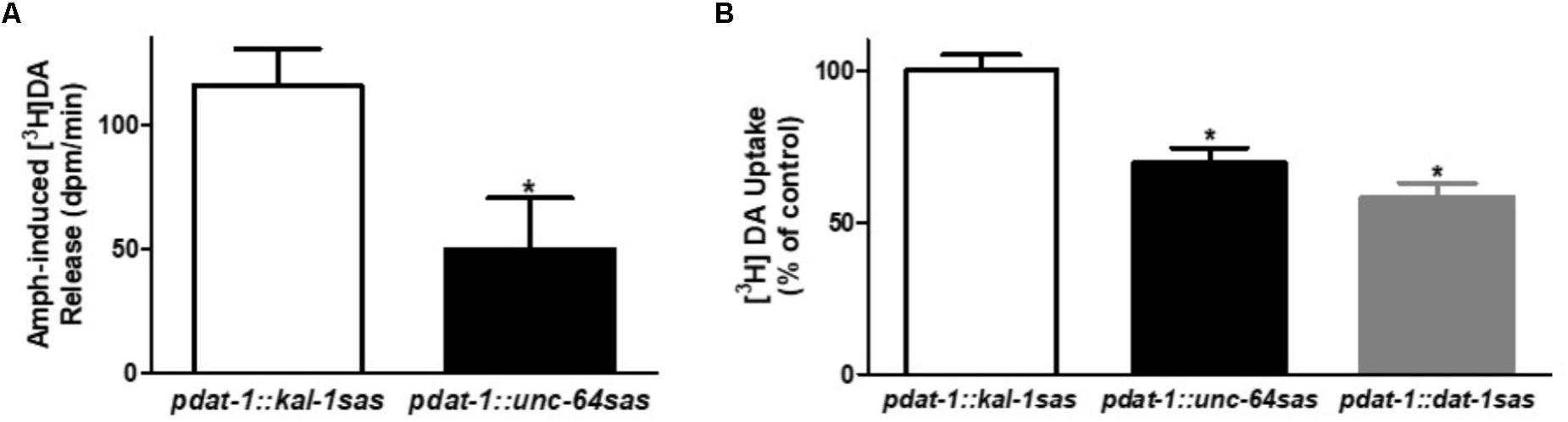
FIGURE 3. Dopamine neuron-selective unc-64 knockdown decreases DA release and DA uptake. Cultured dopaminergic neurons isolated from transgenic animals were used to measure the Amph-induced DA release (A) and DA uptake (B). (A) unc-64 silenced cells (pdat-1::unc-64 sas) show a statistically significant reduction of [3H]DA release (∗p ≤ 0.0001) with respect to control cells (pdat-1::kal-1 sas). t-Test was used to performed statistical analysis. Data are presented as the difference of dpm (disintegration per minute) between samples treated with Amph and samples treated with control solution. (B) pdat-1::unc-64 sas cells show a statistically significant 30% reduction of [3H]DA uptake (∗p ≤ 0.001) with respect to control neurons (pdat-1::kal-1 sas). A similar reduction (40%) was observed in cells silenced for dat-1 (pdat-1::dat-1 sas). Statistical analysis was performed using one-way ANOVA and Bonferroni post-test. Both data are average of values obtained from three independent experiments.
Previous studies showed that Syntaxin-1A interacts and regulates the activity of DAT (Binda et al., 2008; Carvelli et al., 2008; Cervinski et al., 2010). For example, Cervinski et al. (2010) showed that heterologous co-expression of Syntaxin-1A with rat DAT led to a reduction in DAT surface expression, which resulted in a reduction of DA uptake. We tested if this was also true in our native cultured cells by performing [3H]DA uptake experiments (Figure 3B). We found a significant reduction (31 ± 5%) in the uptake of the pdat-1::unc-64 sas cells with respect to the controls (pdat-1::kal-1 sas cells). Interestingly, this reduction was comparable to that obtained in pdat-1::dat-1 sas cells derived from animals in which dat-1 gene was silenced using the RNAi sas technique (42 ± 5%). Because DA is accumulated inside the neurons by DAT, these results suggest that reduced expression of UNC-64 in the dopaminergic neurons causes a reduction of DAT activity or, as Cervinski et al. (2010) previously showed, a reduction of DAT expression on the cell membrane. Moreover, these results suggest that the reduced [3H]DA release observed in the pdat-1::unc-64 sas cells (Figure 3A) may result from less [3H]DA moving inside the neurons as seen in our uptake results (Figure 3B).
Discussion
In the present study, we explored the role played by the Syntaxin-1A C. elegans homolog, unc-64, in the dopaminergic neurons. We used C. elegans because this model is amenable to genetic manipulations and conserves a high homology with the human dopaminergic-signaling pathway. To overcome the limitations presented by genetic null/hypomorphic mutants or systemic RNAi technique, we applied an alternative RNAi strategy, named RNAi sense and antisense (RNAi sas), to generate transgenic animals in which the function of unc-64 gene was knocked down only in the dopaminergic neurons. This strategy, originally developed in our laboratory (Esposito et al., 2007), has been adopted by several groups to successfully silence various genes in almost all C. elegans tissues including neurons. In this study, we determined that the dopaminergic-specific promoter pdat-1 is able to specifically and efficiently drive the knockdown of a reporter-gene, such as gfp, in the dopaminergic neurons only. The fact that pdat-1 was more efficient than pcat-2 in silencing the reporter gene may be due to a higher transcriptional activity of the promoter sequence we have chosen; hence a higher concentration of RNA silencing molecules was produced. Indeed, a clear difference in gfp intensity was visible when the same promoter was used to express the GFP as reporter gene (data not shown). The dat-1 promoter sequence we chose contains three CRM or DA motifs, which are required and sufficient to drive expression in all dopaminergic neurons; on the other hand, the cat-2 promoter we used had only one CRM motif (Flames and Hobert, 2009). Importantly, the dat-1 promoter was very efficient in silencing genes that are endogenously expressed in the dopaminergic neurons, i.e., cat-2 and dat-1, and therefore, pdat-1 became the candidate promoter for silencing unc-64 in these neurons.
Several studies have recognized the SNARE protein Syntaxin-1A as a key player of neurotransmitter release (Salaün et al., 2004) and one of the regulatory proteins of DAT. By binding to the DAT N-terminal domain (Lee et al., 2004), Syntaxin-1A modulates the release, transport, and ion channel activities of DAT (Binda et al., 2008; Carvelli et al., 2008; Cervinski et al., 2010). Thus, Syntaxin-1A may represent an important key element in the dopaminergic circuit that controls the amount of DA in the synaptic cleft. Using the RNAi sas technique, we created transgenic lines silenced for unc-64 specifically in the dopaminergic neurons that are viable, able to grow and do not exhibit severe locomotor dysfunctions. This allowed us to overcome the limitations observed using classical unc-64 RNAi, such as defects in growth and in locomotion (Shephard et al., 2011). Moreover, since the RNAi sas is a transgenic-based approach, the silencing constructs are heritable. This was a crucial requirement for the feasibility of both our in vivo and in vitro experiments (Figures 2, 3). Two distinct behavioral assays, BSR and SWIP, were used to test whether we effectively silenced the expression of unc-64 in the dopaminergic neurons of pdat-1::unc-64 sas mutants. Both assays depend on the ability of the dopaminergic neurons to release DA, which ultimately makes the animals to slow down when they encounter food, BSR (Sawin et al., 2000), or to stop swimming if the DAT-1 function is ablated, SWIP (McDonald et al., 2007). The pdat-1::unc-64 sas mutants did not exhibit BSR, suggesting therefore that the lack of DA release is caused by the silencing of unc-64 in the dopaminergic neurons (Figure 2A). Moreover, the SWIP behavior observed in the dat-1 ko mutants was recovered in the dat-1 ko;pdat-1::unc-64 sas double mutants, i.e., in animals that cannot release DA. Taken together, these results suggest that the RNAi sas strategy we used effectively silences unc-64 in the dopaminergic neurons. We cannot exclude that the pdat-1::unc-64 sas mutants are deficient of unc-64 in additional cells other than the dopaminergic neurons; however, we can speculate that the lack of obvious developmental defects seen in these animals makes this possibility quite unlikely. This hypothesis is also supported by our behavioral data (Figure 2A). In fact, while the hypomorphic unc-64 mutants (unc-64(e246)) move very slowly in absence of food (four body bends/20 s), the pdat-1::unc-64 sas mutants move almost three times faster (10 body bends/20 s) than the unc-64 hypomorphic mutants, and only slightly slower than the wild type animals (13 body bends/20 s).
Three pdat-1::unc-64 sas transgenic lines obtained with the RNAi sas technique (Figure 2C), exhibited a strong reduction in Amph-induced SWIP. Because the increase of extracellular DA is one of the causes generating Amph-induced SWIP (Carvelli et al., 2010; Safratowich et al., 2013, 2014), the reduction in SWIP seen in pdat-1::unc-64 sas animals treated with Amph could be due to a reduced amount of DA released in the synaptic cleft. We tested this hypothesis by performing in vitro assays, and we found that indeed the release of DA triggered by Amph was diminished in dopaminergic neurons of pdat-1::unc-64 sas mutants. Previous studies have shown that Amph evokes DA release through two separate mechanisms (Siciliano et al., 2014), one vesicle-independent and DAT-mediated (Sulzer et al., 1993; Jones et al., 1998) and one DAT independent and vesicle-mediated (Daberkow et al., 2013). Because UNC-64 is required to dock and fuse the vesicles at the cell membrane such that the neurotransmitter can be released, the reduced Amph-induced DA release measured in neurons silenced for unc-64 could indicate that fewer vesicles empty their DA into the synaptic cleft. We also found that the reduced expression of unc-64 in the dopaminergic neurons (pdat-1::unc-64 sas) diminished the DA uptake (Figure 3B). These results are in accordance with previous published data showing that Syntaxin-1A decreases DA uptake by reducing the amount of DAT on the cell membrane (Cervinski et al., 2010). If we assume that a reduction in the number of DAT proteins is responsible of the diminished DA uptake observed in the pdat-1::unc-64 sas neurons (Figure 3B), then we can speculate that less Amph is taken up inside these neurons since Amph, like DA, is a DAT substrate. This, in turn, would be the cause of the reduced DA release we measured in the pdat-1::unc-64 sas neurons (Figure 3A). In fact, with less Amph moved inside, we get less DA released out. Regardless of these speculations and interpretations, we may conclude here that in C. elegans, Syntaxin-1A besides controlling the basal release of DA also moderates the behavioral effects generated by Amph by reducing the amount of DA in the synaptic cleft.
Author Contributions
AL carried out the molecular genetic manipulations, microscope analysis, and BSR assays. BDS performed SWIP assays, the [3H]DA release and uptake experiments. IG did the initial molecular genetic manipulations and initial characterization. GZ participated in the genetic manipulations. SRK performed the BSR assays. LC and EDS conceived, designed, coordinated the study and wrote the manuscript. All authors read and approved the final manuscript.
Funding
This work was supported by the grants: RF2009-1473235 and FIRB-Merit grant RBNE08LN4P_002 to AL and EDS and the NIH R21 DA024797 and R01 DA042156 to LC.
Conflict of Interest Statement
The authors declare that the research was conducted in the absence of any commercial or financial relationships that could be construed as a potential conflict of interest.
Acknowledgments
The authors thank P. Bazzicalupo for critical reading of the manuscript; S. Emmons (Albert Einstein College of Medicine, New York, United States), C. Bargmann (The Rockefeller University, New York, United States), J. McGhee (University of Calgary, Canada), and A. Fire (Stanford University, United States) for reagents and strains; the Caenorhabditis Genetics Center (CGC), which is funded by NIH Office of Research Infrastructure Programs (P40 OD010440), for strains; Mariarosaria Aletta for bibliographic support; Wormbase.
Abbreviations
C. elegans, Caenorhabditis elegans; BSR, basal slowing response; DAT, dopamine transporter; DA, dopamine; Amph, amphetamine; GFP, green fluorescent protein; sas, sense and antisense; RNAi, RNA interference; SWIP, swimming-induced paralysis.
References
Arien, H., Wiser, O., Arkin, I. T., Leonov, H., and Atlas, D. (2003). Syntaxin 1A modulates the voltage-gated L-type calcium channel (Ca v 1.2) in a cooperative manner. J. Biol. Chem. 278, 29231–29239. doi: 10.1074/jbc.M301401200
Binda, F., Dipace, C., Bowton, E., Robertson, S. D., Lute, B. J., Fog, J. U., et al. (2008). Syntaxin 1A interaction with the dopamine transporter promotes amphetamine-induced dopamine efflux. Mol. Pharmacol. 74, 1101–1108. doi: 10.1124/mol.108.048447
Cartier, E., Hamilton, P. J., Belovich, A. N., Shekar, A., Campbell, N. G., Saunders, C., et al. (2015). Rare autism-associated variants implicate syntaxin 1 (STX1 R26Q) phosphorylation and the dopamine transporter (hDAT R51W) in dopamine neurotransmission and behaviors. EBioMedicine 2, 135–146. doi: 10.1016/j.ebiom.2015.01.007
Carvelli, L., Blakely, R. D., and DeFelice, L. J. (2008). Dopamine transporter/syntaxin 1A interactions regulate transporter channel activity and dopaminergic synaptic transmission. Proc. Natl. Acad. Sci. U.S.A. 105, 14192–14197. doi: 10.1073/pnas.0802214105
Carvelli, L., Matthies, D. S., and Galli, A. (2010). Molecular mechanisms of amphetamine actions in Caenorhabditis elegans. Mol. Pharmacol. 78, 151–156. doi: 10.1124/mol.109.062703
Carvelli, L., McDonald, P. W., Blakely, R. D., and DeFelice, L. J. (2004). Dopamine transporters depolarize neurons by a channel mechanism. Proc. Natl. Acad. Sci. U.S.A. 101, 16046–16051. doi: 10.1073/pnas.0403299101
Cervinski, M. A., Foster, J. D., and Vaughan, R. A. (2010). Syntaxin 1A regulates dopamine transporter activity, phosphorylation and surface expression. Neuroscience 170, 408–416. doi: 10.1016/j.neuroscience.2010.07.025
Chase, D. L., Pepper, J. S., and Koelle, M. R. (2004). Mechanism of extrasynaptic dopamine signaling in Caenorhabditis elegans. Nat. Neurosci. 7, 1096–1103. doi: 10.1038/nn1316
Christensen, M., Estevez, A., Yin, X., Fox, R., Morrison, R., McDonnell, M., et al. (2002). A primary culture system for functional analysis of C. elegans neurons and muscle cells. Neuron 33, 503–514. doi: 10.1016/S0896-6273(02)00591-3
Condliffe, S. B., Zhang, H., and Frizzell, R. A. (2004). Syntaxin 1A regulates ENaC channel activity. J. Biol. Chem. 279, 10085–10092. doi: 10.1074/jbc.M313592200
Daberkow, D. P., Brown, H. D., Bunner, K. D., Kraniotis, S. A., Doellman, M. A., Ragozzino, M. E., et al. (2013). Amphetamine paradoxically augments exocytotic dopamine release and phasic dopamine signals. J. Neurosci. 33, 452–463. doi: 10.1523/JNEUROSCI.2136-12.2013
Deken, S. L., Beckman, M. L., Boos, L., and Quick, M. W. (2000). Transport rates of GABA transporters: regulation by the N-terminal domain and syntaxin 1A. Nat. Neurosci. 3, 998–1003. doi: 10.1038/79939
Di Chiara, G., and Imperato, A. (1988). Drugs abused by humans preferentially increase synaptic dopamine concentrations in the mesolimbic system of freely moving rats. Proc. Natl. Acad. Sci. U.S.A. 85, 5274–5278. doi: 10.1073/pnas.85.14.5274
Esposito, G., Di Schiavi, E., Bergamasco, C., and Bazzicalupo, P. (2007). Efficient and cell specific knock-down of gene function in targeted C. elegans neurons. Gene 395, 170–176. doi: 10.1016/j.gene.2007.03.002
Fan, H.-P., Fan, F.-J., Bao, L., and Pei, G. (2006). SNAP-25/Syntaxin 1A complex functionally modulates neurotransmitter γ-Aminobutyric acid reuptake. J. Biol. Chem. 281, 28174–28184. doi: 10.1074/jbc.M601382200
Flames, N., and Hobert, O. (2009). Gene regulatory logic of dopamine neuron differentiation. Nature 458, 885–889. doi: 10.1038/nature07929
Gallotta, I., Mazzarella, N., Donato, A., Esposito, A., Chaplin, J. C., Castro, S., et al. (2016). Neuron-specific knock-down of SMN1 causes neuron degeneration and death through an apoptotic mechanism. Hum. Mol. Genet. 25, 2564–2577. doi: 10.1093/hmg/ddw119
Geerlings, A., Núñez, E., López-Corcuera, B., and Aragón, C. (2001). Calcium- and syntaxin 1-mediated trafficking of the neuronal glycine transporter GLYT2. J. Biol. Chem. 276, 17584–17590. doi: 10.1074/jbc.M010602200
Haase, J., Killian, A. M., Magnani, F., and Williams, C. (2001). Regulation of the serotonin transporter by interacting proteins. Biochem. Soc. Trans. 29, 722–728. doi: 10.1042/bst0290722
Horton, N., and Quick, M. W. (2001). Syntaxin 1A up-regulates GABA transporter expression by subcellular redistribution. Mol. Membr. Biol. 18, 39–44. doi: 10.1080/09687680010029383
Illiano, P., Lanzo, A., Leo, D., Paglione, M., Zampi, G., Gainetdinov, R. R., et al. (2017). A Caenorhabditis elegans model to study dopamine transporter deficiency syndrome. Eur. J. Neurosci. 45, 207–214. doi: 10.1111/ejn.13366
Jayanthi, L. D., Apparsundaram, S., Malone, M. D., Ward, E., Miller, D. M., Eppler, M., et al. (1998). The Caenorhabditis elegans gene T23G5.5 encodes an antidepressant- and cocaine-sensitive dopamine transporter. Mol. Pharmacol. 54, 601–609.
Jones, S. R., Gainetdinov, R. R., Wightman, R. M., and Caron, M. G. (1998). Mechanisms of amphetamine action revealed in mice lacking the dopamine transporter. J. Neurosci. 18, 1979–1986. doi: 10.1523/JNEUROSCI.18-06-01979.1998
Kamath, R. S., Fraser, A. G., Dong, Y., Poulin, G., Durbin, R., Gotta, M., et al. (2003). Systematic functional analysis of the Caenorhabditis elegans genome using RNAi. Nature 421, 231–237. doi: 10.1038/nature01278
Khoshbouei, H., Wang, H., Lechleiter, J. D., Javitch, J. A., and Galli, A. (2003). Amphetamine-induced dopamine efflux: a voltage-sensitive and intracellular Na+-dependent mechanism. J. Biol. Chem. 278, 12070–12077. doi: 10.1074/jbc.M212815200
Lee, K.-H., Kim, M.-Y., Kim, D.-H., and Lee, Y.-S. (2004). Syntaxin 1A and receptor for activated C kinase interact with the N-terminal region of human dopamine transporter. Neurochem. Res. 29, 1405–1409. doi: 10.1023/B:NERE.0000026404.08779.43
Markota, M., Sin, J., Pantazopoulos, H., Jonilionis, R., and Berretta, S. (2014). Reduced dopamine transporter expression in the amygdala of subjects diagnosed with schizophrenia. Schizophr. Bull. 40, 984–991. doi: 10.1093/schbul/sbu084
McDonald, P. W., Hardie, S. L., Jessen, T. N., Carvelli, L., Matthies, D. S., and Blakely, R. D. (2007). Vigorous motor activity in Caenorhabditis elegans requires efficient clearance of dopamine mediated by synaptic localization of the dopamine Transporter DAT-1. J. Neurosci. 27, 14216–14227. doi: 10.1523/JNEUROSCI.2992-07.2007
Mello, C. C., Kramer, J. M., Stinchcomb, D., and Ambros, V. (1991). Efficient gene transfer in C.elegans: extrachromosomal maintenance and integration of transforming sequences. EMBO J. 10, 3959–3970.
Miller, G. W., Gainetdinov, R. R., Levey, A. I., and Caron, M. G. (1999). Dopamine transporters and neuronal injury. Trends Pharmacol. Sci. 20, 424–429. doi: 10.1016/S0165-6147(99)01379-6
Naren, A. P., Quick, M. W., Collawn, J. F., Nelson, D. J., and Kirk, K. L. (1998). Syntaxin 1A inhibits CFTR chloride channels by means of domain-specific protein-protein interactions. Proc. Natl. Acad. Sci. U.S.A. 95, 10972–10977. doi: 10.1073/pnas.95.18.10972
Ogawa, H., Harada, S. I., Sassa, T., Yamamoto, H., and Hosono, R. (1998). Functional properties of the unc-64 gene encoding a Caenorhabditis elegans syntaxin. J. Biol. Chem. 273, 2192–2198. doi: 10.1074/jbc.273.4.2192
Quick, M. W. (2003). Regulating the conducting states of a mammalian serotonin transporter. Neuron 40, 537–549. doi: 10.1016/S0896-6273(03)00605-6
Quick, M. W. (2006). The role of SNARE proteins in trafficking and function of neurotransmitter transporters. Handb. Exp. Pharmacol. 175, 181–196. doi: 10.1007/3-540-29784-7_9
Rugarli, E. I., Di Schiavi, E., Hilliard, M. A., Arbucci, S., Ghezzi, C., Facciolli, A., et al. (2002). The Kallmann syndrome gene homolog in C. elegans is involved in epidermal morphogenesis and neurite branching. Development 129, 1283–1294.
Safratowich, B. D., Hossain, M., Bianchi, L., and Carvelli, L. (2014). Amphetamine potentiates the effects of -phenylethylamine through activation of an amine-gated chloride channel. J. Neurosci. 34, 4686–4691. doi: 10.1523/JNEUROSCI.3100-13.2014
Safratowich, B. D., Lor, C., Bianchi, L., and Carvelli, L. (2013). Amphetamine activates an amine-gated chloride channel to generate behavioral effects in Caenorhabditis elegans. J. Biol. Chem. 288, 21630–21637. doi: 10.1074/jbc.M113.484139
Salaün, C., James, D. J., Greaves, J., and Chamberlain, L. H. (2004). Plasma membrane targeting of exocytic SNARE proteins. Biochim. Biophys. Acta 1693, 81–89. doi: 10.1016/j.bbamcr.2004.05.008
Sawin, E. R., Ranganathan, R., and Horvitz, H. R. (2000). C. elegans locomotory rate is modulated by the environment through a dopaminergic pathway and by experience through a serotonergic pathway. Neuron 26, 619–631. doi: 10.1016/S0896-6273(00)81199-X
Shephard, F., Adenle, A. A., Jacobson, L. A., and Szewczyk, N. J. (2011). Identification and Functional clustering of genes regulating muscle protein degradation from amongst the known C. elegans muscle mutants. PLoS One 6:e24686. doi: 10.1371/journal.pone.0024686
Siciliano, C. A., Calipari, E. S., Ferris, M. J., and Jones, S. R. (2014). Biphasic mechanisms of amphetamine action at the dopamine terminal. J. Neurosci. 34, 5575–5582. doi: 10.1523/JNEUROSCI.4050-13.2014
Sulzer, D., Maidment, N. T., and Rayport, S. (1993). Amphetamine and other weak bases act to promote reverse transport of dopamine in ventral midbrain neurons. J. Neurochem. 60, 527–535. doi: 10.1111/j.1471-4159.1993.tb03181.x
Sung, U., Apparsundaram, S., Galli, A., Kahlig, K. M., Savchenko, V., Schroeter, S., et al. (2003). A regulated interaction of syntaxin 1A with the antidepressant-sensitive norepinephrine transporter establishes catecholamine clearance capacity. J. Neurosci. 23, 1697–1709. doi: 10.1523/JNEUROSCI.23-05-01697.2003
Tsuk, S., Michaelevski, I., Bentley, G. N., Joho, R. H., Chikvashvili, D., and Lotan, I. (2004). Kv2.1 channel activation and inactivation is influenced by physical interactions of both syntaxin 1A and the syntaxin 1A/Soluble N-Ethylmaleimide-Sensitive Factor-25 (t-SNARE) complex with the c terminus of the channel. Mol. Pharmacol. 67, 480–488. doi: 10.1124/mol.104.005314
van Swinderen, B., Metz, L. B., Shebester, L. D., Mendel, J. E., Sternberg, P. W., and Crowder, C. M. (2001). Goalpha regulates volatile anesthetic action in Caenorhabditis elegans. Genetics 158, 643–655.
Wang, D., Deken, S. L., Whitworth, T. L., and Quick, M. W. (2003). Syntaxin 1A Inhibits GABA flux, efflux, and exchange mediated by the rat brain GABA transporter GAT1. Mol. Pharmacol. 64, 905–913. doi: 10.1124/mol.64.4.905
Keywords: Syntaxin-1A, dopamine, amphetamine, C. elegans, dopamine transporter
Citation: Lanzo A, Safratowich BD, Kudumala SR, Gallotta I, Zampi G, Di Schiavi E and Carvelli L (2018) Silencing of Syntaxin 1A in the Dopaminergic Neurons Decreases the Activity of the Dopamine Transporter and Prevents Amphetamine-Induced Behaviors in C. elegans. Front. Physiol. 9:576. doi: 10.3389/fphys.2018.00576
Received: 19 January 2018; Accepted: 01 May 2018;
Published: 22 May 2018.
Edited by:
Robert Huber, Bowling Green State University, United StatesReviewed by:
Eric Andrew Engleman, Indiana University Bloomington, United StatesMaria de la Paz Fernandez, Harvard Medical School, United States
Copyright © 2018 Lanzo, Safratowich, Kudumala, Gallotta, Zampi, Di Schiavi and Carvelli. This is an open-access article distributed under the terms of the Creative Commons Attribution License (CC BY). The use, distribution or reproduction in other forums is permitted, provided the original author(s) and the copyright owner are credited and that the original publication in this journal is cited, in accordance with accepted academic practice. No use, distribution or reproduction is permitted which does not comply with these terms.
*Correspondence: Elia Di Schiavi, ZWxpYS5kaXNjaGlhdmlAaWJici5jbnIuaXQ= Lucia Carvelli, bGNhcnZlbGxpQGZhdS5lZHU=